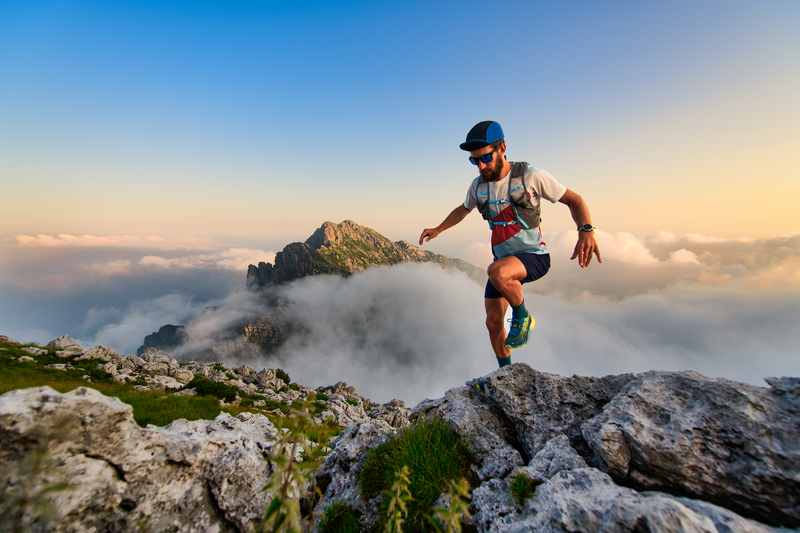
95% of researchers rate our articles as excellent or good
Learn more about the work of our research integrity team to safeguard the quality of each article we publish.
Find out more
REVIEW article
Front. Neurol. , 26 March 2019
Sec. Pediatric Neurology
Volume 10 - 2019 | https://doi.org/10.3389/fneur.2019.00236
This article is part of the Research Topic Pediatric Neurology Editor's Pick 2021 View all 10 articles
Virtual-reality-based training can influence gait recovery in children with cerebral palsy. A consensus concerning its influence on spatiotemporal gait parameters and effective training dosage is still warranted. This study analyzes the influence of virtual-reality training (relevant training dosage) on gait recovery in children with cerebral palsy. A search was performed by two reviewers according to Preferred Reporting Items for Systematic Reviews and Meta-Analyses (PRISMA) guidelines on nine databases: PEDro, EBSCO, PubMed, Cochrane, Web of Science, EMBASE, ICI, Scopus, and PROQUEST. Of 989 records, 16 studies involving a total of 274 children with cerebral palsy met our inclusion criteria. Eighty-eight percent of the studies reported significant enhancements in gait performance after training with virtual reality. Meta-analyses revealed positive effects of virtual-reality training on gait velocity (Hedge's g = 0.68), stride length (0.30), cadence (0.66), and gross motor function measure (0.44). Subgroup analysis reported a training duration of 20–30 min per session, ≤4 times per week across ≥8 weeks to allow maximum enhancements in gait velocity. This study provides preliminary evidence for the beneficial influence of virtual-reality training in gait rehabilitation for children with cerebral palsy.
Gait dysfunctions are prominent in children with cerebral palsy (1, 2). Reduction in gait velocity, cadence and stride length are common spatiotemporal gait characteristics exhibited by children with cerebral palsy (2). Recent experimental and review studies have reported the beneficial influence of virtual-reality training strategies to considerably influence gait performance in children with cerebral palsy (3, 4). According to Aminov et al. (5), virtual reality is a superior rehabilitative approach when compared with conventional therapeutic approaches. The authors suggest that this strategy can allow a patient to (re)learn motor skills while interacting with real-life scenarios in an ecological yet patient-centric manner (6).
The application of this intervention is dynamic as it allows real-time “multisensory” feedback of executed movement to both the performer and the medical practitioner. This further can simultaneously facilitate the motor planning and perception of the performer and allow the medical practitioner to monitor and control the complexity of the virtual-reality task/environment according to each performer's capability (7). Several underlying mechanisms through which virtual-reality training can facilitate motor rehabilitation have been reported. For instance, amplification of sensorimotor representation by augmented sensory feedback (8–12), enhancement of error feedback (13), reduction of cognitive load (14–17), reduction of musculoskeletal coactivation (18), increased arousal (19), and motivation (20) are few of the reasons by which virtual-reality training might enhance gait recovery (3, 4, 21). Moreover, neuroimaging studies have reported that training with virtual reality can facilitate recovery by instigating cortical reorganization (22) and neural plasticity (23, 24), thus suggesting a strong potential for virtual-reality-based training for recovering gait in children with cerebral palsy.
Recent systematic reviews have reported the beneficial effects of virtual-reality-based training on gait performance in children with cerebral palsy (3, 4). However, to the best of our knowledge, only one study has elucidated the influence of virtual-reality training on gait performance in children with cerebral palsy statistically, i.e., a meta-analysis (3). Chen et al. (3) performed a meta-analysis on eight studies and reported a positive effect size of 0.75 (0.34–1.16) on the ambulation function after training with virtual reality. Although the findings of this study are in line with previous reviews, there were certain limitations. Firstly, the authors did not explore the cause of heterogeneity observed in the analysis, i.e., I2 = 59%. Secondly, the authors did not describe the specific variables evaluated in the ambulation function, i.e., no information was provided as to what these enhancements were applicable on, for instance, gait velocity, stride length, etc. Thirdly, the authors included some studies in the analysis that, on re-evaluation, were found to not have evaluated any gait parameter at all.
In the present systematic review and meta-analysis, our aim is to develop a state of evidence defining the influence of virtual-reality training on spatiotemporal gait parameters in children with cerebral palsy. Moreover, the importance of determining training dosages in neurological rehabilitation has been emphasized in several studies (25–31). Therefore, as a secondary objective, this present review also aims to elucidate effective training dosages for virtual-reality-based gait training that could be incorporated by medical practitioners during gait rehabilitation for children with cerebral palsy.
This review and meta-analysis was performed according to PRISMA guidelines (32). A systematic search of literature was performed across nine academic databases. The inclusion criteria were as follows: (i) Randomized controlled trials (RCTs) and Controlled clinical trials (CCTs), (ii) virtual-reality interventions (any training duration and setting), (iii) spatiotemporal gait parameters evaluated, (iv) gross motor function and/or performance measure evaluated, (v) ≥4 PEDro score, (vi) children with cerebral palsy (age range: 6–18 years), (vii) peer-reviewed publications, and (viii) in English, German, Hindi, Punjabi, and Sanskrit languages. For a detailed method section, see the Supplementary Material.
The quality of the reviewed studies was assessed using the PEDro scale by both the reviewers (33).
A level of evidence analysis, i.e., strength of recommendation, was assigned to each outcome measure, i.e., gait velocity, stride length, cadence, stride width, and gross motor function measure. This assessment was combinedly based on the methodological quality and design of the evaluated studies (34).
A within-group, i.e., pre–post meta-analysis, approach was performed to develop a better quantitative interpretation of the virtual-reality intervention (35). The meta-analyses were conducted using CMA (Comprehensive meta-analysis V 2.0, USA). The meta-analyses evaluated the influence of virtual-reality training on gait velocity, cadence, stride length, stride width, and gross motor function measure score. An analysis for publication bias was performed by Duval and Tweedie's trim and fill procedure (36). The alpha level was set at 5%.
The initial search across the nine academic databases yielded a total of 989 studies, which, upon implementing the inclusion criteria, were reduced to 16 (Figure 1). Thereafter, both qualitative and quantitative data were extracted from all the studies (Supplementary Table 2). Of the 16 included studies, 5 were randomized controlled trials and 11 were controlled clinical trials.
Figure 1. PRISMA flowchart for the inclusion of studies (32).
A total of 274 participants were analyzed in the 16 incorporated studies. The included studies provided data on 120 females and 154 males. Descriptive statistics relating to the age (mean ± standard deviation, range) have been mentioned in Supplementary Table 3. In the included studies, three studies did not define the gender distribution (37–39).
Individual scores attained by the studies using the PEDro scale for each factor have been mentioned (Supplementary Table 4). The average PEDro score of the 18 included studies was computed to be (M ± S.D). 5.7 ± 1.4 out of 10, indicating, on average, a “good” quality of the studies. Here, one study scored 9 (40), one study scored 8 (41), three scored 7 (39, 42, 43), two scored 6 (44, 45), six scored 5 (37, 46–50), and three studies scored 4 (38, 51, 52). The risk of biasing across the studies has been illustrated in Figure 2. Individual scoring by the studies on each parameter has been mentioned in Supplementary Table 3.
In this present study, publication bias was analyzed by Duval and Tweedie's trim and fill method. The graph plots the evaluated weighted effect size, i.e., Hedge's g values against standard error (Figure 3). Here, the absence of publication bias is determined by symmetrical distribution of the studies about the combined effect size. The trim and fill test elucidated any missing studies based on a fixed effect model in the present analysis. However, the method suggests that no studies are missing. Under the random effects model, the point estimate is 0.48 and the 95% confidence interval (CI) is 0.26–0.71 for the combined studies. Using trim and fill, the imputed point estimate is 0.66 and the 95% CI is 0.43–0.89.
Figure 3. Trim and fill funnel plot for Hedge's g and standardized effect for each value in the meta-analysis. Each of the effect is represented in the plot as a circle. Funnel boundaries represent area where 95% of the effects are expected to lie if there were no publication biases. The vertical line represents the mean standardized effect of zero.
The analysis of level of evidence based on evidence-based nursing care guidelines revealed a “III Level of Evidence,” supporting the beneficial effects of virtual-reality training on gait and motor performance in children with cerebral palsy. This level of evidence was awarded to all the evaluated parameters and dose–response subgroup analyses. The appraisal of level of evidence score was based on the design and scoring of the included studies, i.e., controlled clinical trials.
The current qualitative and quantitative evidence from the review suggests the beneficial effects of virtual-reality training on spatiotemporal gait parameters for children with cerebral palsy. Nine included studies reported significant enhancement in gait performance for children with cerebral palsy after virtual-reality training. Two studies reported no influence of virtual-reality training gait on spatiotemporal gait parameters (see Supplementary Table 3) (48, 50).
Gait velocity was assessed among 13 studies. Additional data were extracted from one study (40). The analysis of studies revealed (Figure 4) a medium effect size in the positive domain (g = 0.68, 95% CI: 0.35 to 1.01) with negligible heterogeneity (I2 = 13.1%, p > 0.05). In the included studies, only one study did not utilize a training intervention with virtual reality (50). Therefore, in a subsequent analysis, we removed this study and reperformed the analysis to elucidate the influence of virtual-reality training on gait velocity. The analysis of studies revealed (Supplementary Figure 1) a large effect size in the positive domain (g = 0.76, 95% CI: 0.44 to 1.07) with negligible heterogeneity (I2 = 10.7%, p > 0.05).
Figure 4. Forest plot illustrating individual studies evaluating the effects of virtual-reality training on gait velocity among children with cerebral palsy. Weighted effect sizes, Hedge's g (boxes), and 95% CI (whiskers) are presented, demonstrating repositioning errors for individual studies. The (Diamond) represents pooled effect sizes and 95% CI.
20–30 min: A subgroup analysis was performed on nine studies. The analysis revealed a large effect size (Supplementary Figure 2) in the positive domain (g = 0.88, 95% CI: 0.51 to 1.24) with negligible heterogeneity (I2 = 12.1%, p > 0.05).
40–45 min: A subgroup analysis was performed on three studies. The analysis revealed a medium effect size (Supplementary Figure 3) in the positive domain (g = 0.26, 95% CI: −0.24 to 0.77) with no heterogeneity (I2 = 0%, p > 0.05).
≤4 sessions per week: A subgroup analysis was performed on six studies. The analysis revealed a large effect size (Supplementary Figure 4) in the positive domain (g = 0.78, 95% CI: 0.09 to 1.47) with negligible heterogeneity (I2 = 1.4%, p > 0.05).
≥5 sessions per week: A subgroup analysis was performed on six studies. The analysis revealed a medium effect size (Supplementary Figure 5) in the positive domain (g = 0.69, 95% CI: 0.42 to 0.97) with negligible heterogeneity (I2 = 2.4%, p > 0.05).
≥8 weeks: A subgroup analysis was performed on four studies. The analysis revealed a medium effect size (Supplementary Figure 6) in the positive domain (g = 0.69, 95% CI: 0.25 to 1.13) with negligible heterogeneity (I2 = 2.0%, p > 0.05).
≤7 weeks: A subgroup analysis was performed on eight studies. The analysis revealed a medium effect size (Supplementary Figure 7) in the positive domain (g = 0.65, 95% CI: 0.35 to 0.94) with no heterogeneity (I2 = 0.69%, p > 0.05).
Stride length was assessed among four studies. Additional data were extracted from one study (40). The analysis of the studies revealed (Figure 5) a medium effect size in the positive domain (g = 0.30, 95% CI: −0.40 to 1.01) with no heterogeneity (I2 = 0%, p > 0.05). In the included studies, only one study did not utilize a training intervention with virtual reality (50). Therefore, in a subsequent analysis, we removed this study and reperformed the analysis to elucidate the influence of virtual-reality training on stride length. The analysis of studies revealed (Supplementary Figure 8) a medium effect size in the positive domain (g = 0.50, 95% CI: −0.20 to 1.24) with no heterogeneity (I2 = 0%, p > 0.05).
Figure 5. Forest plot illustrating individual studies evaluating the effects of virtual-reality training on stride length among children with cerebral palsy. Weighted effect sizes, Hedge's g (boxes), and 95% CI (whiskers) are presented, demonstrating repositioning errors for individual studies. The (diamond) represents pooled effect sizes and 95% CI.
Cadence was assessed among two studies. Additional data were extracted from one study (40). The analysis of studies revealed (Supplementary Figure 9) a medium effect size in the positive domain (g = 0.66, 95% CI: −0.52 to 1.84) with negligible heterogeneity (I2 = 10.8%, p > 0.05).
Stride width was assessed among three studies. Additional data were extracted from one study (40). The analysis of studies revealed (Figure 6) a small effect size in the negative domain (g = −0.07, 95% CI: −0.57 to 0.43) with negligible heterogeneity (I2 = 4.5%, p > 0.05). In the included studies, only one study did not utilize a training intervention with virtual reality (50). Therefore, in a subsequent analysis, we removed this study and reperformed the analysis to elucidate the influence of virtual-reality training on stride width. The analysis of studies revealed (Supplementary Figure 10) a small effect size in the negative domain (g = −0.23, 95% CI: −0.53 to 0.06) with moderate heterogeneity (I2 = 0%, p > 0.05).
Figure 6. Forest plot illustrating individual studies evaluating the effects of virtual-reality training on stride width among children with cerebral palsy. Weighted effect sizes, Hedge's g (boxes), and 95% CI (whiskers) are presented, demonstrating repositioning errors for individual studies. The (diamond) represents pooled effect sizes and 95% CI.
Gross motor function measure was assessed among six studies. The analysis of studies revealed (Figure 7) a medium effect size in the positive domain (g = 0.44, 95% CI: 0.06 to 0.83) with negligible heterogeneity (I2 = 0.54%, p > 0.05).
Figure 7. Forest plot illustrating individual studies evaluating the effects of virtual-reality training on gross motor function measure among children with cerebral palsy. Weighted effect sizes, Hedge's g (boxes), and 95% CI (whiskers) are presented, demonstrating repositioning errors for individual studies. The (diamond) represents pooled effect sizes and 95% CI.
20–30 min: A subgroup analysis was performed on four studies. The analysis revealed a medium effect size (Supplementary Figure 11) in the positive domain (g = 0.56, 95% CI: 0.05 to 1.07) with negligible heterogeneity (I2 = 0.08%, p > 0.05).
40–45 min: A subgroup analysis was performed on two studies. The analysis revealed a small effect size (Supplementary Figure 12) in the positive domain (g = 0.14, 95% CI: −0.50 to 0.78) with no heterogeneity (I2 = 0%, p > 0.05).
The primary objective of this present systematic review and meta-analysis was to synthesize the current state of knowledge to determine the effects of virtual-reality training on spatiotemporal gait parameters in children with cerebral palsy. The findings from the current meta-analyses suggest a positive influence of virtual-reality training to enhance gait performance. Spatiotemporal parameters, i.e., gait velocity, cadence, and stride length, which are usually adversely affected in cerebral palsy (53), were enhanced after training with virtual reality, i.e., gait velocity (g = 0.76), stride length (g = 0.76), and cadence (g = 0.80).
Studies have suggested that virtual-reality training can facilitate motor performance by providing a performer with real-time “multisensory” feedback of the executed movement (19, 54, 55). Children with cerebral palsy have been reportedly associated with substantial deficits in sensory perception, which might affect their motor planning and performance (56). Here, the addition of different sensory modalities, for instance, auditory, visual, and proprioceptive feedback, could provide a “sensory deficit” patient with enriched knowledge of performance (movement amplitudes, relative limb position) and result (4, 19, 57–59).
Additionally, the enhancements in spatiotemporal gait parameters could be attributed to substantial changes in force, power, and kinematics at the ankle and knee joints (60). According to Chen et al. (46), virtual-reality-based training can substantially enhance isokinetic muscle strength and the amount of physical activity performed by children with cerebral palsy. This was also demonstrated in our analysis where gross motor function measure was enhanced (0.45) after training with virtual reality. In terms of movement kinematics, we presume that the explicit multisensory (i.e., visual–auditory–proprioceptive) feedback concerning the movement execution within the virtual environment could have allowed the patients to specifically time and control their movement patterns [see guidance hypothesis (61, 62)], thereby promoting a smooth movement pattern with reduced musculoskeletal co-contraction (18). In this review, several studies reported enhancement in gait kinematic scores (45, 50, 63, 64), which usually are adversely affected in children with cerebral palsy.
Furthermore, the patient-centered, closed-loop [tailored difficulty progression (65)] approach of virtual-reality training could be an additional reason for the superior influence of this rehabilitation intervention as compared to conventional approaches like resistance training (66), rhythmic auditory cueing (67), and robot-assisted training (68). Here, linking the individual performance measures concerning the motor restrictions and cognitive performance with the adaptive modulation of the task to be trained can provide adaptive mechanics in the virtual environment that might facilitate neuroplasticity (69). For instance, Xiao et al. (22) in a neuroimaging study reported the beneficial influence of virtual-reality-based training on motor planning and execution centers. The authors reported enhanced activations in primary, secondary motor, sensorimotor, and premotor cortices in stroke patients after virtual-reality training [also see (19, 70)]. Interestingly, the authors also reported hyperactivity in the ipsilesional somatosensory cortex with virtual-reality training (22). This enhanced activation in the somatosensory cortex of the affected hemisphere could be interpreted as unmasking of (pre)existent movement patterns (functional recovery via motor relearning) (70, 71).
Finally, we also elucidated specific virtual-reality training dosages that could be beneficially incorporated to attain maximum benefits in gait recovery in children with cerebral palsy. Fluet and Deutsch (72) had previously emphasized future studies for deducing training dosages in neurorehabilitation. The authors hypothesized that larger training dosages might account for more enhancements in motor recovery. However, as per the current findings of this meta-analysis, this was not the case. In terms of the length of training sessions, higher increments in spatiotemporal gait parameters were noted during training interventions lasting for 20–30 min as compared to 40–45 min of training (gait velocity: 0.88 vs. 0.26, gross motor function test: 0.56 vs. 0.14). Likewise, similar increments were noted for the number of sessions per week, i.e., ≤4 sessions allowed higher increments in gait velocity as compared to ≥5 weeks (0.84 vs. 0.65). In terms of the number of weeks of training, more number of weeks was observed to allow a greater influence on training, i.e., ≥8 weeks allowed better performance as compared to ≤7 weeks of training (0.83 vs. 0.65).
Two major limitations persisted in the present review. First, this study was not pre-registered in a prospective register for systematic reviews, such as PROSPERO. Second, a dose–response meta-analysis was performed for some variables with very few number of studies. This could raise concerns regarding the reliability of some outcome measures, i.e., incurring a type II error. We strongly recommend the reader to interpret the results with caution. Nevertheless, our findings are in line with previously published “high-quality” systematic reviews and meta-analyses that report positive or negligible effects of virtual-reality training on gait performance in children with cerebral palsy (3, 4). However, this present review extends the findings of these studies due to several reasons. Firstly, the present review incorporates a higher number of experimental studies that support our conclusion, i.e., 16 studies (274 participants) as compared to previously published review studies (3, 4). This large difference in the number of included studies could be attributed to the higher number of relevant academic databases searched (with multiple languages), i.e., nine, and the inclusion of controlled clinical trials. Secondly, this current review presents robust evidence to suggest training dosage with virtual reality for allowing enhancements in gait velocity and gross motor function test. Thirdly, this study provides evidence for the beneficial effects of virtual-reality training on generalized physical activity and motor output. It is important for the reader to consider that it is not our intention to disregard previously published reviews and meta-analyses. These reviews have addressed different factors for individuals with cerebral palsy (i.e., quality of life, arm recovery, and postural control), which was not the objective of the present review. Therefore, in our opinion, interpretations should be drawn simultaneously from all the reviews to develop a better understanding of the influence of virtual-reality-based training strategies for gait recovery after cerebral palsy. A III Level of Evidence supported the beneficial effects of virtual-reality-based training on gait performance. This weak level of evidence strongly warrants the need for multiple, high-quality, multicentered, randomized controlled trials to support the application of virtual-reality training on gait performance in children with cerebral palsy.
In conclusion, virtual-reality training facilitates gait performance in children with cerebral palsy. The present study reports the influence of virtual-reality-based training on the most commonly evaluated spatiotemporal gait outcomes; this shall allow the clinicians to better interpret the results with previous studies and other interventions and deduce practical implications for the benefit of children with cerebral palsy. The review, based on limited state of evidence, i.e., III Level of Evidence, suggests a training duration of at least 20–30 min, ≤4 times per week across ≥8 weeks.
SG conceptualized the study, carried out the systematic-review, statistical analysis, and wrote the paper. IG assisted in the systematic-review process and reviewed the manuscript.
IG was employed by Rsgbiogen.
The remaining author declares that the research was conducted in the absence of any commercial or financial relationships that could be construed as a potential conflict of interest.
The Supplementary Material for this article can be found online at: https://www.frontiersin.org/articles/10.3389/fneur.2019.00236/full#supplementary-material
1. Armand S, Decoulon G, Bonnefoy-Mazure A. Gait analysis in children with cerebral palsy. EFORT Open Rev. (2016) 1:448–60. doi: 10.1302/2058-5241.1.000052
2. Marshall S, Teasell R, Bayona N, Lippert C, Chundamala J, Villamere J, et al. Motor impairment rehabilitation post acquired brain injury. Brain Inj. (2007) 21:133–60. doi: 10.1080/02699050701201383
3. Chen Y, Fanchiang HD, Howard A. Effectiveness of virtual reality in children with cerebral palsy: a systematic review and meta-analysis of randomized controlled trials. Phys Ther. (2018) 98:63–77. doi: 10.1093/ptj/pzx107
4. Porras DC, Siemonsma P, Inzelberg R, Zeilig G, Plotnik M. Advantages of virtual reality in the rehabilitation of balance and gait: systematic review. Neurology. (2018) 90:1017–25. doi: 10.1212/WNL.0000000000005603
5. Aminov A, Rogers JM, Middleton S, Caeyenberghs K, Wilson PH. What do randomized controlled trials say about virtual rehabilitation in stroke? A systematic literature review and meta-analysis of upper-limb and cognitive outcomes. J NeuroEng Rehabil. (2018) 15:29. doi: 10.1186/s12984-018-0370-2
6. Ghai S, Ghai I, Effenberg AO. “Low road” to rehabilitation: a perspective on subliminal sensory neuroprosthetics. Neuropsychiatr Dis Treat. (2018) 14:301–7. doi: 10.2147/NDT.S153392
7. Saposnik G, Levin M. Virtual reality in stroke rehabilitation: a meta-analysis and implications for clinicians. Stroke. (2011) 42:1380–6. doi: 10.1161/STROKEAHA.110.605451
8. Lamontagne A, Fung J, McFadyen BJ, Faubert J. Modulation of walking speed by changing optic flow in persons with stroke. J NeuroEng Rehabil. (2007) 4:22. doi: 10.1186/1743-0003-4-22
9. Ghai S, Ghai I. Effects of (music-based) rhythmic auditory cueing training on gait and posture post-stroke: a systematic review & dose-response meta-analysis. Sci Rep. (2019) 9:2183. doi: 10.1038/s41598-019-38723-3
10. Ghai S, Schmitz G, Hwang TH, Effenberg AO. Training proprioception with sound: effects of real-time auditory feedback on intermodal learning. Ann N Y Acad Sci. (2019) 1438:50–61. doi: 10.1111/nyas.13967
11. Ghai S, Schmitz G, Hwang TH, Effenberg AO. Auditory Proprioceptive Integration: effects of real-time kinematic auditory feedback on knee proprioception. Front Neurosci. (2018) 12:142. doi: 10.3389/fnins.2018.00142
12. Ghai S, Ghai I. Role of sonification and rhythmic auditory cueing for enhancing gait associated deficits induced by neurotoxic cancer therapies: a perspective on auditory neuroprosthetics. Front Neurol. (2019) 10:21. doi: 10.3389/fneur.2019.00021
13. White D, Burdick K, Fulk G, Searleman J, Carroll J. A virtual reality application for stroke patient rehabilitation. In: IEEE International Conference Mechatronics and Automation. Singapore (2005). p. 1081–1086.
14. Andersen SAW, Mikkelsen PT, Konge L, Cayé-Thomasen P, Sørensen MS. Cognitive load in distributed and massed practice in virtual reality mastoidectomy simulation. Laryngoscope. (2016) 126:E74–E79. doi: 10.1002/lary.25449
15. Ghai S, Ghai I, Effenberg AO. Effects of dual tasks and dual-task training on postural stability: a systematic review and meta-analysis. Clinical Intervent Aging. (2017) 12:557–77. doi: 10.2147/CIA.S125201
16. Ghai S. Proprioception and Performance: The Role of Below-Knee Compression Garments and Secondary Tasks. Thesis, Master of Sport and Leisure Studies (MSpLS), University of Waikato, Hamilton (2016). Retrieved from: https://hdl.handle.net/10289/10575
17. Ghai S. Merging of the Senses: Interactions Between Auditory and Proprioceptive Modalities. Hannover: Gottfreid Wilhelm Leibniz Universität Diss (2019).
18. Yoo JW, Lee DR, Sim YJ, You JH, Kim CJ. Effects of innovative virtual reality game and EMG biofeedback on neuromotor control in cerebral palsy. Biomed Mater Eng. (2014) 24:3613–8. doi: 10.3233/BME-141188
19. Cameirão MS, Si Badia B, Oller ED, Verschure PF. Neurorehabilitation using the virtual reality based Rehabilitation Gaming System: methodology, design, psychometrics, usability and validation. J NeuroEng Rehabil. (2010) 7:48. doi: 10.1186/1743-0003-7-48
20. Epure P, Holte MB. Analysis of motivation in virtual reality stroke rehabilitation. In: Interactivity, Game Creation, Design, Learning, and Innovation. Cham: Springer International Publishing (2018). p. 282–93. Available online at: https://www.springerprofessional.de/analysis-of-motivation-in-virtual-reality-stroke-rehabilitation/15514020
21. Ravi DK, Kumar N, Singhi P. Effectiveness of virtual reality rehabilitation for children and adolescents with cerebral palsy: an updated evidence-based systematic review. Physiotherapy. (2017) 103:245–58. doi: 10.1016/j.physio.2016.08.004
22. Xiao X, Lin Q, Lo W-L, Mao Y-R, Shi X-C, Cates RS, et al. Cerebral reorganization in subacute stroke survivors after virtual reality-based training: a preliminary study. Behav. Neurol. (2017) 2017:6261479. doi: 10.1155/2017/6261479
23. Berger CC, Gonzalez-Franco M, Tajadura-Jiménez A, Florencio D, Zhang Z. Generic HRTFs may be good enough in virtual reality. Improving source localization through cross-modal plasticity. Front Neurosci. (2018) 12:21. doi: 10.3389/fnins.2018.00021
24. Cheung KL, Tunik E, Adamovich SV, Boyd LA. Neuroplasticity and virtual reality. In: Weiss PL, Keshner EA, Levin MF, editors. Virtual Reality for Physical and Motor Rehabilitation. New York, NY: Springer New York (2014). p. 5–24.
25. Byl NN, Pitsch EA, Abrams GM. Functional outcomes can vary by dose: learning-based sensorimotor training for patients stable poststroke. NeuroRehabil Neural Repair. (2008) 22:494–504. doi: 10.1177/1545968308317431
26. Lang CE, Lohse KR, Birkenmeier RL. Dose and timing in neurorehabilitation: prescribing motor therapy after stroke. Curr Opin Neurol. (2015) 28:549–55. doi: 10.1097/WCO.0000000000000256
27. Ghai S. Effects of real-time (sonification) and rhythmic auditory stimuli on recovering arm function post stroke: a systematic review and meta-analysis. Front Neurol. (2018) 9:488. doi: 10.3389/fneur.2018.00488
28. Ghai S, Ghai I, Effenberg AO. Effect of rhythmic auditory cueing on aging gait: a systematic review and meta-analysis. Aging Dis. (2018) 9:901–23. doi: 10.14336/AD.2017.1031
29. Ghai S, Ghai I, Schmitz G, Effenberg AO. Effect of rhythmic auditory cueing on Parkinsonian gait: A systematic review and meta-analysis. Sci Rep. (2018) 8:506.
30. Ghai S, Ghai I. Effects of rhythmic auditory cueing in gait rehabilitation for multiple sclerosis: a mini systematic review and meta-analysis. Front Neurol. (2018) 9:386. doi: 10.3389/fneur.2018.00386
31. Ghai S, Ghai I. Virtual reality training enhances gait post-stroke: a training dose-response meta-analysis. Sci Rep. (In press).
32. Moher D, Liberati A, Tetzlaff J, Altman DG, Group P. Preferred reporting items for systematic reviews and meta-analyses: the PRISMA statement. PLoS Med. (2009) 6:e1000097. doi: 10.1371/journal.pmed.1000097
33. de Morton NA. The PEDro scale is a valid measure of the methodological quality of clinical trials: a demographic study. Aust J Physiother. (2009) 55:129–33. doi: 10.1016/S0004-9514(09)70043-1
34. Ackley BJ. Evidence-Based Nursing Care Guidelines: Medical-Surgical Interventions. Elsevier Health Sciences (2008). Available online at: https://www.elsevier.com/books/evidence-based-nursing-care-guidelines/ackley/978-0-323-04624-4
35. Borenstein M, Hedges LV, Higgins J, Rothstein HR. A basic introduction to fixed-effect and random-effects models for meta-analysis. Res Synth Methods. (2010) 1:97–111. doi: 10.1002/jrsm.12
36. Sue D, Richard T. Trim and fill: a simple funnel-plot–based method of testing and adjusting for publication bias in meta-analysis. Biometrics. (2000) 56:455–63. doi: 10.1111/j.0006-341X.2000.00455.x
37. AlSaif AA, Alsenany S. Effects of interactive games on motor performance in children with spastic cerebral palsy. J Phys Ther Sci. (2015) 27:2001–3. doi: 10.1589/jpts.27.2001
38. Brien M, Sveistrup H. An intensive virtual reality program improves functional balance and mobility of adolescents with cerebral palsy. Pediatr Phys Ther. (2011) 23:258–66. doi: 10.1097/PEP.0b013e318227ca0f
39. Cho C, Hwang W, Hwang S, Chung Y. Treadmill training with virtual reality improves gait, balance, and muscle strength in children with cerebral palsy. Tohoku J Exp Med. (2016) 238:213–8. doi: 10.1620/tjem.238.213
40. Collange Grecco LA, de Almeida Carvalho Duarte N, Mendonça ME, Galli M, Fregni F, Oliveira CS. Effects of anodal transcranial direct current stimulation combined with virtual reality for improving gait in children with spastic diparetic cerebral palsy: a pilot, randomized, controlled, double-blind, clinical trial. Clin Rehabil. (2015) 29:1212–23. doi: 10.1177/0269215514566997
41. Sajan JE, John JA, Grace P, Sabu SS, Tharion G. Wii-based interactive video games as a supplement to conventional therapy for rehabilitation of children with cerebral palsy: a pilot, randomized controlled trial. Dev Neurorehabil. (2017) 20:361–7. doi: 10.1080/17518423.2016.1252970
42. Mitchell LE, Ziviani J, Boyd RN. A randomized controlled trial of web-based training to increase activity in children with cerebral palsy. Dev Med Child Neurol. (2016) 58:767–73. doi: 10.1111/dmcn.13065
43. Tarakci D, Ersoz Huseyinsinoglu B, Tarakci E, Razak Ozdincler A. Effects of Nintendo Wii-Fit® video games on balance in children with mild cerebral palsy. Pediatr Int. (2016) 58:1042–50. doi: 10.1111/ped.12942
44. Curtis DJ, Bencke J, Mygind B. The effect of training in an interactive dynamic stander on ankle dorsiflexion and gross motor function in children with cerebral palsy. Dev Neurorehabil. (2014) 17:393–7. doi: 10.3109/17518423.2013.844738
45. Gagliardi C, Turconi AC, Biffi E, Maghini C, Marelli A, Cesareo A, et al. Immersive virtual reality to improve walking abilities in cerebral palsy: a pilot study. Ann Biomed Eng. (2018) 46:1376–84. doi: 10.1007/s10439-018-2039-1
46. Chen C-L, Chen C-Y, Liaw M-Y, Chung C-Y, Wang C-J, Hong W-H. Efficacy of home-based virtual cycling training on bone mineral density in ambulatory children with cerebral palsy. Osteoporosis Int. (2013) 24:1399–406. doi: 10.1007/s00198-012-2137-0
47. Chiu H-C, Ada L, Lee S-D. Balance and mobility training at home using Wii Fit in children with cerebral palsy: a feasibility study. BMJ Open. (2018) 8:e019624. doi: 10.1136/bmjopen-2017-019624
48. Levac D, McCormick A, Levin MF, Brien M, Mills R, Miller E, et al. Active video gaming for children with cerebral palsy: does a clinic-based virtual reality component offer an additive benefit? A pilot study. Phys Occup Ther Pediatr. (2018) 38:74–87. doi: 10.1080/01942638.2017.1287810
49. Luna-Oliva L, Ortiz-Gutiérrez RM, Cano-de la Cuerda R, Piédrola RM, Alguacil-Diego IM, Sánchez-Camarero C, et al. Kinect Xbox 360 as a therapeutic modality for children with cerebral palsy in a school environment: a preliminary study. NeuroRehabilitation. (2013) 33:513–21. doi: 10.3233/NRE-131001
50. van der Krogt MM, Sloot LH, Harlaar J. Overground versus self-paced treadmill walking in a virtual environment in children with cerebral palsy. Gait Posture. (2014) 40:587–93. doi: 10.1016/j.gaitpost.2014.07.003
51. Burdea GC, Cioi D, Kale A, Janes WE, Ross SA, Engsberg JR. Robotics and gaming to improve ankle strength, motor control, and function in children with cerebral palsy-a case study series. IEEE Trans Neural Syst Rehabil Eng. (2013) 21:165–73. doi: 10.1109/TNSRE.2012.2206055
52. Jung S-H, Song S-H, Kim S-D, Lee K, Lee G-C. Does virtual reality training using the Xbox Kinect have a positive effect on physical functioning in children with spastic cerebral palsy? A case series. J Pediatr Rehabil Med. (2018) 11:95–101. doi: 10.3233/PRM-160415
53. Beyaert C, Vasa R, Frykberg GE. Gait post-stroke: pathophysiology and rehabilitation strategies. Neurophysiol Clin/Clin Neurophysiol. (2015) 45:335–55. doi: 10.1016/j.neucli.2015.09.005
54. de Rooij IJ, van de Port IG, Meijer J-WG. Effect of virtual reality training on balance and gait ability in patients with stroke: systematic review and meta-analysis. Phys. Ther. (2016) 96:1905–18. doi: 10.2522/ptj.20160054
55. Laver KE, Lange B, George S, Deutsch JE, Saposnik G, Crotty M. Virtual reality for stroke rehabilitation. Cochrane Database Syst. Rev. (2017) 11:CD008349. doi: 10.1002/14651858.CD008349.pub4
56. Hoon AHJr, Stashinko EE, Nagae LM, Lin DD, Keller J, Bastian A, et al. Sensory and motor deficits in children with cerebral palsy born preterm correlate with diffusion tensor imaging abnormalities in thalamocortical pathways. Dev Med Child Neurol. (2009) 51:697–704. doi: 10.1111/j.1469-8749.2009.03306.x
57. Adamovich S, August K, Merians A, Tunik E. A virtual reality-based system integrated with fmri to study neural mechanisms of action observation-execution: a proof of concept study. Restor Neurol Neurosci. (2009) 27:209–23. doi: 10.3233/RNN-2009-0471
58. Ghai S, Driller M, Ghai I. Effects of joint stabilizers on proprioception and stability: a systematic review and meta-analysis. Phys Therapy Sport. (2017) 25:65–75. doi: 10.1016/j.ptsp.2016.05.006
59. Ghai S, Driller MW, Masters RSW. The influence of below-knee compression garments on knee-joint proprioception. Gait Posture. (2018) 60:258–61. doi: 10.1016/j.gaitpost.2016.08.008
60. Mirelman A, Patritti BL, Bonato P, Deutsch JE. Effects of virtual reality training on gait biomechanics of individuals post-stroke. Gait Posture. (2010) 31:433–7. doi: 10.1016/j.gaitpost.2010.01.016
61. Ronsse R, Puttemans V, Coxon JP, Goble DJ, Wagemans J, Wenderoth N, et al. Motor learning with augmented feedback: modality-dependent behavioral and neural consequences. Cerebral Cortex. (2011) 21:1283–94. doi: 10.1093/cercor/bhq209
62. Wierinck E, Puttemans V, Swinnen S, van Steenberghe D. Effect of augmented visual feedback from a virtual reality simulation system on manual dexterity training. Eur J Dent Educ. (2005) 9:10–6. doi: 10.1111/j.1600-0579.2004.00351.x
63. Beretta E, Romei M, Molteni E, Avantaggiato P, Strazzer S. Combined robotic-aided gait training and physical therapy improve functional abilities and hip kinematics during gait in children and adolescents with acquired brain injury. Brain Inj. (2015) 29:955–62. doi: 10.3109/02699052.2015.1005130
64. Biffi E, Beretta E, Cesareo A, Maghini C, Turconi AC, Reni G, et al. An immersive virtual reality platform to enhance walking ability of children with acquired brain injuries. Methods Inf Med. (2017) 56:119–26. doi: 10.3414/ME16-02-0020
65. Mishra J, Gazzaley A. Closed-loop rehabilitation of age-related cognitive disorders. Sem Neurol. (2014) 34:584–90. doi: 10.1055/s-0034-1396011
66. Moreau NG, Bodkin AW, Bjornson K, Hobbs A, Soileau M, Lahasky K. Effectiveness of rehabilitation interventions to improve gait speed in children with cerebral palsy: systematic review and meta-analysis. Phys Ther. (2016) 96:1938–54. doi: 10.2522/ptj.20150401
67. Ghai S, Ghai I, Effenberg AO. Effect of rhythmic auditory cueing on gait in cerebral palsy: a systematic review and meta-analysis. Neuropsychiatr Dis Treat. (2018) 14:43–59. doi: 10.2147/NDT.S148053
68. Carvalho I, Pinto SM, das Virgens Chagas D, dos Santos JLP, de Sousa Oliveira T, Batista LA. Robotic gait training for individuals with cerebral palsy: a systematic review and meta-analysis. Arch Phys Med Rehabil. (2017) 98:2332–44. doi: 10.1016/j.apmr.2017.06.018
69. Mishra J, Gazzaley A. Harnessing the neuroplastic potential of the human brain and the future of cognitive rehabilitation. Front Human Neurosci. (2014) 8:218. doi: 10.3389/fnhum.2014.00218
70. Saleh S, Bagce H, Qiu Q, Fluet G, Merians A, Adamovich S, et al. Mechanisms of neural reorganization in chronic stroke subjects after virtual reality training. In: Engineering in Medicine and Biology Society, EMBC, 2011 Annual International Conference of the IEEE. (IEEE). (2011). p. 8118–21.
71. Wang Z-R, Wang P, Xing L, Mei L-P, Zhao J, Zhang T. Leap Motion-based virtual reality training for improving motor functional recovery of upper limbs and neural reorganization in subacute stroke patients. Neural Regener Res. (2017) 12:1823–31. doi: 10.4103/1673-5374.219043
Keywords: cerebral palsy, gait, virtual reality, brain injury, rehabilitation
Citation: Ghai S and Ghai I (2019) Virtual Reality Enhances Gait in Cerebral Palsy: A Training Dose-Response Meta-Analysis. Front. Neurol. 10:236. doi: 10.3389/fneur.2019.00236
Received: 28 August 2018; Accepted: 22 February 2019;
Published: 26 March 2019.
Edited by:
Tomoki Arichi, King's College London, United KingdomReviewed by:
Ana Carolina Coan, Campinas State University, BrazilCopyright © 2019 Ghai and Ghai. This is an open-access article distributed under the terms of the Creative Commons Attribution License (CC BY). The use, distribution or reproduction in other forums is permitted, provided the original author(s) and the copyright owner(s) are credited and that the original publication in this journal is cited, in accordance with accepted academic practice. No use, distribution or reproduction is permitted which does not comply with these terms.
*Correspondence: Shashank Ghai, c2hhc2hhbmsuZ2hhaUBzcG9ydHdpc3MudW5pLWhhbm5vdmVyLmRl
Disclaimer: All claims expressed in this article are solely those of the authors and do not necessarily represent those of their affiliated organizations, or those of the publisher, the editors and the reviewers. Any product that may be evaluated in this article or claim that may be made by its manufacturer is not guaranteed or endorsed by the publisher.
Research integrity at Frontiers
Learn more about the work of our research integrity team to safeguard the quality of each article we publish.