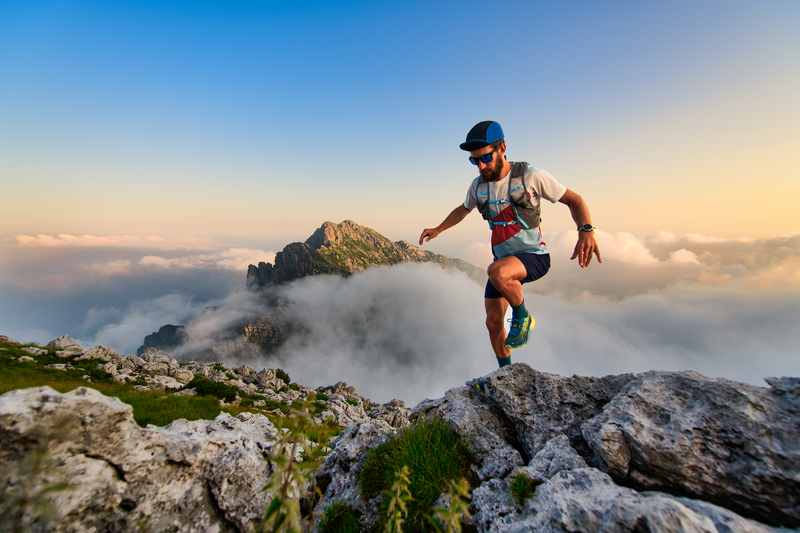
94% of researchers rate our articles as excellent or good
Learn more about the work of our research integrity team to safeguard the quality of each article we publish.
Find out more
ORIGINAL RESEARCH article
Front. Neurol. , 08 May 2019
Sec. Neurorehabilitation
Volume 10 - 2019 | https://doi.org/10.3389/fneur.2019.00408
Objective: A modified reach-to-grasp task has been developed for the purpose of investigating arm-hand coordination in a supine position in the functional magnetic resonance imaging environment. The objective of this study was to investigate the kinematics of the reach-to-grasp task, in stroke and healthy participants.
Design: Observational cohort study.
Setting: Movement laboratory.
Participants: Ten stroke participants and 10 age-matched healthy participants performed 10 repetitions of the modified reach-to-grasp task in two conditions—a natural condition and a standardized condition in a splint.
Intervention: Not applicable.
Main Outcome Measures: Kinematic variables of start time of transport, start time of aperture, movement duration, time of peak velocity (PV), percentage time of PV, peak deceleration (PD), percentage time of PD, peak aperture (PA), time of PA, and percentage time of PA were recorded. The correlation between key events in the grasp and transport trajectories were investigated. Performance between conditions and groups were compared.
Results: Both groups demonstrated a significant correlation between the start time of aperture and the start time of transport and between the time of PA and PV in both conditions. A significant correlation was found between the time of PA and the PD in both conditions for the healthy group, but in neither condition for the stroke group. Movements by participants with stroke had a significantly longer movement duration, a smaller PV, and an earlier absolute time of PV and PD, and an earlier percentage time of PV and PD. They also had a smaller aperture than healthy participants. Wearing the splint resulted in a significantly higher PV, later absolute and percentage time of PV, PD, and PA, and a smaller PA compared to moving without the splint. The timing of transport variables time to peak velocity and time to peak deceleration, were strongest determinants of movement duration.
Conclusion: The modified reach-to-grasp movement performed without the constraint of the splint, demonstrates similar motor control and coordination between the grasp and transport components of reach-to-grasp as in seated reach-to-grasp. This provides a new task that may be used to explore reach-to-grasp in the fMRI environment.
Approximately 77% of people with stroke experience impaired coordination of their upper limb (1). Coordination is defined as an ability to maintain a context-dependent and phase-dependent cyclical relationship between different body segments or joints in both spatial and temporal domains (2). A key functional movement of the upper limb is the ability to reach and grasp an object, therefore how this ability is affected following stroke is of great interest. Investigations of reach-to-grasp typically study reaching movements performed in a sitting position, however sitting does not suit all research environments, such as for example the fMRI environment. The primary objective of this study was therefore to investigate the kinematics of a reach-to-grasp task, performed in supine, in a group of people with stroke and a group of healthy individuals.
The kinematics of reach to grasp include a transport of the hand phase describing the movement of the hand toward the object, driven by extrinsic factors such as the location of the object (3, 4), and a grasp component describing the shaping of the hand to reflect the intrinsic factors such as size and shape of the object to be picked up (3, 4).
These two independent components of the reach-to-grasp movement must be coordinated to effectively grasp an object (5). Evidence of an invariant temporal relationship between these components has been demonstrated in the seated reach-to-grasp movement (5, 6) in healthy people. The initiation of grasp aperture is correlated with the transport component, occurring between 0.2 and 0.4 s of the beginning of the transport phase (5, 7). The peak velocity of the hand occurs within the first half of the movement duration, and the peak hand opening and peak deceleration of the hand occur in the second half of the movement duration, with peak hand opening preceding peak deceleration. There is a correlation between the time of peak hand opening and the time of peak deceleration (7) and between the time of peak hand opening and the time of peak velocity (7, 8). It is suggested these correlations ensure a coordinated representation stored in memory referred to as motor schema, for reach-to-grasp (5).
Substantive data from both computer simulations and real individuals support the idea that reach-to-grasp is controlled with respect to the expected movement duration to the target via a consistent hand closure time (6, 9, 10). There is a two-way interaction between the neural processes controlling transport and grasp, so that the expected duration to the target of each of these trajectories is compared and adjusted, so that they are temporally matched (6). For example, when both object size and location are “perturbed” at movement onset, peak aperture (PA) and peak deceleration (PD) are both delayed to allow them to be temporally matched again after adjustment to each component has been made (11). The expected duration to the target may be determined by a motor schema (12). Alternatively, the expected duration may be derived from internal models (13), acquired and stored in part by the cerebellum.
Motor impairment resulting from a stroke has a significant impact on the co-ordination of a reach-to-grasp movement. Previous research has found common trends including longer movement durations (14, 15), longer deceleration phase (7), abnormal timing of grasp (16) such as an earlier peak aperture (14, 17, 18) and delayed initiation of grasp aperture until the deceleration phase of transport (19), disruption of interjoint coordination (20), increased variability of peak aperture size (7, 18), and deficits in accuracy of transport and grasp (18). It has been reported that some stroke patients can reach directly to a target with relatively normal movement speed, but fail to shape their hand accurately for grasping (15, 18), while other patients reach to an incorrect location, even though they can form a proper grasp (21, 22).
A brief description of the brain activity subserving these kinematics is given here, though longer summaries are available (4). Different areas of the cortex are activated when performing either grasp, transport, or both components together. During the grasp phase, activation of the bilateral anterior intraparietal sulcus (aIPS) (23, 24) and the ventral premotor cortex (vPM area 6), occurs (24). For transport, the superior parieto-occipital cortex (24), the left rostral superior parietal lobe (area 5L), the dorsal premotor area 6 (24) and the medial intraparietal sulcus, and the precuneus (medial aspect of parietal lobe) (25) are activated. When the transport and grasp components are combined, the dorsal premotor cortex (PMd), supplementary motor area (SMA), and the somatomotor areas (S1, S2, and M1) are activated (24). There are also two parietofrontal neural circuits dedicated to determination of object location for hand transport (superior parietal lobule to dorsal premotor area 6) (26) and object size and shape for hand shaping (inferior parietal lobule to ventral premotor area 6) (27).
Much of our understanding of the effect of stroke on the motor control of reach-to-grasp has come from studying the motor performance of people with lesions in specific brain areas. For example, lesions in the parietal cortex result in delayed hand opening (28) and if the lateral bank of the anterior intraparietal area in the posterior parietal cortex is involved, in poor pre-shaping of the hand (29). Cerebellar lesions cause greater variability in the velocity profile, and more velocity peaks compared to healthy people (30) and grasp shows a larger and earlier peak aperture expressed as a percentage of movement duration (30) compared to healthy subjects and these deficits are accentuated in fast movements (31). People with lesions in the posterior limb of internal capsule demonstrate longer movement duration, later time to peak velocity, and a longer deceleration time (32).
However, neuroimaging studies in people with stroke using measures of brain activity such as fMRI during performance of meaningful movement tasks are sparse, being mostly limited to movements such as finger tapping or button pressing. fMRI studies involving reach-to-grasp movements would help to quantify the extent to which a lesion interrupts expected pathways and/or functional networks in individuals or distinct groups of patients. Analysis of the impact of the lesion on brain networks as well as knowledge of viable brain networks has potential to guide rehabilitation clinicians in stroke rehabilitation (4). The lesion may be mapped relative to functional brain regions and networks that are known to be important for particular functions and tasks. There would then be potential to individually tailor treatment according to the underlying neurobiology (33).
Practical problems associated with performing reach-to-grasp movements whilst in the fMRI environment have resulted in limited fMRI studies of arm movements related to everyday function, in people after stroke. The usual seated reach-to-grasp experimental paradigms cannot be applied, so these movements must be adapted to be performed in supine. One concern is that reach-to-grasp movements can result in associated head motion, which potentially creates noise that may be spuriously correlated with the fMRI signal [the blood-oxygenation-level dependent (BOLD) signal]. In order to distinguish these head motion artifacts from the fMRI signal, corrective algorithms must be applied to the data (34). To a certain extent, head motion can be prevented by instructions and training given to the participant, or by restraints such as vacuum cushions that fit inside the head coil. However, these methods are no guarantee for prevention of head motion. A reach-to-grasp movement that can be performed in the fMRI scanner with minimal head motion is therefore desirable.
Where the goal is to understand functional brain networks involved in the control of reach-to-grasp, it is also important that the task shows similar characteristics to tasks performed in everyday life, such as the seated reach-to-grasp task. Bearing these factors in mind, we have developed a modified reach-to-grasp movement that can be performed within the fMRI environment. In developing this test movement, an important consideration was whether task performance should be standardized by a splint, to ensure consistency of movement amplitude between trials and individuals as much as possible, or whether the movement should be performed naturally using visual cues to guide amplitude, which is more ecologically valid. The splint introduces the adverse possibility of altering the kinematic characteristics of the natural movement and so it would be useful to compare the kinematic characteristics during a splint and a natural condition.
The specific hypotheses to be tested in this study were that, as in the seated reach-to-grasp task, first: (a) the start time of hand opening would be correlated with the start of hand transport toward the object; (b) the time of maximum hand opening would be correlated with the time of peak deceleration of the hand; and (c) the time of maximum hand opening would be correlated with the time of peak velocity of the hand. Second, it was hypothesized that use of a splint to guide movement would cause significant changes to performance compared to movement without the splint. Third, people with stroke were expected to be significantly different to that of healthy individuals, with a longer movement duration, smaller peak velocity and peak aperture, and later time of peak velocity, peak deceleration and peak aperture. Fourth it was expected that the degree to which the timing of these variables covaried with movement duration, would be decreased in people with stroke compared to healthy individuals.
Ten participants with stroke were recruited consecutively according to the following inclusion criteria: (1) A clinically confirmed diagnosis of ischemic or haemorrhagic stroke; (2) Remaining upper limb movement deficits, i.e., Scoring < 75 on Wolf Motor Function test; (3) Reside within 50 km of the laboratory; and (4) Give informed written consent. People were excluded if they had: (1) Upper limb movement deficits attributable to non-stroke pathology; and/or (2) Moderate to severe receptive aphasia (< 10 on “receptive skills” of Sheffield Screening Test for Acquired Language Disorders). Eight of the participants were male, mean age was 63.6 (SD = 11), mean time since stroke was 77 months (SD = 58). Participants underwent a structural scan, using a 3T (magnetom Skyra), Siemens Healthcare, T1-weighted image (TR, 5,000 ms; TE, 2.98 ms; field of view, 256 mm; matrix, 256_256; slice thickness, 1.0 mm, 176 slices) to identify the site of the lesion. Scans for 2 of the participants were unavailable due to contraindications (metal implant and pacemaker). Lesion locations for the remaining eight participants (Table 1) were identified visually from these scans by a trained radiographer and additionally confirmed by importing scans into mriCro, drawing around the lesion and superimposing an anatomical template (Automated Anatomical Labeling) onto the scan, which provided anatomical labels of the lesioned area.
The following assessments were used to characterize the group of stroke participants, with results displayed in Table 1. Physical tests to determine upper limb function were the Action Research Arm Test (35), the Wolf Motor Function Test (36); Nottingham Sensory Assessment [Erasmus MC modification (37)], Short-Form McGill Pain Questionnaire (38) and Tardieu Scale (39). To determine the participants cognition the Star Cancellation assessment, Sheffield Screening test for Acquired Language Disorders, Rey-Osterrieth Complex Figure (40), and the Mini Mental State Examination (41) were administered. The Stroke Impact Scale (42) was used to assess quality of life.
Ten healthy participants, matched to stroke participants on age, gender and hand dominance were also recruited from the Hunter Medical Research Institute Research Registry in Newcastle. All healthy participants' upper limb function was within normal limits of the Box and Block test (43).
Participants were required to perform two conditions; a standardized (using a splint) and a natural performance of a modified reach-to-grasp movement. The movement consisted of holding a cylindrical dowel, then opening the hand, flexing the elbow through a range of 20 degrees, and closing the hand on the cylindrical dowel again. Then the hand opened again, the elbow was extended 20 degrees and the hand was closed on the dowel again. Ten repetitions of the movement were performed in each condition. For both conditions the participants were supine on a 600 × 1,820 mm plinth, and the starting position was resting the mid-pronated forearm and hand in the base of the splint which measured 480 mm (h) × 85 mm (w) with a foam wedge to position the participant's upper limb into 20° shoulder flexion and 45° elbow flexion. The splint was attached with velcro to a curved edge lap desk (510 × 380 × 69 mm) that was positioned on the participant's trunk. The hand was visible by the participant throughout the movement. For the standardized condition, the forearm was secured to the splint with velcro strapping. The splint was made from lightweight plastic material, attached to a plastic base via a hinge joint set to lock at 20 degrees. A removable perspex rod 110 mm high and 15 mm diameter was attached to the base in one of two positions for right or left handed trials. Figure 1 shows the task set-up for the splint condition. In the natural condition, the midpronated forearm rested in the same splint base, but movement was performed without the perimeter limiting hand opening or the lock at 20° elbow flexion. Instead, the amount of elbow flexion was guided visually by the top of the rod, measuring a height of 210 mm, which when grasped, approximated 20° elbow flexion. Hand opening was guided by practice prior to data collection, where subjects copied the amount experienced in the splint with the perimeter, in the natural condition. Participants moved at their preferred speed. The order of conditions was randomized using a computerized random sequence. Each stroke participant performed a different random sequence. Healthy participants performed the same random sequence as their matched stroke participant, with the arm matching the side affected by the stroke participant, i.e., dominant or non-dominant.
Prior to data collection, the participant practiced at least four trials of each condition; two in the splint and two natural movements, with more practice if necessary. For both conditions the participants were instructed to “Grasp the bottom marker of the rod using your thumb and finger tips, with your forearm resting in the splint (in the position demonstrated). When I say ‘start' open your hand whilst bending your elbow and grasp the top marker, then open your hand to return to the start position.” The study was approved by Hunter New England Research Ethics Committee (Ref. No. 11/04/20/4.05). Informed written consent was obtained from all participants.
Data were captured using a Qualisys™ 3D motion analysis system with three infrared cameras positioned in an arc near the foot of the plinth, at a sample rate of 200 Hz. The cameras recorded the movement of reflective markers attached to the hand and wrist, to capture the modified reach-to-grasp movement. Two spherical 7 mm reflective markers were fixed with double-sided tape to the lateral surface of the index finger (between the distal interphalangeal joint of the finger and the finger nail), and the medial surface of the thumb (between the distal interphalangeal joint of the thumb and the thumb nail) to measure grasp. A third 12 mm reflective marker was placed on the dorsal side of the forearm, on the radius, 7 cm proximal to the radial styloid process (to measure the transport phase) (44). The wrist marker was elevated by a 2 cm foam cube attached via a velcro strap to ensure visibility of the marker.
Data were processed using Qualisys Track Manager software and analyzed using custom Matlab programmes. Data were filtered using a low pass zero-phase Butterworth digital filter with a cut-off of 8 Hz. Trajectory, velocity, and acceleration were calculated from the three dimensional coordinates of each marker. Movement onset was defined as the time at which the 3D velocity exceeded 5% of the peak velocity value of the wrist (7). The transport component of the modified reach-to-grasp movement was described by the trajectory, velocity, and acceleration of the wrist marker (7). End of transport was defined as the first time at which the maximum distance of the wrist marker, in the combined x,y (horizontal) plane, was achieved. Distance was defined as the distance between the start and end point.
Grasp was described by the trajectory of the thumb and finger markers (7). The start of hand opening was defined as the time at which the planar (3-dimensional) between the thumb and finger marker exceeded 5% of the peak grasp aperture. Peak grasp aperture was defined as the maximum planar distance between the thumb and finger marker. The time to wrist peak velocity, wrist peak deceleration and peak aperture were determined and expressed in absolute and proportional (i.e., as a percentage of movement duration) terms.
Statistical analysis was conducted using the Statistica (Version 13.3) statistical software. To identify whether a relationship existed between the following kinematic parameters (a) start time of hand opening and start time of arm transport; (b) time of peak grasp aperture and time of peak deceleration; and (c) time of peak grasp aperture and time of peak velocity, Pearson's Product Moment Correlation Coefficients were calculated. Within-group correlation coefficients were calculated separately for each condition. Correlations were defined as strong (≥0.7), moderate (0.4–0.69), or low ( ≤ 0.39) (45).
To test the second and third hypotheses i.e., that the splint would cause significant changes to performance compared to movement without the splint, and that performance of the task by people with stroke would be significantly different to that of healthy individuals, differences in the means from the two groups (stroke and healthy) and the two conditions (natural and splint) were tested using multivariate analyses of variance (MANOVA) (46). Results were adjusted for multiple comparisons using least squares means of main effects and interaction effects. MANOVA was chosen instead of separate ANOVA tests for several reasons: (a) increased power—given the response variables are correlated, MANOVA can detect differences too small to be detected through individual ANOVAs; (b) MANOVA can detect multivariate response patterns: the factors [condition, group (stroke vs. healthy)] may influence the relationship between responses (dependent variables) rather than affecting a single response. Single-response ANOVAs can miss these multivariate patterns; (c) MANOVA reduces the likelihood of Type I error: the chance of incorrectly rejecting the null hypothesis increases with each successive ANOVA, whereas running one MANOVA to test all response variables simultaneously keeps the family error rate equal to the alpha level.
As mentioned earlier, movement duration is a key control parameter in reach-to-grasp, with the reach being planned with respect to the expected movement duration to the target. To give a more complete understanding of how these kinematic variables reflect the effort to ensure that the expected duration to the target of each of transport and grasp are temporally matched within an overall model for control of reach to grasp, additional analyses were conducted using ANCOVA (46) to determine how the multiple kinematic variables covaried with movement duration as the independent outcome variable.
To further explore how the multiple kinematic variables covaried with each other, and whether variables behaving similarly could be grouped into a smaller number of construct variables, a factor analysis was performed (46). Then, the same MANOVA and ANCOVA analyses were performed on the factors.
The start of transport of the hand and start of grasp aperture were significantly correlated in all conditions for both groups. Correlations were low for the stroke group: Stroke/natural (r = 0.234, p = 0.037); Stroke/splint (r = 0.275, p = 0.015); and strong for the healthy group: Healthy/natural (r = 0.864, p = 0.000); Healthy/splint (r = 0.822, p = 0.000).
In three of the conditions (stroke/natural, healthy natural, and healthy/splint) the group mean absolute time of peak velocity occurred first, followed by the mean absolute peak aperture time and then the mean absolute time of peak deceleration (Table 2). In the stroke/splint condition, mean absolute peak aperture time occurred first, followed by the mean absolute time of peak velocity, then the mean absolute time of peak deceleration. The mean percentage times of these kinematic parameters follow the same pattern as the absolute times, except for the healthy natural condition, where the % peak aperture preceded % peak velocity.
The absolute time of peak velocity and the absolute time of peak grasp aperture were significantly and positively correlated in all conditions for both groups. Correlations ranged from low to moderate in the stroke group: Stroke/natural (r = 0.272, p = 0.015); Stroke/splint (r = 0.495, p = 0.000); and were moderate in the healthy group: Healthy/natural (r = 0.510, p = 0.000); Healthy/splint (r = 0.573, p = 0.000).
The absolute time of peak deceleration and the absolute time of peak grasp aperture were significantly and positively correlated in both conditions in the healthy group with a moderate level of correlation: Healthy/natural (r = 0.422, p = 0.000); Healthy/splint (r = 0.625, p = 0.000). This relationship was not significant for the stroke group in the splint condition (r = 0.076, p = 0.509), or the natural condition (r = 0.002, p = 0.984).
Means and standard deviation for all kinematic variables are shown in Table 2. Results for MANOVA of group, condition and their interaction modeling all variables together shows a highly significant Wilks Lambda statistic for group (Λ = 0.547 [F(11, 290) = 21.8, p = 0.000], condition (Λ = 0.608, [F(11, 290) = 17, p = 0.000] and group by condition interaction (Λ0.821 [F(11, 290) = 5.8, p = 0.000]). At the univariate level, the group comparison showed a significant difference between the stroke group and the healthy group for all variables except for percentage peak aperture time. Thus, for the stroke group, movement duration was longer [F(1, 300) = 73.98; p < 0.0001], peak velocity was smaller [F(1, 300) = 63.3; p < 0.0001], peak aperture was smaller [F(1, 300) = 21.5; p < 0.0001], and the absolute times of peak velocity [F(1, 300) = 35.2; p < 0.0001], peak deceleration [F(1, 300) = 34.0; p < 0.0001] and peak aperture were later [F(1, 300) = 30.9; p < 0.0001], and percentage time of peak velocity [F(1, 300) = 3.9; p = 0.048] and percentage time of peak deceleration [F(1, 300) = 8.68; p = 0.004] were earlier (Table 2).
The univariate comparisons for condition showed significant differences for all variables except movement duration and absolute time of peak aperture. Thus, for the splint condition, peak velocity size was higher [F(1, 300) = 73.9; p < 0.0001], absolute time of peak velocity [F(1, 300) = 10.6; p < 0.0001], and peak deceleration were later [F(1, 300) = 6.4; p = 0.012], percentage time of peak velocity [F(1, 300) = 37.0; p < 0.0001], peak deceleration [F(1, 300) = 25.8; p < 0.0001], and peak aperture [F(1, 300) = 5.4; p = 0.021] were later and peak aperture was smaller [F(1, 300) = 9.6; p = 0.002] (Table 2).
There were significant group by condition interactions for the following variables: peak velocity size [F(1, 300) = 15.8; p < 0.0001], percentage peak deceleration time [F(1, 300) = 6.9; p = 0.009], absolute peak aperture time [F(1, 300) = 5.9; p = 0.017], and percentage peak aperture time [F(1, 300) = 6.75; p = 0.010]. Thus, the increased velocity size and the later absolute and percentage peak aperture time observed with the splint, were most evident in the healthy group, compared to the stroke group (Table 2). The later percentage peak deceleration time in the splint condition however, was more prevalent in the stroke group than the healthy group.
Factor analysis showed that overall, the kinematic variables could be grouped into four factors (Table 3) which accounted for 70% of the variance. An eigenvalue of >1 was used to justify the factors. Each factor contained linear relationships of two or more variables. Factor 1 consisted of the following variables for the timing of hand transport: time to peak velocity, percentage time to peak velocity, time to peak deceleration, and percentage time to peak deceleration. Factor 2 was comprised of the variables for timing of grasp: time to peak aperture and percentage time to peak aperture. Factor 3 related to speed of transport and aperture size: size of peak velocity and size of peak aperture, and factor 4 was a combination of the start time of each of transport and grasp, along with the distance moved by the wrist. For ease of description, the factors will be labeled as follows: Factor 1 = “Timing of transport;” Factor 2 = “Timing of grasp;” Factor 3 = “Speed and aperture size;” and Factor 4 = “Getting started and distance moved.” “Timing of transport” was the most influential, accounting for 26% of the variance, followed by “Timing of grasp,” accounting for 19%.
Comparison of factors between groups and conditions were performed using MANOVAs. Results for group, condition and their interaction modeling all factors together shows a highly significant Wilks Lambda statistic for group (Λ = 0.636, [F(4, 297) = 42.6, p = 0.000]), condition (Λ = 0.744, [F(4, 297) = 25.6, p = 0.000]) and group by condition interaction (Λ = 0.950 [F(4, 297) = 3.9, p = 0.004]).
At the univariate level, the group comparison of factors showed a significant difference between the stroke group and the healthy group for “Timing of grasp” [F(1, 300) = 4.94, p = 0.027], “Speed and aperture size” [F(1, 300) = 71.8, p = 0.000], and “Getting started and distance moved” (F (1, 300) = 64.3, p = 0.000), but at 10% significance for “Timing of transport” [F(1, 300) = 3.80, p = 0.052]. Thus, for the stroke group, the “timing of grasp component” was later, “speed and aperture size” were smaller, and “Getting started and distance moved” was later.
The univariate comparisons for condition showed significant differences for factors “Timing of transport” [F(1, 300) = 26.5, p = 0.000], “Speed and aperture size” [F(1, 300) = 36.4, p = 0.000]; and “Getting started and distance moved” [F(1, 300) = 34.7, p = 0.000], but not for “Timing of grasp.” Thus, for the splint condition, “Timing of transport component” was later, “Speed and aperture size” were larger and values for “Getting started and distance moved” were later.
There were significant group by condition interactions for “Timing of transport” [F(1, 300) = 4.05, p = 0.045], “Timing of grasp” [F(1, 300) = 8.56, p = 0.004], and “Speed and aperture size” [F(1, 300) = 3.88, p = 0.050, significant at 10%], but not for “Getting started and distance moved.” So for “timing of transport,” the splint caused later transport values in the stroke group compared to the healthy group; for “timing of grasp,” the splint caused earlier values for the stroke group but later values for the healthy group; and the increase in “speed and aperture size” were smaller for the stroke group compared to the healthy group. Table 4 shows a summary of significant findings for both kinematic variables and factors from the MANOVAs.
Table 4. Summary of significant findings for both kinematic variables and factors, showing effect of group, condition, and interaction.
A MANCOVA analysis was done to determine the influence of the kinematic variables on movement duration, in different groups and conditions (Table 5). A later absolute time to peak velocity and peak deceleration in all groups and conditions, and absolute time to peak aperture in only the stroke group (both conditions) was predictive of a longer movement duration. However, an earlier percentage time to peak velocity and peak deceleration in all conditions and percentage time to peak aperture in only the stroke group (both conditions) was predictive of a longer movement duration. A smaller size of peak velocity was predictive of a longer movement duration, only in the stroke group. Peak aperture size and distance moved were not predictive of movement duration in any condition or group. In the stroke, natural condition, a later start time of transport was predictive of a shorter movement duration whereas a later start time of aperture was predictive of a longer movement duration.
Table 5. ANCOVA parameter estimates and p-values for each group and condition for kinematic variables (parameter estimates show increase or decrease in comparison to the intercept, thus a positive value indicates a positive correlation of the variable with movement duration, and “–” indicates a negative correlation with movement duration).
A later “timing of transport” in the stroke group was predictive of a longer movement duration in the natural condition (Table 6). A later “timing of grasp” was predictive of a longer movement duration in both groups for the natural condition, but just in the stroke group for the splint condition. A greater “speed and aperture size” was predictive of a shorter movement duration in all groups and conditions. Finally, a later/greater “getting started and distance moved” was predictive of a longer movement duration in all groups and conditions.
Table 6. ANCOVA parameter estimates and p-values for each group and condition for FACTORS (parameter estimates show increase or decrease in comparison to the intercept, thus a positive value indicates a positive correlation of the factor with movement duration, and “–” indicates a negative correlation with movement duration).
The timing of key kinematic parameters in the performance of the modified reach-to-grasp task was such that the median peak velocity occurred within 50% of movement duration (Table 2) and during the movement, the peak velocity occurred first, followed by peak aperture and then peak deceleration in three out of the four conditions.
The results also demonstrate that the temporal coupling between key grasp and transport events are present in this modified reach-to-grasp task, in healthy people and in the stroke group, with the exception of the correlation between time of peak aperture and time of peak deceleration. Correlations ranged from moderate to strong in the healthy group and from low to moderate in the stroke group.
Wearing the splint did cause some differences in the kinematics of the movement compared to the more natural performance, as hypothesized. Peak velocity was higher, absolute and percentage time of peak velocity and peak deceleration were later, percentage time of peak aperture was later, and peak aperture size was smaller, when wearing the splint.
The later timing of the peak velocity and peak deceleration implies that the splint caused a more ballistic mode of control, with less use of feedback during a shortened deceleration phase, and more reliance on the generation of centralized motor commands prior to movement execution. This effect was more evident in the stroke group with regard to percentage time of peak deceleration. As people with stroke have an abnormally long deceleration phase, wearing the splint appears to have actually normalized their movement in this respect.
The later percentage peak aperture time follows the later times of the transport events, which likely reflects a preservation of the invariant temporal relationship between transport and grasp that ensures a coordinated reach-to-grasp. Stroke participants were less able to make this adjustment of aperture timing (Table 4), which concurs with previous research showing weaker correlation of transport and grasp events in people with stroke (7, 14).
The splint also caused a further distance moved by the wrist (Table 2). This could account for the higher peak velocity and later absolute times of peak velocity and peak deceleration, which may have been required to generate enough force to cover the larger distance. This effect was demonstrated less by the stroke group, who had a smaller increase in wrist distance and peak velocity than in healthy participants, when wearing the splint. This is understandable, as people with stroke have difficulty in generating force to adjust movement extent (47).
The MANOVA on the factors followed the findings from the MANOVA on the kinematic variables. The splint led to later timing of transport events and a later timing of grasp as a proportion of movement duration. The smaller peak aperture found with the splint condition did not appear in the factor “speed and aperture size,” and this could be because the increase in speed outweighed the relatively smaller decrease in aperture size.
Therefore, the natural condition is a more appropriate task to use when performing the modified reach-to-grasp task than the splint condition, because the splint significantly alters the kinematic measures that reflect motor control.
Our third hypothesis concerned whether the performance of the task by people with stroke would be significantly different to that of healthy individuals.
The stroke group showed a smaller aperture (18), a longer movement duration (15) with a corresponding smaller peak velocity (15) and an earlier percentage time of peak velocity and peak deceleration (7), indicating a longer deceleration phase. The longer deceleration phase allows more time to use visual and proprioceptive feedback mechanisms to guide the movement, supposedly to compensate for altered generation of motor commands from damaged neural networks (4). The absolute times to reach peak velocity, peak aperture, and peak deceleration were longer in the stroke group, reflecting the greater wrist distances that were moved by stroke participants (Table 2). Similarly, previous studies demonstrated that people with stroke show errors in movement extent (47) and tend to overshoot close targets (21).
In general, the MANOVA on the factors followed the findings from the MANOVA on the kinematic variables. That is, stroke caused a smaller speed and aperture size, later timing of grasp, and a larger distance moved and later start time. Therefore, in future studies it might be possible to use the factors instead of all the individual kinematic variables in the statistical analyses.
Because of the importance of movement duration as a determining parameter in controlling reach-to-grasp, we analyzed how the dependent variables and the factors derived from these, related to movement duration. Interestingly, kinematic variables relating to timing of transport (and the “timing of transport” factor) were predictive of movement duration in both groups, whereas kinematic variables relating to timing of grasp were only predictive of movement duration in the stroke group. The stronger relationship between grasp and movement duration in the stroke group could be interpreted as indicating that grasp events mirrored those of transport more often in stroke participants, suggesting they were more dependent on this coordination between grasp and transport, to accomplish the task, than the healthy group. This relationship is also demonstrated by a later start time of aperture predicting a longer movement duration, in the stroke, natural condition, but not in the healthy group. This likely reflects the difficulty people with stroke have in generating force in finger extensors to open the hand, so they need longer to accomplish this. Studies of the kinematics of reach-to-grasp rarely report measures of start time of hand aperture, so the finding that this aspect was influential in planning movement duration in a reach-to-grasp task is interesting.
This adaptation of movement duration to a later start time of aperture also implies a preservation of the ability to adapt movement duration to compensate for deficits in aspects of the reach-to-grasp in the stroke group. This preservation is also reflected in the fact that a later start time of transport was predictive of a shorter movement duration, in the stroke, natural condition, which was presumably compensatory for a delayed start time.
Peak aperture size was not an important determining factor for movement duration in either group or condition, and neither was the distance moved. Although the factors containing peak aperture and distance covaried with movement duration, this was caused by other elements within the factors—the size of peak velocity and start time.
Finally, it is interesting to note whether the timing of key kinematic parameters in the performance of the modified reach-to-grasp task follows a similar movement organization to the more commonly reported seated reach-to-grasp tasks. As in previous studies [see (4) for a review], the median peak velocity occurs within 50% of movement duration (Table 2) and during the movement, the peak velocity occurs first, followed by peak aperture and then peak deceleration in three out of the four conditions.
The results also demonstrate that the temporal coupling between key grasp and transport events reported in the literature for seated reach-to-grasp movements, are also present in this modified reach-to-grasp task, in healthy people and for the most part in the stroke group, with the exception of the correlation between time of peak aperture and time of peak deceleration. Correlations ranged from moderate to strong in the healthy group and from low to moderate in the stroke group. Thus, in this respect, the modified reach-to-grasp movement is similar to seated reach-to-grasp movements (7, 8) and is similarly indicative of a coordinated motor schema for reach-to-grasp in the brain (5).
As with previous studies comparing seated reach-to-grasp in people with stroke to healthy performance, the stroke group showed a smaller aperture (18), a longer movement duration (15) with a corresponding smaller peak velocity (15) and an earlier percentage time of peak velocity and peak deceleration (7), indicating a longer deceleration phase.
The findings regarding covariation of dependent variables with movement duration are also in agreement with studies of seated reach-to-grasp in healthy subjects, where timing of transport events are frequently associated with movement duration (9, 48). Our finding that peak aperture size was unimportant in relation to movement duration, however, is in contrast to a previous study investigating potential algorithms used by the central nervous system to control seated reach-to-grasp (49) which found that peak velocity size, peak aperture size and hand acceleration were all used to regulate reach-to-grasp within a model determining hand closure distance.
Because these findings are in common with studies with seated reach-to-grasp tasks, it can be concluded that it is possible to demonstrate the expected differences between stroke and healthy populations using this modified reach-to-grasp task.
The participants with stroke included in this study had mild to moderate arm motor impairment. Therefore, it is not yet known whether the results can be generalized to people with more severe deficits. The suitability of the modified reach-to-grasp task should be evaluated in people with more severe impairment in future research. Our method did not shed any light on muscle activation occurring during reach-to-grasp in supine, which one would expect to be adjusted for the altered relationship with gravity, compared to the seated position.
Overall the modified reach-to-grasp task performed in supine shows similar kinematic characteristics to seated reach-to-grasp tasks and is therefore a method that could be used in the constraints of the fMRI environment to explore and better understand coordination and motor control of reach-to-grasp in people with stroke. Such research is sorely needed (50) and will increase knowledge about the neurobiological basis of recovery to inform the discovery of new treatments to improve motor control. Our recommendation is to have participants perform the movement without the splint, since wearing it caused differences in key kinematic variables compared to the natural condition. Instead, we suggest that participants receive sufficient training of the task beforehand, to encourage consistent performance, and that their performance is recorded with the use of motion capture systems compatible with the MRI environment.
This task may also have applications outside of the fMRI environment. Many people in the stroke population do not have sufficient generation of force in their arm muscles to perform reach-to-grasp movement in a sitting position, where the whole arm must move against gravity. Therefore, the modified reach-to-grasp movement could potentially enable reach-to-grasp coordination and motor control to be assessed in a wider number of people, earlier in the recovery process than previously.
The study was approved by Hunter New England Research Ethics Committee (Ref. No. 11/04/20/4.05). Informed written consent was obtained from all participants.
PvV designed the study, supervised the work and led the writing of this manuscript. KB conducted the study as part of her Honours Bachelor in Occupational Therapy degree, assisted by fellow Honours Bachelor in Occupational Therapy student KP, and research assistant AD. IH led and conducted the statistical analyses. JA and TZ advised on MRI scans. HT identified lesion sites. JK and GC provided computer algorithms for data analysis.
KB was supported by a National Stroke Foundation Honours grant and PvV was supported by an Australian Research Council Future Fellowship (Grant no. 100100439) during the period of this study.
The authors declare that the research was conducted in the absence of any commercial or financial relationships that could be construed as a potential conflict of interest.
1. Lawrence ES, Coshall C, Dundas R, Stewart J, Rudd AG, Howard R, et al. Estimates of the prevalence of acute stroke impairments and disability in a multiethnic population. Stroke. (2001) 32:1279–84. doi: 10.1161/01.STR.32.6.1279
2. Krasovsky T, Levin M. Review: toward a better understanding of coordination in healthy and poststroke gait. Neurorehabil Neural Repair. (2010) 24:213–24. doi: 10.1177/1545968309348509
3. Jeannerod M. The timing of natural prehension movements. J Motor Behav. (1984) 26:235–54. doi: 10.1080/00222895.1984.10735319
4. vanVliet P, Pelton T, Hollands K, Carey L, Wing A. Neuroscience findings on coordination of reaching to grasp an object- implications for research. Neurorehabil. Neural Repair. (2013) 27:622–35. doi: 10.1177/1545968313483578
5. Jeannerod M. The representing brain: neural correlates of motor intention and imagery. Behav Brain Sci. (1994) 17:187–245. doi: 10.1017/S0140525X00034026
6. Hoff B, Arbib MA. Models of trajectory formation and temporal interaction of reach and grasp. J Motor Behav. (1993) 25:175–92. doi: 10.1080/00222895.1993.9942048
7. vanVliet PM, Sheridan MR. Coordination between reaching and grasping in patients with hemiparesis and normal subjects. Arch Phys Med Rehabil. (2007) 88:1325–31. doi: 10.1016/j.apmr.2007.06.769
8. Wallace SA, Weeks DL, Kelso JAS. Temporal constraints in reaching and grasping behaviour. Hum Mov Sci. (1990) 9:69–93. doi: 10.1016/0167-9457(90)90036-D
9. Castiello U, Bennett K, Chambers H. Reach to grasp: the response to a simultaneous perturbation of object position and size. Exp Brain Res. (1998) 120:31–40. doi: 10.1007/s002210050375
10. Vilaplana JM, Coronado JL. A neural network model for coordination of hand gesture during reach to grasp. Neural Netw. (2006) 19:12–30. doi: 10.1016/j.neunet.2005.07.014
11. Paulignan Y, Toni VG, Jeannerod M. Influence of object position and size on human prehension movements. Exp Brain Res. (1997) 114:226–34. doi: 10.1007/PL00005631
13. Kawato M. Internal models for motor control and trajectory planning. Curr Opin Neurobiol. (1999) 9:718–27. doi: 10.1016/S0959-4388(99)00028-8
14. Pelton TA, Wing AM, Fraser D, vanVliet P. Differential effects of parietal and cerebellar stroke in response to object location perturbation. Front Human Neurosci. (2015) 9:293. doi: 10.3389/fnhum.2015.00293
15. Thielmann GT, Dean CM, Gentile AM. Rehabilitation of reaching after stroke: task-related training versus progressive resisted exercise. Arch Phys Med Rehabil. (2004) 85:1613–8. doi: 10.1016/j.apmr.2004.01.028
16. Michaelsen SM, Jacobs S, Roby-Brami A, Levin MF. Compensation for distal impairments of grasping in adults with hemiparesis. Exp Brain Res. (2004) 157:162–73. doi: 10.1007/s00221-004-1829-x
17. Nowak A, Grefkes C, Dafotakis M, Kust J, Karbe H, Fink GR. Dexterity is impaired at both hands following unilateral subcortical middle cerebral artery stroke. Eur J Neurosci. (2007) 25:3173–84. doi: 10.1111/j.1460-9568.2007.05551.x
18. Lang CE, Wagner JM, Bastian AJ, Hu Q, Edwards DF, Sahrmann SA, et al. Deficits in grasp versus reach during acute hemiparesis. Exp Brain Res. (2005) 166:126–36. doi: 10.1007/s00221-005-2350-6
19. Lum PS, Mulroy S, Amdur RL, Requejo P, Prilutsky BI, Dromerick AW. Gains in upper extremity function after stroke via recovery or compensation: potential differential effects on amount of real-world limb use. Top Stroke Rehabil. (2009) 16:237–53. doi: 10.1310/tsr1604-237
20. Cirstea MC, Mitnitski AB, Feldman AG, Levin MF. Interjoint coordination dynamics during reaching in stroke. Exp Brain Res. (2003) 151:289–300. doi: 10.1007/s00221-003-1438-0
21. vanVliet PM, Sheridan MR. Ability to adjust reach extent in the hemiplegic arm. Physiotherapy. (2009) 95:176–84. doi: 10.1016/j.physio.2009.03.004
22. Cavina-Pratesi C, Ietswaart M, Humphreys GW, Lestou V, Milner AD. Impaired grasping in a patient with optic ataxia: primary visuomotor deficit or secondary consequence of misreaching? Neuropsychologia. (2010) 48:226–34. doi: 10.1016/j.neuropsychologia.2009.09.008
23. Frey SH, Vinton D, Norlund R, Grafton ST. Cortical topography of human anterior intraparietal cortex active during visually guided grasping. Brain Res Cogn Brain Res. (2005) 23:397–405. doi: 10.1016/j.cogbrainres.2004.11.010
24. Cavina-Pratesi C, Monaco S, Fattori P, Galletti C, McAdam TD, Quinlan DJ, et al. Functional magnetic resonance imaging reveals the neural substrates of arm transport and grip formation in reach-to-grasp actions in humans. J Neurosci. (2010) 30:10306–23. doi: 10.1523/JNEUROSCI.2023-10.2010
25. Culham JC, Cavina-Pratesi C, Singhal A. The role of parietal cortex in visuomotor control: what have we learned from neuroimaging? Neuropsychologia. (2006) 44:2668–84. doi: 10.1016/j.neuropsychologia.2005.11.003
26. Messier J, Kalaska JF. Covariation of primate dorsal premotor cell activity with direction and amplitude during a memorized-delay reaching task. J Neurophysiol. (2000) 84:152–65. doi: 10.1152/jn.2000.84.1.152
27. Fattori P, Breveglieri R, Marzocchi N, Filippini D, Bosco A, Galleti C. Hand orientation during reach-to-grasp movements modulates neuronal activity in the medial posterior parietal area V6. J Neurosci. (2009) 29:1928–36. doi: 10.1523/JNEUROSCI.4998-08.2009
28. Merians AS, Poizner H, Boian R, Burdea G, Adamovich S. Sensorimotor training in a virtual reality environment: does it improve functional recovery poststroke? Neurorehabil Neural Repair. (2006) 20:252–67. doi: 10.1177/1545968306286914
29. Binkofsky F, Dohle C, Posse S, Stephan KM, Hefter H, Seitz RJ, et al. Human anterior intrapareital area subserves prehension. A combined lesion and functional magnetic resonance imaging activation study. Neurology. (1998) 50:1253–9. doi: 10.1212/WNL.50.5.1253
30. Rand MK, Shimansky Y, Stelmach GE. Effects of accuracy constraints on reach-to-grasp movements in cerebellar patients. Exp Brain Res. (2000) 135:179–88. doi: 10.1007/s002210000528
31. Topka H, Konczak J, Dichgans J. Coordination of mulitjoint arm movements in cerebellar ataxia: analysis of hand and angular kinematics. Exp Brain Res. (1998) 119:483–92. doi: 10.1007/s002210050364
32. Wenzelburger R, Kopper F, Frenzel A, Stolze H, Klebe S, Brossman A, et al. Hand coordination following capsular stroke. Brain. (2005) 128:64–74. doi: 10.1093/brain/awh317
33. vanVliet P, Carey L, Nilsson M. Targeting stroke treatment to the individual. Int J Stroke. (2012) 7:480–1. doi: 10.1111/j.1747-4949.2012.00867.x
34. Culham JC. Functional neuroimaging: experimental design and analysis. In: Cabeza AKR, editor. Handbook of Functional Neuroimaging of Cognition. Cambridge, MA: MIT Press (2006). p. 53–82.
35. VanderLee JH, DeGroot V, Beckerman H, Wagenaar R, Lankhorst G, Bouter L. The intra- and interater reliability of the Action Research Arm Test: a practical test of upper extermity function in patients with stroke. Arch Phys Med Rehabil. (2001) 82:14–9. doi: 10.1053/apmr.2001.18668
36. Morris DM, Uswatte G, Crago JE, Cook EW, Taub E. The reliability of the Wolf Motor Function Test for assessing upper extremity function after stroke. Arch Phys Med Rehabil. (2001) 82:750–5. doi: 10.1053/apmr.2001.23183
37. Stolk-Hornsveld F, Crow JL, Hendriks EP, vanderBaan R, Harmeling-vanderWel BC. The Erasmus MC modifications to the (revised) Nottingham Sensory Assessment: a reliable somatosensory assessment measure for patients with intracranial disorders. Clin Rehabil. (2006) 20:160–72. doi: 10.1191/0269215506cr932oa
38. Melzack R. The short-form McGill pain questionnaire. Pain. (1987) 30:191–7. doi: 10.1016/0304-3959(87)91074-8
39. Patrick E, Ada L. The Tardieu Scale differentiates contracture from spasticity whereas the Ashworth Scale is confounded by it. Clin Rehabil. (2006) 20:173–82. doi: 10.1191/0269215506cr922oa
40. Manly T. Assessment of unilateral spatial neglect: Scoring star cancellation performance from video recording- method, reliability, benefits, and normative data. Neuropsychology. (2009) 23:519–28. doi: 10.1037/a0015413
41. Cockrell J, Folstein M. Mini-mental state examination (MMSE). Psychopharmacol Bull. (1988) 24:689–92.
42. Duncan P. The stroke impact scale version 2.0, evaluation of reliability, validity and sensitivity to change. Stroke. (1999) 30:2131–40. doi: 10.1161/01.STR.30.10.2131
43. Mathiowetz V, Volland G, Kashman N, Wever K. Adult norms for the Box and Block test of manual dexterity. Am J Occup Ther. (1985) 39:386–91. doi: 10.5014/ajot.39.6.386
44. Nowak DA. The impact of stroke on the performance of grasping: usefulness of kinetic and kinematic motion analysis. Neurosci Biobehav Rev. (2008) 32:1439–50. doi: 10.1016/j.neubiorev.2008.05.021
45. Streiner DL, Norman GR. Health Measurement Scales: A Practical Guide to Their Development and Use. New York, NY: Oxford University Press (2008).
46. Johnson RA, Wichern DW. Applied Multivariate Statistical Analysis. Englewood Cliffs, NJ: Prentice Hall (1992).
47. Archambault P, Pigeon P, Feldman AG, Levin MF. Recruitment and sequencing of different degrees of freedom during pointing movements involving the trunk in healthy and hemiparetic subjects. Exp Brain Res. (1999) 126:55–67. doi: 10.1007/s002210050716
48. Castiello U, Bennett KMB, Stelmach GE. Reach to grasp: the natural response to perturbation of object size. Exp Brain Res. (1993) 94:163–78. doi: 10.1007/BF00230479
49. Rand MK, Squire LM, Stelmach GE. Effect of speed manipulation on the control of aperture closure during reach to grasp movements. Exp Brain Res. (2006) 174:74–85. doi: 10.1007/s00221-006-0423-9
Keywords: stroke, upper limb, reaching, coordination, grasp
Citation: Broome K, Hudson I, Potter K, Kulk J, Dunn A, Arm J, Zeffiro T, Cooper G, Tian H and van Vliet P (2019) A Modified Reach-to-Grasp Task in a Supine Position Shows Coordination Between Elbow and Hand Movements After Stroke. Front. Neurol. 10:408. doi: 10.3389/fneur.2019.00408
Received: 31 January 2019; Accepted: 04 April 2019;
Published: 08 May 2019.
Edited by:
Emilia Michou, University of Manchester, United KingdomReviewed by:
Margit Alt Murphy, University of Gothenburg, SwedenCopyright © 2019 Broome, Hudson, Potter, Kulk, Dunn, Arm, Zeffiro, Cooper, Tian and van Vliet. This is an open-access article distributed under the terms of the Creative Commons Attribution License (CC BY). The use, distribution or reproduction in other forums is permitted, provided the original author(s) and the copyright owner(s) are credited and that the original publication in this journal is cited, in accordance with accepted academic practice. No use, distribution or reproduction is permitted which does not comply with these terms.
*Correspondence: Paulette van Vliet, UGF1bGV0dGUudmFudmxpZXRAbmV3Y2FzdGxlLmVkdS5hdQ==
Disclaimer: All claims expressed in this article are solely those of the authors and do not necessarily represent those of their affiliated organizations, or those of the publisher, the editors and the reviewers. Any product that may be evaluated in this article or claim that may be made by its manufacturer is not guaranteed or endorsed by the publisher.
Research integrity at Frontiers
Learn more about the work of our research integrity team to safeguard the quality of each article we publish.