- 1Department of Physiology, Universidade Federal de São Paulo, São Paulo, Brazil
- 2Harquail Centre for Neuromodulation, Sunnybrook Health Sciences Centre, University of Toronto, Toronto, ON, Canada
- 3Epilepsy Center, Neurological Institute, Cleveland Clinic, Cleveland, OH, United States
Mesial temporal lobe epilepsy (MTLE) caused by hippocampal sclerosis is one of the most frequent focal epilepsies in adults. It is characterized by focal seizures that begin in the hippocampus, sometimes spread to the insulo-perisylvian regions and may progress to secondary generalized seizures. Morphological alterations in hippocampal sclerosis are well defined. Among them, hippocampal sclerosis is characterized by prominent cell loss in the hilus and CA1, and abnormal mossy fiber sprouting (granular cell axons) into the dentate gyrus inner molecular layer. In this review, we highlight the role of mossy fiber sprouting in seizure generation and hippocampal excitability and discuss the response of alternative treatment strategies in terms of MFS and spontaneous recurrent seizures in models of TLE (temporal lobe epilepsy).
Introduction
Epilepsy is one of the most frequent neurological disorders, affecting more than 60 million people worldwide (1). It is a complex and disabling disease, with no cure and often without effective treatment (2). Current drug therapies are still not able to stop the disease or inhibit its development, but merely control convulsive seizures (3). The clinical hallmark of epilepsy is the occurrence of spontaneous recurrent seizures. It is estimated that up to 50% of all cases are triggered by “initial precipitating injuries,” such as status epilepticus (SE), stroke, and traumatic brain injury (TBI) (4, 5). Despite the huge number of preclinical and clinical studies, there is still a limited understanding of the basic mechanisms underlying epilepsy development or “epileptogenesis” (3).
Current epilepsy therapies rely on symptomatic strategies, either pharmacological or surgical, both aiming to suppress seizures, but do not target epileptogenesis (6). As the disease progresses, up to 30% of patients with epilepsy, particularly those with mesial temporal lobe epilepsy (MTLE) caused by hippocampal sclerosis, become pharmacoresistant, exhibiting medically intractable recurrent seizures (2). These patients are often amenable to surgical removal of the sclerotic structures (7). With this in mind, the rationale for the development of new antiepileptogenesis strategies, involving epilepsy prevention and seizure reduction (8, 9) might start with a better understanding of the pathophysiological mechanisms underlying epileptogenesis. In this review, (1) we describe the main histopathological findings of hippocampal sclerosis and their effect on the process of epileptogenesis, (2) we focus and explore the contribution of mossy fiber sprouting (MFS) to seizure generation and, (3) we discuss the possible role of MFS as a therapeutic target in MTLE.
MTLE and Histopathological Features in Hippocampus
Three major phases are involved in MTLE (9, 10): (i) acute injury (e.g., Status epilepticus, prolonged and complex febrile seizure, or traumatic brain injury); (ii) a latent phase; and (iii) a chronic phase, characterized by spontaneous recurrent seizures. The development of an epileptic condition and/or the progression of epilepsy is called epileptogenesis (11, 12). The hippocampal formation is highly susceptible to epileptic activity (13, 14) and is the site of seizure initiation in patients with MTLE (15–17). Clinical and experimental evidence demonstrated that the hippocampus plays a significant role in the pathogenesis of MTLE. In rodents (18–20) and non-human primate (21) models of temporal lobe epilepsy, status epilepticus (SE) induction results in hippocampal sclerosis, characterized by selective neuronal loss (22–26), astrogliosis (27–29), and inflammation (30–32). Within hours to days after the initial injury, hippocampal histopathological features include apoptosis (24, 33–36), dentate gyrus neurogenesis (37–42), the production of ectopic granule cells (43–47), and basal dendrites (48–51). Synaptic reorganization (52–54) and granule cell dispersion (55–58) are late features and their appearance may coincide with the onset of spontaneous seizures (59).
In humans, mortality associated with SE may be as high as 30% due to widespread neuronal damage (4) which is sparse after a spontaneous seizure (60). Similarly, in various animal models of TLE, cell death/damage or apoptosis markers were described along the different time lines of disease progression suggesting that, at least in SE models, the precipitating injury causes massive cell loss, while a spontaneous seizure leads to a variable level of injury (22, 36, 61, 62). However, whether hippocampal neuronal cell loss is the cause or consequence of a seizure, is a topic of discussion in epilepsy research [for review, see (63)]. Despite the reported controversies, a major challenge is to understand how the precipitating injury can produce long-lasting changes in neuronal circuitry and excitability. Different types of injuries may lead to epileptogenesis, sharing underlying mechanisms. Thus, the identification of common components may prevent the abnormal reorganization that transforms a normal brain into an epileptic brain. The search for such epileptogenesis pathways may provide the foundation for the development of novel antiepileptogenic and perhaps, preventive therapies.
There are two types of TLE: one that involves the mesial or internal structures of the temporal lobe; and one called neocortical temporal lobe epilepsy, which involves the outer portion of the temporal lobe. The most common is mesial temporal lobe epilepsy (MTLE), characterized on a pathological level by hippocampal sclerosis (HS) (7). HS is present in 30–45% of all epilepsy syndromes, while it is present in 56% of MTLE [for review, see (64)]. Several schemes have been proposed to classify subtypes of HS, mainly based on the subfield distribution, as well as the extent of hippocampal neuronal loss and gliosis, but a recent consensus classification system, validated by the neuropathology taskforce of the International League Against Epilepsy (ILAE), incorporated aspects of all previous schemes (65). This recent classification does not incorporate other frequent alterations as MFS and interneuron changes. ILAE type 1 HS (moderate to extensive neuronal loss and gliosis in CA1 > CA4, CA3 with sparing of CA2) has the highest seizure-free rate (70–85%) post-resective surgery at 2 years and is commonly associated with febrile seizures (50–76%) (64). About 30–40% of TLE patients present normal appearing hippocampi on magnetic resonance imaging (MRI) studies, with no or only mild neuronal loss on histological examination (66). Patients with no neuronal loss and no gliosis have a poorer postsurgical seizure-free outcome (42–58%) (64). With or without hippocampal sclerosis, patients investigated with MRI have shown that structural damage is not limited to the temporal lobe, with extension of damage to regions such as in the entorhinal cortex, parahippocampal, and fusiform gyrus, thalamus, basal ganglia, amygdala, and frontal and parietal lobe (66–68).
Hippocampal Sclerosis
Hippocampal sclerosis is characterized by intensive gliosis combined with a selective loss of neurons in the hippocampal formation. In the dentate gyrus, the loss of hilar inhibitory interneurons that project to the distal dendrites of granule cells, was hypothesized to produce a direct disinhibitory effect on granule cells (69–71). The loss of excitatory hilar mossy cells was also hypothesized to cause granule cell hyperexcitability (72). This would be indirectly related to the decreased excitation of surviving inhibitory basket cells, which are normally excited by mossy cells. Recently, these hypotheses were re-evaluated by using a transgenic mouse line with toxin-mediated mossy cell ablation. Using these mutant animals, investigators demonstrated that extensive ablation of mossy cells caused granule cell hyperexcitability, although the lack of mossy cells per se appeared insufficient to cause clinical epilepsy (73). Neuronal cell loss and gliosis affect other components of the hippocampus such as CA1 and CA3 pyramidal cells, in particular the loss of GABAergic interneurons (62), as well as of the limbic system, the amygdala, entorhinal, or perirhinal cortices (23, 61, 74). This phenomenon is also thought to contribute to the increased excitability of the epileptic hippocampus, possibly resulting in additional cell loss. Although multiple factors might be implicated in the genesis of hippocampal sclerosis, it is still not clear why some individuals are more likely to develop hippocampal sclerosis than others (7).
Astrogliosis
Astrogliosis is another prominent feature of epileptic foci evident in up to 90% of surgically resected epileptic hippocampi (75, 76). As shown in the kindling model, it may play a causal role in the development of seizures and the persistence of seizure disorders (28). Experimental evidence shows that reactive astrocytes are able to secrete molecules with pro-synaptogenic effects [for review, see (77)] that may support the observed neo-synaptogenesis during the latent period in models of epilepsy (78, 79). The expression of the astroglial-derived synaptogenic molecule thrombospondin 1 (TSP-1) is augmented in astrocytes following brain injury and seizures (80). While TSP-1 has the ability to induce excitatory synapses (81), gabapentin, an antagonist of TSP-1 receptor α2δ1, reduces the incidence of epileptiform discharge, and has neuroprotective effects probably achieved by suppressing the formation of excitatory synapses after trauma (77, 82).
Mossy Fiber Sprouting
In addition to hippocampal sclerosis and astrogliosis, the aberrant sprouting of granule cell axons, known as mossy fiber sprouting (MFS), is a frequent histopathological finding in TLE (83–85). Formation of MFS occurs in two phases: (1) the injury per se induces neuronal activity and the release of growth factors (86–88), and (2) the growth and extension of the granule cell axon (89–91). Morphologically, the hippocampal dentate gyrus contains three layers: molecular layer, granule cell layer, and polymorphic layer, also known as the hilus. This well-characterized pattern is conserved across mammalian species. The molecular layer is considered cell-free, as it contains the apical dendrites of granule cells and the excitatory terminals that convey information from either the entorhinal cortex (to the outer molecular layer) or from the commissural projections (to the inner molecular layer). The granule cell layer is densely packed with small diameter cell bodies (granule cells). The granule cell axons (also named mossy fibers) extend to the hilus and project to the excitatory interneurons (mossy cells) and to inhibitory interneurons before running through a narrow area called the stratum lucidum, to synapse onto CA3 pyramidal neurons (Figure 1A). Rarely, mossy fibers synapse onto other granule cells (92). The mossy cell axons project to contralateral granule cell dendrites in the inner molecular layer (associational pathway of the dentate gyrus or the commissural pathway in rodents) as well as to inhibitory basket cells, located within the granule cell layer. Thus, an excitatory input generated in the entorhinal cortex reaches the granule cells and extends to mossy cells, which in turn inhibit the granule cells. For a detailed description of hippocampal circuitry see Amaral et al. (93).
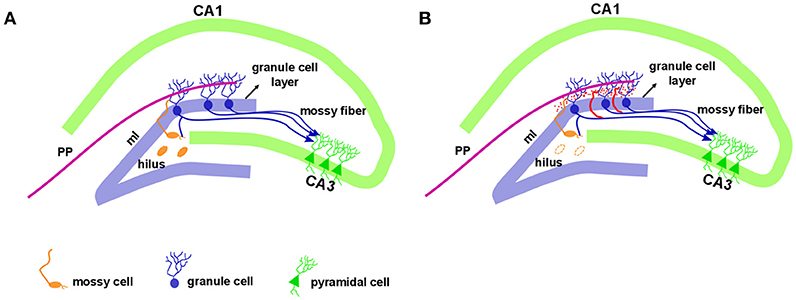
Figure 1. Hippocampal formation in the normal and epileptic brain. The dentate gyrus granule cell layer is densely packed with small diameter cell bodies named granule cells. Just above the granule cell layer is the molecular layer (ml) that is considered cell-free, as it contains the apical dendrites of granule cells. The outer molecular layer receives entorhinal cortex information via a performant pathway (PP). Granule cell axons, named mossy fibers, extend to the hilus with projection to the mossy cells and CA3 pyramidal neurons. The mossy cell axons project to contralateral granule cell dendrites in the inner molecular layer (A). In the epileptic hippocampus, with the loss of mossy fibers target in the hilus, the granule cell axons sprout and extensively innervate the dentate inner molecular layer of the hippocampus, a phenomenon called mossy fiber sprouting, illustrated in red (B).
While mossy fibers are hardly seen in the molecular layer of human non-MTLE resected hippocampal tissue, a robust density of mossy fibers containing zinc-rich terminals can be visualized with neo-Timm staining in MTLE specimen (94). Originally described in MTLE patients (94), the mossy fiber sprouting refers to an abnormal and extensive innervation of mossy fibers to the dentate inner molecular layer of the hippocampus (Figure 1B). Similar findings were soon demonstrated after kainate-induced seizures (95), and widely replicated in other animal models [for review, see (96)]. While the neo-Timm staining protocol is considered the gold standard to identify the aberrant zinc terminals in the inner molecular layer, in many animal models of TLE (red dots in the Figure 1B), recent imaging data has revealed that MFS can be tracked in vivo in longitudinal studies, by means of the manganese-enhanced signal in T1-weigthed images (97–100), allowing many possible correlational studies (Figure 2).
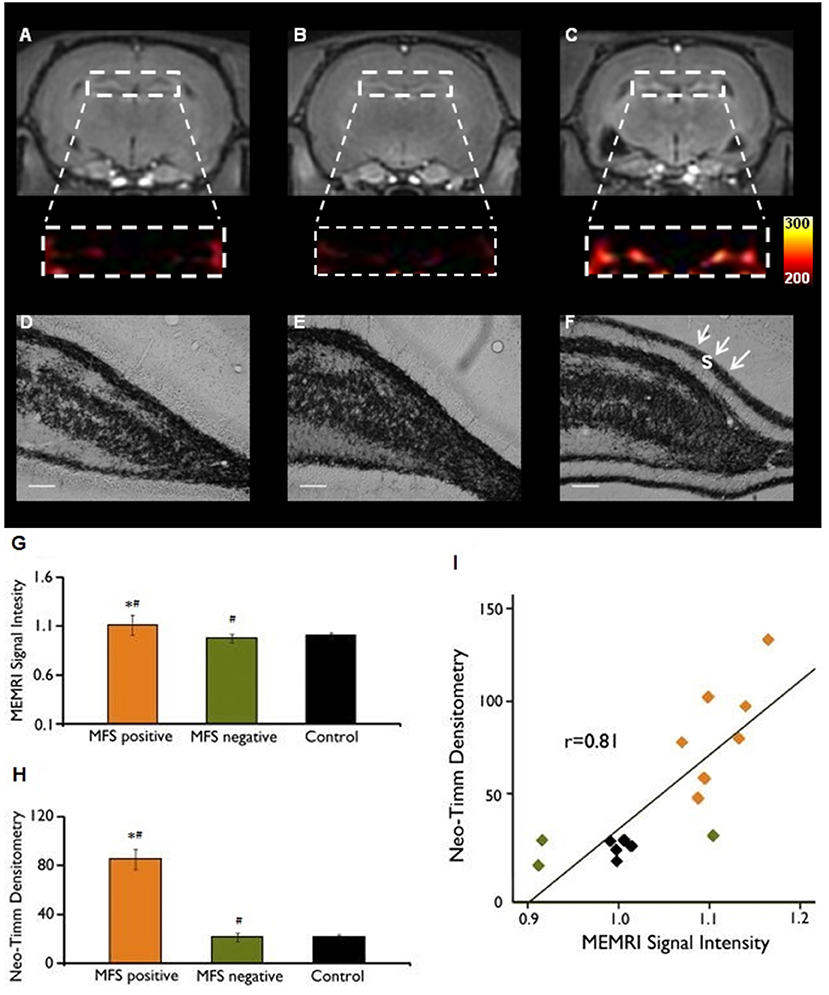
Figure 2. MEMRI and neo-Timm analyses for MFS positive and MFS negative animals. T1-weighted Manganese-enhanced MRI (MEMRI) (A–C) and neo-Timm staining (D–F) in Pilocarpine-treated animals. The protein synthesis inhibitor cycloheximide administered before SE onset was able to reduce the mossy fiber sprouting (A) at control levels (B) when compared with the Pilocarpine group (C). Differences were identified between MFS positive and MFS negative (#P < 0.05) and MFS positive and control animals (*P < 0.05) (G–H). Neo-Timm densitometry and MEMRI signal is strongly correlated (r = 0.81; P < 0,001) in the dispersion graph (I). s: supragranular mossy fiber sprouting (white arrows in F). Scale bars = 50 μm. Modified from our previous study (99).
The aberrant MFS initiates with the formation of new sprouts from mossy fibers within the dentate hilus, which is likely triggered by an injury-related increase in neuronal activity and the upregulation of brain-derived neurotrophic factor (86–88). Two distinctive mechanisms were proposed to contribute to the extension of mossy fiber sprouts to the inner molecular layer: the vacancy of synaptic sites (101) in granule cell proximal dendrites, caused by hilar cell loss after injury (70) and the downregulation of chemorepellents, such as Sema3A (102). Sema3A, normally secreted by entorhinal axons projecting to the dentate molecular layer, interacts via a receptor complex composed of neuropinlin-1 and plexinA present in dendrites of adult granular, hilar and pyramidal cells, suggesting that this signaling pathway is active in the hippocampal formation [for review see (103)], but is lost after status epilepticus. This suggests that the downregulation of chemorepellents, such as Sema3A, may act as a molecular element that facilitates the formation of recurrent projections of mossy fibers into the inner dentate molecular layer after status.
Originally described as a functional recurrent excitatory projection to dentate granule cells (95), caused by the loss of mossy fiber target cells in CA4 and CA3, the origin (104) and the role of MFS in MTLE has been disputed (105, 106). Hilar cell loss is an early finding in experimental models of TLE (107). However, there is no clear evidence that it is the specific loss of mossy cells and not the loss of other hilar interneurons, that triggers the MFS (108). Nowadays the topic of whether MFS is “epileptogenic” or “restorative” remains controversial. Although intrahippocampal circuit reorganization may be a cause of hippocampal epileptiform activity, recent studies indicate that sprouting is not necessarily associated with the occurrence of spontaneous seizures (109). The supportive evidence for both hypotheses is discussed below.
Mossy Fiber Sprouting: Excitatory or Inhibitory Role in Hippocampal Circuitry?
The evidence for a pro-epileptogenic role of MFS includes its presence in about 60% of patients with mesial temporal lobe epilepsy (94, 110) and animal models of hippocampal epilepsy (59, 111, 112). Re-assessed in the 1990's (113), the “mossy fiber sprouting hypothesis” holds that the increased excitability of dentate granule cells is the consequence of a pathological rearrangement of neuronal circuitry on which the excitatory granule cells innervate themselves, building up recurrent excitatory circuits. This hypothesis was supported by several lines of evidence, some described below. Electron microscopic studies show that sprouted mossy fiber terminals form asymmetric (excitatory) contacts with dendritic spines of granule cells (83). Electrophysiological evidence was obtained with perforant pathway stimulation (PPS). A single PPS in hippocampal slices of normal rats was able to produce an excitatory postsynaptic potential (EPSP) and a population action potential in the granule cells. If a second PPS is triggered 40 ms later, it evoked the EPSP but not the population action potential, possibly due to an inhibitory recurrent activation—defining the concept of a “gate” role for dentate granule cell layer. When the same experimental setup was performed in hippocampal slices of kainate-treated rats, the second stimulation evoked multiple populations of action potentials, indicating that granule cells became disinhibited and thus, hyperexcitable. These results were correlated with the presence of robust MFS in these slices, as revealed by Timm staining (95), suggesting that the aberrant sprouting of mossy fibers into the molecular layer could be associated with the loss of the dentate “gate.” Similar results were obtained in slices from kainate-treated animals, when antidromic stimulation of granule cells provoked seizure-like bursts of action potentials (111, 112). Important evidence of the pro- epileptogenic role of MFS was demonstrated in the kindling model, in which the density of sprouting increases with the number of induced seizures (114).
Aberrant MFS positively correlates with mossy cell loss in MTLE patients (115) and animal models (45, 116) but is it necessary and sufficient to generate seizures? Arguing against that view, evidence shows that MFS can be induced experimentally without seizures, after long-term potentiation (117), lesion of the perforant pathway (118, 119), or genetic mutation (120). Similarly, after electrical stimulation of the amygdala, some animals develop seizures but not MFS (106). In the prolonged febrile seizures model, MFS is well-developed 3 months after the initial injury, despite the absence of significant cell loss or increased dentate neurogenesis (121). In both the pilocarpine and kainate models, the presence and the intensity of MFS are positively correlated with higher T2 relaxation time values, number of spontaneous seizures, and the degree of cell loss in the granule cell layer, CA1 and CA3 pyramidal cell layer, but not in the hilus (116). This data is consistent with previous reports (122, 123) and indicates that although important, MFS may develop independently of mossy fiber target loss (124), it is present in animals with spontaneous seizures but its presence is not necessarily associated with the occurrence of spontaneous seizures (106).
Some data indicate that MFS is an active phenomenon, possibly a normal adaptive mechanism that becomes pathogenic (125, 126). Evidence that favors the idea that MFS is an active phenomenon came from studies using the mTOR pathway inhibitor, rapamycin. Rapamycin is an Akt (protein kinase B)-mTOR inhibitor, which is related to various neuronal functions, including synaptic plasticity, neurogenesis, and dendritic and axonal plasticity (127, 128). Treatment of epileptic animals with rapamycin for 2 months after SE onset reduced MFS by half. Later evaluations indicated that once the rapamycin treatment ended 2 months after SE, MFS resumed and became fully developed 6 months after SE (126–128). Another Akt inhibitor called perifosine, was used prior to SE induced by kainic acid (KA). Pretreatment with perifosine suppressed the KA-induced neuronal death and MFS, resulting in a decrease in spontaneous seizures (129). These data support the hypothesis that MFS, after the epileptogenesis process, may lead to, but it is not necessary for the formation of recurrent excitatory circuits in dentate granule cells.
Accordingly, although more zinc-containing terminals are seen (by using Timm staining) in animals that have more seizures, the ultrastructural analysis of dentate molecular layer failed to show an increased number of excitatory synapses, favoring the idea that MFS is related to replacement or restoration of lost contacts rather than to increased excitability (125, 130). Other evidence against the excitatory role of MFS has also been suggested in electrophysiological studies. Even though commissural fibers are excitatory and might be predicted to excite granule cells, the activation of this pathway in vivo has a predominantly inhibitory effect on granule cells. This is likely because the mossy cell-derived commissural pathway, directly excites inhibitory basket cells (131–133). MFS may re-innervate both basket cells and granule cells, which were found to be disinhibited and hyperexcitable immediately after hilar neuron loss, prior to MFS (84, 134). Another proposed mechanism is dependent on Neuropeptide Y (NPY) which is highly expressed in mossy fibers of pilocarpine-treated rats. When spontaneously released from the recurrent mossy fiber terminals, NPY reduces glutamate release by presynaptic activation of Y2 receptors, depressing the epileptiform activity of granule cells dependent on the recurrent innervation (135, 136). Thus, the imbalance between excitatory/inhibitory inputs is believed to be the underlying mechanism of the MFS action. Accordingly, optogenetic approaches have been used to induce excitation of GABAergic interneurons and thus, seizure suppression (137–139). However, recent evidence argues for a possible “excitatory” role of GABAergic cells, depending on the context in epileptic circuitry. The dual roles of GABAergic interneurons was recently addressed, demonstrating that these neurons can (1) excite postsynaptic neurons due to the elevated reversal potential of Cl− in the postsynaptic cells; (2) be GABA-depleted with continuous activity; (3) synchronize network activity during seizures; and (4) inhibit other interneurons, causing disinhibition of principal neurons and network excitability (140).
Although MFS is a common finding in MTLE, not all patients with spontaneous seizures develop MFS (141, 142), a finding that is corroborated in some animal models (99, 143, 144). As a result, MFS would not be necessary for triggering or maintaining hippocampal hyperexcitability. Favoring this idea, cycloheximide, a protein synthesis inhibitor, when used at SE onset did not interfere with induced and spontaneous seizures, but suppressed MFS in the pilocarpine and KA models (101, 143–145), as confirmed by electron microscopy (130). The mechanisms underlying such suppression remain unclear, but may be associated with a neuroprotection of hilar cells (38). Although complete suppression of MFS by cycloheximide treatment was not confirmed by other laboratories, their results indeed demonstrated a reduction of MFS (146). Regardless of whether MFS is suppressed or reduced after cycloheximide, these studies demonstrate that MFS can be modulated and does not directly interfere with the occurrence of spontaneous and recurrent seizures.
Thus, MFS is neither pro- nor anti-epileptic and has also been suggested to be an epiphenomenon (147). Adult-born granule cells robustly contribute to MFS and form functional recurrent synapses (148) as do the neonatally born neurons (40, 149). Considering the increased neurogenesis rate after seizures, it is likely that continued neurogenesis results in increased MFS, which is further reinforced by subsequent spontaneous seizures and results in increased dentate excitability. However, it was recently demonstrated that despite the presence of robust morphological MFS from granule cells born after status epilepticus, these synapses were not functionally active, unable to drive recurrent excitation (148).
Alternative Epilepsy Treatments With or Without Effect on Mossy Fiber Sprouting
MTLE is one of the most prevalent forms of refractory symptomatic epilepsy. Despite the effectiveness of pharmacological therapy in controlling seizures in more than two thirds of cases, some patients develop unacceptable pharmacological side effects (150), which makes continuing the search for better treatment options of utmost importance. Although MFS's role in epileptogenesis is not entirely understood, the close association between aberrant MFS and epileptogenesis indicates that therapeutic strategies capable of suppressing MFS into the inner molecular layer, may have potential clinical significance. Thus, MFS may be an important therapeutic target for treatments designed to interfere with, or modulate the axonal guidance system.
When conventional antiepileptic drugs (AEDs) fail to achieve their desired effects and the surgical resection of the focus is not an option, alternative methods are usually explored. Some of these methods include a ketogenic diet (151, 152), vagus nerve stimulation (VNS) (153, 154), deep brain stimulation (DBS) (155–157), cell therapy (158), or new experimental compounds (159, 160). When tested in animal models, the ketogenic diet produced contradictory effects (161, 162), while VNS (163, 164), and DBS (165, 166) led to a reduction in hippocampal excitability and/or spontaneous seizures (165, 166). VNS and DBS are surgical alternative procedures for patients who are not responsive to conventional AEDs and are not candidates for surgical resections (e.g., due to multiple seizure foci or foci in eloquent regions).
Vagus Nerve Stimulation
One possible underlying mechanism for the effects of VNS, is the increase of extracellular norepinephrine concentrations (167) in the hippocampus (168), amygdala (169), and cortex (168). VNS could inhibit seizure activity in PTZ-kindled rats (170) and delayed the development of seizures in cats after KA treatment (171). Recently it was demonstrated that intermittent VNS is able to increase the expression of the fibroblast growth factor (FGF) and the brain-derived neurotrophic factor (BDNF) in the hippocampus and cortex of rats (172, 173); to increase proliferation in the dentate gyrus (174). There is however, no evidence that VNS affects the MFS.
Deep Brain Stimulation
Deep brain stimulation of the anterior thalamic nucleus (AN) has been approved for the treatment of medically-refractory partial epilepsy (155). In preclinical models, AN DBS was shown to reduce the frequency of seizures (165) and increase the latency for SE (175, 176). The anticonvulsant effects of AN stimulation were also demonstrated prior to pilocarpine treatment, resulting in an increased latency for seizures and SE (175, 176). In the long-term, AN DBS during SE, results in an increased latency for the development of chronic recurrent seizures and neuroprotection in the dentate gyrus and CA1 regions (158). The neuroprotective role of AN DBS during status, or in the chronic phase, may occur due to a reduction in apoptosis and neuroinflammation (33), as well as hippocampal excitability (166) and increases hippocampal adenosine levels (166), suggesting that adenosine is involved with neuroprotection, in this model. Similar results were observed when DBS was applied to other brain targets (e.g., hippocampus) (159). Despite its seizure-modulating actions, AN DBS does not alter neo-Timm expression in the pilocarpine model of epilepsy (177).
Cell Transplantation Therapy
Recent studies have focused on cell transplantation, as an attempt to replace neuronal loss in various hippocampal subfields and/or to explore its potential to release disease-modifying substances. The effects of transplants on spontaneous seizure suppression, are promising. On one hand, there was no reduction in the percentage of rats that developed spontaneous seizures, but transplanted rats displayed fewer spontaneous seizures than sham-transplanted controls after KA treatment (178). Using cell therapy, Bortolotto and colleagues reduced the number of spontaneous seizures, but there was no difference in the kindling susceptibility following grafting (179). In another recent study, a long-term reduction in the number of spontaneous seizures was found in mice after the intra hippocampal transplantation of progenitor cells from embryonic medial eminence after pilocarpine-induced SE (180). Endogenous cell transplantation can be genetically manipulated to affect and modify disease progression. Important results were found using GABAergic progenitor cells. These grafts of GABAergic neurons were able to suppress seizures by enhancing synaptic inhibition in a chronic epileptic animal model of pilocarpine in mice (180–182). Therefore, despite a few controversial studies, there is some work showing a great therapeutic potential for the transplanted cells.
Human fetal stem cell treatment was assessed in the pilocarpine model of TLE to reduce seizures. This treatment showed extensive migration of the implanted cells around the injection site, along with differentiation (24% produced GABA); increased glial cell-derived neurotrophic factor (GDNF) levels, but did not reverse MFS (183). Opposing results were recently demonstrated following the intravenous infusion of mesenchymal stem cells from rat bone marrow, which was associated with the neuroprotection, reduction of cognitive deficits and suppression of MFS (184). The authors concluded that grafts might reduce epileptogenesis through the suppression of aberrant MFS (184). Grafts of CA3 fetal cells enriched with FGF-2 and BDNF exhibit robust integration and inhibit the abnormal MFS in the injured hippocampus (185). CA3 cell grafts transplanted into the injured rat hippocampus 4 or 45 days after KA, dramatically reduced the extent of aberrant MFS (~70%). This shows that such techniques may be promising for partially restoring hippocampal pathology after damage and the release of substances that could modulate and interfere with MFS formation and axonal guidance.
Recently, a group of epilepsy resistant patients was treated with AED supplemented with a single intravenous administration of undifferentiated autologous mesenchymal stem cells. These patients either achieved remission (no seizures for 1 year and more) or became respondent to AEDs (158) indicating that stem cell treatment may be promising for the treatment of pharmacoresistant patients.
Other Experimental Therapies
MFS was prevented after repetitive administration of nicardipine, an L-type Ca++ channel blocker that also ameliorated the cognitive deterioration but had no anticonvulsant action against pilocarpine seizures (159). A list of a few relevant studies with different subjects, models and treatments for MTLE is listed in Table 1. In this table we listed studies that had success in suppressing both the MFS and spontaneous seizures (184, 186, 189); suppressed MFS but had no effect on spontaneous seizures (101, 109, 129, 143, 144, 187, 188) or suppressed seizures but had no effect on MFS (183). Among these studies, systemically infused mesenchymal stem cells (MSCs) suppressed aberrant MFS in the hippocampus in the lithium-pilocarpine injection model (184). However, the use of human neural stem/progenitor cells (huNSPCs) in the pilocarpine model suppressed seizures, but did not reverse MFS (183). Based on that, cellular therapy can be effective in the remission of spontaneous recurrent seizures, which could also be verified in studies with patients (158), but is not always effective in suppressing MFS. Some therapeutic compounds such as resveratrol treatment have the potential for reducing the intensity of injury-chronic epilepsy (192) by decreasing the frequency of spontaneous seizures, protecting against kainate-induced neuronal cell death in the CA1 and CA3 hippocampus and suppressing MFS (190). However, rapamycin and curcumin treatments did not change epileptogenesis (191). Rapamycin, which was effective in suppressing seizures, as long as its blood levels were sufficiently high, had no effects on MFS in electrical post-SE model. According to the authors, curcumin's lack of effect was possibly because it did not reach the brain at adequate levels (191).
Conclusion
In this article we reviewed MFS, as a pathological substrate for MTLE (Figure 3). Other morphological alterations include hippocampal sclerosis, astrogliosis, neurogenesis, cell dispersion to name a few. The contributing role of each one of these malformations for the development of epileptogenesis is not clearly understood. Although MFS is a frequent finding in MTLE it is not necessarily present. Although MFS makes recurrent excitatory circuits, these are likely not sufficient to generate seizures. Based on different strategies such as, protein synthesis inhibitors, calcium channel blockers, stem cell therapy, and rapamycin studies, we learned that MFS is an active process that can be manipulated, but has little or inconsistent effects on seizure suppression Thus, we conclude that MFS is related to replacement or restoration of lost synaptic contacts, rather than to increased excitability in hippocampal circuitry.
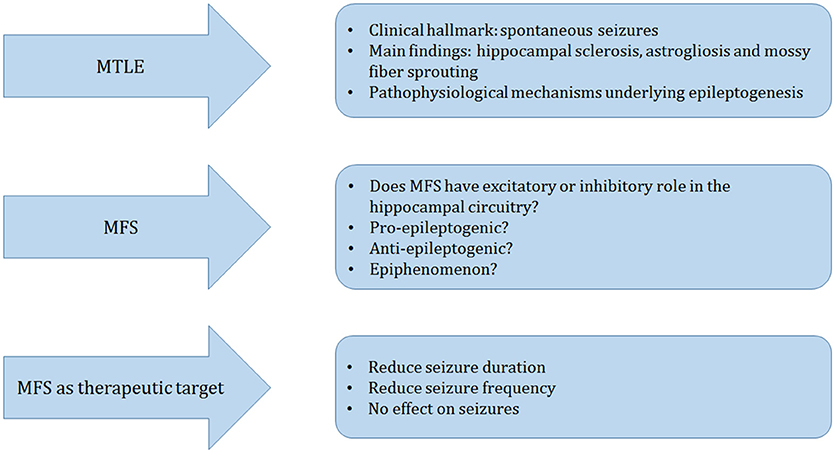
Figure 3. Schematic representation of the main topics of this manuscript. Mesial temporal lobe epilepsy (MTLE) is clinically hallmarked by spontaneous seizures. The main pathological findings in the surgically resected hippocampus of patients and animal models of TLE are hippocampal sclerosis, astrogliosis, and mossy fiber sprouting (MFS), which are frequently studied to understand the mechanisms that may underlie epileptogenesis. We discussed three main different perspectives: Does MFS have a pro- or anti-epileptogenic role or is it an epiphenomenon? We finalize our review with a series of current alternative therapeutic approaches to reduce seizures and excitability that may affect or not the MFS.
Author Contributions
CC and JM worked on the literature search and prepared the first draft of the manuscript. CH and IN participated in the writing process and LC established the manuscript structure, coordinated, and participated in the revision writing.
Conflict of Interest Statement
The authors declare that the research was conducted in the absence of any commercial or financial relationships that could be construed as a potential conflict of interest.
Acknowledgments
The authors would like to thank Katharina Quinlan (University of Rhode Island) for her assistance proof reading the article. This work was supported by CNPq (200970/2015-1) (Brazil).
References
1. McNamara J. Emerging insights into the genesis of epilepsy. Nature (1999) 399:A15–22. doi: 10.1038/399a015
2. Engel J Jr. Mesial temporal lobe epilepsy: what have we learned? Neuroscientist (2001) 7:340–52. doi: 10.1177/107385840100700410
4. DeLorenzo R, Pellock J, Towne A, Boggs J. Epidemiology of status epilepticus. J Clin Neurophysiol. (1995) 12:316–25. doi: 10.1097/00004691-199512040-00003
6. Loscher W, Schmidt D. New horizons in the development of antiepileptic drugs: the search for new targets. Epilepsy Res. (2004) 60:77–159.
7. Lewis D. Losing neurons: selective vulnerability and mesial temporal sclerosis.Epilepsia (2005) 46:39–44. doi: 10.1111/j.1528-1167.2005.00306.x
8. Pitkänen A. Theraputic approaches to epileptogenesis - hope on the horizon. Epilepsia (2010) 51:2–17. doi: 10.1111/j.1528-1167.2010.02602.x
9. Pitkanen A, Sutula T. Is epilepsy a progressive disorder? Prospects for new therapeutic approaches in temporal-lobe epilepsy. Lancet Neurol. (2002) 1 173–81. doi: 10.1016/S1474-4422(02)00073-X
10. Lothman E, Bertram E. Epileptogenic effects of status epilepticus. Epilepsia (1993) 34:59–70. doi: 10.1111/j.1528-1157.1993.tb05907.x
11. Pitkänen A, Nehlig A, Brooks-Kayal A, Dudek F, Friedman D, Galanopoulou A, et al. Issues related to development of antiepileptogenic therapies. Epilepsia (2013) 54:35–43. doi: 10.1111/epi.12297
12. Simonato M, Brooks-Kayal A, Engel JJ, Galanopoulou A, Jensen F, Moshé S, et al. The challenge and promise of anti-epileptic therapy development in animal models. Lancet Neurol. (2014) 13:949–60. doi: 10.1016/S1474-4422(14)70076-6
13. Engel J Jr. Introduction to temporal lobe epilepsy. Epilepsy Res. (1996) 26:141–50. doi: 10.1016/S0920-1211(96)00043-5
14. Schwartzkroin P. Role of the hippocampus in epilepsy. Hippocampus (1994) 4:239–42. doi: 10.1002/hipo.450040302
15. Quesney L. Clinical and EEG features of complex partial seizures of temporal lobe origin. Epilepsia (1986) 27(Suppl 2):S27–45. doi: 10.1111/j.1528-1157.1986.tb05738.x
16. Spencer S, Williamson P, Spencer D, Mattson R. Human hippocampal seizure spread studied by depth and subdural recording: the hippocampal commissure. Epilepsia (1987) 28:479–89. doi: 10.1111/j.1528-1157.1987.tb03676.x
17. Williamson P, Thadani V, French J, Darcey T, Mattson R, Spencer S, et al. Medial temporal lobe epilepsy: videotape analysis of objective clinical seizure characteristics. Epilepsia (1998) 39:1182–8. doi: 10.1111/j.1528-1157.1998.tb01309.x
18. Ben Ari Y. Limbic seizure and brain damage produced by kainic acid: mechanisms and relavance to human temporal lobe epilepsy. Neuroscience (1985) 14:375–403. doi: 10.1016/0306-4522(85)90299-4
19. Cavalheiro E, Leite J, Bortolotto Z, Turski W, Ikonomidou C, et al. Long-term effects of pilocarpine in rats: structural damage of the brain triggers kindling and spontaneous recurrent seizures. Epilepsia (1991) 32:778–82. doi: 10.1111/j.1528-1157.1991.tb05533.x
20. Olney J, Collins R, Sloviter R. Excitotoxicity mechanisms of epileptic brain damage. Adv Neurol. (1986) 44:857–77.
21. Perez-Mendes P, Blanco M, Calcagnotto M, Cinini S, Bachiega J, Papoti D, et al. Modeling epileptogenesis and temporal lobe epilepsy in a non-human primate. Epilepsy Res (2011) 96:45–57. doi: 10.1016/j.eplepsyres.2011.04.015
22. Bertram E, Lothman E, Lenn N. The hippocampus in experimental chronic epilepsy: a morphometric analysis. Ann Neurol. (1990) 27:43–8. doi: 10.1002/ana.410270108
23. Covolan L, Mello L. Temporal profile of neuronal injury following pilocarpine or kainic acid-induced status epilepticus. Epilepsy Res. (2000) 39:133–52. doi: 10.1016/S0920-1211(99)00119-9
24. Covolan L, Smith R, Mello L. Ultrastructural identification of dentate granule cell death from pilocarpine-induced seizures. Epilepsy Res. (2000) 41:9–21. doi: 10.1016/S0920-1211(00)00122-4
25. Mello L, Cavalheiro E, Tan A, Kupfer W, Pretorius J, Babb T, et al. Circuit mechanisms of seizures in the pilocarpine model of chronic epilepsy: cell loss and mossy fiber sprouting. Epilepsia (1993) 34:985–95. doi: 10.1111/j.1528-1157.1993.tb02123.x
26. Mouritze Dam A. Hippocampal neuron loss in epilepsy and after experimental seizures. Acta Neurol Scand. (1982) 66:601–42. doi: 10.1111/j.1600-0404.1982.tb04528.x
27. Kang T, Kim D, Kwak S, Kim J, Won M, Kim D, et al. Epileptogenic roles of astroglial death and regeneration in the dentate gyrus of experimental temporal lobe epilepsy. Glia (2006) 54:258–71. doi: 10.1002/glia.20380
28. Khurgel M, Ivy G. Astrocytes in kindling: relevance to epileptogenesis. Epilepsy Res. (1996) 26:163–75. doi: 10.1016/S0920-1211(96)00051-4
29. Khurgel M, Switzer 3rd R, Teskey G, Spiller A, Racine R, Ivy G. Activation of astrocytes during epileptogenesis in the absence of neuronal degeneration. Neurobiol Dis. (1995) 2:23–35. doi: 10.1006/nbdi.1995.0003
30. Vezzani A, Balosso S, Ravizza T. The role of cytokines in the pathophysiology of epilepsy. Brain Behav Immun. (2008) 22:797–803. doi: 10.1016/j.bbi.2008.03.009
31. Vezzani A, Granata T. Brain inflammation in epilepsy: experimental and clinical evidence. Epilepsia (2005) 46:1724–43. doi: 10.1111/j.1528-1167.2005.00298.x
32. Vezzani A, Moneta D, Richichi C, Aliprandi M, Burrows S, Ravizza T, et al. Functional role of inflammatory cytokines and antiinflammatory molecules in seizures and epileptogenesis. Epilepsia (2002) 43:30–5. doi: 10.1046/j.1528-1157.43.s.5.14.x
33. Amorim B, Covolan L, Ferreira E, Brito J, Nunes D, Morais D, et al. Deep brain stimulation induces antiapoptotic and anti-inflammatory effects in epileptic rats. J Neuroinflamm. (2015) 12:162–6. doi: 10.1186/s12974-015-0384-7
34. Bengzon J, Kokaia Z, Elmér E, Nanobashvili A, Kokaia M, Lindvall O. Apoptosis and proliferation of dentate gyrus neurons after single and intermittent limbic seizures. Proc Natl Acad Sci USA. (1997) 94:10432–7.
35. Fujikawa D. The temporal evolution of neuronal damage from pilocarpine-induced status epilepticus. Brain Res. (1996) 725:11–22. doi: 10.1016/0006-8993(96)00203-X
36. Henshall D, Meldrum B. Cell death and survival mechanisms after single and repeated brief seizures. In: Noebels J, Avoli M, Rogawski M, Olsen R, Delgado-Escueta A, editors. Jasper's Basic Mechanisms of the Epilepsies, Bethesda, MD: National Center for Biotechnology Information (2012).
37. Bielefeld P, van Vliet E, Gorter J, Lucassen P, Fitzsimons C. Different subsets of newborn granule cells: a possible role in epileptogenesis? Eur J Neurosci. (2014) 39:1–11. doi: 10.1111/ejn.12387
38. Covolan L, Ribeiro L, Longo B, Mello L. Cell damage and neurogenesis in the dentate granule cell layer of adult rats after pilocarpine- or kainate-induced status epilepticus. Hippocampus (2000) 10:169–80. doi: 10.1002/(SICI)1098-1063(2000)10:2<169::AID-HIPO6=3.0.CO;2-W
39. Hester M, Danzer S. Accumulation of abnormal adult-generated hippocampal granule cells predicts seizure frequency and severity. J Neurosci. (2013) 33:8926–36. doi: 10.1523/JNEUROSCI.5161-12.2013
40. Parent J, Yu T, Leibowitz R, Geschwind D, Sloviter R, Lowenstein D. Dentate granule cell neurogenesis is increased by seizures and contributes to aberrant network reorganization in the adult rat hippocampus. J Neurosci. (1997) 17:3727–38. doi: 10.1523/JNEUROSCI.17-10-03727.1997
41. Scott B, Wojtowicz J, Burnham W. Neurogenesis in the dentate gyrus of the rat following electroconvulsive shock seizures. Exp Neurol. (2000) 165:231–6. doi: 10.1006/exnr.2000.7458
42. Shapiro L, Figueroa-Aragon S, Ribak C. Newly generated granule cells show rapid neuroplastic changes in the adult rat dentate gyrus during the first five days following pilocarpine-induced seizures. Eur J Neurosci. (2007) 26:583–92. doi: 10.1111/j.1460-9568.2007.05662.x
43. Dudek F. Seizure-induced neurogenesis and epilepsy: involvement of ectopic granule cells? Epilepsy Curr. (2004) 4:103–4. doi: 10.1111/j.1535-7597.2004.43007.x
44. Parent J, Elliott R, Pleasure S, Barbaro N, Lowenstein D. Aberrant seizure-induced neurogenesis in experimental temporal lobe epilepsy. Ann Neurol. (2006) 59:81–91. doi: 10.1002/ana.20699
45. Pierce J, Melton J, Punsoni M, McCloskey D, Scharfman H. Mossy fibers are the primary source of afferent input to ectopic granule cells that are born after pilocarpine-induced seizures. Exp Neurol. (2005) 196:316–31. doi: 10.1016/j.expneurol.2005.08.007
46. Pierce J, Punsoni M, McCloskey D, Scharfman H. Mossy cell axon synaptic contacts on ectopic granule cells that are born following pilocarpine-induced seizures. Neurosci Lett. (2007) 422:136–40. doi: 10.1016/j.neulet.2007.06.016
47. Scharfman H, Goodman J, McCloskey D. Ectopic granule cells of the rat dentate gyrus. Dev Neurosci. (2007) 29:14–27. doi: 10.1159/000096208
48. Kelly T, Beck H. Functional properties of granule cells with hilar basal dendrites in the epileptic dentate gyrus. Epilepsia (2017) 58:160–71. doi: 10.1111/epi.13605
49. Sanchez R, Ribak C, Shapiro L. Synaptic connections of hilar basal dendrites of dentate granule cells in a neonatal hypoxia model of epilepsy. Epilepsia (2012) 53:98–108. doi: 10.1111/j.1528-1167.2012.03481.x
50. Spigelman I, Yan X, Obenaus A, Lee E, Wasterlain C, Ribak C. Dentate granule cells form novel basal dendrites in a rat model of temporal lobe epilepsy. Neuroscience (1998) 86:109–20. doi: 10.1016/S0306-4522(98)00028-1
51. Avanzi RD, Cavarsan CF, Santos JG Jr, Hamani C, Mello LE, Covolan L. Basal dendrites are present in newly born dentate granule cells of young but not aged pilocarpine-treated chronic epileptic rats. Neuroscience (2010) 170:687–91. doi: 10.1016/j.neuroscience.2010.08.004
52. Kienzler F, Norwood B, Sloviter R. Hippocampal injury, atrophy, synaptic reorganization, and epileptogenesis after perforant pathway stimulation-induced status epilepticus in the mouse. J Comp Neurol. (2009) 515:181–96. doi: 10.1002/cne.22059
53. Sloviter R. Status epilepticus-induced neuronal injury and network reorganization. Epilepsia (1999) 40 S34–9. doi: 10.1111/j.1528-1157.1999.tb00876.x
54. Zhang Y, Xiong T, Tan B, Song Y, Li S, Yang L, et al. Pilocarpine-induced epilepsy is associated with actin cytoskeleton reorganization in the mossy fiber-CA3 synapses. Epilepsy Res. (2014) 108:379–89. doi: 10.1016/j.eplepsyres.2014.01.016
55. El Bahh B, Lespinet V, Lurton D, Coussemacq M, Le Gal La Salle G, Rougier A. Correlations between granule cell dispersion, mossy fiber sprouting, and hippocampal cell loss in temporal lobe epilepsy. Epilepsia (1999) 40:1393–401. doi: 10.1111/j.1528-1157.1999.tb02011.x
56. Houser C. Granule cell dispersion in the dentate gyrus of humans with temporal lobe epilepsy. Brain Res. (1990) 535:195–204. doi: 10.1016/0006-8993(90)91601-C
57. Jessberger S, Römer B, Babu H, Kempermann G. Seizures induce proliferation and dispersion of doublecortin-positive hippocampal progenitor cells. Exp Neurol (2005) 196:342–51. doi: 10.1016/j.expneurol.2005.08.010
58. Mello L, Cavalheiro E, Tan A, Pretorius J, Babb T, Finch D. Granule cell dispersion in relation to mossy fiber sprouting, hippocampal cell loss, silent period and seizure frequency in the pilocarpine model of epilepsy. Epilepsy Res. (1992) 9:51–9.
59. Mathern G, Cifuentes F, Leite J, Pretorius J, Babb T. Hippocampal EEG excitability and chronic spontaneous seizures are associated with aberrant synaptic reorganization in the rat intrahippocampal kainate model. Electroencephalogr Clin Neurophysiol. (1993) 87:326–39. doi: 10.1016/0013-4694(93)90186-Y
60. Thom M, Zhou J, Martinian L, Sisodiya S. Quantitative post-mortem study of the hippocampus in chronic epilepsy: seizures do not inevitably cause neuronal loss. Brain (2005) 128:1344–57. doi: 10.1093/brain/awh475
61. Covolan L, Mello L. Assessment of the progressive nature of cell damage in the pilocarpine model of epilepsy. Braz J Med Biol Res. (2006) 39:915–24. doi: 10.1590/S0100-879X2006000700010
62. Mello L, Covolan L. Spontaneous seizures preferentially injure interneurons in the pilocarpine model of chronic spontaneous seizures. Epilepsy Res. (1996) 26:123–9. doi: 10.1016/S0920-1211(96)00048-4
63. Engel J Jr. So what can we conclude–do seizures damage the brain? Prog Brain Res. (2002) 135:509–12. doi: 10.1016/S0079-6123(02)35048-9
64. Thom M. Hippocampal sclerosis in epilepsy: a neuropathology review. Neuropathol Appl Neurobiol. (2014) 40:520–43. doi: 10.1111/nan.12150
65. Blumcke I, Thom M, Aronica E, Armstrong D, Bartolomei F, Bernasconi A, et al. International consensus classification of hippocampal sclerosis in temporal lobe epilepsy: a Task Force report from the ILAE Commission on Diagnostic Methods. Epilepsia (2013) 54:1315–29. doi: 10.1111/epi.12220
66. Mueller S, Laxer K, Schuff N, Weiner M. Voxel-based T2 relaxation rate measurements in temporal lobe epilepsy (TLE) with and without mesial temporal sclerosis. Epilepsia (2007) 48:220–8. doi: 10.1111/j.1528-1167.2006.00916.x
67. Bower S, Vogrin S, Morris K, Cox I, Murphy M, Kilpatrick C, et al. Amygdala volumetry in “imaging-negative” temporal lobe epilepsy. J Neurol Neurosurg Psychiatry (2003) 74:1245–9. doi: 10.1136/jnnp.74.9.1245
68. Keller S, Wilke M, Wieshmann U, Sluming V, Roberts N. Comparison of standard and optimized voxel-based morphometry for analysis of brain changes associated with temporal lobe epilepsy. Neuroimage (2004) 23:860–8. doi: 10.1016/j.neuroimage.2004.07.030
69. Doherty J, Dingledine R. Reduced excitatory drive onto interneurons in the dentate gyrus after status epilepticus. Neuroscience (2001) 21:2048–57. doi: 10.1523/JNEUROSCI.21-06-02048.2001
70. Sloviter R. Decreased hippocampal inhibition and selective loss of interneurons in experimental epilepsy. Science (1987) 235:73–6. doi: 10.1126/science.2879352
71. Sloviter R, Bumanglag A, Schwarcz R, Frotscher M. Abnormal dentate gyrus network circuitry in temporal lobe epilepsy. In: Noebels J, Avoli M, Rogawski M, Olsen R, Delgado-Escueta A, editors. Jasper's Basic Mechanisms of the Epilepsies, Bethesda, MD: National Center for Biotechnology Information (2012).
72. Sloviter R. Permanently altered hippocampal structure, excitability, and inhibition after experimental status epilepticus in the rat: the dormant basket cell hypothesis and its possible relevance to temporal lobe epilepsy. Hippocampus (1991) 1:41–66. doi: 10.1002/hipo.450010106
73. Jinde S, Zsiros V, Nakazawa k. Hilar mossy cell circuitry controlling dentate granule cell excitability. Front Neural Circuits (2013) 7:14. doi: 10.3389/fncir.2013.00014
74. Wieser H, Neurosurgery ICo. Mesial temporal lobe epilepsy with hippocampal sclerosis. Epilepsia (2004) 45:695–714. doi: 10.1111/j.0013-9580.2004.09004.x
75. Briellmann R, Kalnins R, Berkovic S, Jackson G. Hippocampal pathology in refractory temporal lobe epilepsy: T2-weighted signal change reflects dentate gliosis. Neurology (2002) 58:265–71. doi: 10.1212/WNL.58.2.265
76. Thom M, Sisodiya S, Beckett A, Martinian L, Lin W, Harkness W, et al. Cytoarchitectural abnormalities in hippocampal sclerosis. J Neuropathol Exp Neurol. (2002) 61:510–9. doi: 10.1093/jnen/61.6.510
77. Kim S, Nabekura J, Koizumi S. Astrocyte-mediated synapse remodeling in the pathological brain. Glia (2017) 65:1719–27. doi: 10.1002/glia.23169
78. Shen Y, Qin H, Chen J, Mou L, He Y, Yan Y, et al. Postnatal activation of TLR4 in astrocytes promotes excitatory synaptogenesis in hippocampal neurons. J Cell Biol. (2016) 215:719–34. doi: 10.1083/jcb.201605046
79. Yu C, Gui W, He H, Wang X, Zuo J, Huang L, et al. Neuronal and astroglial TGFβ-Smad3 signaling pathways differentially regulate dendrite growth and synaptogenesis. NeuroMol Med. (2014) 16:457–72. doi: 10.1007/s12017-014-8293-y
80. Okada-Tsuchioka M, Segawa M, Kajitani N, Hisaoka-Nakashima K, Shibasaki C, Morinobu S, et al. Electroconvulsive seizure induces thrombospondin-1 in the adult rat hippocampus. Prog Neuropsychopharmacol Biol Psychiatry (2014) 48:236–44. doi: 10.1016/j.pnpbp.2013.10.001
81. Christopherson K, Ullian E, Stokes C, Mullowney C, Hell J, Agah A, et al. Thrombospondins are astrocyte-secreted proteins that promote CNS synaptogenesis. Cell (2005) 120:421–33. doi: 10.1016/j.cell.2004.12.020
82. Li H, Graber K, Jin S, McDonald W, Barres B, Prince D. Gabapentin decreases epileptiform discharges in a chronic model of neocortical trauma. Neurobiol Dis. (2012) 48:429–38. doi: 10.1016/j.nbd.2012.06.019
83. Represa A, Jorquera I, Le Gal La Salle G, Ben-Ari Y. Epilepsy induced collateral sprouting of hippocampal mossy fibers: does it induce the development of ectopic synapses with granule cell dendrites? Hippocampus (1993) 3:257–68. doi: 10.1002/hipo.450030303
84. Sloviter R, Zappone C, Harvey B, Frotscher M. Kainic acid-induced recurrent mossy fiber innervation of dentate gyrus inhibitory interneurons: possible anatomical substrate of granule cell hyper-inhibition in chronically epileptic rats. J Comp Neurol. (2006) 494:944–60. doi: 10.1002/cne.20850
85. Sutula T, He X, Cavazos J, Scott G. Synaptic reorganization in the hippocampus induced by abnormal functional activity. Science (1988) 239:1147–50. doi: 10.1126/science.2449733
86. Binder DK, Croll SD, Gall CM, Scharfman HE. BDNF and epilepsy: too much of a good thing? Trends Neurosci. (2001) 24:47–53. doi: 10.1016/S0166-2236(00)01682-9
87. Ikegaya Y. Abnormal targeting of developing hippocampal mossy fibers after epileptiform activities via L-type Ca2+ channel activation in vitro. J Neurosci. (1999) 19:802–12. doi: 10.1523/JNEUROSCI.19-02-00802.1999
88. Koyama R, Yamada MK, Fujisawa S, Katoh-Semba R, Matsuki N, Ikegaya Y. Brain-derived neurotrophic factor induces hyperexcitable reentrant circuits in the dentate gyrus. J Neurosci. (2004) 24:7215–24. doi: 10.1523/JNEUROSCI.2045-04.2004
89. Bekirov IH, Nagy V, Svoronos A, Huntley GW, Benson DL. Cadherin-8 and N-cadherin differentially regulate pre- and postsynaptic development of the hippocampal mossy fiber pathway. Hippocampus (2008) 18:349–63. doi: 10.1002/hipo.20395
90. Shibata K, Nakahara S, Shimizu E, Yamashita T, Matsuki N, Koyama R. Repulsive guidance molecule a regulates hippocampal mossy fiber branching in vitro. Neuroreport (2013) 24:609–15. doi: 10.1097/WNR.0b013e3283632c08
91. Song MY, Tian FF, Wang YZ, Huang X, Guo JL, Ding DX. Potential roles of the RGMa-FAK-Ras pathway in hippocampal mossy fiber sprouting in the pentylenetetrazole kindling model. Mol Med Rep (2015) 11:1738–44. doi: 10.3892/mmr.2014.2993
92. Fricke R, Prince D. Electrophysiology of dentate gyrus granule cells. J Neurophysiol. (1984) 51:195–209. doi: 10.1152/jn.1984.51.2.195
93. Amaral D, Scharfman H, Lavenex P. The dentate gyrus: fundamental neuroanatomical organization (dentate gyrus for dummies). Prog Brain Res. (2007) 163:3–22. doi: 10.1016/S0079-6123(07)63001-5
94. Sutula T, Cascino G, Cavazos J, Parada I, Ramirez L. Mossy fiber synaptic reorganization in the epileptic human temporal lobe. Ann Neurol. (1989) 26:321–30. doi: 10.1002/ana.410260303
95. Tauck D, Nadler J. Evidence of functional mossy fiber sprouting in hippocampal formation of kainic acid-treated rats. J Neurosci. (1985) 5:1016–22. doi: 10.1523/JNEUROSCI.05-04-01016.1985
96. Buckmaster P. Mossy fiber sprouting in the dentate gyrus. In: Noebels J, Avoli M, Rogawski M, Olsen R, Delgado-Escueta A, editors. Jasper's Basic Mechanisms of Epilepsies, Bethesda, MD: National Center for Biotechnology Information (2012).
97. Immonen R, Kharatishvili I, Sierra A, Einula C, Pitkänen A, Gröhn O. Manganese enhanced MRI detects mossy fiber sprouting rather than neurodegeneration, gliosis or seizure-activity in the epileptic rat hippocampus. Neuroimage (2008) 40:1718–30. doi: 10.1016/j.neuroimage.2008.01.042
98. Malheiros J, Paiva F, Longo B, Hamani C, Covolan L. Manganese-enhanced MRI: biological applications in neuroscience. Front Neurol. (2015) 6:161. doi: 10.3389/fneur.2015.00161
99. Malheiros J, Polli R, Paiva F, Longo B, Mello L, Silva A, et al. Manganese-enhanced magnetic resonance imaging detects mossy fiber sprouting in the pilocarpine model of epilepsy. Epilepsia (2012) 53:1225–32. doi: 10.1111/j.1528-1167.2012.03521.x
100. Nairismägi J, Pitkänen A, Narkilahti S, Huttunen J, Kauppinen R, Gröhn O. Manganese-enhanced magnetic resonance imaging zof mossy fiber plasticity in vivo. Neuroimage (2006) 30:130–5. doi: 10.1016/j.neuroimage.2005.09.007
101. Longo B, Covolan L, Chadi G, Mello L. Sprouting of mossy fibers and the vacating of postsynaptic targets in the inner molecular layer of the dentate gyrus. Exp Neurol. (2003) 181:57–67. doi: 10.1016/S0014-4886(02)00046-8
102. Holtmaat A, Gorter J, De Wit J, Tolner E, Spijker S, Giger R, et al. Transient downregulation of Sema3A mRNA in a rat model for temporal lobe epilepsy. A novel molecular event potentially contributing to mossy fiber sprouting. Exp Neurol. (2003) 182:142–50. doi: 10.1016/S0014-4886(03)00035-9
103. Tamagnone L, Comoglio P. Signalling by semaphorin receptors: cell guidance and beyond. Trends Cell Biol. (2000) 10:377–83. doi: 10.1016/S0962-8924(00)01816-X
104. Schmeiser B, Li J, Brandt A, Zentner J, Doostkam S, Freiman T. Different mossy fiber sprouting patterns in ILAE hippocampal sclerosis types. Epilepsy Res. (2017) 136:115–22. doi: 10.1016/j.eplepsyres.2017.08.002
105. Elmer E, Kokaia Z, Kokaia M, Lindvall O, McIntyre D. Mossy fibre sprouting: evidence against a facilitatory role in epileptogenesis. Neuroreport (1997) 8:1193–6. doi: 10.1097/00001756-199703240-00027
106. Nissinen J, Lukasiuk K, Pitkänen A. Is mossy fiber sprouting present at the time of the first spontaneous seizures in rat experimental temporal lobe epilepsy? Hippocampus (2001) 11:299–310. doi: 10.1002/hipo.1044
107. Mello L, Covolan L. Neuronal injury and progressive cell damage. In: Schwartzkroin PA, editor. Encyclopedia of Basic Epilepsy Research. Cambridge, MA; London: Academic Press (2009). p. 125–8.
108. Gorter J, van Vliet E, Aronica E, Lopes da Silva F. Progression of spontaneous seizures after status epilepticus is associated with mossy fibre sprouting and extensive bilateral loss of hilar parvalbumin and somatostatin-immunoreactive neurons. Eur J Neurosci Biobehav Rev. (2001) 13:657–69. doi: 10.1046/j.1460-9568.2001.01428.x
109. Heng K, Haney M, Buckmaster P. High-dose rapamycin blocks mossy fiber sprouting but not seizures in a mouse model of temporal lobe epilepsy. Epilepsia (2013) 54:1535–41. doi: 10.1111/epi.12246
110. Isokawa M, Levesque M, Babb T, Engel J Jr. Single mossy fiber axonal system of human dentate granule cells studied in hippocampal slices from patients with temporal lobe epilepsy. J Neurosci. (1993) 13:1511–22. doi: 10.1523/JNEUROSCI.13-04-01511.1993
111. Cronin J, Obenaus A, Houser C, Dudek F. Electrophysiology of dentate granule cells after kainate-induced synaptic reorganization of the mossy fibers. Brain Res. (1992) 573:305–10. doi: 10.1016/0006-8993(92)90777-7
112. Wuarin J, Dudek F. Excitatory synaptic input to granule cells increases with time after kainate treatment. J Neurophysiol. (2001) 85:1067–77. doi: 10.1152/jn.2001.85.3.1067
113. McNamara J. Cellular and molecular basis of epilepsy. J Neurosci. (1994) 14:3413–25. doi: 10.1523/JNEUROSCI.14-06-03413.1994
114. Cavazos J, Golarai G, Sutula T. Mossy fiber synaptic reorganization induced by kindling: time course of development, progression, and permanence. J Neurosci. (1991) 11:2795–803. doi: 10.1523/JNEUROSCI.11-09-02795.1991
115. Schmeiser B, Zentner J, Prinz M, Brandt A, Freiman T. Extent of mossy fiber sprouting in patients with mesiotemporal lobe epilepsy correlates with neuronal cell loss and granule cell dispersion. Epilepsy Res. (2017) 129:51–8. doi: 10.1016/j.eplepsyres.2016.11.011
116. Polli R, Malheiros J, Dos Santos R, Hamani C, Longo B, Tannús A, et al. Changes in hippocampal volume are correlated with cell loss but not with seizure frequency in two chronic models of temporal lobe epilepsy. Front Neurol. (2014) 5:111. doi: 10.3389/fneur.2014.00111
117. Adams B, Lee M, Fahnestock M, Racine R. Long-term potentiation trains induce mossy fiber sprouting. Brain Res. (1997) 775:193–7. doi: 10.1016/S0006-8993(97)01061-5
118. Zimmer J. Changes in the Timm sulfide silver staining patter of the rat hippocampus and fascia dentata following early postnatal deaferentiation. Brain Res. (1973) 64:313–26. doi: 10.1016/0006-8993(73)90186-8
119. Zimmer J. Long-term synaptic reorganization in rat fascia dentata deafferented at adolescent and adult stages: observations with the Timm method. Brain Res. (1974) 76:336–42. doi: 10.1016/0006-8993(74)90465-X
120. Colling S, Khana M, Collinge J, Jefferys J. Mossy fibre reorga-nization in the hippocampus of prion protein null mice. Brain Res. (1997) 755:28–35. doi: 10.1016/S0006-8993(97)00087-5
121. Bender R, Dubé C, Gonzalez-Vega R, Mina E, Baram T. Mossy fiber plasticity and enhanced hippocampal excitability, without hippocampal cell loss or altered neurogenesis, in an animal model of prolonged febrile seizures. Hippocampus (2003) 13:399–412. doi: 10.1002/hipo.10089
122. Liu R, Lemieux L, Bell G, Sisodiya S, Bartlett P, Shorvon S, et al. Cerebral damage in epilepsy: a population-based longitudinal quantitative MRI study. Epilepsia (2005) 46:1482–94. doi: 10.1111/j.1528-1167.2005.51603.x
123. Mitsueda-Ono T, Ikeda A, Sawamoto N, Aso T, Hanakawa T, Kinoshita M, et al. Internal structural changes in the hippocampus observed on 3-tesla MRI in patients with mesial temporal lobe epilepsy. Intern Med. (2013) 52:877–85. doi: 10.2169/internalmedicine.52.8852
124. Ratzliff A, Santhakumar V, Howard A, Soltesz I. Mossy cells in epilepsy: rigor mortis or vigor mortis? Trends Neurosci. (2002) 25: 140–4. doi: 10.1016/S0166-2236(00)02122-6
125. Buckmaster P. Does mossy fiber sprouting give rise to the epileptic state? In: Scharfman H, Buckmaster P, editors. Issues in Clinical Epileptology: A View From the Bench, Advances in Experimental Medicine and Biology. Vol. 813. Dordrecht: Springer (2014). doi: 10.1007/978-94-017-8914-1_13
126. Yamawaki R, Thind K, Buckmaster PS. Blockade of excitatory synaptogenesis with proximal dendrites of dentate granule cells following rapamycin treatment in a mouse model of temporal lobe epilepsy. J Comp Neurol. (2015) 523:281–97. doi: 10.1002/cne.23681
127. Wong M. Mammalian target of rapamycin (mTOR) pathways in neurological diseases. Biomed J. (2013) 36:40–50. doi: 10.4103/2319-4170.110365
128. Zoncu R, Efeyan A, Sabatini DM. mTOR: from growth signal integration to cancer, diabetes and ageing. Nat Rev Mol Cell Biol. (2011) 12:21–35. doi: 10.1038/nrm3025
129. Zhu F, Kai J, Chen L, Wu M, Dong J, Wang Q, et al. Akt inhibitor perifosine prevents epileptogenesis in a rat model of temporal lobe epilepsy. Neurosci Bull (2018) 34:283–90. doi: 10.1007/s12264-017-0165-7
130. Bittencourt S, Covolan L, C H, Longo B, Faria F, Freymuller E, et al. Replacement of asymmetric synaptic profiles in the molecular layer of dentate gyrus following cycloheximide in the pilocarpine model in rats. Front Psychiatry (2015) 6:157. doi: 10.3389/fpsyt.2015.00157
131. Buzsáki G, Eidelberg E. Direct afferent excitation and long-term potentiation of hippocampal interneurons. J Neurophysiol. (1982) 48:597–607.
132. Buzsàki G, Eidelberg E. Commissural projection to the dentate gyrus of the rat: evidence for feed-forward inhibition. Brain Res. (1981) 230:346–50.
133. Ribak C, Peterson G. Intragranular mossy fibers in rats and gerbils form synapses with the somata and proximal dendrites of basket cells in the dentate gyrus. Hippocampus (1991) 1:355–64. doi: 10.1002/hipo.450010403
134. Sloviter R. Possible functional consequences of synaptic reorganization in the dentate gyrus of kainate-treated rats. Neurosci Lett. (1992) 137:91–6. doi: 10.1016/0304-3940(92)90306-R
135. Nadler J, Tu B, Timofeeva O, Jiao Y, Herzog H. Neuropeptide Y in the recurrent mossy fiber pathway. Peptides (2007) 28:357–64. doi: 10.1016/j.peptides.2006.07.026
136. Tu B, Timofeeva O, Jiao Y, Victor Nadler J. Spontaneous release of neuropeptide Y tonically inhibits recurrent mossy fiber synaptic transmission in epileptic brain. J Neurosci. (2005) 25:1718–29. doi: 10.1523/JNEUROSCI.4835-04.2005
137. Krook-Magnuson E, Armstrong C, Oijala M, Soltesz I. On-demand optogenetic control of spontaneous seizures in temporal lobe epilepsy. Nat Commun. (2013) 4:1376. doi: 10.1038/ncomms2376
138. Ladas T, Chiang C, Gonzalez-Reyes L, Nowak T, Durand D. Seizure reduction through interneuron-mediated entrainment using low frequency optical stimulation. Exp Neurol. (2015) 269:120–32. doi: 10.1016/j.expneurol.2015.04.001
139. Ledri M, Madsen M, Nikitidou L, Kirik D, Kokaia M. Global optogenetic activation of inhibitory interneurons during epileptiform activity. J Neurosci. (2014) 34:3364–77. doi: 10.1523/JNEUROSCI.2734-13.2014
140. Ye H, Kaszuba S. Inhibitory or excitatory? Optogenetic interrogation of the functional roles of GABAergic interneurons in epileptogenesis. J Biomedical Sci. (2017) 24:93. doi: 10.1186/s12929-017-0399-8
141. de Lanerolle N, Kim J, Williamson A, Spencer S, Zaveri H, Eid T, et al. A retrospective analysis of hippocampal pathology in human temporal lobe epilepsy: evidence for distinctive patient subcategories. Epilepsia (2003) 44:677–87. doi: 10.1046/j.1528-1157.2003.32701.x
142. Matsukawa L, Uruno K, Sperling M, O'connor M, Burdette L. The functional relationship between antidromically evoked field responses of the dentate gyrus and mossy fiber reorganization in temporal lobe epileptic patients. Brain Res. (1992) 579:119–27. doi: 10.1016/0006-8993(92)90750-4
143. Longo B, Mello L. Blockade of pilocarpine- or kainate-induced mossy fiber sprouting by cycloheximide does not prevent subsequent epileptogenesis in rats. Neurosci Lett. (1997) 226:163–6. doi: 10.1016/S0304-3940(97)00267-X
144. Longo B, Mello L. Supragranular mossy fiber sprouting is not necessary for spontaneous seizures in the intrahippocampal kainate model of epilepsy in the rat. Epilepsy Res. (1998) 32:172–82. doi: 10.1016/S0920-1211(98)00049-7
145. Longo B, Sanabria E, Gabriel S, Mello L. Electrophysiologic abnormalities of the hippocampus in the pilocarpine/cycloheximide model of chronic spontaneous seizures. Epilepsia (2002) 43:203–8. doi: 10.1046/j.1528-1157.43.s.5.4.x
146. Williams P, Wuarin J-P, Dou P, Ferraro D, Dudek F. Reassessment of the effects of cycloheximide on mossy fiber sprouting and epileptogenesis in the pilocarpine model of temporal lobe epilepsy. J Neurophysiol. (2002) 88:2075–87. doi: 10.1152/jn.2002.88.4.2075
148. Hendricks L, Chen Y, Bensen A, Westbrook G, Schnell E. Short-term depression of sprouted mossy fiber synapses from adult-born granule cells. J Neurosci. (2017) 37:5722–35. doi: 10.1523/JNEUROSCI.0761-17.2017
149. Althaus A, Zhang H, Parent J. Axonal plasticity of age-defined dentate granule cells in a rat model of mesial temporal lobe epilepsy. Neurobiol Dis. (2016) 86:187–96. doi: 10.1016/j.nbd.2015.11.024
150. Wong I, Chadwick D, Fenwick P, Mawer G, Sander J. The long-term use of gabapentin, lamotrigine, and vigabatrin in patients with chronic epilepsy. Epilepsia (1999) 40:1439–45. doi: 10.1111/j.1528-1157.1999.tb02017.x
151. Freeman J, Veggiotti P, Lanzi G, Tagliabue A, Perucca E. The ketogenic diet: from molecular mechanisms to clinical effects. Epilepsy Res. (2006) 68:145–80. doi: 10.1016/j.eplepsyres.2005.10.003
152. Freeman J, Vining E, Pillas D, Pyzik P, Casey J, Kelly L. The efficacy of the ketogenic diet-1998: a prospective evaluation of intervention in 150 children. Pediatrics (1998) 102:1358–63. doi: 10.1542/peds.102.6.1358
153. Handforth A, DeGiorgio C, Schachter S, Uthman B, Naritoku D, Tecoma E, et al. Vagus nerve stimulation therapy for partial-onset seizures: a randomized active-control trial. Neurology (1998) 51:48–55. doi: 10.1212/WNL.51.1.48
154. Vonck K, Thadani V, Gilbert K, Dedeurwaerdere S, De Groote L, De Herdt V, et al. Vagus nerve stimulation for refractory epilepsy: a transatlantic experience. J Clin Neurophysiol. (2004) 21:283–9. doi: 10.1097/01.WNP.0000139654.32974.4E
155. Fisher R, Salanova V, Witt T, Worth R, Henry T, Gross R, et al. Electrical stimulation of the anterior nucleus of thalamus for treatment of refractory epilepsy. Epilepsia (2010) 51:899–908. doi: 10.1111/j.1528-1167.2010.02536.x
156. Vonck K, Boon P, Achten E, De Reuck J, Caemaert J. Long-term amygdalohippocampal stimulation for refractory temporal lobe epilepsy. Ann Neurol. (2002) 52:556–65. doi: 10.1002/ana.10323
157. Vonck K, Boon P, Claeys P, Dedeurwaerdere S, Achten R, Van Roost D. Long-term deep brain stimulation for refractory temporal lobe epilepsy. Epilepsia (2005) 46:98–9. doi: 10.1111/j.1528-1167.2005.01016.x
158. Hlebokazov F, Dakukina T, Ihnatsenko S, Kosmacheva S, Potapnev M, Shakhbazau A, et al. Treatment of refractory epilepsy patients with autologous mesenchymal stem cells reduces seizure frequency: An open label study. Adv Med Sci. (2017) 62:273–9. doi: 10.1016/j.advms.2016.12.004
159. Ikegaya Y, Nishiyama N, Matsuki N. L-type Ca(2+) channel blocker inhibits mossy fiber sprouting and cognitive deficits following pilocarpine seizures in immature mice. Neuroscience (2000) 98:647–59. doi: 10.1016/S0306-4522(00)00188-3
160. Kiasalari Z, Roghani M, Khalili M, Rahmati B, Baluchnejadmojarad T. Antiepileptogenic effect of curcumin on kainate-induced model of temporal lobe epilepsy. Pharm Biol. (2013) 51:1572–8. doi: 10.3109/13880209.2013.803128
161. Linard B, Ferrandon A, Koning E, Nehlig A, Raffo E. Ketogenic diet exhibits neuroprotective effects in hippocampus but fails to prevent epileptogenesis in the lithium-pilocarpine model of mesial temporal lobe epilepsy in adult rats. Epilepsia (2010) 51:1829–36. doi: 10.1111/j.1528-1167.2010.02667.x
162. Noh H, Kim Y, Lee H, Chung K, Kim D, Kang S, et al. The protective effect of a ketogenic diet on kainic acid-induced hippocampal cell death in the male ICR mice. Epilepsy Res. (2003) 53:119–28. doi: 10.1016/S0920-1211(02)00262-0
163. Dedeurwaerdere S, Gilby K, Vonck K, Delbeke J, Boon P, McIntyre D. Vagus nerve stimulation does not affect spatial memory in fast rats, but has both anti-convulsive and pro-convulsive effects on amygdala-kindled seizures. Neuroscience (2006) 140:1443–51. doi: 10.1016/j.neuroscience.2006.03.014
164. Grimonprez A, Raedt R, Dauwe I, Mollet L, Larsen L, Meurs A, et al. Vagus nerve stimulation has antidepressant effects in the kainic acid model for temporal lobe epilepsy. Brain Stimul. (2015) 8:13–20. doi: 10.1016/j.brs.2014.09.013
165. Covolan L, de Almeida A, Amorim B, Cavarsan C, Miranda M, Aarão M, et al. Effects of anterior thalamic nucleus deep brain stimulation in chronic epileptic rats. PLoS ONE (2014) 9:e97618. doi: 10.1371/journal.pone.0097618
166. Miranda M, Hamani C, de Almeida A, Amorim B, Macedo C, Fernandes M, et al. Role of adenosine in the antiepileptic effects of deep brain stimulation. Front Cell Neurosci. (2014) 8:312. doi: 10.3389/fncel.2014.00312
167. Raedt R, Clinckers R, Mollet L, Vonck K, El Tahry R, Wyckhuys T, et al. Increased hippocampal noradrenaline is a biomarker for efficacy of vagus nerve stimulation in a limbic seizure model. J Neurochem. (2011) 117:461–9. doi: 10.1111/j.1471-4159.2011.07214.x
168. Roosevelt RW, Smith DC, Clough RW, Jensen RA, Browning RA. Increased extracellular concentrations of norepinephrine in cortex and hippocampus following vagus nerve stimulation in the rat. Brain Res. (2006) 1119:124–32. doi: 10.1016/j.brainres.2006.08.048
169. Hassert DL, Miyashita T, Williams CL. The effects of peripheral vagal nerve stimulation at a memory-modulating intensity on norepinephrine output in the basolateral amygdala. Behav Neurosci. (2004) 118:79–88. doi: 10.1037/0735-7044.118.1.79
170. Kaya M, Becker AJ, Gurses C. Blood-brain barrier, epileptogenesis, and treatment strategies in cortical dysplasia. Epilepsia (2012) 53(Suppl 6):31–6. doi: 10.1111/j.1528-1167.2012.03700.x
171. Fernandez-Guardiola A, Martinez A, Valdes-Cruz A, Magdaleno-Madrigal VM, Martinez D, Fernandez-Mas R. Vagus nerve prolonged stimulation in cats: effects on epileptogenesis (amygdala electrical kindling): behavioral and electrographic changes. Epilepsia (1999) 40:822–9. doi: 10.1111/j.1528-1157.1999.tb00787.x
172. Biggio F, Gorini G, Utzeri C, Olla P, Marrosu F, Mocchetti I, et al. Chronic vagus nerve stimulation induces neuronal plasticity in the rat hippocampus. Int J Neuropsychopharmacol. (2009) 12:1209–21. doi: 10.1017/S1461145709000200
173. Follesa P, Biggio F, Gorini G, Caria S, Talani G, Dazzi L, et al. Vagus nerve stimulation increases norepinephrine concentration and the gene expression of BDNF and bFGF in the rat brain. Brain Res. (2007) 1179:28–34. doi: 10.1016/j.brainres.2007.08.045
174. Revesz D, Tjernstrom M, Ben-Menachem E, Thorlin T. Effects of vagus nerve stimulation on rat hippocampal progenitor proliferation. Exp Neurol. (2008) 214:259–65. doi: 10.1016/j.expneurol.2008.08.012
175. Hamani C, Ewerton FI, Bonilha SM, Ballester G, Mello LE, Lozano AM. Bilateral anterior thalamic nucleus lesions and high-frequency stimulation are protective against pilocarpine-induced seizures and status epilepticus. Neurosurgery (2004) 54:191–5. doi: 10.1227/01.NEU.0000097552.31763.AE
176. Hamani C, Hodaie M, Chiang J, del Campo M, Andrade DM, Sherman D, et al. Deep brain stimulation of the anterior nucleus of the thalamus: effects of electrical stimulation on pilocarpine-induced seizures and status epilepticus. Epilepsy Res. (2008) 78:117–23. doi: 10.1016/j.eplepsyres.2007.09.010
177. Ferreira E, Vieira L, Moraes D, Amorim B, Malheiros J, Hamani C, et al. Long-term effects of anterior thalamic nucleus deep brain stimulation on spatial learning in the pilocarpine model of temporal lobe epilepsy. Neuromodulation (2018) 21:160–7. doi: 10.1111/ner.12688
178. Holmes GL, Thompson JL, Huh K, Holmes C, Carl GF. Effect of neural transplants on seizure frequency and kindling in immature rats following kainic acid. Brain Res Dev Brain Res. (1991) 64:47–56. doi: 10.1016/0165-3806(91)90208-Z
179. Bortolotto ZA, Calderazzo L, Cavalheiro EA. Some evidence that intrahippocampal grafting of noradrenergic neurons suppresses spontaneous seizures in epileptic rats. Braz J Med Biol Res. (1990) 23:1267–9.
180. Cunningham M, Cho JH, Leung A, Savvidis G, Ahn S, Moon M, et al. hPSC-derived maturing GABAergic interneurons ameliorate seizures and abnormal behavior in epileptic mice. Cell Stem Cell (2014) 15:559–73. doi: 10.1016/j.stem.2014.10.006
181. Casalia ML, Howard MA, Baraban SC. Persistent seizure control in epileptic mice transplanted with gamma-aminobutyric acid progenitors. Ann Neurol. (2017) 82:530–42. doi: 10.1002/ana.25021
182. Henderson KW, Gupta J, Tagliatela S, Litvina E, Zheng X, Van Zandt MA, et al. Long-term seizure suppression and optogenetic analyses of synaptic connectivity in epileptic mice with hippocampal grafts of GABAergic interneurons. J Neurosci. (2014) 34:13492–504. doi: 10.1523/JNEUROSCI.0005-14.2014
183. Lee H, Yun S, Kim IS, Lee IS, Shin JE, Park SC, et al. Human fetal brain-derived neural stem/progenitor cells grafted into the adult epileptic brain restrain seizures in rat models of temporal lobe epilepsy. PLoS ONE (2014) 9:e104092. doi: 10.1371/journal.pone.0104092
184. Fukumura S, Sasaki M, Kataoka-Sasaki Y, Oka S, Nakazaki M, Nagahama H, et al. Intravenous infusion of mesenchymal stem cells reduces epileptogenesis in a rat model of status epilepticus. Epilepsy Res. (2018) 141:56–63. doi: 10.1016/j.eplepsyres.2018.02.008
185. Rao MS, Hattiangady B, Shetty AK. Fetal hippocampal CA3 cell grafts enriched with FGF-2 and BDNF exhibit robust long-term survival and integration and suppress aberrant mossy fiber sprouting in the injured middle-aged hippocampus. Neurobiol Dis. (2006) 21:276–90. doi: 10.1016/j.nbd.2005.07.009
186. Chen L, Gao B, Fang M, Li J, Mi X, Xu X, et al. Lentiviral vector-induced overexpression of RGMa in the hippocampus suppresses seizures and mossy fiber sprouting. Mol Neurobiol. (2017) 54:1379–91. doi: 10.1007/s12035-016-9744-2
187. Buckmaster P, Lew F. Rapamycin suppresses mossy fiber sprouting but not seizure frequency in a mouse model of temporal lobe epilepsy. J Neurosci. (2011) 31:2337–47. doi: 10.1523/JNEUROSCI.4852-10.2011
188. Buckmaster P, Ingram E, Wen X. Inhibition of the mammalian target of rapamycin signaling pathway suppresses dentate granule cell axon sprouting in a rodent model of temporal lobe epilepsy. J Neurosci. (2009) 29:8259–69. doi: 10.1523/JNEUROSCI.4179-08.2009
189. Li S, Uri Saragovi H, Racine R, Fahnestock M. A ligand of the p65/p95 receptor suppresses perforant path kindling, kindling-induced mossy fiber sprouting, and hilar area changes in adult rats. Neuroscience (2003) 119:1147–56. doi: 10.1016/S0306-4522(03)00239-2
190. Wu Z, Xu Q, Zhang L, Kong D, Ma R, Wang L. Protective effect of resveratrol against kainate-induced temporal lobe epilepsy in rats. Neurochem Res. (2009) 34:1393–400. doi: 10.1007/s11064-009-9920-0
191. Drion C, Borm L, Kooijman L, Aronica E, Wadman W, Hartog A, et al. Effects of rapamycin and curcumin treatment on the development of epilepsy after electrically induced status epilepticus in rats. Epilepsia (2016) 57:688–97. doi: 10.1111/epi.13345
Keywords: temporal lobe epilepsy, mossy fibers sprouting, epileptogenesis, deep brain stimulation, hippocampus
Citation: Cavarsan CF, Malheiros J, Hamani C, Najm I and Covolan L (2018) Is Mossy Fiber Sprouting a Potential Therapeutic Target for Epilepsy?. Front. Neurol. 9:1023. doi: 10.3389/fneur.2018.01023
Received: 06 April 2018; Accepted: 13 November 2018;
Published: 30 November 2018.
Edited by:
Ching Liang Hsieh, China Medical University, TaiwanReviewed by:
Luiz Eduardo Betting, São Paulo State University, BrazilBharathi Hattiangady, Texas A&M University College of Medicine, United States
Maria Centeno, University College London, United Kingdom
Dinesh Upadhya, Manipal Academy of Higher Education, India
Olagide Wagner Castro, Federal University of Alagoas, Brazil
Copyright © 2018 Cavarsan, Malheiros, Hamani, Najm and Covolan. This is an open-access article distributed under the terms of the Creative Commons Attribution License (CC BY). The use, distribution or reproduction in other forums is permitted, provided the original author(s) and the copyright owner(s) are credited and that the original publication in this journal is cited, in accordance with accepted academic practice. No use, distribution or reproduction is permitted which does not comply with these terms.
*Correspondence: Luciene Covolan, bHVjb3ZvbGFuQGdtYWlsLmNvbQ==