- 1Department of Neurology, University of Greifswald, Greifswald, Germany
- 2Department of Computing, Goldsmiths, University of London, London, United Kingdom
- 3Department of Neurology, Charité Universitätsmedizin Berlin, Berlin, Germany
- 4Department of Neurophysics, Max Planck Institute for Human Cognitive and Brain Sciences, Leipzig, Germany
- 5Psychologische Hochschule Berlin, Berlin, Germany
The relevance of infections as risk factor for cerebrovascular disease is being increasingly recognized. Nonetheless, the pathogenic link between the two entities remains poorly understood. Consistent with recent advances in medicine, the present work addresses the hypothesis that infection-induced immune responses may affect human proteins associated with stroke. Applying established procedures in bioinformatics, the pathogen antigens and the human proteins were searched for common sequences using pentapeptides as probes. The resulting data demonstrate massive peptide sharing between infectious pathogens—such as Chlamydia pneumoniae, Streptococcus pneumoniae, Tannerella forsythia, Haemophilus influenzae, Influenza A virus, and Cytomegalovirus—and human proteins related to risk of ischemic and hemorrhagic stroke. Moreover, the shared peptides are also evident in a number of epitopes experimentally proven immunopositive in the human host. The present findings suggest cross-reactivity as a potential mechanistic link between infections and stroke.
Introduction
When considered separately from other cardiovascular diseases, stroke ranks fifth among all causes of death (1) and, critically, its incidence is on the rise (2).
The etiology of stroke is multifactorial with various environmental and genetic risk factors. Hypertension, diabetes and insulin resistance, smoking, dyslipidemia, obesity, heavy alcohol consumption, atrial fibrillation, and carotid stenosis are all established and well-investigated modifiable risk factors of stroke (3–5).
Additionally, there is evidence that environmental factors may also increase risk of stroke, including viral and bacterial infections, such as periodontitis (6) and respiratory infections (7), and infection with Chlamydia pneumoniae (8) or Cytomegalovirus (9). However, relatively little is known so far about the role of different pathogens as well as the molecular basis and the mechanisms that potentially link infections to stroke.
Here we set out to investigate whether or not infections can induce immune responses capable of cross-reacting with human proteins that, when altered, have been associated with stroke. Our hypothesis was that immune responses induced by infectious agents might cross-react with crucial stroke-related proteins, thus contributing to the multifactorial pathogenesis of cerebrovascular disease.
To address this hypothesis, we analyzed pathogens, as well as proteins that are known to be associated with increased risk of ischemic and hemorrhagic stroke by searching for common peptides that might underlie cross-reactions.
Specifically, we analyzed antigens from the following pathogens that have been reported to have a possible influence on stroke: the periodontal bacterium Tannerella forsythia (10), Haemophilus influenza (11), Streptococcus pneumoniae (7), Chlamydia pneumoniae (8), Influenza A viruses (12, 13), and Human Cytomegalovirus (9).
Methods
We analyzed the amino acid (aa) primary sequence of pathogen antigens (with short name and UniProt ID in parentheses):
• Surface antigen repeat/outer membrane protein (OMP; UniProtKB: A0A0F7WYE8_CHLPN) from Chlamydia pneumoniae;
• Pneumococcal vaccine antigen A (PVAA;UniProtKB: PVAA_STRR6) from Streptococcus pneumoniae;
• Surface antigen BspA (BspA; UniProtKB: O68831_TANFO) from Tannerella forsythia;
• Outer membrane antigenic lipoprotein B (LPPB; UniProtKB: LPPB_HAEIN) from Haemophilus influenzae (strain ATCC 51907);
• Hemagglutinin (HA H1N1; UniProtKB: HEMA_I34A1) from Influenza A virus (strain A/Puerto Rico/8/1934 H1N1);
• Hemagglutinin (HA H5N1; UniProtKB: HEMA_I96A0) from Influenza A virus (strain A/Goose/Guangdong/1/1996 H5N1);
• Hemagglutinin (HA H3N2; UniProtKB: HEMA_I68A6) from Influenza A virus (strain A/Northern Territory/60/1968 H3N2); and
• 65 kDa phosphoprotein (pp65; UniProtKB: PP65_HCMVM) from Human Cytomegalovirus (HCMV; strain Merlin).
The primary sequence of pathogen antigens was dissected into partially overlapping pentapeptides with a one-residue-offset: i.e., MFKRI, FKRIR, KRIRR, and so on. Then, each pentapeptide was analyzed for occurrences within a library consisting of primary sequences of human proteins involved in stroke. The human protein library was a priori chosen from the UniProtKB Database (https://www.uniprot.org) (14) using the keyword “stroke.” We obtained an unbiased list of 74 human proteins (in)directly associated with stroke (Table S1). Stroke-related proteins are indicated as UniProtKB entry names throughout the present article, except when discussed in detail. The pathogen antigens and the human proteins were searched for common sequences using the pentapeptide as a probe unit because a pentapeptide is an immunobiological determinant sufficient for epitope-paratope interaction and for inducing specific immune responses (15–18).
The immunologic potential of the shared peptides was analyzed using the Immune Epitope Database (IEDB; www.iedb.org) (19). All evaluations were based only on epitopic sequences that had been experimentally validated as immunopositive in the human host.
This linear peptide similarity analysis procedure has been used and described before (20, 21).
Results
In a detailed overview, Table 1 shows that 49 out of the 74 human stroke-related proteins share peptide sequences with antigens from pathogens that proved to be (in)directly involved in stroke (6–10). It can be seen that
• The pathogen vs. human peptide overlap is unexpectedly high when considering that the probability for two proteins to share a pentapeptide is 1 out of 20−5, that is, 0.0000003125 or close to zero.
• The peptide overlap varies widely, with T. forsythia BspA and Influenza A HA H3N2 being the pathogen more and less involved in the peptide sharing, respectively.
• The high number of stroke-related proteins involved in the viral peptide overlap precludes a detailed protein-by-protein analysis. However, an example worth noting is the human ATP-binding cassette sub-family C member nine (ABCC9 or SUR2) that shares peptide sequences with all of the pathogen antigens analyzed, with the exception of the Influenza A HA H3N2 virus. ABCC9 is a subunit of ATP-sensitive potassium channels (KATP) that can form cardiac and smooth muscle-type KATP channels with KCNJ11 and mediates neuroprotection (22).
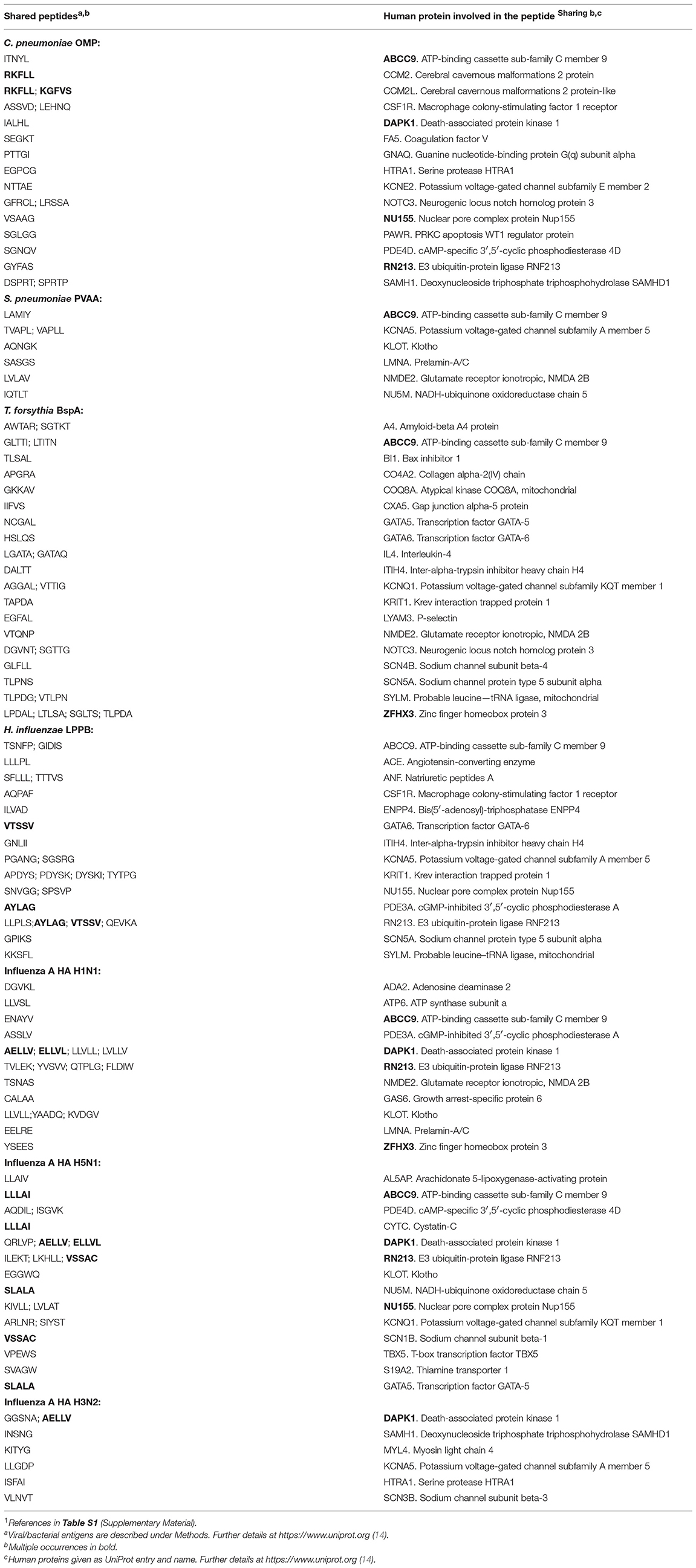
Table 1. Peptide sharing between pathogen antigens and human proteins that have been associated with stroke1.
In summary, Table 1 describes a peptide platform that connects the infectious agents under analysis human proteins related to stroke.
Subsequently, in order to define the immunologic potential of the shared peptides, we conducted analyses throughout the peptide immunome cataloged in the Immune Epitope Database (IEDB; www.iedb.org) (19). The search was finalized to identify epitopic sequences corresponding to (or containing) the peptide sequences shared between stroke-related infectious agents and stroke-related human proteins. It was found that a great number of the shared peptides listed in Table 1 are also distributed through hundreds of epitopic sequences with an immunological potential. A list of such epitopic sequences is reported in Table 2.
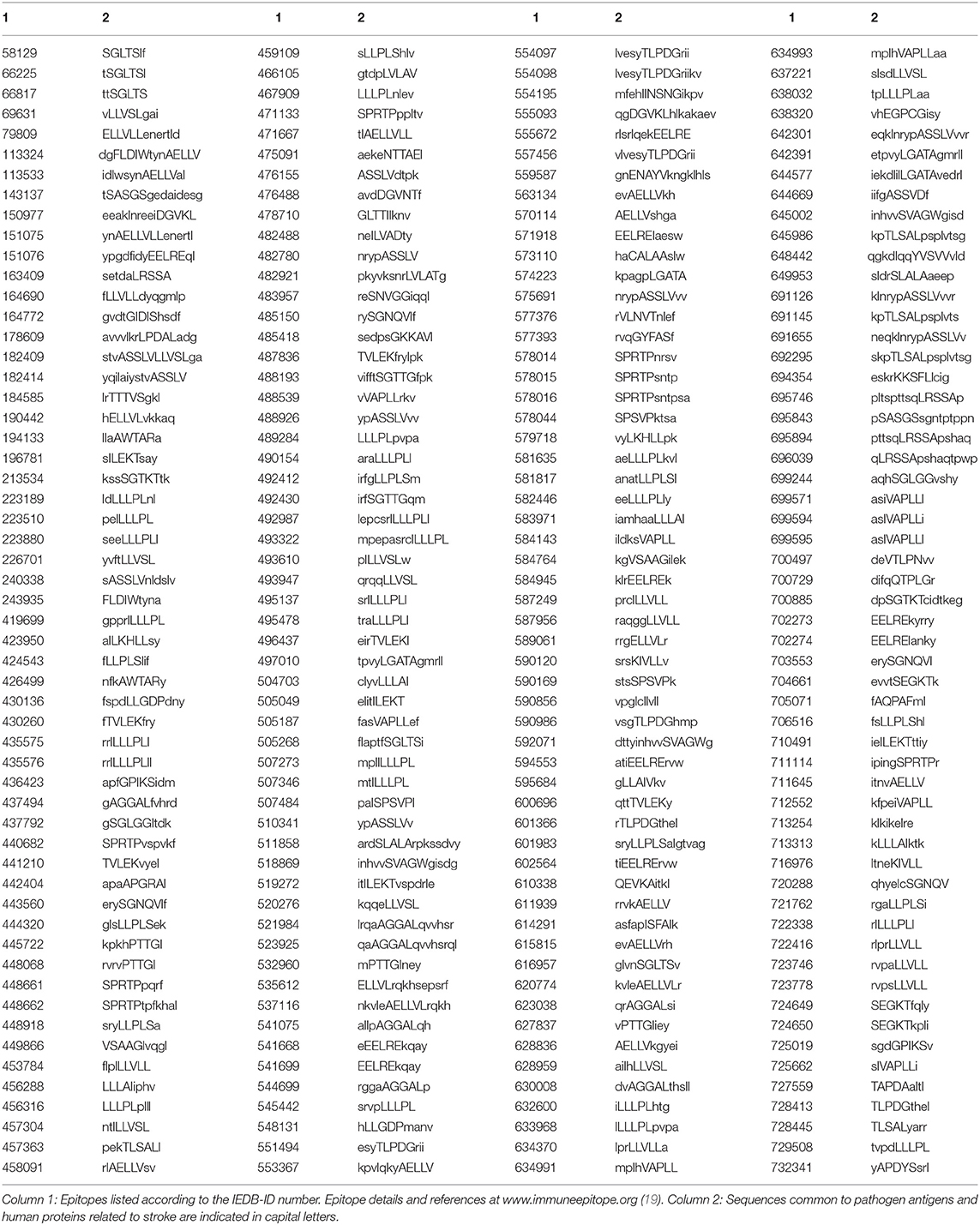
Table 2. Epitopes immunopositive in the human host and containing peptide sequences common to antigens from stroke-related pathogens and human proteins associated with stroke.
Conclusion
Stroke risk appears to be the result of a complex combination of multiple genetic non-modifiable and environmental modifiable factors that can be further classified as either “traditional” or new, “emerging” ones (23). As highlighted by Grau et al. (24, 25), the occurrence of stroke is only partially explained by traditional modifiable cardiovascular risk factors, such as increasing blood pressure, cigarette smoking, and diabetes mellitus. Most importantly, infectious diseases appear to play a key role in contributing to the risk of stroke and are to be counted among “emerging” modifiable risk factors that receive increasing scientific interest (6–10, 23, 26, 27).
Searching for possible immunopathogenic links between infection and risk of stroke, the present study aimed to analyze the potential immunologic relationship between pathogens and human proteins that, when altered, have been associated with risk of stroke. In line with our hypothesis, we found that immune cross-reactions between infectious pathogens and human stroke-related proteins might occur, thus increasing the risk of stroke (see Tables 1, 2).
The immunologically relevant peptide sharing reported in the present study depicts a complex scenario. Some potential molecular targets of cross-reactions are proteins belonging to the cardiovascular system, thus possibly directly accounting for cerebrovascular damage. Other possible targets are proteins of the immune system, thus suggesting mechanisms resulting in immune dysregulation which could lead to cerebrovascular damage.
An example of the first type of potential targets are ion-channels, particularly potassium (K+) and sodium (Na+) channels (ABCC9, KCNE2, KCNA5, KCNQ1, SCN4B, SCN5A, SCN1B, SCN3B, see Table 1). Accordingly, a growing body of evidence points to the involvement of cardiac K+ and Na+ channel dysfunction (cardiac channelopathies as a result of genetic mutations and/or inflammatory mechanisms) in the pathogenesis of atrial fibrillation (AF), an established risk factor for stroke (28, 29). Moreover, autoantibodies targeting ion-channels may be involved in cardiac arrhythmias (30). In light of the potential cross-reactivity suggested by the observed peptide sharing, AF and subsequent stroke could result from antibodies primarily targeting epitopes of infective agents but also cross-reacting with cardiac ion channels. For instance, activating antibodies could lead to a gain-of-function of K+-channels and inhibiting antibodies to a loss-of-function of of Na+-channels. This could promote re-entry or increase susceptibility to early and/or delayed afterdepolarizations, two mechanisms that can generate AF (31, 32).
The second class of potential targets includes proteins that actively modulate the inflammatory response, such as cytokines and colony-stimulating factor receptors (IL-4, macrophage colony-stimulating factor 1 receptor, see Table 1) (33, 34). IL-4, for example, is a well-investigated tolerogenic cytokine that is able to suppress inflammatory responses and organ-specific autoimmunity in both animal models and humans (35, 36). It is then conceivable that autoantibodies downregulating the function of these proteins can promote inflammatory responses, thus increasing the risk of cerebrovascular damage and stroke. Indeed, inflammatory responses appear to be crucial in the pathogenesis of stroke by inducing atherosclerosis progression, pro-thrombotic activation, and AF—among other mechanisms (37). Inflammation can therefore be considered as one key factor underpinning the relationship between classical stroke risk factors and comorbidities. It appears that not just single infections, but overall infectious burden from multiple agents predicts stroke incidence. Moreover, poor outcome may be proportional to systemic inflammatory burden both in patients and experimental models. For instance, Influenza and Streptococcus infection seem to contribute to stroke incidence and outcome, and evidence from experimental models indicate that blocking inflammatory processes might be an effective prevention strategy (38, 39).
The increasingly recognized relevance of inflammation in stroke is consistent with a possible role of peptide sharing-based cross-reactivity as contributing factors to cerebrovascular damage. In fact, the past two decades of immunologic research have radically changed the way we think of inflammation and innate immunity. It is now known that innate immune responses can “specifically” drive the following adaptive responses through recognition of pathogen-associated molecular patterns (PAMPs) (40, 41). That is to say that peptide epitopes, cell-wall components, and other PAMPs activate immune cells already from the very first stages of immune reactions and drive inflammation. Indeed, there are examples of cross-reactivity between host and pathogen-associated molecular patterns: identical inflammasomes and toll-like receptors (TLRs) recognizing molecular fingerprints of both pathogens (the PAMPs) and injured host cells (so-called danger-associated molecular patterns; DAMPs). For instance, both bacteria LPS and HMGB1 from injured host cells activate TLR4, with consequent inflammation in various tissues including the brain (41, 42). TLR- and inflammasome-dependent pathways seem to be important drivers of inflammation, vascular disease, and reportedly contribute to stroke outcome (43, 44).
Our preliminary results underline the importance of further experimental efforts to define the molecular basis through which microbial infections might contribute to an increased risk of stroke (45–50). Future studies should evaluate immunoreactivity against the peptides shared by infectious pathogens and human stroke-related proteins in sera from stroke patients. Possibly, such serological analyses could also help identify specific markers predicting a higher risk of stroke and might therefore be useful to design preventive strategies following an infection. The ultimate translational relevance of our finding lies in the possibility of adopting effective individualized primary and secondary preventive strategies in patients at risk for stroke after infections. Generic hygienic measure, as well as antibiotic prophylaxis and vaccination campaigns have already been proposed and tested with contrasting results (37). Identifying and stratifying patients according to individual biomarker profiles would allow to personalize treatment for each patient, thus possibly increasing overall efficacy.
Until now, stroke is a leading cause of preventable death and adult disability (1–5, 47–52), but preventive strategies mostly concentrate on traditional cardiovascular risk factors (53–56). Moreover, “cryptogenic” stroke (i.e., ischemic stroke with no obvious cause) poses a challenge in terms of primary and secondary prevention (57, 58).
Given the burden of cerebrovascular disease, and the potential to identify immunological markers that may then serve as prognostic indicators of risk of cerebrovascular damage after an infection, our results justify further intensive research on the cross-reactive link between infections and risk of stroke.
Author Contributions
GL formulated the hypothesis, analyzed the data and wrote the manuscript. GL, AF, and BS interpreted the data, revised and finalized the manuscript.
Funding
We acknowledge support for the Article Processing Charge from the DFG (German Research Foundation, 393148499) and the Open Access Publication Fund of the University of Greifswald and from the Deutsche Forschungsgemeinschaft to AF (SFB1315 TP B03).
Conflict of Interest Statement
AF received consulting fees from Bayer and Novartis and honoraria for oral presentations from Novartis, Böhringer-Ingelheim, Lilly, and Biogen Idec (unrelated to current research).
The remaining authors declare that the research was conducted in the absence of any commercial or financial relationships that could be construed as a potential conflict of interest.
Supplementary Material
The Supplementary Material for this article can be found online at: https://www.frontiersin.org/articles/10.3389/fneur.2019.00469/full#supplementary-material
References
1. Benjamin EJ, Virani SS, Callaway CW, Chamberlain AM, Chang AR, Cheng S, et al. Heart disease and stroke statistics-2018 update: a report from the American Heart Association. Circulation. (2018) 137:e67–e492. doi: 10.1161/CIR.0000000000000573
2. Cabral NL, Freire AT, Conforto AB, Dos Santos N, Reis FI, Nagel V, et al. Increase of stroke incidence in young adults in a middle-income country: a 10-year population-based study. Stroke. (2017) 48:2925–30. doi: 10.1161/STROKEAHA.117.018531
3. Kirshner HS. Differentiating ischemic stroke subtypes: risk factors and secondary prevention. J Neurol Sci. (2009) 279:1–8. doi: 10.1016/j.jns.2008.12.012
4. Tonk M, Haan J. A review of genetic causes of ischemic and hemorrhagic stroke. J Neurol Sci. (2007) 257:273–9. doi: 10.1016/j.jns.2007.01.037
6. Leira Y, Seoane J, Blanco M, Rodríguez-Yáñez M, Takkouche B, Blanco J, et al. Association between periodontitis and ischemic stroke: a systematic review and meta-analysis. Eur J Epidemiol. (2017) 32:43–53. doi: 10.1007/s10654-016-0170-6
7. Blackburn R, Zhao H, Pebody R, Hayward A, Warren-Gash C. Laboratory-confirmed respiratory infections as predictors of hospital admission for myocardial infarction and stroke: time-series analysis of english data for 2004-2015. Clin Infect Dis. (2018) 67:8–17. doi: 10.1093/cid/cix1144
8. Wimmer ML, Sandmann-Strupp R, Saikku P, Haberl RL. Association of chlamydial infection with cerebrovascular disease. Stroke. (1996) 27:2207–10. doi: 10.1161/01.STR.27.12.2207
9. Wang H, Peng G, Bai J, He B, Huang K, Hu X, et al. Cytomegalovirus infection and relative risk of cardiovascular disease (ischemic heart disease, stroke, and cardiovascular death): a meta-analysis of prospective studies up to 2016. J Am Heart Assoc. (2017) 6:e005025. doi: 10.1161/JAHA.116.005025
10. Chukkapalli SS, Rivera-Kweh MF, Velsko IM, Chen H, Zheng D, Bhattacharyya I, et al. Chronic oral infection with major periodontal bacteria Tannerella forsythia modulates systemic atherosclerosis risk factors and inflammatory markers. Pathog Dis. (2015) 73:ftv009. doi: 10.1093/femspd/ftv009
11. Takeoka M, Takahashi T. Infectious and inflammatory disorders of the circulatory system and stroke in childhood. Curr Opin Neurol. (2002) 15:159–64. doi: 10.1097/00019052-200204000-00006
12. Ekstrand JJ. Neurologic complications of influenza. Semin Pediatr Neurol. (2012) 19:96–100. doi: 10.1016/j.spen.2012.02.004
13. Urbanek C, Palm F, Grau AJ. Influenza and stroke risk: a key target not to be missed? Infect Disord Drug Targets. (2010) 10:122–31. doi: 10.2174/187152610790963474
14. UniProt Consortium T. UniProt: the universal protein knowledgebase. Nucleic Acids Res. (2018) 46:2699. doi: 10.1093/nar/gky092
15. Reddehase MJ, Rothbard JB, Koszinowski UH. A pentapeptide as minimal antigenic determinant for MHC class I-restricted T lymphocytes. Nature. (1989) 337:651–3. doi: 10.1038/337651a0
16. Frank SA. Immunology and Evolution of Infectious Disease. Princeton, NJ: Princeton University Press (2002).
17. Kanduc D. Pentapeptides as minimal functional units in cell biology and immunology. Curr Protein Pept Sci. (2013) 14:111–20. doi: 10.2174/1389203711314020003
18. Kanduc D. Homology, similarity, and identity in peptide epitope immunodefinition. J Pept Sci. (2012) 18:487–94. doi: 10.1002/psc.2419
19. Vita R, Overton JA, Greenbaum JA, Ponomarenko J, Clark JD, Cantrell JR, et al. The immune epitope database (IEDB) 3.0. Nucleic Acids Res. (2015) 43 (Database issue): D405–12. doi: 10.1093/nar/gku938
20. Lucchese G, Capone G, Kanduc D. Peptide sharing between influenza A H1N1 hemagglutinin and human axon guidance proteins. Schizophr Bull. (2014) 40:362–75. doi: 10.1093/schbul/sbs197
21. Lucchese G, Stahl B. Peptide sharing between viruses and DLX Proteins: a potential cross-reactivity pathway to neuropsychiatric disorders. Front Neurosci. (2018) 12:150. doi: 10.3389/fnins.2018.00150
22. Liu R, Wang H, Xu B, Chen W, Turlova E, Dong N, et al. Cerebrovascular safety of sulfonylureas: the role of KATP channels in neuroprotection and the risk of stroke in patients with type 2 diabetes. Diabetes. (2016) 65:2795–809. doi: 10.2337/db15-1737
23. Boehme AK, Esenwa C, Elkind MS. Stroke risk factors, genetics, and prevention. Circ Res. (2017) 120:472–95. doi: 10.1161/CIRCRESAHA.116.308398
24. Grau AJ, Brandt T. Do multiple chronic infections increase the risk of stroke? the infectious burden concept. Arch Neurol. (2010) 67:16–17. doi: 10.1001/archneurol.2009.301
25. Grau AJ, Urbanek C, Palm F. Common infections and the risk of stroke. Nat Rev Neurol. (2010) 6:681–94. doi: 10.1038/nrneurol.2010.163
26. Manousakis G, Jensen MB, Chacon MR, Sattin JA, Levine RL. The interface between stroke and infectious disease: infectious diseases leading to stroke and infections complicating stroke. Curr Neurol Neurosci Rep. (2009) 9:28–34. doi: 10.1007/s11910-009-0005-x
27. Elkind MS, Ramakrishnan P, Moon YP, Boden-Albala B, Liu KM, Spitalnik SL, et al. Infectious burden and risk of stroke: the northern Manhattan study. Arch Neurol. (2010) 67:33–8. doi: 10.1001/archneurol.2009.271
28. Hucker WJ, Saini H, Lubitz SA, Ellinor PT. Atrial fibrillation genetics: is there a practical clinical value now or in the future? Can J Cardiol. (2016) 32, 1300–5. doi: 10.1016/j.cjca.2016.02.032
29. Lazzerini PE, Capecchi PL, El-Sherif N, Laghi-Pasini F, Boutjdir M. Emerging arrhythmic risk of autoimmune and inflammatory cardiac channelopathies. J Am Heart Assoc. (2018) 7:e010595. doi: 10.1161/JAHA.118.010595
30. Lazzerini PE, Capecchi PL, Laghi-Pasini F, Boutjdir M. Autoimmune channelopathies as a novel mechanism in cardiac arrhythmias. Nat Rev Cardiol. (2017) 14:521–35. doi: 10.1038/nrcardio.2017.61
31. Nattel S, Burstein B, Dobrev D. Atrial remodeling and atrial fibrillation: mechanisms and implications. Circ Arrhythm Electrophysiol. (2008) 1:62–73. doi: 10.1161/CIRCEP.107.754564
32. Nattel S, Dobrev D. Electrophysiological and molecular mechanisms of paroxysmal atrial fibrillation. Nat Rev Cardiol. (2016) 13:575–90. doi: 10.1038/nrcardio.2016.118
33. Sredni-Kenigsbuch D. TH1/TH2 cytokines in the central nervous system. Int J Neurosci. (2002) 112:665–703. doi: 10.1080/00207450290025725
34. Hamilton JA, Achuthan A. Colony stimulating factors and myeloid cell biology in health and disease. Trends Immunol. (2013) 34:81–9. doi: 10.1016/j.it.2012.08.006
35. Yang WC, Hwang YS, Chen YY, Liu CL, Shen CN, Hong WH, et al. Interleukin-4 supports the suppressive immune responses elicited by regulatory T cells. Front Immunol. (2017) 8:1508. doi: 10.3389/fimmu.2017.01508
36. Guenova E, Skabytska Y, Hoetzenecker W, Weindl G, Sauer K, Tham M, et al. IL-4 abrogates TH17 cell-mediated inflammation by selective silencing of IL-23 in antigen-presenting cells. Proc Natl Acad Sci USA. (2015) 112:2163–8. doi: 10.1073/pnas.1416922112
37. Esenwa CC, Elkind MS. Inflammatory risk factors, biomarkers and associated therapy in ischaemic stroke. Nat Rev Neurol. (2016) 12:594–604. doi: 10.1038/nrneurol.2016.125
38. Muhammad S, Haasbach E, Kotchourko M, Strigli A, Krenz A, Ridder DA, et al. Influenza virus infection aggravates stroke outcome. Stroke. (2011) 42:783–91. doi: 10.1161/STROKEAHA.110.596783
39. Dénes Á, Pradillo JM, Drake C, Sharp A, Warn P, Murray KN, et al. Streptococcus pneumoniae worsens cerebral ischemia via interleukin 1 and platelet glycoprotein Ibα. Ann Neurol. (2014) 75:670–83. doi: 10.1002/ana.24146
40. Root-Bernstein R, Fairweather D. Complexities in the relationship between infection and autoimmunity. Curr Allergy Asthma Rep. (2014) 14:407. doi: 10.1007/s11882-013-0407-3
41. Mills K. H. TLR-dependent T cell activation in autoimmunity. Nat Rev Immunol. (2011) 11:807–22. doi: 10.1038/nri3095
42. Aucott H, Lundberg J, Salo H, Klevenvall L, Damberg P, Ottosson L, et al. Neuroinflammation in response to intracerebral injections of different HMGB1 redox isoforms. J Innate Immun. (2018) 10:215–27. doi: 10.1159/000487056
43. Kim ID, Lee H, Kim SW, Lee HK, Choi J, Han PL, et al. Alarmin HMGB1 induces systemic and brain inflammatory exacerbation in post-stroke infection rat model. Cell Death Dis. (2018) 9:426. doi: 10.1038/s41419-018-0438-8
44. Vogelgesang A, May VE, Grunwald U, Bakkeboe M, Langner S, Wallaschofski H, et al. Functional status of peripheral blood T-cells in ischemic stroke patients. PLoS ONE. (2010) 5:e8718. doi: 10.1371/journal.pone.0008718
45. Emsley HC, Hopkins SJ. Acute ischaemic stroke and infection: recent and emerging concepts. Lancet Neurol. (2008) 7:341–53. doi: 10.1016/S1474-4422(08)70061-9
46. Lindsberg PJ, Grau AJ. Inflammation and infections as risk factors for ischemic stroke. Stroke. (2003) 34:2518–32. doi: 10.1161/01.STR.0000089015.51603.CC
47. Kuklina EV, Tong X, George MG, Bansil P. Epidemiology and prevention of stroke: a worldwide perspective. Expert Rev Neurother. (2012) 12:199–208. doi: 10.1586/ern.11.99
48. Kim AS, Johnston SC. Temporal and geographic trends in the global stroke epidemic. Stroke. (2013) 44 (6 Suppl. 1):S123–5. doi: 10.1161/STROKEAHA.111.000067
49. Tan RY, Markus HS. Monogenic causes of stroke: now and the future. J Neurol. (2015) 262:2601–16. doi: 10.1007/s00415-015-7794-4
50. Kim YD, Cha MJ, Kim J, Lee DH, Lee HS, Nam CM, et al. Long-term mortality in patients with coexisting potential causes of ischemic stroke. Int J Stroke. (2015) 10:541–6. doi: 10.1111/ijs.12013
51. Carrera E, Maeder-Ingvar M, Rossetti AO, Devuyst G, Bogousslavsky J. Trends in risk factors, patterns and causes in hospitalized strokes over 25 years: the Lausanne Stroke Registry. Cerebrovasc Dis. (2007) 24:97–103. doi: 10.1159/000103123
52. Inzitari D, Eliasziw M, Gates P, Sharpe BL, Chan RK, Meldrum HE, et al. The causes and risk of stroke in patients with asymptomatic internal-carotid-artery stenosis. North American Symptomatic Carotid Endarterectomy Trial Collaborators. N Engl J Med. (2000) 342:1693–700. doi: 10.1056/NEJM200006083422302
53. Rothstein L, Jickling GC. Ischemic stroke biomarkers in blood. Biomark Med. (2013) 7:37–47. doi: 10.2217/bmm.12.104
54. Simats A, García-Berrocoso T, Montaner J. Neuroinflammatory biomarkers: from stroke diagnosis and prognosis to therapy. Biochim Biophys Acta. (2016) 1862:411–24. doi: 10.1016/j.bbadis.2015.10.025
55. Pullagurla SR, Baird AE, Adamski MG, Soper SA. Current and future bioanalytical approaches for stroke assessment. Bioanalysis. (2015) 7:1017–35. doi: 10.4155/bio.15.40
56. Salat D, Penalba A, García-Berrocoso T, Campos-Martorell M, Flores A, Pagola J, et al. Immunological biomarkers improve the accuracy of clinical risk models of infection in the acute phase of ischemic stroke. Cerebrovasc Dis. (2013) 35:220–7. doi: 10.1159/000346591
57. Fonseca AC, Ferro JM. Cryptogenic stroke. Eur J Neurol. (2015) 22:618–23. doi: 10.1111/ene.12673
Keywords: stroke, infections, cross-reactivity, peptides, inflammation
Citation: Lucchese G, Flöel A and Stahl B (2019) Cross-Reactivity as a Mechanism Linking Infections to Stroke. Front. Neurol. 10:469. doi: 10.3389/fneur.2019.00469
Received: 27 January 2019; Accepted: 17 April 2019;
Published: 14 May 2019.
Edited by:
Gregory Jaye Bix, University of Kentucky, United StatesReviewed by:
Adam Denes, Institute of Experimental Medicine (MTA), HungaryPietro Enea Lazzerini, University of Siena, Italy
Copyright © 2019 Lucchese, Flöel and Stahl. This is an open-access article distributed under the terms of the Creative Commons Attribution License (CC BY). The use, distribution or reproduction in other forums is permitted, provided the original author(s) and the copyright owner(s) are credited and that the original publication in this journal is cited, in accordance with accepted academic practice. No use, distribution or reproduction is permitted which does not comply with these terms.
*Correspondence: Guglielmo Lucchese, Z3VnbGllbG1vLmx1Y2NoZXNlQHVuaS1ncmVpZnN3YWxkLmRl