- 1The Ritchie Centre, Hudson Institute of Medical Research, Melbourne, VIC, Australia
- 2Department of Obstetrics and Gynaecology, Monash University, Melbourne, VIC, Australia
- 3Department of Medicine, Monash University, Melbourne, VIC, Australia
- 4Department of Physiology, Anatomy and Microbiology, La Trobe University, Melbourne, VIC, Australia
- 5Australian Regenerative Medicine Institute, Monash University, Melbourne, VIC, Australia
Stroke is the second leading cause of death and physical disability, with a global lifetime incidence rate of 1 in 6. Currently, the only FDA approved treatment for ischemic stroke is the administration of tissue plasminogen activator (tPA). Stem cell clinical trials for stroke have been underway for close to two decades, with data suggesting that cell therapies are safe, feasible, and potentially efficacious. However, clinical trials for stroke account for <1% of all stem cell trials. Nevertheless, the resources devoted to clinical research to identify new treatments for stroke is still significant (53–64 million US$, Phase 1–4). Notably, a quarter of cell therapy clinical trials for stroke have been withdrawn (15.2%) or terminated (6.8%) to date. This review discusses the bottlenecks in delivering a successful cell therapy for stroke, and the cost-to-benefit ratio necessary to justify these expensive trials. Further, this review will critically assess the currently available data from completed stroke trials, the importance of standardization in outcome reporting, and the role of industry-led research in the development of cell therapies for stroke.
Introduction
Background
Stroke has a devastating effect on the society worldwide. In addition to its significant mortality rate of 50% as reported in 5-year survival studies (1), it affects as many as 1 in 6 people in their lifetimes, and is the leading cause of disability worldwide (2). A stroke results in a complex interplay of inflammation and repair with effects on neural, vascular, and connective tissue in and around the affected areas of the brain (3). Therefore, sequelae of stroke such as paralysis, chronic pain, and seizures can persist long term and prevent the patient from fully reintegrating into society. Stroke therefore remains the costliest healthcare burden as a whole (4). In 2012, the total cost of stroke in Australia was estimated to be about $5 billion with direct health care costs attributing to $881 million of the total (5).
Unfortunately, treatment options for stroke are still greatly limited. Intravenous recombinant tissue plasminogen activator (tPA) and endovascular thrombectomy (EVT) are currently the only effective treatments available for acute stroke. However, there is only a brief window of opportunity where they can be successfully applied. EVT is performed until up to 24 h of stroke onset (6), while tPA is applied within 4.5 h of stroke onset. Notably, the recent WAKE-UP (NCT01525290) (7) and EXTEND (NCT01580839) trials have shown that this therapeutic window can be safely extended to 9 h from stroke onset. Furthermore, advancements in acute stroke care and neurorehabilitation have shown to be effective in improving neurological function (8). However, there are no treatments that offer restoration of function and as a result, many patients are left with residual deficits following a stroke. Cell-based therapies have shown promising results in animal models addressing the recovery phase following stroke (9). This is encouraging as currently, there are no approved treatment options addressing the reversal of neurological damages once a stroke has occurred (10).
The majority of data from animal studies and clinical trials demonstrate the therapeutic potential of stem cells in the restoration of central nervous system (CNS) function (11, 12), applicable to neurodegenerative diseases as well as traumatic brain injury. Transplanted stem cells were reportedly able to differentiate into neurons and glial cells, whilst supporting neural reconstruction and angiogenesis in the ischemic region of the brain (13). Previous work demonstrated the ability of mesenchymal stem cells (MSCs) to differentiate into neurons, astrocytes (14), endothelial cells (15, 16), and oligodendrocyte lineage cells (17) such as NG2-positive cells (18) in vitro, and undergo neuronal or glial differentiation in vivo (19). Bone marrow-derived mesenchymal stem cells (BMSCs) have shown potential to differentiate into endothelial cells in vitro (20). Additionally, both BMSCs and adipose stem cells (ASCs) have been shown to demonstrate neural lineage differentiation potential in vitro (21–23). Furthermore, stem cells are able to modulate multiple cell signaling pathways involved in endogenous neurogenesis, angiogenesis, immune modulation and neural plasticity, sometimes in addition to cell replacement (3). The delivery of stem cells from the brain, bone marrow, umbilical cord, and adipose tissue, have been reported to reduce infarct size and improve functional outcomes regardless of tissue source (9). While these were initially exciting reports, they raise the question as to the validity of the findings to date since these preclinical reports are almost uniformly positive. The absence of scientific skepticism and robust debate may in fact have negated progress in this field.
Cell-based therapies have been investigated as a clinical option since the 1990s. The first pilot stroke studies in 2005 investigated the safety of intracranial delivery of stem cells (including porcine neural stem cells) to patients with chronic basal ganglia infarcts or subcortical motor strokes (24, 25). However, since the publication of these reports, hundreds of preclinical studies have shown that a variety of cell types including those derived from non-neural tissues can enhance structural and functional recovery in stroke. Cell therapy trials, mainly targeted at small cohorts of patients with chronic stroke, completed in the 2000s, showed satisfactory safety profiles and suggestions of efficacy (10). Current treatments such as tPA and EVT only have a narrow therapeutic window, limited efficacy in severe stroke and may be accompanied by severe side effects. Specifically, the side effects of EVT include intracranial hemorrhage, vessel dissection, emboli to new vascular territories, and vasospasm (26). The benefit of tPA for patients with a severe stroke with a large artery occlusion can vary significantly (27). This is mainly due to the failure (<30%) of early recanalisation of the occlusion. Thus, despite the treatment options stroke is still a major cause of mortality and morbidity, and there is need for new and improved therapies.
Stem cells have been postulated to significantly extend the period of intervention and target subacute as well as the chronic phase of stroke. Numerous neurological disorders such as Parkinson's disease (12, 28), Alzheimer's disease (29), age-related macular degeneration (30), traumatic brain injury (31), and malignant gliomas (32) have been investigated for the applicability of stem cell therapy. These studies have partly influenced the investigation of stem cell therapies for stroke. A small fraction of stem cell research has been successfully translated to clinical trials. As detailed in Table 1, most currently active trials use neuronal stem cells (NSCs), MSCs or BMSCs (35–37), including conditionally immortalized neural stem-cell line (CTX-DP) CTX0E03 (38), neural stem/progenitor cells (NSCs/NPSCs) (e.g., NCT03296618), umbilical cord blood (CoBis2, NCT03004976), adipose (NCT02813512), or amnion epithelial cells (hAECs, ACTRN 1261800076279) (39).
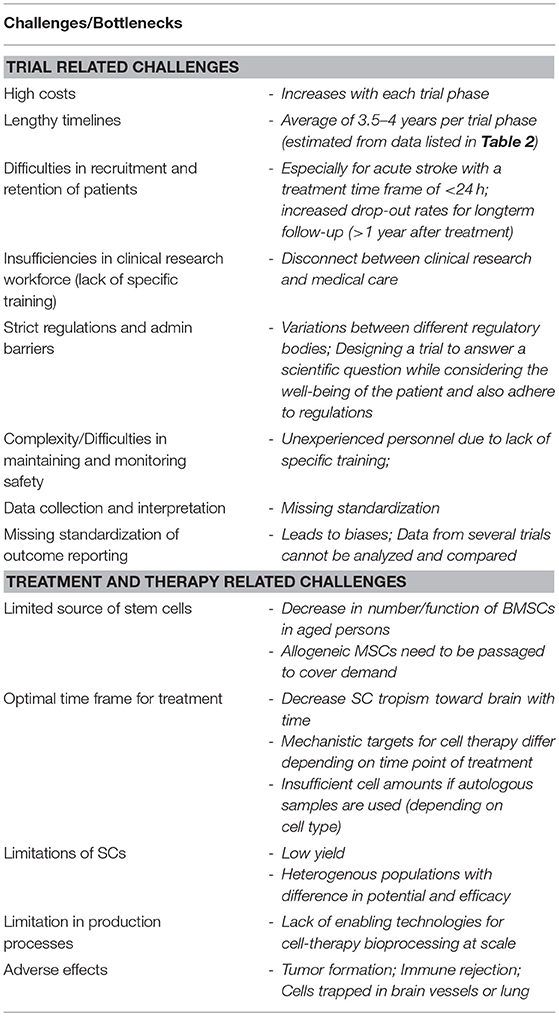
Table 1. Challenges and bottlenecks of stem cell therapy and clinical trials using stem cells (33, 34).
Bottlenecks and Challenges of Cell Therapies
The development of a cell therapy for stroke is challenging for a number of reasons and these are detailed in Table 1. Each cell type requires testing for safety and efficacy to mitigate risks such as tumor formation. Identifying the ideal cell type for stroke has been hampered by the lack of data around clinical efficacy, as well as by the complex logistics and ethical concerns. The latter being a great hurdle for the use of fetal and embryonic stem cells in particular. The mechanisms of action of each cell type (i.e., cell replacement, growth factor secretion, and/or sequestration of inflammation) must be considered when choosing the appropriate route and timing of administration as these can directly influence treatment efficacy.
A critical translational consideration for stroke is the identification of the optimal route of administration. Different routes have been used in animal models for the transplantation of stem cells, including intracerebral (40), intracranial (41, 42), intranasal (43) or via stereotaxic infusion (44), and it is worth noting that all of these studies reported improvement in functional outcomes. Several different routes have been used in clinical trials (see Table 2); where the most common routes are intravenous infusion and intracerebral transplant. While different routes of administration have been compared in several reviews (58, 59), the optimal route has yet to be defined. Nevertheless, there is no evidence that a specific route of administration has significant effect on clinical efficacy (60–63).
Multiple factors influence the efficacy of cell transplantation and treatment outcomes, and these considerations may be specific to the cell type. Therefore, thorough investigation must be undertaken in order to develop the most effective and safest combination of cell type, dosage, route of administration and timing of delivery (10, 64). Stroke type and hence infarct size and location need to be considered to enable targeted treatment. The choice of cell type and delivery method will enable the homing of the cells to the site of injury and the level of efficacy that can be achieved. Furthermore, there are additional considerations if the cell therapy is administered to treat a subacute or a chronic stroke since the blood brain barrier will be less permeable compared to the acute stages of stroke (65). In these instances, the cell delivery would have to be intrathecal. This approach is more invasive and would require the patient to stop anti-thrombotic medication, thereby risking the recurrence of recurrence during this period.
In addition to the clinical challenges to applying a cell therapy for stroke, there are the manufacturing challenges. There are limited enabling technologies for manufacturing cells at a commercial scale (33). This process must be developed for each cell type, and optimized for the production of a high yielding, quality product. Development of cell manufacturing processes is exhaustive, expensive, and time-consuming. Even when the processes is optimized, the use of autologous BMSCs can be limited by the fact that they require in vitro expansion for a week or longer to obtain a therapeutic dose of cells (53). This eliminates the possibility of autologous treatment within a few hours of stroke onset. This might be the reason that most of the active trials to date have focused on allogeneic cell therapies as detailed in Table 2. Many cell lines are additionally immortalized and/or otherwise modified (e.g., MASTERS trial, ACTIsSIMA trial; see section Importance of Standardization in Outcome Reporting). This eliminates the variability in yield and potency as would be the case with autologous cell lines and is amenable to a streamlined production process with predetermined product quality and yield.
For successful trials reaching a phase where patients will be recruited at multiple sites on an international scale another challenge arises. The regulatory frameworks and authorities differ between countries. For example in the US clinical trials are regulated by the Food and Drug Administration (FDA) whereas in Australia Therapeutic Goods Administration (TGA) and in the UK it is The Medicines and Healthcare Products Regulatory Agency (MHRA). Their authorities, tasks and processing times differ from country to country. In an attempt to support the planning and implementation of international clinical research the NIH offers an online database, ClinRegs (https://clinregs.niaid.nih.gov), which compares the country-specific research regulatory information between 20 different countries (e.g., US, Canada, UK, China, India, Australia, and South Africa). Commonly discussed challenges for sponsors of multiregional trials are planning and trial design, data recording and analysis (statistics), clinical (medical standards of care, access to care, and qualification of personel), regulatory operational, and ethical practices (66). Important points to consider such as differences in patient populations, efficacy, clinical investigator sites was recently summarized by Shenoy (67). The review gives examples with a focus on China and the United States.
In current agreement trials follow the principles of Good Clinical Practice (GCP). These have their origin in the World Medical Association's Declaration of Helsinki, 1964 and were used as a basis for the guidelines published by the International Council for Harmonization (www.ich.org) in 1996. These guidelines have been adopted by several regulatory agencies from different countries. They are recently being updated (67).
Cell Therapy Clinical Trials for Stroke
Several reports which have set out to analyze the outcomes of different cell therapy clinical trials for stroke have pointed out the risk for biases (3, 68, 69). The most commonly found was attrition bias (incomplete outcome data), reporting bias (selective reporting of results), and selection bias (random sequence generation, allocation concealment bias). There is, therefore, a need for updated guidelines and the implementation of standardization of recording and reporting data from cell therapy trials for stroke and likely, other conditions. The formation of clinical trial networks attempts to address some of the challenges of running a clinical trial. It offers support with trial coordination in general, site and data management, statistical analysis, patient recruitment in particular (70). This may especially benefit investigator-initiated trials where the trial team has limited experience with cell-based therapies.
To date, several pre-clinical and clinical trials indicate that cell-based therapies are generally safe, however the mechanisms through which the cells exert their therapeutic efficacy requires further investigation (25, 35, 36, 38, 44, 52, 53, 71). Agreeable safety profiles with functional improvements in patients with stroke have been reported for example after transplantation of neuronal cells differentiated from a teratocarcinoma cell line (24), immortalized human neural stem cell (38), transformed allogeneic BMSCs (44), and autologous BMSCs (72). The guidelines on the development of cell therapies for stroke, Stem Cell as an Emerging Paradigm in Stroke (STEPS) (73–75) (see Chapter 4 for more details on STEPS) outlined the need for long-term safety testing when the cells used are highly proliferative and easily differentiate. In a follow-up study from a trial published in 2005 (76). Lee et al. (71) analyzed long-term safety in an open-label, placebo controlled trial with 85 patients who suffered from ischemic stroke within the last 90 days (both trials have no NCT number). 5 × 107 autologous BMSCs were administered intravenously twice; 4 and 6 weeks after bone marrow aspiration. Patients were followed up for 5 years, and it was found that the SC transplant was safe. Another trial by Fang et al. (77) followed up their patients for 4 years (NCT01468064). This trial was a two-center, randomized, placebo-controlled phase I/IIa trial treating 18 patients suffering from acute cerebral infarct within 7 days of stroke onset. 5 × 106 cells/kg body weight BMSCs or endothelial progenitor cells (EPCs) were administered intravenously in 2 doses 4 and 5 weeks after bone marrow aspiration. This study also found that the treatment was safe. Long-term studies are currently still the exception, and more data is needed to understand if safety can be guaranteed for every cell type used in a potential therapy.
Most results reported up to date, demonstrate safety, but do not show sufficient data for clinical efficacy. The trial mentioned above by Fang et al. (77) for example reported no significant improvement in functional outcome. Recently, adjunctive therapies have been discussed to be a way to tackle low efficacy. The combination of a stem cell therapy with a drug that is able to improve neurogenesis and angiogenesis and/or reduce inflammation and hence working along the same pathways as stem cells do, could amplify efficacy. Several drugs with such biological activity have been already identified (78). For example, for the treatment of stroke G-CSF (granulocyte-colony stimulating factor) has been proposed as an adjunct therapy to stem cell treatment of human umbilical cord blood cells (79). Further preclinical studies are required for the translation of combinatorial therapies into the clinic.
A successful stem cell therapy for stroke must be safe, effective, applicable to a broad spectrum of stroke patients, and economically viable. Current trials differ in cell type, route, dose, and time of administration, as well as patient recruitment criteria. However, independent of the heterogeneity between trials, the most noteworthy adverse events such as seizures, headaches, and administration procedure-related events have been similar.
Patient selection is a critical component in reducing heterogeneity within any given trial cohort, however, this is particularly the case for stroke which has a heterogeneous clinical presentation. As such, an investigator may wish to exclude patients with certain comorbidities, and this should be incorporated into the trial design in order to limit heterogeneity and more accurately report on efficacy. Given that biological markers of stroke recovery are currently unavailable, the methods for ascertaining clinical improvement must be carefully chosen in order to provide meaningful data. The following section describes three industry-sponsored and two investigator-initiated cell therapy clinical trials as examples of trial design, execution and evaluation.
MASTERS and Treasure Trials
The Athersys Inc. funded MASTERS clinical trial (NCT01436487) was a phase 2, randomized, double-blinded, placebo controlled, dose-escalation trial, using allogeneic, bone marrow-derived, multipotent adult progenitor cells (MultiStem) (52). The MASTERS trial concluded in 2015, after treating 126 patients (134 enrolled) diagnosed with moderate to moderate-severe ischemic stroke (53). Patients were divided into three treatment groups: 1. Treatment 24–36 h after stroke onset with 400 million cells or placebo, 2. Treatment 24–36 h after stroke onset with 1.2 billion cells or placebo, and 3. Treatment 24–48 h after stroke onset with 1.2 billion cells or placebo. Sixty-five patients received cells and 61 patients received the placebo. No dose-limiting toxicity events or treatment-emergent adverse events (TEAE) were recorded. The investigators concluded that intravenously administered MultiStem was safe and well-tolerated, even at the higher dose. While changes in pro-inflammatory cytokines were noted, there was significant clinical improvement (at 90 days: mRS ≤ 2, Barthel Index ≥95, NIHSS ≥75% improvement). A major learning from the MASTERS trial is perhaps that of the logistics around cell manufacturing and provision of a living biologic within a relatively short treatment window. The MASTERS trial was initially designed with treatment within 24–36 h. However, the investigators ultimately changed their protocol to include a cohort at 24–48 h due to the logistical challenges in delivering cell products within the original timeframe (53). Upon conclusion of the MASTERS trial, the investigators concluded that the timing of the treatment is absolutely crucial, such that clinical efficacy was lost within the 36–48 h time window, thereby supporting an earlier intervention (53).
Indeed, this concept of an earlier intervention is currently being investigated by Athersys in the MASTERS-2 (NCT03545607) trial which commenced recruitment in 2018. The MASTERS-2 trial is a phase 3 quadruple-blind, randomized control trial to study the safety and efficacy of MultiStem, in patients suffering from acute ischemic stroke. The treatment (1.2 billion cells) are administered intravenously within 18–36 h of stroke onset. In addition to the MASTERS-2 trial, a different sponsor, Helios, is currently running a placebo controlled, multicentre phase 2/3 trial, TREASURE (NCT02961504) (80) where patients are recruited exclusively from Japan. The TREASURE trial also administers the cells intravenously within 18–36 h of stroke onset where MultiStem is administered at the same dose of 1.2 billion. Both trials are currently recruiting as of the preparation of this review and are estimated to conclude in 2020.
ACTIsSIMA Trial
The ACTIsSIMA trial is a SanBio sponsored Phase 2 double-blinded, sham-surgery controlled trial using allogeneic BMSCs transfected with a plasmid coding for a Notch I domain (SB623, NCT02448641). The SB623 cells are administered via stereotactic, intracranial injection to eligible patients suffering from chronic ischemic stroke. Preclinical studies indicate that these gene-edited allogeneic BMSCs surpassed the outcome of unmanipulated BMSCs in rodent stroke models (44) and were tested in a phase I/II dose escalation trial (NCT01287936) (36, 81). The open-label phase 1/2a safety trial enrolled 18 patients having suffered from a subcortical stroke within the past 6–60 months. Doses of 2.5, 5, or 10 million SB263 cells were administered via a stereotactic placement within the margin of the site of the infarct. The only TEAE recorded were related to the procedure, rather than the cells. Of the 18 recruited patients, 16 have completed the 12 months follow-up. Significant improvements were recorded in this study—European Stroke Scale: mean increase 6.88; National Institutes of Health Stroke Scale (NIHSS): mean decrease 2.00; Fugl-Meyer total score: mean increase 19.20; Fugl-Meyer motor function total score: mean increase 11.40. No changes on the modified Rankin Scale (mRS) were recorded (36, 51). Based on the conclusion that SB623 cells were safe and associated with an improvement in clinical outcome, the Phase 2 ACTIsSIMA trial commenced in 2016 where two cohorts of patients received either a dose of SB623 cells at 2.5 or 5 million cells, or a sham placebo will be randomized in a 1:1:1 ratio. The trial is expected to conclude in 2019.
Pisces Trial
Another industry sponsored trial to show promise in the cell therapy space for stroke is the ReNeuron sponsored trial investigating the potential of genetically modified human fetal cortical neuroepithelial cells for stroke (82). This cell therapy product is genetically modified using a retro-viral insertion of the modified growth factor c-mycERTAM (CTX0E03 DP) which overcomes the manufacturing problem of slow growing MSC through the transient expression of c-myc using a tamoxifen-estrogen receptor system (38, 81). The Pilot Investigation of Stem Cells in Stroke Trial (PISCES) was a Phase 1/2 open-label, dose-escalation safety trial (NCT01151124). The trial was based on preclinical studies in rats. Specifically, the injection of CTX0E03 DP was tested in a rat model of stroke induced by middle cerebral artery obstruction, where 450,000, 45,000 or 4,500 CTX0E03 DP were injected 4 weeks after MCOA (middle cerebral artery occlusion). Functional outcomes were assessed 2 weeks after cell implantation. Notably, significant functional improvement was only noted at the highest dose (41) and only when cells were delivered via an intraparenchymal injection, but not when delivered via intracerebroventricular injection which failed to achieve graft survival or functional improvement (42). Positive outcomes were associated with endogenous neurogenesis (83) and angiogenesis (84).
In PISCES, 11 patients suffering from ischemic stroke received a stereotactic ipsilateral putamen injection of CTX0E03 DP 6–60 months after stroke onset. Doses of 2, 5, 10, or 20 million cells were administered. As was the case for the MASTERS trial, the investigators struggled with the logistics of cell therapies and were able to treat only two patients at the highest dose. Safety was assessed over a 2-year period and no TEAE were recorded. Overall, the investigators concluded that this cell therapy is feasible and safe. Following this study PISCES II was launched in 2014 (NCT02117635). PISCES II is a phase 2a, open-label, safety trial where 20 million CTX0E03 DP cells were administered intracranially via stereotaxic neurosurgery. As of the preparation of this review, 23 patients have been recruited to PISCES II and received CTX0E03 DP cells. Results to date are available on the ReNeuron website (www.reneuron.com) but not compiled in a peer-reviewed publication. The 12-month follow-up showed an improvement in mRS and Barthel Index in 50 and 41% of enrolled patients, respectively. It was concluded that the treatment is safe and feasible, and a placebo-controlled, randomized phase 2b trial was started in 2018—PISCES III (NCT03629275)—where a larger number of patients will be recruited (110) and is estimated to conclude by late 2019. No further detailed analysis of the trial data is currently available.
I-ACT Trial
The I-ACT trial is an investigator-initiated single site trial. I-ACT is a open-label, dose escalation safety phase 1 study (39). Patients will receive an intravenous infusion of 2, 4, 8, 16, and 32 million cells per kg body weight of allogeneic placenta-derived hAECs within 24 h of stroke onset. The study is based on preclinical data in several mouse and marmoset models of cerebral ischemia demonstrating neuroprotection and the facilitation of mechanisms of repair and recovery (85). The trial is estimated to conclude mid 2020 including a 1-year follow up.
CoBIS2 Trial
The CoBIS2 trial is a continuation of CoBIS1. CoBIS1 was an investigator-initiated, multicentre, open-label, phase 1 safety study where 10 patients were recruited (37, 55, 86, 87). Allogeneic umbilical cord blood (UCB) containing 0.5–50 million total nucleated cells per kg bodyweight was administered intravenously 3–10 days post-stroke onset. Patients showed functional improvement 3 months past stroke onset. Fifty percent of patients showed improvement of one grade of mRS (mean mRS of 2.8 ± 0.9). NIHSS improved by at least 4 points (mean 5.9 ± 1.4). All patients demonstrated improvement in activities of daily living (Barthel Index mean increase 52.0 ± 24.7). The results conclude that the treatment is safe, feasible and suggestive of functional improvement. Based on these outcomes CoBIS2 was initiated. CoBIS2 is a multicenter, placebo controlled, randomized, double blinded, phase 2 study. CoBIS2 plans to recruit 100 participants. Doses of UCB and time frame of treatment remained the same. The trial is expected to conclude in 2020.
As can be seen from the trials above, the choice of route of administration is either intracranial or intravenous. And while some trials deliver a cell dosage based on bodyweight (I-ACT and CoBIS2), others deliver a fixed dose of cells. The completed trials preceding these current trials have provided clear results on treatment safety, but clinical efficacy remains uncertain. The MASTERS trial (52, 53), the InVeSt trial (44) and another phase 1 trial administering autologous BMSCs (88), did not report significant functional improvement. The PISCES trial however, linked improved neurological function in patients with chronic stroke to the treatment with genetically modified, immortalized human NSCs (38). This is certainly encouraging despite the absence of significant efficacy. Furthermore, the treatment in some cases (e.g., MASTERS trial) was linked with lower rates of mortality and TEAE (52).
Importance of Standardization in Outcome Reporting
In order to assess the overall potential and combined outcomes of stem cell therapy for stroke, it is important to assess the outcomes across the various completed trials. The need for quality standards and documentation of study outcomes for pre-clinical and clinical stem cell research in stroke was identified a decade ago. In 2009, experts from academia and industry, members of the National Institute of Health (NIH) and the Food and Drug Administration (FDA) published their first meeting report, Stem Cell as an Emerging Paradigm in Stroke (STEPS) as a consensus-based guideline on the development of cell therapies for stroke, with a focus on the translation of pre-clinical studies and the design and conduct of early- and late-stage clinical trials for acute and chronic stroke (73). These guidelines have since been updated in 2011 (74) and 2014 (75).
Many of the problems identified in this exercise were also reported in several meta-analyses, in particular the lack of consistent reporting of safety and efficacy data for combinatorial (89) or mono-therapies (3), as well as heterogeneity in study design [e.g., single-arm (90)], a cell type [e.g., MSCs, (91)] or a stroke type [ischemic (69)]. Recently, Nagpal et al. (3) compared several different early-phase cell therapy trials while disregarding the cell type, treatment administration or study design differences. Overall, they concluded that the administration of different types of stem cells was feasible and safe. None of the adverse events reported could be ascertained to be related to the respective cell therapies. Nevertheless, additional research is still needed in order to demonstrate efficacy and enable market approval. As full recovery is unlikely, the outcome of any given stroke trial is dependent on the estimation of functional neurological improvement and structural recovery. Nagpal et al. drew conclusions based on changes to the Barthell index, the modified Rankin scale, and NIH Stroke Scale values across different trials, where despite indications of improvement, the magnitude of impact was small. Currently, it is impossible to draw conclusions with regard to optimal treatment protocols due to the limited data available from a small number of clinical studies comprised of small cohorts in a notoriously heterogeneous disease.
Cost-to-Benefit of Cell Therapy Trials
More than 6,000 trials are registered with www.clinicaltrials.gov employing different types of stem cells, addressing different types of disorders and/or diseases (excluding unknown status trials). Currently, 41.2% of these stem cell clinical trials are active and 44.5% are completed. And of the registered stem cell trials, only 0.73% address stroke, with 38.6% being completed, and 27.3% currently active. Interestingly, 25% of stroke trials have been withdrawn and another 9.1% were terminated or suspended. See Figure 1 for details. Most trials were withdrawn or terminated due to low recruitment rates or lack or termination of funding. Strikingly, all completed trials are phase 1 and 2 trials, with only 1 in 5 trials moving on to the next phase. Of the 13 currently active trials only two trials have reached phase 3. These are the MASTERS-2 trial (Athersys Inc.) investigating a stem cell treatment for adults who have suffered an acute ischemic stroke, and the STARTING-2 trial (Samsung Medical Center, Korea) determining the efficacy of intravenous transplantation of autologous MSCs to treat acute ischemic stroke. On average clinical trials took 3.3 years from start to completion. From the data collected (see Table 2), one can estimate that a cell therapy for stroke will take >10 years to progress from phase 1 to phase 3 without considering any preceding preclinical research or process development.
Given this extremely long “gestation” for translating cell therapies for stroke, it is important to also consider the cost-to-benefit ratio. The US Department of Health & Human Services published a comprehensive report on clinical trial costs as part of their analysis of current barriers for drug development (92). The top three cost drivers of clinical trial expenditures were clinical procedure costs (15–22% of total), administrative staff costs (11–29% of total), and site monitoring costs (9–14% of total). Generally, costs increase with every trial phase: phase 1 on average being about US$ 4 million, phase 2 about US$ 13 million and phase 3 and 4 about US$ 20 million each. This totals to an average of US$ 57 million to take a therapeutic through its clinical trial stages. The costs of a trial depend largely on the therapeutic area being targeted. The most expensive clinical trials (phase 1–3) focus on pain and anesthesia US$ 71.3 million, ophthalmology US$ 49.8 million, and anti-infectives US$ 41.2 million. Trials on treatments focused on the CNS are estimated to cost US$ 37 million, and US$ 34.4 million on cardiovascular diseases. In order to understand if the costs of a trial are economically justifiable, one has to consider the financial costs that stroke has on a society, which was analyzed on an international level in 2004 (93). This study was done over a decade ago, and an update would certainly be necessary as the prevalence of stroke has continued to rise. In 2012, the total cost of stroke to Australia was estimated at AU$ 5 billion with health cost being AU$ 881 million and productivity cost being AU$ 3 billion (5). The costs of stroke to the UK health and social services in the same year were estimated at AU$ 5.2 billion (£ 2.9 billion) (94). Efficacious stroke interventions are expected to significantly reduce the financial costs. However, this does not take into consideration the costs of stem cell-based therapies. This is despite attempts to assess the cost and benefits of medical research since the 1990's (95), with analysis of funds going into specific areas of medical research (e.g., stroke, cancer, and dementia) (94). Information on the actual costs of clinical trials in general or even specific clinical trials is scarce.
In 2017 the Australian Government published an economic evaluation of 25 clinical trials to assess the overall health and economic impact of investigator-initiated clinical trials (70). This report only included independent investigator-initiated trials that were part of clinical trial networks in Australia, in phase 2 and beyond. Included in this report were 7 trials conducted within the Australasian Stroke Trials Network between 2004 and 2014. The combined costs of 4 trials was estimated to be AU$ 32 million (excluding early phase trials, pilot and feasibility trials and observation studies). The gross economic benefit of these trials was estimated at AU$ 327 million. Thus, the benefit for these late-phase trials was estimated to be AU$ 10 per AU$ 1 invested. The report estimated a benefit of AU$ 5 for every AU$ 1 invested with a total gross benefit of AU$ 2 billion for all 25 trials analyzed, with a cumulative reduction in health service costs in the order of AU$ 580 million. Since none of the trials included in the report used any kind of cell therapy, an estimation of the benefit of cell-therapy clinical trials could not be made. However, it is clear that clinical trials are quantifiably beneficial and while the analysis covered a decade's worth of trials, it did not include any data from the early stage trial counterparts. This further emphasizes the length of time required for clinical development of any new treatment for stroke before clinical benefit is seen, let alone to see profit.
In 2018, the costs of producing for autologous cell therapies have been estimated to be US$ 94 per million cells (96). For a dose of 2 million cells per kg, assuming that a patient weighs 70 kg, the costs would be US$ 13,160 per dose. The costs of drug development influences the pricing of any clinical therapy, but several factors can also contribute to the cost, such as market size, competing products, the quality-adjusted life year (QALY), and what the consumer is willing to pay (97, 98). At the moment, there is no approved stem cell therapy for stroke. Stem cell therapies approved by the FDA include cord blood and a small number of cell lines (e.g., modified T-cells, chondrocytes, and fibroblasts). There are three different approved stem cell therapies on the market. The European commission approved a stem cell product, Alofisel (Takeda) in 2018, however FDA approval is still pending at the time of the writing of this review. Alofisel is an ASC treatment for perineal fistulas in patients with Crohn's disease. One course of treatment is US$ 61,000 (99). Another approved product is Holoclar (Holostem Terapie Avanzante), which was the first stem cell therapy to receive market authorization in the EU. Holoclar is comprised of corneal epithelial cells used to treat chemical burns of the eye, costing US$ 102,000 (99). TEMCELL (JCR Pharmaceuticals) is approved in Japan for the use of BMSCs to treat Graft vs. Host Disease, costing about US$ 170,000 per course (100). The price tags on these approved therapies can only hint at expected costs of cell therapies for stroke. Furthermore, every country has their own health care board deciding if a treatment would be covered by the national health care system, thus making it impossible to estimate the actual out-of-pocket costs a patient would incur. It is evident that a treatment which improves functional outcomes in stroke, as can be seen from other recent interventions, will have a significant benefit on health costs (5).
The Role of Industry-Led Research in the Development of Cell Therapies for Stroke
When assessing the success of clinical trials there is always the question if industry-led research is more successful than academic research. This has been critically analyzed and reviewed over the last decade as scientists and public are aware of the inherent risk of biases in industry funded studies (101–104). Recently, Lundh et al. (105) compared studies with and without industry funding from 75 papers on reported efficacy, conclusions and risk of bias. The papers selected were on primary research studies, empirical studies and randomized clinical trials (58 papers) focusing on drug and device development, but without a focus on a specific disease or type of therapy. Industry funded projects were found to demonstrate favorable efficacy toward tested treatments, with less substantive conclusions. Over 60% of published findings from cell therapy clinical trials reported positive outcomes, with a trend toward a higher proportion of positive reports from industry funded trials (106). Expectedly, the more complete and detailed studies were published in higher impact factor journals where data are presumably subjected to a higher level of scrutiny.
Reporting according to CONSORT (107) or also SPIRIT (Standard Protocol Items: Recommendations for Interventional Trials) (108) identifies funding sources and possible conflicts of interest but are not without limits compared to more recent recommendations by Hakoum et al. (109) that specifically pay address the characteristics of funding of clinical trials. External influences exerted on research and clinical trials are difficult to trace and often remain unclear when the results are published at the end of a trial (104). Currently about 28.5% of all active, interventional clinical trials registered with www.clinicaltrials.gov are industry funded. In relation to current active, interventional trials focused on stem cell therapies for stroke, 66.7% are industry funded (Table 2). Late-stage trials (phase 2 and 3) are predominantly industry funded (58.3%) whereas early-stage trials (phase 1 and 1/2) are funded by other means (62.5%). This might be due to the fact that the later phases of clinical testing incur larger costs that are beyond the funding quanta of philanthropic or government bodies, or that promising late-stage trials may be of greater interest to industry.
Conclusion
It is clear that there are challenges in the use of cell therapies for stroke (Table 1) that remain to be addressed in the future. A major issue certainly is the need for standardized outcome reporting that is free of bias and enables comparison of different trials. Furthermore, optimized and more efficient bioprocesses need to be urgently developed to reduce the cost of production and in doing so, treatment costs. Most studies showed safety and feasibility for cell therapy for stroke independent of cell type and route of administration. However, there remains limited proof of efficacy. We and others will be watching closely for the outcomes of current stroke clinical trials utilizing cell therapies, as we await the evidence for clinical efficacy and impactful functional improvement that is desperately needed to spur this field ahead.
Author Contributions
MK and RL drafted the manuscript. MK prepared all figures and tables. All authors provided input according to their expertise and discussed the manuscript.
Funding
This work was supported by the NHMRC CDF GNT1159277 (personal grant to RL) and the GNT1159277.
Conflict of Interest Statement
The authors declare that the research was conducted in the absence of any commercial or financial relationships that could be construed as a potential conflict of interest.
References
2. Martínez-Garza D, Cantú-Rodríguez O, Jaime-Pérez J, Gutiérrez-Aguirre C, Góngora-Rivera J, Gómez-Almaguer D. Current state and perspectives of stem cell therapy for stroke. Med Universit. (2016) 18:169–80. doi: 10.1016/j.rmu.2016.07.005
3. Nagpal A, Choy FC, Howell S, Hillier S, Chan F, Hamilton-Bruce MA, et al. Safety and effectiveness of stem cell therapies in early-phase clinical trials in stroke: a systematic review and meta-analysis. Stem Cell Res Ther. (2017) 8:191. doi: 10.1186/s13287-017-0643-x
4. Teasell RW. Long-term sequelae of stroke: How should you handle stroke complications? Can Fam Physician. (1992) 38:381–8.
5. Foundation S. The Economic Impact of Stroke in Australia. (2013). Available online at: https://www2.deloitte.com/au/en/pages/economics/articles/economic-impact-stroke-australia.html (accessed April 30, 2019).
6. Nogueira RG, Jadhav AP, Haussen DC, Bonafe A, Budzik RF, Bhuva P, et al. Thrombectomy 6 to 24 hours after stroke with a mismatch between deficit and infarct. N Engl J Med. (2018) 378:11–21. doi: 10.1056/NEJMoa1706442
7. Thomalla G, Simonsen CZ, Boutitie F, Andersen G, Berthezene Y, Cheng B, et al. MRI-guided thrombolysis for stroke with unknown time of onset. N Engl J Med. (2018) 379:611–22. doi: 10.1056/NEJMoa1804355
8. Bushnell C, McCullough LD, Awad IA, Chireau MV, Fedder WN, Furie KL, et al. Guidelines for the prevention of stroke in women: a statement for healthcare professionals from the American Heart Association/American Stroke Association. Stroke. (2014) 45:1545–88. doi: 10.1161/01.str.0000442009.06663.48
9. Savitz SI, Dinsmore JH, Wechsler LR, Rosenbaum DM, Caplan LR. Cell therapy for stroke. NeuroRx. (2004) 1:406–14. doi: 10.1602/neurorx.1.4.406
10. Kenmuir CL, Wechsler LR. Update on cell therapy for stroke. Stroke Vasc Neurol. (2017) 2:59–64. doi: 10.1136/svn-2017-000070
11. Lindvall O, Kokaia Z. Stem cells for the treatment of neurological disorders. Nature. (2006) 441:1094. doi: 10.1038/nature04960
12. Song CGY, Zhang ZH, Wu NX, Cao LC, Guo JY, Li QM, et al. Stem cells: a promising candidate to treat neurological disorders. Neural Regener Res. (2018) 13:1294–304. doi: 10.4103/1673-5374.235085
13. Ryu SS, Lee H, Kim SU, Yoon BW. Human neural stem cells promote proliferation of endogenous neural stem cells and enhance angiogenesis in ischemic rat brain. Neural Regener Res. (2016) 11, 298–304. doi: 10.4103/1673-5374.177739
14. Wislet-Gendebien S, Bruyère F, Hans G, Leprince P, Moonen G, Rogister B. Nestin-positive mesenchymal stem cells favour the astroglial lineage in neural progenitors and stem cells by releasing active BMP4. BMC Neurosci. (2004) 5:33. doi: 10.1186/1471-2202-5-33
15. Hess DC, Hill WD, Martin-Studdard A, Carroll J, Brailer J, Carothers J. Bone marrow as a source of endothelial cells and NeuN-expressing cells after stroke. Stroke. (2002) 33:1362–8. doi: 10.1161/01.STR.0000014925.09415.C3
16. Portalska KJ, Leferink A, Groen N, Fernandes H, Moroni L, van Blitterswijk C, et al. Endothelial differentiation of mesenchymal stromal cells. PLoS ONE. (2012) 7:e46842. doi: 10.1371/journal.pone.0046842
17. Steffenhagen CF, Dechant X, Oberbauer E, Furtner T, Weidner N, Küry P, et al. Mesenchymal stem cells prime proliferating adult neural progenitors toward an oligodendrocyte fate. Stem Cells Develop. (2011) 21:1838–51. doi: 10.1089/scd.2011.0137
18. Shen LH, Li Y, Chen J, Zhang J, Vanguri P, Borneman J, et al. Intracarotid transplantation of bone marrow stromal cells increases axon-myelin remodeling after stroke. Neuroscience. (2006) 137:393–9. doi: 10.1016/j.neuroscience.2005.08.092
19. Guzman R, Choi R, Gera A, De Los Angeles A, Andres RH, Steinberg GK. Intravascular cell replacement therapy for stroke. Neurosurg Focus. (2008) 24:E15. doi: 10.3171/FOC/2008/24/3-4/E14
20. Wang C, Li Y, Yang M, Zou Y, Liu H, Liang Z, et al. Efficient differentiation of bone marrow mesenchymal stem cells into endothelial cells in vitro. Eur J Vasc Endovasc Surg. (2018) 55:257–65. doi: 10.1016/j.ejvs.2017.10.012
21. Ashjian PH, Elbarbary AS, Edmonds B, DeUgarte D, Zhu M, Zuk PA, et al. In vitro differentiation of human processed lipoaspirate cells into early neural progenitors. Plastic Reconstruct Surg. (2003) 111:1922–31. doi: 10.1097/01.PRS.0000055043.62589.05
22. Cho KJ, Trzaska KA, Greco SJ, McArdle J, Wang FS, Ye JH, et al. Neurons derived from human mesenchymal stem cells show synaptic transmission and can be induced to produce the neurotransmitter substance P by interleukin-1α. Stem Cells. (2005) 23:383–91. doi: 10.1634/stemcells.2004-0251
23. Wislet-Gendebien S, Hans G, Leprince P, Rigo JM, Moonen G, Rogister B. Plasticity of cultured mesenchymal stem cells: switch from nestin-positive to excitable neuron-like phenotype. Stem Cells. (2005) 23:392–402. doi: 10.1634/stemcells.2004-0149
24. Kondziolka D, Steinberg GK, Wechsler L, Meltzer CC, Elder E, Gebel J, et al. Neurotransplantation for patients with subcortical motor stroke: a phase 2 randomized trial. J Neurosurg. (2005) 103:38–45. doi: 10.3171/jns.2005.103.1.0038
25. Savitz SI, Dinsmore J, Wu J, Henderson GV, Stieg P, Caplan LR. Neurotransplantation of fetal porcine cells in patients with basal ganglia infarcts: a preliminary safety and feasibility study. Cerebrovasc Dis. (2005) 20:101–7. doi: 10.1159/000086518
26. Behme D, Gondecki L, Fiethen S, Kowoll A, Mpotsaris A, Weber W. Complications of mechanical thrombectomy for acute ischemic stroke—a retrospective single-center study of 176 consecutive cases. Neuroradiology. (2014) 56:467–76. doi: 10.1007/s00234-014-1352-0
27. Bhatia R, Hill MD, Shobha N, Menon B, Bal S, Kochar P, et al. Low rates of acute recanalization with intravenous recombinant tissue plasminogen activator in ischemic stroke: real-world experience and a call for action. Stroke. (2010) 41:2254–8. doi: 10.1161/STROKEAHA.110.592535
28. Gandhi V, Burle S, Kosalge S. Stem cell therapy for Parkinson's disease: a review. Pharma Tutor. (2018) 6:1–8. doi: 10.29161/PT.v6.i6.2018.1
29. Hunsberger JG, Rao M, Kurtzberg J, Bulte JW, Atala A, LaFerla FM, et al. Accelerating stem cell trials for Alzheimer's disease. Lancet Neurol. (2016) 15:219–30. doi: 10.1016/S1474-4422(15)00332-4
30. Singh MS, MacLaren RE. Stem cell treatment for age-related macular degeneration: the ChallengesStem Cells for AMD treatment. Investigat Ophthalmol Vis Sci. (2018) 59:AMD78–82. doi: 10.1167/iovs.18-24426
31. Weston NM, Sun D. The potential of stem cells in treatment of traumatic brain injury. Curr Neurol Neurosci Rep. (2018) 18:1. doi: 10.1007/s11910-018-0812-z
32. Zhang Q, Xiang W, Yi DY, Xue BZ, Wen WW, Abdelmaksoud A, et al. Current status and potential challenges of mesenchymal stem cell-based therapy for malignant gliomas. Stem Cell Res Ther. (2018) 9:228. doi: 10.1186/s13287-018-0977-z
33. Ratcliffe E, Thomas RJ, Williams DJ. Current understanding and challenges in bioprocessing of stem cell-based therapies for regenerative medicine. Br Med Bull. (2011) 100:137–55. doi: 10.1093/bmb/ldr037
34. Bang OY, Kim EH, Cha JM, Moon GJ. Adult stem cell therapy for stroke: challenges and progress. J Stroke. (2016) 18:256. doi: 10.5853/jos.2016.01263
35. Kondziolka D, Wechsler L, Goldstein S, Meltzer C, Thulborn K, Gebel J, et al. Transplantation of cultured human neuronal cells for patients with stroke. Neurology. (2000) 55:565–9. doi: 10.1212/WNL.55.4.565
36. Steinberg GK, Kondziolka D, Wechsler LR, Lunsford LD, Coburn ML, Billigen JB, et al. Clinical outcomes of transplanted modified bone marrow–derived mesenchymal stem cells in stroke: a phase 1/2a study. Stroke. (2016) 47:1817–24. doi: 10.1161/STROKEAHA.116.012995
37. Laskowitz DT, Bennett ER, Durham RJ, Volpi JJ, Wiese JR, Frankel M, et al. Allogeneic umbilical cord blood infusion for adults with ischemic stroke: clinical outcomes from a phase I safety study. Stem Cells Transl Med. (2018) 7:521–9. doi: 10.1002/sctm.18-0008
38. Kalladka D, Sinden J, Pollock K, Haig C, McLean J, Smith W, et al. Human neural stem cells in patients with chronic ischaemic stroke (PISCES): a phase 1, first-in-man study. Lancet. (2016) 388:787–96. doi: 10.1016/S0140-6736(16)30513-X
39. Phan TG, Ma H, Lim R, Sobey CG, Wallace EM. Phase 1 trial of amnion cell therapy for ischemic stroke. Front Neurol. (2018) 9:198. doi: 10.3389/fneur.2018.00198
40. Chen J, Li Y, Wang L, Lu M, Zhang X, Chopp M. Therapeutic benefit of intracerebral transplantation of bone marrow stromal cells after cerebral ischemia in rats. J Neurol Sci. (2001) 189:49–57. doi: 10.1016/S0022-510X(01)00557-3
41. Stroemer P, Patel S, Hope A, Oliveira C, Pollock K, Sinden J. The neural stem cell line CTX0E03 promotes behavioral recovery and endogenous neurogenesis after experimental stroke in a dose-dependent fashion. Neurorehabil Neural Repair. (2009) 23:895–909. doi: 10.1177/1545968309335978
42. Smith EJ, Stroemer RP, Gorenkova N, Nakajima M, Crum WR, Tang E, et al. Implantation site and lesion topology determine efficacy of a human neural stem cell line in a rat model of chronic stroke. Stem Cells. (2012) 30:785–96. doi: 10.1002/stem.1024
43. Wei N, Yu SP, Gu X, Taylor TM, Song DX, Liu F, et al. Delayed intranasal delivery of hypoxic-preconditioned bone marrow mesenchymal stem cells enhanced cell homing and therapeutic benefits after ischemic stroke in mice. Cell Transplant. (2013) 22:977–91. doi: 10.3727/096368912X657251
44. Kameshwar Prasad D, Sharma A, Garg A, Mohanty S, Bhatnagar S, Sharat Johri DD, et al. Intravenous autologous bone marrow mononuclear stem cell therapy for ischemic stroke. Stroke. (2014) 45:3618–24. doi: 10.1161/STROKEAHA.114.007028
45. Dallas MH, Triplett B, Shook DR, Hartford C, Srinivasan A, Laver J, et al. Long-term outcome and evaluation of organ function in pediatric patients undergoing haploidentical and matched related hematopoietic cell transplantation for sickle cell disease. Biol Blood Marrow Transplant. (2013) 19:820–30. doi: 10.1016/j.bbmt.2013.02.010
46. Banerjee S, Bentley P, Hamady M, Marley S, Davis J, Shlebak A, et al. Intra-arterial immunoselected CD34+ stem cells for acute ischemic stroke. Stem Cells Translat Med. (2014) 3:1322–30. doi: 10.5966/sctm.2013-0178
47. Prasad K, Mohanty S, Bhatia R, Srivastava M, Garg A, Srivastava A, et al. Autologous intravenous bone marrow mononuclear cell therapy for patients with subacute ischaemic stroke: a pilot study. Indian J Med Res. (2012) 136:221.
48. Moniche F, Gonzalez AJ, Gonzalez-Marcos R, Carmona M, Piñero P, Espigado I, et al. Intra-arterial bone marrow mononuclear cells in ischemic stroke: a pilot clinical trial. Stroke. (2012) 43:2242–4. doi: 10.1161/STROKEAHA.112.659409
49. Mackie AR, Losordo DW. CD34-positive stem cells in the treatment of heart and vascular disease in human beings. Texas Heart Inst J. (2011) 38:474–85.
50. Savitz SI, Misra V, Kasam M, Juneja H, Cox CS Jr, Alderman S, et al. Intravenous autologous bone marrow mononuclear cells for ischemic stroke. Ann Neurol. (2011) 70:59–69. doi: 10.1002/ana.22458
51. Steinberg GK, Kondziolka D, Wechsler LR, Lunsford LD, Kim AS, Johnson JN, et al. Two-year safety and clinical outcomes in chronic ischemic stroke patients after implantation of modified bone marrow–derived mesenchymal stem cells (SB623): a phase 1/2a study. J Neurosurg. (2018) 1:1–11. doi: 10.3171/2018.5.JNS173147
52. Hess DC, Sila CA, Furlan AJ, Wechsler LR, Switzer JA, Mays RW. A double-blind placebo-controlled clinical evaluation of MultiStem for the treatment of ischemic stroke. Int J Stroke. (2014) 9:381–6. doi: 10.1111/ijs.12065
53. Hess DC, Wechsler LR, Clark WM, Savitz SI, Ford GA, Chiu D, et al. Safety and efficacy of multipotent adult progenitor cells in acute ischaemic stroke (MASTERS): a randomised, double-blind, placebo-controlled, phase 2 trial. Lancet Neurol. (2017) 16:360–8. doi: 10.1016/S1474-4422(17)30046-7
54. Díez-Tejedor E, Gutiérrez-Fernández M, Martínez-Sánchez P, Rodríguez-Frutos B, Ruiz-Ares G, Lara ML, et al. Reparative therapy for acute ischemic stroke with allogeneic mesenchymal stem cells from adipose tissue: a safety assessment: a phase II randomized, double-blind, placebo-controlled, single-center, pilot clinical trial. J Stroke Cerebrovasc Dis. (2014) 23:2694–700. doi: 10.1016/j.jstrokecerebrovasdis.2014.06.011
55. Kurtzberg J, Troy JD, Bennett E, Durham R, Shpall EJ, Wiese J, et al. Allogeneic umbilical Cord Blood Infusion for Adults with Ischemic Stroke (CoBIS): clinical outcomes from a phase 1 study. Biol Blood Marrow Transplant. (2017) 23:S173–4. doi: 10.1016/j.bbmt.2016.12.288
56. Kim SJ, Moon GJ, Chang WH, Kim YH, Bang OY. Intravenous transplantation of mesenchymal stem cells preconditioned with early phase stroke serum: current evidence and study protocol for a randomized trial. Trials. (2013) 14:317. doi: 10.1186/1745-6215-14-317
57. Moniche F, Escudero I, Zapata-Arriaza E, Usero-Ruiz M, Prieto-León MJ, de la Torre J, et al. Intra-arterial bone marrow mononuclear cells (BM-MNCs) transplantation in acute ischemic stroke (IBIS trial): protocol of a phase II, randomized, dose-finding, controlled multicenter trial. Int J Stroke. (2015) 10:1149–52. doi: 10.1111/ijs.12520
58. Willing AE, Shahaduzzaman M. Delivery routes for cell therapy in stroke. In: Jolkkonen J, Walczak P, editors. Cell-Based Therapies in Stroke. Wien: Springer (2013). p. 15–28. doi: 10.1007/978-3-7091-1175-8_2
59. Rodríguez-Frutos B, Otero-Ortega L, Gutiérrez-Fernández M, Fuentes B, Ramos-Cejudo J, Díez-Tejedor E. Stem cell therapy and administration routes after stroke. Translat Stroke Res. (2016) 7:378–87. doi: 10.1007/s12975-016-0482-6
60. Iihoshi S, Honmou O, Houkin K, Hashi K, Kocsis JD. A therapeutic window for intravenous administration of autologous bone marrow after cerebral ischemia in adult rats. Brain Res. (2004) 1007:1–9. doi: 10.1016/j.brainres.2003.09.084
61. Janowski M, Walczak P, Date I. Intravenous route of cell delivery for treatment of neurological disorders: a meta-analysis of preclinical results. Stem Cells Develop. (2010) 19:5–16. doi: 10.1089/scd.2009.0271
62. Dulamea AO. The potential use of mesenchymal stem cells in stroke therapy—From bench to bedside. J Neurol Sci. (2015) 352:1–11. doi: 10.1016/j.jns.2015.03.014
63. Sun JM, Kurtzberg J. Cord blood for brain injury. Cytotherapy. (2015) 17:775–85. doi: 10.1016/j.jcyt.2015.03.004
64. Liska MG, Crowley MG, Borlongan CV. Regulated and unregulated clinical trials of stem cell therapies for stroke. Transl Stroke Res. (2017) 8:93–103. doi: 10.1007/s12975-017-0522-x
65. Garbuzova-Davis S, Haller E, Williams SN, Haim D, Tajiri N, Hernandez-Ontiveros G, et al. Compromised blood–brain barrier competence in remote brain areas in ischemic stroke rats at the chronic stage. J Compar Neurol. (2014) 522:3120–37. doi: 10.1002/cne.23582
66. Minisman G, Bhanushali M, Conwit R, Wolfe GI, Aban I, Kaminski HJ, et al. Implementing clinical trials on an international platform: challenges and perspectives. J Neurol Sci. (2012) 313:1–6. doi: 10.1016/j.jns.2011.10.004
67. Shenoy P. Multi-regional clinical trials and global drug development. Perspect Clin Res. (2016) 7:62–7. doi: 10.4103/2229-3485.179430
68. Lees JS, Sena ES, Egan KJ, Antonic A, Koblar SA, Howells DW, et al. Stem cell-based therapy for experimental stroke: a systematic review and meta-analysis. Int J Stroke. (2012) 7:582–8. doi: 10.1111/j.1747-4949.2012.00797.x
69. Bang OY. Clinical trials of adult stem cell therapy in patients with ischemic stroke. J Clin Neurol. (2016) 12:14–20. doi: 10.3988/jcn.2016.12.1.14
70. Australian Commission on Safety and Quality in Health Care (2017). Economic Evaluation of Investigator-Initiated Clinical Trials Conducted by Networks. Australian Clinical Science Alliance.
71. Lee JS, Hong JM, Moon GJ, Lee PH, Ahn YH, Bang OY. A long-term follow-up study of intravenous autologous mesenchymal stem cell transplantation in patients with ischemic stroke. Stem Cells. (2010) 28:1099–106. doi: 10.1002/stem.430
72. Yavagal D, Huang D, Graffagnino C, Rappard G, Budzik R, Likosky W, et al. Intra-arterial delivery of autologous ALDHbr cells in ischemic stroke: final 1-year results of the recovery-stroke trial. Int J Stroke. (2015) 10:13.
73. Participants S. Stem cell Therapies as an Emerging Paradigm in Stroke (STEPS) bridging basic and clinical science for cellular and neurogenic factor therapy in treating stroke. Stroke. (2009) 40:510–5. doi: 10.1161/STROKEAHA.108.526863
74. Savitz SI, Chopp M, Deans R, Carmichael S, Phinney D, Wechsler L, et al. Stem cell therapy as an emerging paradigm for stroke (STEPS) II. Stroke. (2011) 42:825–9. doi: 10.1161/STROKEAHA.110.601914
75. Savitz SI, Cramer SC, Wechsler LS, Wechsler L, STEPS 3 Consortium Stem cells as an emerging paradigm in stroke 3: enhancing the development of clinical trials. Stroke. (2014) 45:634–9. doi: 10.1161/STROKEAHA.113.003379
76. Bang OY, Lee JS, Lee PH, Lee G. Autologous mesenchymal stem cell transplantation in stroke patients. Ann Neurol. (2005) 57:874–82. doi: 10.1002/ana.20501
77. Fang J, Guo Y, Tan S, Li Z, Xie H, Chen P, et al. Autologous endothelial progenitor cells transplantation for acute ischemic stroke: a 4-year follow-up study. Stem Cells Translat Med. (2019) 8:14–21. doi: 10.1002/sctm.18-0012
78. Napoli E, Borlongan CV. Recent advances in stem cell-based therapeutics for stroke. Transl Stroke Res. (2016) 7:452–7. doi: 10.1007/s12975-016-0490-6
79. dela Peña I, Borlongan CV. Translating G-CSF as an adjunct therapy to stem cell transplantation for stroke. Translat Stroke Res. (2015) 6:421–9. doi: 10.1007/s12975-015-0430-x
80. Osanai T, Houkin K, Uchiyama S, Minematsu K, Taguchi A, Terasaka S. Treatment Evaluation of Acute Stroke for Using in Regenerative Cell Elements (TREASURE) Trial: Rationale and Design. London: SAGE Publications Sage (2018). doi: 10.1177/1747493017743057
81. Borlongan CV. Preliminary reports of stereotaxic stem cell transplants in chronic stroke patients. Mol Ther. (2016) 24:1710–1. doi: 10.1038/mt.2016.186
82. Borlongan CV. Age of PISCES: stem-cell clinical trials in stroke. Lancet. (2016) 388:736–8. doi: 10.1016/S0140-6736(16)31259-4
83. Hassani Z, O'Reilly J, Pearse Y, Stroemer P, Tang E, Sinden J, et al. Human neural progenitor cell engraftment increases neurogenesis and microglial recruitment in the brain of rats with stroke. PLoS ONE. (2012) 7:e50444. doi: 10.1371/journal.pone.0050444
84. Hicks C, Stevanato L, Stroemer RP, Tang E, Richardson S, Sinden JD. In vivo and in vitro characterization of the angiogenic effect of CTX0E03 human neural stem cells. Cell Transplant. (2013) 22:1541–52. doi: 10.3727/096368912X657936
85. Evans MA, Lim R, Kim HA, Chu HX, Gardiner-Mann CV, Taylor KW, et al. Acute or delayed systemic administration of human amnion epithelial cells improves outcomes in experimental stroke. Stroke. (2018) 49:700–9. doi: 10.1161/STROKEAHA.117.019136
86. Kurtzberg J, Durham R, Laskowitz D, Balber AE, Bennett E. Allogeneic umbilical cord blood infusion for adults with ischemic stroke. Biol Blood Marrow Transplant. (2016) 22:S141–2. doi: 10.1016/j.bbmt.2015.11.481
87. Kurtzberg J, Troy JD, Bennett E, Belagaje S, Shpall EJ, Wiese J, et al. Allogeneic umbilical Cord Blood Infusion for Adults with Ischemic Stroke (CoBIS): clinical outcomes from a phase 1 safety study. Am Soc Hematol. (2016) 7:521–29.
88. Ghali AA, Yousef MK, Ragab OA, ElZamarany EA. Intra-arterial infusion of autologous bone marrow mononuclear stem cells in subacute ischemic stroke patients. Front Neurol. (2016) 7:228. doi: 10.3389/fneur.2016.00228
89. Unsworth DJ, Mathias JL, Dorstyn DS. Safety and efficacy of cell therapies administered in the acute and subacute stages after stroke: a meta-analysis. Regenerat Med. (2016) 11:725–41. doi: 10.2217/rme-2016-0063
90. Jeong H, Yim HW, Cho Y-S, Kim YI, Jeong S-N, Kim H-B, et al. Efficacy and safety of stem cell therapies for patients with stroke: a systematic review and single arm meta-analysis. Int J Stem Cells. (2014) 7:63–9. doi: 10.15283/ijsc.2014.7.2.63
91. Cao W, Li P. Effectiveness and safety of autologous bone marrow stromal cells transplantation after ischemic stroke: a meta-analysis. Med Sci Monitor. (2015) 21:2190–5. doi: 10.12659/MSM.895081
92. Sertkaya AH, Wong H, Jessup A, Beleche T. Key cost drivers of pharmaceutical clinical trials in the United States. Clin Trials. (2016) 13:117–26. doi: 10.1177/1740774515625964
93. Evers SM, Struijs JN, Ament AJ, van Genugten ML, Jager JC, van den Bos GA. International comparison of stroke cost studies. Stroke. (2004) 35:1209–15. doi: 10.1161/01.STR.0000125860.48180.48
94. Luengo-Fernandez R, Leal J, Gray A. UK research spend in 2008 and 2012: comparing stroke, cancer, coronary heart disease and dementia. BMJ Open. (2015) 5:e006648. doi: 10.1136/bmjopen-2014-006648
95. Drummond MF, Davies LM, Ferris FL III. Assessing the costs and benefits of medical research: the diabetic retinopathy study. Soc Sci. Med. (1992) 34:973–81. doi: 10.1016/0277-9536(92)90128-D
96. Lopes AG, Sinclair A, Frohlich B. Cost analysis of cell therapy manufacture: autologous cell therapies, part 1. BioProcess Int. (2018) 16:S3–S8.
97. Rafiq Q, Thomas R. The evolving role of automation in process development & manufacture of cell & gene-based therapies. Cell Gene Ther Insights. (2016) 2:473–9. doi: 10.18609/cgti.2016.058
98. Rafiq Q. Emerging automated approaches for cell and gene therapy manufacture. Cell Gene Ther Insights. (2018) 4:911–94. doi: 10.18609/cgti.2018.070
99. Abou-El-Enein M, Elsanhoury A, Reinke P. Overcoming challenges facing advanced therapies in the EU market. Cell Stem Cell. (2016) 19:293–7. doi: 10.1016/j.stem.2016.08.012
100. Galipeau J, Sensébé L. Mesenchymal stromal cells: clinical challenges and therapeutic opportunities. Cell Stem Cell. (2018) 22:824–33. doi: 10.1016/j.stem.2018.05.004
101. Bekelman JE, Li Y, Gross CP. Scope and impact of financial conflicts of interest in biomedical research: a systematic review. JAMA. (2003) 289:454–65. doi: 10.1001/jama.289.4.454
102. Patsopoulos NA, Ioannidis JP, Analatos AA. Origin and funding of the most frequently cited papers in medicine: database analysis. BMJ. (2006) 332:1061–4. doi: 10.1136/bmj.38768.420139.80
103. Ioannidis JP, Greenland S, Hlatky MA, Khoury MJ, Macleod MR, Moher D, et al. Increasing value and reducing waste in research design, conduct, and analysis. Lancet. (2014) 383:166–75. doi: 10.1016/S0140-6736(13)62227-8
104. Janiaud P, Cristea IA, Ioannidis JP. Industry-funded versus non-profit-funded critical care research: a meta-epidemiological overview. Intensive Care Med. (2018) 44:1613–27. doi: 10.1007/s00134-018-5325-3
105. Lundh A, Sismondo S, Lexchin J, Busuioc OA, Bero L. Industry sponsorship and research outcome. Cochrane Database Syst Rev. (2012) 12. doi: 10.1002/14651858.MR000033.pub2
106. Fung M, Yuan Y, Atkins H, Shi Q, Bubela T. Responsible translation of stem cell research: an assessment of clinical trial registration and publications. Stem Cell Rep. (2017) 8:1190–201. doi: 10.1016/j.stemcr.2017.03.013
107. Schulz KF, Altman DG, Moher D. CONSORT 2010 statement: updated guidelines for reporting parallel group randomized trials. Ann Int Med. (2010) 152:726–32. doi: 10.7326/0003-4819-152-11-201006010-00232
108. Chan A-W, Tetzlaff JM, Altman DG, Dickersin K, Moher D. SPIRIT 2013: new guidance for content of clinical trial protocols. Lancet. (2013) 381:91–2. doi: 10.1016/S0140-6736(12)62160-6
Keywords: stroke, clinical trial, stem cells, cell therapy, clinical trial costs
Citation: Krause M, Phan TG, Ma H, Sobey CG and Lim R (2019) Cell-Based Therapies for Stroke: Are We There Yet? Front. Neurol. 10:656. doi: 10.3389/fneur.2019.00656
Received: 01 March 2019; Accepted: 04 June 2019;
Published: 25 June 2019.
Edited by:
Olivier Detante, Centre Hospitalier Universitaire de Grenoble, FranceReviewed by:
Cesar V. Borlongan, University of South Florida, United StatesClaire Rome, Université Grenoble Alpes, France
Copyright © 2019 Krause, Phan, Ma, Sobey and Lim. This is an open-access article distributed under the terms of the Creative Commons Attribution License (CC BY). The use, distribution or reproduction in other forums is permitted, provided the original author(s) and the copyright owner(s) are credited and that the original publication in this journal is cited, in accordance with accepted academic practice. No use, distribution or reproduction is permitted which does not comply with these terms.
*Correspondence: Mirja Krause, Mirja.krause@hudson.org.au