- Division of Brain Sciences, Department of Medicine, Imperial College London, London, United Kingdom
Alzheimer's disease (AD) is characterized by memory loss and decline of cognitive function, associated with progressive neurodegeneration. While neuropathological processes like amyloid plaques and tau neurofibrillary tangles have been linked to neuronal death in AD, the precise role of glial activation on disease progression is still debated. It was suggested that neuroinflammation could occur well ahead of amyloid deposition and may be responsible for clearing amyloid, having a neuroprotective effect; however, later in the disease, glial activation could become deleterious, contributing to neuronal toxicity. Recent genetic and preclinical studies suggest that the different activation states of microglia and astrocytes are complex, not as polarized as previously thought, and that the heterogeneity in their phenotype can switch during disease progression. In the last few years, novel imaging techniques e.g., new radiotracers for assessing glia activation using positron emission tomography and advanced magnetic resonance imaging technologies have emerged, allowing the correlation of neuro-inflammatory markers with cognitive decline, brain function and brain pathology in vivo. Here we review all new imaging technology in AD patients and animal models that has the potential to serve for early diagnosis of the disease, to monitor disease progression and to test the efficacy and the most effective time window for potential anti-inflammatory treatments.
Introduction
Neuroinflammation is the term used to denote the response of the central nervous system to harmful stimuli such as protein aggregation, pathogens, and any other insult to the brain. While timely initiated and resolved, the inflammatory response is necessary reaction to noxious stimuli and hence protective, sustained and/or disproportionate (neuro)inflammation will likely contribute, exacerbate or induce tissue damage and thereby aggravate disease pathology (1). The nature of the inflammatory process is therefore complex and dynamic and changes along different stages of the disease, involving phenotypic alterations in all cells present within the CNS including neurons, microglia, astrocytes, and other inflammatory cells.
Microglia, act as part of the innate immune system, are constantly scanning and surveying the local microenvironment for signals of infection and injury [for a review see (2)]. Amyloid-β has been reported to “prime” or activate microglial cells (3). Different activation states were described in the past, so called “M1” or classically activated microglia or “M2” alternatively activated (2). The classically activated or pro-inflammatory phenotype has been associated with disease aggravation.
However, this classification has been recently challenged by single-cells transcriptomics, which suggests that the gene expression profile progressively switches with the disease and that this may even depend on how close they are to the amyloid plaques (4).
Astrocytes, are key mediators of many essential processes in the CNS. As for microglia, astrocytes have been classified into two distinct reactive states, A1 (inflammatory) and A2 (ischemic) states (5), although this classification seems to be over-simplistic. Accumulation of hypertrophic reactive astrocytes around senile plaques has been observed in post-mortem human tissue from AD patients [Reviewed by (6)] and in animal models of the disease (7). It is worth noting that astrocytes and microglia communicate with each other and this cross talk is important in promoting glial activation (8, 9).
In order to follow-up changes in microglial and astrocytic activation in vivo, radioactive tracers for positron emission and single-photon emission computed tomography (PET and SPECT) have been developed in the past decades. In this review, we will analyze different in vivo imaging techniques that allow the visualization of changes in neuroinflammation in animals and humans.
In vivo Imaging of Microglia Activation
Pet Imaging With TSPO Ligands in AD Patients
Following activation, microglia proliferate, and express a series of genes for pro-inflammatory cytokines and certain receptors on their surface, including the 18 kDa translocator protein (TSPO). TSPO, primarily but not exclusively expressed in the mitochondrial membrane of microglia, was previously identified as the peripheral-type benzodiazepine receptor (PBR) (10). Besides microglia, TSPO has been detected in other types of gliosis as well, such as in astrocytoma (11), and is generally expressed in highly proliferating cells. While the exact function of TSPO still remains to be elucidated, initially its role was associated with the transport of cholesterol, with the TSPO complex being a rate-limiting step in the synthesis of steroid hormones (12). Initial attempts to create TSPO knockout mice reported a non-viable phenotype [described in (13)]. However, the successful development of conditional TSPO knockout mice suggested that TSPO might not be a crucial part of steroid hormone synthesis, e.g., testosterone production (14). In addition, the mitochondrial expression-paradigm was challenged by reports of TSPO expression in other subcellular locations (15), e.g., nuclear/perinuclear-located TSPO, where is believed to play a part in cell proliferation (16). Moreover, plasma membrane bound TSPO has been observed as well, for instance in mature human red blood cells, lacking mitochondria (17, 18).
In the mammalian brain, the expression of TSPO turned out to be very low, compared to other tissues (19). Only the olfactory bulb and non-parenchymal regions, such as the ependymal and choroid plexus, showed higher TSPO densities in comparison with most gray and white matter structures (20, 21). However, under conditions of local inflammatory responses, e.g., caused by a multitude of brain injuries, neoplasms and infections, TSPO appears to be upregulated. This effect was quickly recognized and made TSPO a potentially ideal and sensitive biomarker of brain injury (10, 11, 22–25). Therefore, PET tracers for TSPO were developed in the past decade as markers for microglia activation and neuroinflammation in AD (26, 10) (see Table 1).
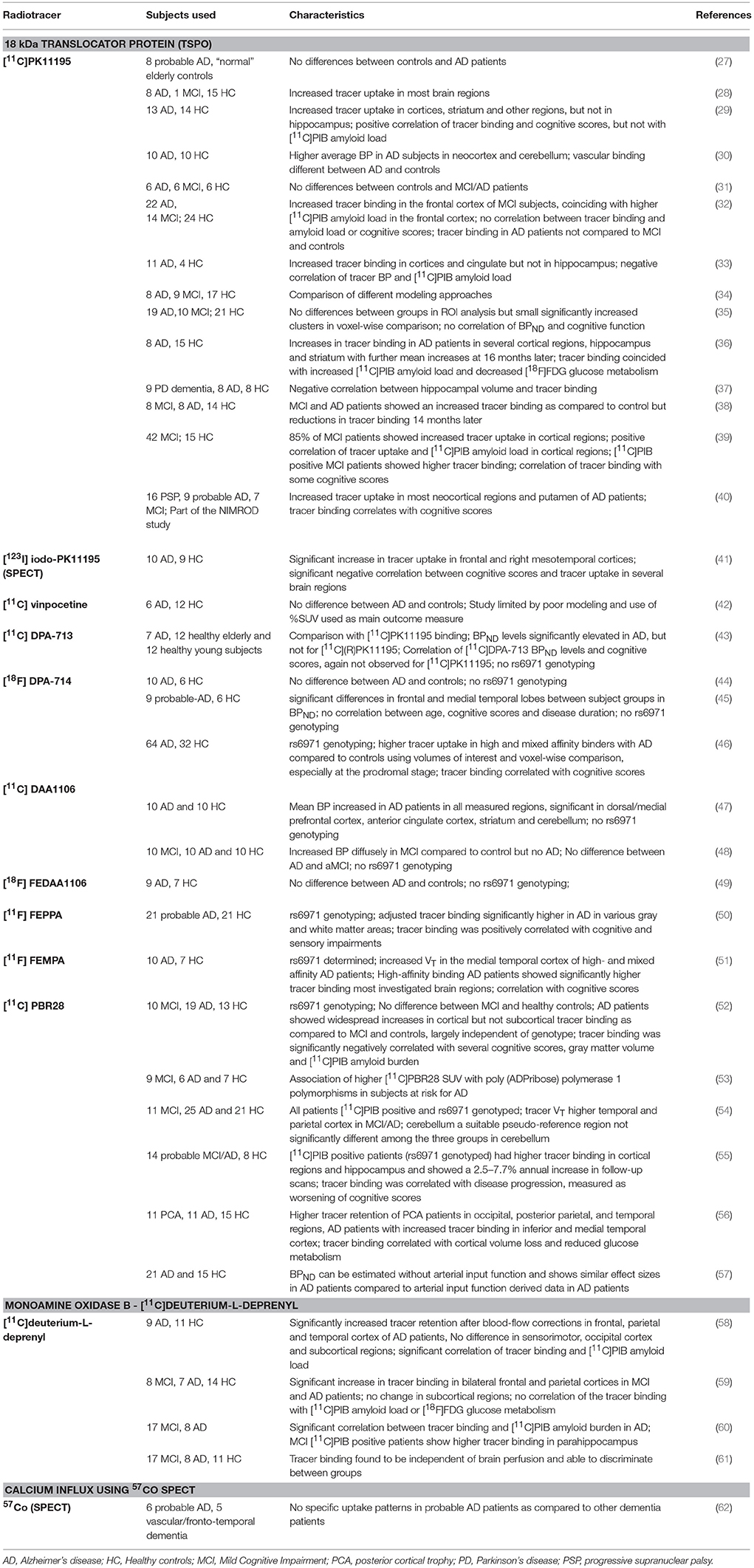
Table 1. In vivo imaging studies in patients with Alzheimer's disease or Mild Cognitive Impairment (MC) with the primary purpose of investigating neuroinflammatory changes through radiolabeled tracers.
The first and probably most widely used TSPO radiotracers, with over thousand publications, are the antagonist PK11195 [1-(2-chlorophenyl)-N-methyl-N-(1-methylpropyl)-3-isoquinoline carboxamide] and the agonist Ro5-4864 (63). Initial studies with PK11195 were conducted more than two decades ago, with numerous subsequent papers demonstrating upregulation in different neurodegenerative diseases and in neuroinflammatory conditions (64–67). Unsurprisingly, increased TSPO expression was reported by autoradiography in a wide range of brain regions of post-mortem samples from AD patients, including hippocampus, frontal, temporal, and parietal cortices (22, 68, 69).
Cagnin et al. published the first PET study using [11C]PK11195 in 2001, demonstrating an increase of tracer uptake in AD cases (28). While subsequent reports generally have shown increased tracer binding in AD brains, some publications found no differences between Alzheimer's subjects and healthy individuals (29, 33, 35). In spite of these conflicting results, it is generally accepted that there is increased microglial activation in AD. Recent studies have extended this to patients with Mild cognitive impairment (MCI), showing that glia activation can precede clinical AD (70).
Recent reports have evaluated the relationship between amyloid load and neuroinflammation, suggesting that microglial activation is associated with amyloid load. Interestingly, using [11C]PK11195 and the amyloid tracer [11C]PIB, one study did not show any correlation between the binding of these tracers, while another one suggested a negative correlation between amyloid-β and TSPO density (31, 33). The reason behind these different outcomes could be related to the limitations of the current amyloid ligands; while we are able to image amyloid plaque deposition using amyloid imaging agents, other forms of amyloid, such as β-amyloid oligomers, which may be contributing to the microglial activation, are not currently detectable by PET (36, 71).
However, rigorous quantification of TSPO density using [11C]PK11195 has been confronted by limitations of the ligand, including its modest binding affinity, high non-specific binding and elevated lipophilicity, generating a low signal-to-noise ratio (72). This has led to the development of numerous “second-generation“ TSPO ligands [11C]AC-5216, [18F]PBR111, both 11C and 18F radiolabeled derivatives of PBR06 and PBR28, [18F]FEPPA, [18F]DPA-714 and the SPECT tracer [123I]CLINDE (46, 48, 64, 73–79). Figure 1 illustrates an example of PET imaging with [11C]-PBR28 showing increased binding in an AD patient.
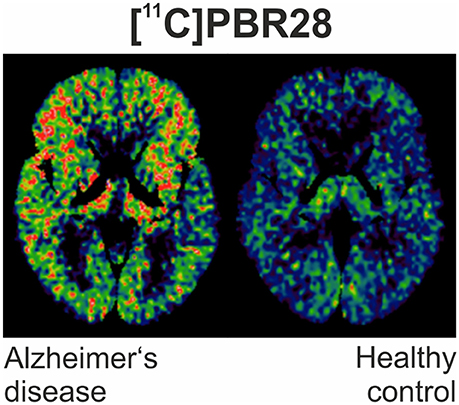
Figure 1. [11C]PBR28 binding is significantly increased in different cortical regions in an Alzheimer's disease subject (MMSE of 22/30) compared to healthy control (MMSE 30/30).
However, while affinity and nonspecific binding properties were usually found to be improved as compared to PK11195, it quickly became apparent that these new TSPO tracers are affected by genetic variability of TSPO binding site induced by the rs6971 single-nucleotide polymorphism (80), resulting in high-, mixed and low-affinity binders. This polymorphism restricts studies with these tracers to high- and mixed-affinity binders. Recently, “third-generation” TSPO tracers, such as 81, GE-180 and ER176 (82) were developed and aimed at allowing TSPO quantification regardless of rs6971 genotype, however with mixed success and no published data in AD patients. Additional considerations to take into account when using these ligands in vivo are the different modeling approaches and reference regions (83), along with other methodological issues reviewed by Donat et al. (84).
In order to follow up changes of TSPO over time, longitudinal studies in AD have recently been published. Two studies (36, 38) revealed reductions in [11C]PK11195 binding in MCI patients with different amyloidosis status, whereas increased binding was found in diagnosed AD patients. A moderate increase of [11C]PBR28 uptake in 14 patients with AD was associated with worsening clinical symptoms (55). The most recent longitudinal study with [18F]DPA-714 demonstrated that prodromal and demented AD patients display an initially higher TSPO density as compared to controls. However, when classifying patients into slow and fast decliners according to functional (Clinical Dementia Rating change) or cognitive (Mini-Mental State Examination score decline) outcomes, it was shown that slow decliners show a higher initial [18F]DPA-714 binding than fast decliners, suggesting that higher initial [18F]DPA-714 binding is associated with better clinical prognosis (85).
Pet Imaging in Animal Models of AD
The first studies carried out in animal models of amyloidosis demonstrated a significant age-dependent increase in the specific binding of [3H]PK11195 in the TASTPM model (APPswxPS1M146V) by autoradiography (8), in agreement with age-dependent increases in CD68 immunoreactivity co-localized with Aβ deposits. However, reports on [11C]PK11195 PET imaging in mouse models of amyloidosis have exposed conflicting results, depending on the model used and age of the animals. A higher [11C]PK11195 uptake was shown in the brains of older APP/PS1 mice when compared with age-matched controls (68, 86). Surprisingly, [11C]PK11195 binding in younger transgenic APP/PS1 mice was not different from their controls, even though immunostaining revealed activated microglia in close proximity of amyloid deposits. Similar to human data, it is likely that different modeling approaches and reference regions may contribute to the seemingly conflicting in vivo findings.
Our own recent autoradiographic and PET data provided evidence of an increased binding of [3H]PBR28 in the brain of the aggressive 5XFAD mouse model, compared with wild-type controls, which coincided with the strongly increased immunoreactivity of the microglial marker Iba1 in the same brain areas (87). These results provided support for the suitability of PBR28 as a tool for monitoring of (micro)-glial activation. [3H]PBR28 binding was significantly higher in female animals and positively correlated between Aβ plaque load and tracer binding. In addition, using [11C]PBR28 in healthy rats, in vitro brain autoradiography showed a 19% increase of binding in aged (19.6 months) as compared in young rats (4 months) (88).
Besides PBR28, other new generation tracers have exhibited similar patterns in animal models of AD. Increases in TSPO density were reported from 10 months old Thy1-hAPPLond/Swe (APPL/S) mice compared with wild-type controls, using ex vivo autoradiography with [18F]PBR06, but this increase was only observed in older mice, at 16 months of age by PET (89). Similar findings were published by Liu et al. (90), who performed [18F]GE180 PET in young and old wild-types (WTs) and APP/PS1dE9 transgenic mice, showing higher uptake in transgenic and WT mice at 24 months of age but not in young 4 months old transgenics (90). In a different study, [18F]GE180 uptake was slightly increased in PS2APP mice at 5 mo and markedly elevated at 16 mo. Over this age range, there was a highly positive correlation between TSPO PET uptake, amyloid load and likewise with tracers for brain metabolism (91). However, a recent study in APP23 mice showed that the increased rate of (micro)glia activation detected with [18F]GE-180 appears to be of less magnitude than the elevation in amyloidosis detected with [11C]PIB over time. In fact, [18F]GE-180 binding seems to plateau at an earlier stage of pathogenesis, whereas amyloidosis continues to increase. These results suggest that TSPO might be a good marker for early pathogenesis detection, but not for tracking long-term disease progression (92).
These tracers have also served to assess and monitor the efficacy of anti-inflammatory treatments. LM11A-31 is a p75 neurotrophin receptor ligand that was shown to reduce the hyperphosphorylation and misfolding of tau, decrease neurite degeneration, and attenuate microglial activation. LM11A-31-treated APPL/S mice displayed significantly lower [18F]GE-180 binding in cortex and hippocampus of as compared to vehicle-treated animals, corresponding to decreased TSPO and Iba1 staining (93).
As AD is characterized by substantial aggregation of hyperphosphorylated tau, second-generation TSPO ligands have also been employed in transgenic models of tau pathology, such as the PS19 mice. Here, uptake of [11C]AC-5216 was found linearly proportional to the phospho-tau immunolabelling (94).
While TSPO is the most widely recognized biomarker of neuroinflammations, other targets have been explored in recent years. Radiolabeled ketoprofen methyl ester, [11C]-KTP-Me is a highly selective tracer for the cyclooxygenase-1 (COX-1). In APP transgenic mice, [11C]-KTP-Me uptake was significantly increased in the brain of 16 to 24 mo old mice in comparison to their age-matched controls, coinciding with the histopathologic appearance of abundant Aβ plaques and activated microglia. Furthermore, [11C]-KTP-Me accumulation was observed in the frontal cortex and hippocampus, whereby COX-1-expressing activated microglia appeared surrounding Aβ plaques, indicating neuroinflammation that originated with Aβ deposition (95). Another currently investigated alternative to TSPO ligands are tracers for the cannabinoid 2 receptor, such as [11C]Sch225336 and [11C]A-836339 (96–98) and tracers for the purinergic receptors P2Y12 and P2X7 (99).
Magnetic Resonance Spectroscopy (MRS)
Magnetic resonance spectroscopy (MRS) is a new technique that can provide information about several relevant metabolites for neuroinflammation and neurodegeneration. Recently, chemical exchange saturation transfer (CEST), as a novel molecular MR imaging approach, has been developed, which uses proton exchange as a means of enhancing the contrast of specific molecules in the body (100). Endogenous CEST compounds include hydroxyl (OH), amine (NH2), and amide groups (NH). In the last few years, several studies have explored the possibility of imaging neuroinflammatory and neurodegeneration biomarkers in vivo with CEST, such as CEST imaging of myo-inositol (101), glutamate (102) and glucose (103) in AD mouse models.
In vivo Imaging of Astrocytes
Pet Imaging of Astrocytes in Humans
The best-known tracer for astrocytes so far is [11C]deuterium-L-deprenyl [[11C]DED], which is an irreversible monoamine oxidase B (MAO-B) inhibitor. This is based on previous findings showing that astrocytes express elevated levels of MAO-B during their activation. The ligand has been therefore employed as biomarker of astrocytosis in pathologies such as AD (59) and amyotrophic lateral sclerosis (ALS) (104). Increased [11C]DED binding throughout the brain was detected in MCI [11C]PIB-positive patients compared with controls and MCI [11C]PIB-negative and AD patients (59). In autosomal dominant AD carriers, astrocytosis measured by [11C]DED was found initially high and then declining, contrasting with the increasing amyloid-β plaque load during disease progression, suggesting astrocyte activation is implicated in the early stages of AD pathology (61).
In the last years, new ligands for the potential imaging of astrocytes have been developed, including those for type-2 imidazoline receptors (I2Rs), which were found to be expressed primarily in astrocytes. These receptors were described for the first time in the 90's and the first studies performing in vitro binding with [3H]idazoxan in postmortem cortical membranes showed increased density in AD patients (105). Later on, work carried out with the I2R PET tracer [11C]FTIMD reported specific binding to these receptors in rat and monkey brains, but exhibiting a relative low binding specificity (106, 107). More recently, [11C]BU99008 (2-(4,5-Dihydro-1H-imidazol-2-yl)-1- methyl-1H-indole) was developed as a more potent PET ligand for I2Rs imaging (108, 109), displaying relatively high binding specificity and brain penetration in the porcine and rhesus monkey brain (108, 110). There are ongoing human PET imaging trials in Alzheimer's and healthy control patients at the moment using [11C]BU99008 and the preliminary results have shown good brain delivery, reversible kinetics, heterogeneous distribution specific binding signal consistent with I2BS distribution and good test-retest reliability (111).
Imaging of Astrocytes in Models of AD
Pet Imaging for Astrocytes
Imaging studies with [11C]DED carried out in transgenic APP Swedish (APPswe) mice and wild–type animals at different ages, have demonstrated that tracer uptake was significantly higher at 6 months than at 18–24 months in APPswe mice, preceding Aβ deposition (112). However, no differences in [3H]-L-deprenyl obtained by autoradiography were observed between WT and APPswe mice across different ages. Furthermore, staining of the astrocyte marker GFAP was increased in older transgenic APPSwe mice as compared to younger mice (112), raising questions about the specificity of this ligand as marker for astrocytes.
in vivo Bioluminescence Imaging (BPI)
Bioluminescence describes the light produced by the enzymatic reaction of a luciferase with its substrate (luciferin) and the emitted light is detected with a camera. The technique allows for fast acquisition times so that subjects can be imaged quickly, serially over time and with minimal distress. The Prusiner's lab developed in vivo bioluminescence imaging and quantitative determination of inflammation in a model of prion related neurodegenerative disease (113). Additionally, bigenic mice overexpressing APP and GFAP-Luc were reported to show an age-dependent increase in signal that was corresponded to major areas of Aβ deposition. Bioluminescence signals began to increase in 7-mo-old Tg(CRND8:Gfap-luc) mice and at 14-mo-old in Tg(APP23:Gfap-luc) mice (114).
Gfap-luciferase reporter mice have also been crossed-bred with hTau40AT/C57BL/6N mice. In vivo bioluminescence imaging (BLI) showed activation of astrocytes in response to aggregation of Tau, from 5 months of age compared with wild-type animals (115).
Conclusions and Future Perspectives
The development of new neuroinflammation tracers in the last decade has allowed characterizing the pattern of glia activation in AD patients, showing that it occurs ahead of amyloid deposition, correlates in many cases with amyloid plaque density and allows limited predictions of disease progression. The longitudinal studies have shown that this glial activation, as detected by PET, fluctuates during disease progression. Although reports in animal models of AD have helped confirming the specificity of TSPO tracers for microglia, the situation is not the same for tracers for astrocytes and more research needs to be done regarding this aspect. In addition, new tracers able to differentiate between the potential M1 and M2 microglial phenotypes would be advantageous in identifying their function in vivo (116).
Future studies should include imaging in patients after intervention with anti-inflammatory drugs; however so far, there are no reports in that aspect in AD cases. Therefore, imaging studies are key to test the efficacy and the most effective time window for potential anti-inflammatory treatments.
Author Contributions
PE and MS designed this review outline, PE performed most of the literature review on TSPO imaging in humans, CD edited and the manuscript, performed the literature review for the table and MS wrote the rest of the manuscript.
Conflict of Interest Statement
The authors declare that the research was conducted in the absence of any commercial or financial relationships that could be construed as a potential conflict of interest.
Acknowledgments
We would like to acknowledge the Royal British Legion Centre for Blast Injury Studies for funding to MS and CD. Paul Edison research is funded by the Higher Education Funding Council for England (HEFCE).
References
1. Lyman M, Lloyd DG, Ji X, Vizcaychipi MP, Ma D. Neuroinflammation: the role and consequences. Neurosci Res. (2014) 79:1–12. doi: 10.1016/j.neures.2013.10.004
2. Solito E, Sastre M. Microglia function in Alzheimer's disease. Front Pharmacol. (2012) 3:14. doi: 10.3389/fphar.2012.00014
3. Barger SW, Harmon AD. Microglial activation by Alzheimer amyloid precursor protein and modulation by apolipoprotein E. Nature (1997) 388:878–81. doi: 10.1038/42257
4. Keren-Shaul H, Spinrad A, Weiner A, Matcovitch-Natan O, Dvir-Szternfeld R, Ulland TK, et al. A unique microglia type associated with restricting development of Alzheimer's Disease. Cell (2017) 169:1276–90 e1217. doi: 10.1016/j.cell.2017.05.018
5. Liddelow SA, Barres BA. Reactive astrocytes: production, function, and therapeutic potential. Immunity (2017) 46:957–67. doi: 10.1016/j.immuni.2017.06.006
6. Medeiros R, LaFerla FM. Astrocytes: conductors of the Alzheimer disease neuroinflammatory symphony. Exp Neurol. (2013) 239:133–8. doi: 10.1016/j.expneurol.2012.10.007
7. Olabarria M, Noristani HN, Verkhratsky A, Rodriguez JJ. Concomitant astroglial atrophy and astrogliosis in a triple transgenic animal model of Alzheimer's disease. Glia (2010) 58:831–8. doi: 10.1002/glia.20967
8. Liu W, Tang Y, Feng J. Cross talk between activation of microglia and astrocytes in pathological conditions in the central nervous system. Life Sci. (2011) 89:141–6. doi: 10.1016/j.lfs.2011.05.011
9. Liddelow SA, Guttenplan KA, Clarke LE, Bennett FC, Bohlen CJ, Schirmer L, et al. Neurotoxic reactive astrocytes are induced by activated microglia. Nature (2017). 541:481–7. doi: 10.1038/nature21029
10. Chen MK, Guilarte TR. Translocator protein 18 kDa (TSPO): molecular sensor of brain injury and repair. Pharmacol Ther. (2008) 118:1–17. doi: 10.1016/j.pharmthera.2007.12.004
11. Su Z, Herholz K, Gerhard A, Roncaroli F, Du Plessis D, Jackson A, et al. [(1)(1)C]-(R)PK11195 tracer kinetics in the brain of glioma patients and a comparison of two referencing approaches. Eur J Nucl Med Mol Imaging (2013) 40:1406–19. doi: 10.1007/s00259-013-2447-2
12. Rupprecht R, Papadopoulos V, Rammes G, Baghai TC, Fan J, Akula N, et al. Translocator protein (18 kDa) (TSPO) as a therapeutic target for neurological and psychiatric disorders. Nat Rev Drug Discov. (2010) 9:971–88. doi: 10.1038/nrd3295
13. Papadopoulos V, Amri H, Boujrad N, Cascio C, Culty M, Garnier M, et al. Peripheral benzodiazepine receptor in cholesterol transport and steroidogenesis. Steroids (1997). 62:21–8.
14. Morohaku K, Pelton SH, Daugherty DJ, Butler WR, Deng W, Selvaraj V. Translocator protein/peripheral benzodiazepine receptor is not required for steroid hormone biosynthesis. Endocrinology (2014) 155:89–97. doi: 10.1210/en.2013-1556
15. Veenman L, Gavish M. The peripheral-type benzodiazepine receptor and the cardiovascular system. Impl Drug Dev Pharmacol Ther. (2006) 110:503–24. doi: 10.1016/j.pharmthera.2005.09.007
16. Brown RC, Degenhardt B, Kotoula M, Papadopoulous V. Location-dependent role of the human glioma cell peripheral-type benzodiazepine receptor in proliferation and steroid biosynthesis. Cancer Lett. (2000) 156:125–32.
17. Olson JM, Ciliax BJ, Mancini WR, Young AB. Presence of peripheral-type benzodiazepine binding sites on human erythrocyte membranes. Eur J Pharmacol. (1988) 152:47–53.
18. Oke BO, Suarez-Quian CA, Riond J, Ferrara P, Papadopoulos V. Cell surface localization of the peripheral-type benzodiazepine receptor (PBR) in adrenal cortex. Mol Cell Endocrinol. (1992) 87:R1–6.
19. Banati RB, Newcombe J, Gunn RN, Cagnin A, Turkheimer F, Heppner F, et al. The peripheral benzodiazepine binding site in the brain in multiple sclerosis: quantitative in vivo imaging of microglia as a measure of disease activity. Brain (2000) 123 (Pt 11):2321–37.
20. Benavides J, Malgouris C, Imbault F, Begassat F, Uzan A, Renault C, et al. ”Peripheral type" benzodiazepine binding sites in rat adrenals: binding studies with [3H]PK 11195 and autoradiographic localization. Arch Int Pharmacodyn Ther. (1983) 266:38–49.
21. Gehlert DR, Yamamura HI, Wamsley JK. Autoradiographic localization of 'peripheral' benzodiazepine binding sites in the rat brain and kidney using [3H]RO5-4864. Eur J Pharmacol. (1983) 95:329–30.
22. Cosenza-Nashat M, Zhao ML, Suh HS, Morgan J, Natividad R, Morgello S, et al. Expression of the translocator protein of 18 kDa by microglia, macrophages and astrocytes based on immunohistochemical localization in abnormal human brain. Neuropathol Appl Neurobiol. (2009) 35:306–28. doi: 10.1111/j.1365-2990.2008.01006.x
23. Scarf AM, Ittner LM, Kassiou M. The translocator protein (18 kDa): central nervous system disease and drug design. J Med Chem. (2009) 52:581–92. doi: 10.1021/jm8011678
24. Dickens AM, Vainio S, Marjamaki P, Johansson J, Lehtiniemi P, Rokka J, et al. Detection of microglial activation in an acute model of neuroinflammation using PET and radiotracers 11C-(R)-PK11195 and 18F-GE-180. J Nucl Med. (2014) 55:466–72. doi: 10.2967/jnumed.113.125625
25. Janssen B, Vugts DJ, Funke U, Molenaar GT, Kruijer PS, van Berckel BN, et al. Imaging of neuroinflammation in Alzheimer's disease, multiple sclerosis and stroke: recent developments in positron emission tomography. Biochim Biophys Acta (2016) 1862:425–41. doi: 10.1016/j.bbadis.2015.11.011
26. Papadopoulos V, Baraldi M, Guilarte TR, Knudsen TB, Lacapere JJ, Lindemann P, et al. Translocator protein (18kDa): new nomenclature for the peripheral-type benzodiazepine receptor based on its structure and molecular function. Trends Pharmacol Sci. (2006) 27:402–9. doi: 10.1016/j.tips.2006.06.005
27. Groom GN, Junck L, Foster NL, Frey KA, Kuhl DE PET of peripheral benzodiazepine binding sites in the microgliosis of Alzheimer's disease. J Nucl Med. (1995) 36:2207–10.
28. Cagnin A, Brooks DJ, Kennedy AM, Gunn RN, Myers R, Turkheimer FE, et al. In-vivo measurement of activated microglia in dementia. Lancet (2001) 358:461–7. doi: 10.1016/S0140-6736(01)05625-2
29. Edison P, Archer HA, Gerhard A, Hinz R, Pavese N, Turkheimer FE, et al. Microglia, amyloid, and cognition in Alzheimer's disease: an [11C](R)PK11195-PET and [11C]PIB-PET study. Neurobiol Dis. (2008) 32:412–9. doi: 10.1016/j.nbd.2008.08.001
30. Tomasi G, Edison P, Bertoldo A, Roncaroli F, Singh P, Gerhard A, et al. Novel reference region model reveals increased microglial and reduced vascular binding of 11C-(R)-PK11195 in patients with Alzheimer's disease. J Nucl Med. (2008) 49:1249–56. doi: 10.2967/jnumed.108.050583
31. Wiley CA, Lopresti BJ, Venneti S, Price J, Klunk WE, DeKosky ST, et al. Carbon 11-labeled Pittsburgh Compound B and carbon 11-labeled (R)-PK11195 positron emission tomographic imaging in Alzheimer disease. Arch Neurol. (2009) 66:60–7. doi: 10.1001/archneurol.2008.511
32. Okello A, Edison P, Archer HA, Turkheimer FE, Kennedy J, Bullock R, et al. Microglial activation and amyloid deposition in mild cognitive impairment: a PET study. Neurology (2009) 72:56–62. doi: 10.1212/01.wnl.0000338622.27876.0d
33. Yokokura M, Mori N, Yagi S, Yoshikawa E, Kikuchi M, Yoshihara Y, et al. In vivo changes in microglial activation and amyloid deposits in brain regions with hypometabolism in Alzheimer's disease. Eur J Nucl Med Mol Imaging (2011) 38:343–51. doi: 10.1007/s00259-010-1612-0
34. Yaqub M, van Berckel BN, Schuitemaker A, Hinz R, Turkheimer FE, Tomasi G, et al. Optimization of supervised cluster analysis for extracting reference tissue input curves in (R)-[(11)C]PK11195 brain PET studies. J Cereb Blood Flow Metab. (2012) 32:1600–8. doi: 10.1038/jcbfm.2012.59
35. Schuitemaker A, Kropholler MA, Boellaard R, van der Flier WM, Kloet RW, van der Doef TF, et al. Microglial activation in Alzheimer's disease: an (R)-[(1)(1)C]PK11195 positron emission tomography study. Neurobiol Aging (2013) 34:128–36. doi: 10.1016/j.neurobiolaging.2012.04.021
36. Fan Z, Okello AA, Brooks DJ, Edison P. Longitudinal influence of microglial activation and amyloid on neuronal function in Alzheimer's disease. Brain (2015) 138(Pt 12):3685–98. doi: 10.1093/brain/awv288
37. Femminella GD, Ninan S, Atkinson R, Fan Z, Brooks DJ, Edison P. Does microglial activation influence hippocampal volume and neuronal function in Alzheimer's disease and parkinson's disease dementia? J Alzheimers Dis. (2016) 51:1275–89. doi: 10.3233/JAD-150827
38. Fan Z, Brooks DJ, Okello A, Edison P. An early and late peak in microglial activation in Alzheimer's disease trajectory. Brain (2017) 140:792–803. doi: 10.1093/brain/aww349
39. Parbo P, Ismail R, Hansen KV, Amidi A, Marup FH, Gottrup H, et al. Brain inflammation accompanies amyloid in the majority of mild cognitive impairment cases due to Alzheimer's disease. Brain (2017) 140:2002–11. doi: 10.1093/brain/awx120
40. Passamonti L, Rodriguez PV, Hong YT, Allinson KSJ, Bevan-Jones WR, Williamson D, et al. [(11)C]PK11195 binding in Alzheimer disease and progressive supranuclear palsy. Neurology (2018) 90:e1989–e1996. doi: 10.1212/WNL.0000000000005610
41. Versijpt JJ, Dumont F, Van Laere KJ, Decoo D, Santens P, Audenaert K, et al. Assessment of neuroinflammation and microglial activation in Alzheimer's disease with radiolabelled PK11195 and single photon emission computed tomography. A pilot study. Eur Neurol. (2003) 50:39–47. doi: 10.1159/000070857
42. Gulyas B, Vas A, Toth M, Takano A, Varrone A, Cselenyi Z, et al. Age and disease related changes in the translocator protein (TSPO) system in the human brain: positron emission tomography measurements with [11C]vinpocetine. Neuroimage (2011) 56:1111–21. doi: 10.1016/j.neuroimage.2011.02.020
43. Yokokura M, Terada T, Bunai T, Nakaizumi K, Takebayashi K, Iwata Y, et al. Depiction of microglial activation in aging and dementia: positron emission tomography with [(11)C]DPA713 versus [(11)C](R)PK11195. J Cereb Blood Flow Metab. (2017) 37:877–89. doi: 10.1177/0271678x16646788
44. Golla SS, Boellaard R, Oikonen V, Hoffmann A, van Berckel BN, Windhorst AD, et al. Quantification of [18F]DPA-714 binding in the human brain: initial studies in healthy controls and Alzheimer's disease patients. J Cereb Blood Flow Metab. (2015) 35:766–72. doi: 10.1038/jcbfm.2014.261
45. Golla SS, Boellaard R, Oikonen V, Hoffmann A, van Berckel BN, Windhorst AD, et al. Parametric binding images of the TSPO Ligand 18F-DPA-714. J Nucl Med. (2016) 57:1543–7. doi: 10.2967/jnumed.116.173013
46. Hamelin L, Lagarde J, Dorothee G, Leroy C, Labit M, Comley RA, et al. Early and protective microglial activation in Alzheimer's disease: a prospective study using 18F-DPA-714 PET imaging. Brain (2016) 139(Pt 4):1252–64. doi: 10.1093/brain/aww017
47. Yasuno F, Ota M, Kosaka J, Ito H, Higuchi M, Doronbekov TK, et al. Increased binding of peripheral benzodiazepine receptor in Alzheimer's disease measured by positron emission tomography with [11C]DAA1106. Biol Psychiatry (2008) 64:835–41. doi: 10.1016/j.biopsych.2008.04.021
48. Yasuno F, Kosaka J, Ota M, Higuchi M, Ito H, Fujimura Y, et al. Increased binding of peripheral benzodiazepine receptor in mild cognitive impairment-dementia converters measured by positron emission tomography with [(1)(1)C]DAA1106. Psychiatry Res. (2012) 203:67–74. doi: 10.1016/j.pscychresns.2011.08.013
49. Varrone A, Mattsson P, Forsberg A, Takano A, Nag S, Gulyas B, et al. In vivo imaging of the 18-kDa translocator protein (TSPO) with [18F]FEDAA1106 and PET does not show increased binding in Alzheimer's disease patients. Eur J Nucl Med Mol Imaging (2013) 40:921–31. doi: 10.1007/s00259-013-2359-1
50. Suridjan I, Pollock BG, Verhoeff NP, Voineskos AN, Chow T, Rusjan PM, et al. In-vivo imaging of grey and white matter neuroinflammation in Alzheimer's disease: a positron emission tomography study with a novel radioligand, [18F]-FEPPA. Mol Psychiatry (2015) 20:1579–87. doi: 10.1038/mp.2015.1
51. Varrone A, Oikonen V, Forsberg A, Joutsa J, Takano A, Solin O, et al. Positron emission tomography imaging of the 18-kDa translocator protein (TSPO) with [18F]FEMPA in Alzheimer's disease patients and control subjects. Eur J Nucl Med Mol Imaging (2015) 42:438–46. doi: 10.1007/s00259-014-2955-8
52. Kreisl WC, Lyoo CH, McGwier M, Snow J, Jenko KJ, Kimura N, et al. In vivo radioligand binding to translocator protein correlates with severity of Alzheimer's disease. Brain (2013) 136(Pt 7):2228–38. doi: 10.1093/brain/awt145
53. Kim S, Nho K, Risacher SL, Inlow M, Swaminathan S, Yoder KK, et al. PARP1 gene variation and microglial activity on [(11)C]PBR28 PET in older adults at risk for Alzheimer's disease. Multimodal Brain Image Anal. (2013) 8159:150–8. doi: 10.1007/978-3-319-02126-3_15
54. Lyoo CH, Ikawa M, Liow JS, Zoghbi SS, Morse CL, Pike VW, et al. Cerebellum can serve as a pseudo-reference region in alzheimer Disease to detect neuroinflammation measured with PET radioligand binding to translocator protein. J Nucl Med. (2015) 56:701–6. doi: 10.2967/jnumed.114.146027
55. Kreisl WC, Lyoo CH, Liow JS, Wei M, Snow J, Page E, et al. (11)C-PBR28 binding to translocator protein increases with progression of Alzheimer's disease. Neurobiol Aging (2016) 44:53–61. doi: 10.1016/j.neurobiolaging.2016.04.011
56. Kreisl WC, Lyoo CH, Liow JS, Snow J, Page E, Jenko KJ, et al. Distinct patterns of increased translocator protein in posterior cortical atrophy and amnestic Alzheimer's disease. Neurobiol Aging (2017) 51:132–40. doi: 10.1016/j.neurobiolaging.2016.12.006
57. Schain M, Zanderigo F, Ogden RT, Kreisl WC. Non-invasive estimation of [(11)C]PBR28 binding potential. Neuroimage (2018) 169:278–85. doi: 10.1016/j.neuroimage.2017.12.002
58. Santillo AF, Gambini JP, Lannfelt L, Langstrom B, Ulla-Marja L, Kilander L, et al. In vivo imaging of astrocytosis in Alzheimer's disease: an (1)(1)C-L-deuteriodeprenyl and PIB PET study. Eur J Nucl Med Mol Imaging (2011) 38:2202–8. doi: 10.1007/s00259-011-1895-9
59. Carter SF, Scholl M, Almkvist O, Wall A, Engler H, Langstrom B, et al. Evidence for astrocytosis in prodromal Alzheimer disease provided by 11C-deuterium-L-deprenyl: a multitracer PET paradigm combining 11C-Pittsburgh compound B and 18F-FDG. J Nucl Med. (2012) 53:37–46. doi: 10.2967/jnumed.110.087031
60. Choo IL, Carter SF, Scholl ML, Nordberg A. Astrocytosis measured by (1)(1)C-deprenyl PET correlates with decrease in gray matter density in the parahippocampus of prodromal Alzheimer's patients. Eur J Nucl Med Mol Imaging (2014) 41:2120–6. doi: 10.1007/s00259-014-2859-7
61. Rodriguez-Vieitez E, Carter SF, Chiotis K, Saint-Aubert L, Leuzy A, Scholl M, et al. Comparison of Early-Phase 11C-Deuterium-l-Deprenyl and 11C-Pittsburgh Compound B PET for assessing brain perfusion in Alzheimer disease. J Nucl Med. (2016) 57:1071–7. doi: 10.2967/jnumed.115.168732
62. Versijpt J, Decoo D, Van Laere KJ, Achten E, Audenaert K, D'Asseler Y, et al. 57Co SPECT, 99mTc-ECD SPECT, MRI and neuropsychological testing in senile dementia of the Alzheimer type. Nucl Med Commun. (2001) 22:713–9.
63. Le Fur G, Vaucher N, Perrier ML, Flamier A, Benavides J, Renault C, et al. Differentiation between two ligands for peripheral benzodiazepine binding sites, [3H]RO5-4864 and [3H]PK 11195, by thermodynamic studies. Life Sci. (1983) 33, 449–457.
64. Chauveau F, Boutin H, Van Camp N, Dolle F, Tavitian B. Nuclear imaging of neuroinflammation: a comprehensive review of [11C]PK11195 challengers. Eur J Nucl Med Mol Imaging (2008) 35:2304–19. doi: 10.1007/s00259-008-0908-9
65. Ramlackhansingh AF, Brooks DJ, Greenwood RJ, Bose SK, Turkheimer FE, Kinnunen KM, et al. Inflammation after trauma: microglial activation and traumatic brain injury. Ann Neurol. (2011) 70:374–83. doi: 10.1002/ana.22455
66. Varley J, Brooks DJ, Edison P. Imaging neuroinflammation in Alzheimer's disease and other dementias: recent advances and future directions. Alzheimers Dement. (2015) 11:1110–20. doi: 10.1016/j.jalz.2014.08.105
67. Roncaroli F, Su Z, Herholz K, Gerhard A, Turkheimer FE. TSPO expression in brain tumours: is TSPO a target for brain tumour imaging? Clin Transl Imaging (2016) 4:145–56. doi: 10.1007/s40336-016-0168-9
68. Venneti S, Lopresti BJ, Wang G, Hamilton RL, Mathis CA, Klunk WE, et al. PK11195 labels activated microglia in Alzheimer's disease and in vivo in a mouse model using PET. Neurobiol Aging (2009) 30:1217–26. doi: 10.1016/j.neurobiolaging.2007.11.005
69. Venneti S, Wiley CA, Kofler J. Imaging microglial activation during neuroinflammation and Alzheimer's disease. J Neuroimmune Pharmacol. (2009) 4:227–43. doi: 10.1007/s11481-008-9142-2
70. Calsolaro V, Edison P. Neuroinflammation in Alzheimer's disease: current evidence and future directions. Alzheimers Dement. (2016) 12:719–32. doi: 10.1016/j.jalz.2016.02.010
71. Fan Z, Calsolaro V, Atkinson RA, Femminella GD, Waldman A, Buckley C, et al. Flutriciclamide (18F-GE180) PET: first-in-human PET study of novel third-generation in vivo marker of human translocator protein. J Nucl Med. (2016) 57:1753–9. doi: 10.2967/jnumed.115.169078
72. Lockhart A, Davis B, Matthews JC, Rahmoune H, Hong G, Gee A, et al. The peripheral benzodiazepine receptor ligand PK11195 binds with high affinity to the acute phase reactant alpha1-acid glycoprotein: implications for the use of the ligand as a CNS inflammatory marker. Nucl Med Biol. (2003) 30:199–206.
73. Fujimura Y, Ikoma Y, Yasuno F, Suhara T, Ota M, Matsumoto R, et al. Quantitative analyses of 18F-FEDAA1106 binding to peripheral benzodiazepine receptors in living human brain. J Nucl Med. (2006) 47:43–50.
74. Fujita M, Imaizumi M, Zoghbi SS, Fujimura Y, Farris AG, Suhara T, et al. Kinetic analysis in healthy humans of a novel positron emission tomography radioligand to image the peripheral benzodiazepine receptor, a potential biomarker for inflammation. Neuroimage (2008) 40:43–52. doi: 10.1016/j.neuroimage.2007.11.011
75. Chauveau F, Van Camp N, Dolle F, Kuhnast B, Hinnen F, Damont A, et al. Comparative evaluation of the translocator protein radioligands 11C-DPA-713, 18F-DPA-714, and 11C-PK11195 in a rat model of acute neuroinflammation. J Nucl Med. (2009) 50:468–76. doi: 10.2967/jnumed.108.058669
76. Kreisl WC, Fujita M, Fujimura Y, Kimura N, Jenko KJ, Kannan P, et al. Comparison of [(11)C]-(R)-PK 11195 and [(11)C]PBR28, two radioligands for translocator protein (18 kDa) in human and monkey: implications for positron emission tomographic imaging of this inflammation biomarker. Neuroimage (2010) 49:2924–32. doi: 10.1016/j.neuroimage.2009.11.056
77. Holland JP, Liang SH, Rotstein BH, Collier TL, Stephenson NA, Greguric I, et al. Alternative approaches for PET radiotracer development in Alzheimer's disease: imaging beyond plaque. J Labelled Comp Radiopharm. (2014) 57:323–31. doi: 10.1002/jlcr.3158
78. Dupont AC, Largeau B, Santiago Ribeiro MJ, Guilloteau D, Tronel C, Arlicot N. Translocator Protein-18 kDa (TSPO) Positron Emission Tomography (PET) imaging and its clinical impact in neurodegenerative diseases. Int J Mol Sci. (2017) 18:785. doi: 10.3390/ijms18040785
79. Tronel C, Largeau B, Santiago Ribeiro MJ, Guilloteau D, Dupont AC, Arlicot N. Molecular targets for PET imaging of activated microglia: the current situation and future expectations. Int J Mol Sci. (2017) 18:802. doi: 10.3390/ijms18040802
80. Owen DR, Yeo AJ, Gunn RN, Song K, Wadsworth G, Lewis A, et al. An 18-kDa translocator protein (TSPO) polymorphism explains differences in binding affinity of the PET radioligand PBR28. J Cereb Blood Flow Metab. (2012) 32:1–5. doi: 10.1038/jcbfm.2011.147
81. Wadsworth H, Jones PA, Chau WF, Durrant C, Fouladi N, Passmore J, et al. [(1)(8)F]GE-180: a novel fluorine-18 labelled PET tracer for imaging Translocator protein 18 kDa (TSPO). Bioorg Med Chem Lett. (2012) 22:1308–13. doi: 10.1016/j.bmcl.2011.12.084
82. Ikawa M, Lohith TG, Shrestha S, Telu S, Zoghbi SS, Castellano S, et al. 11C-ER176, a Radioligand for 18-kDa translocator protein, has adequate sensitivity to robustly image all three affinity genotypes in human brain. J Nucl Med. (2017) 58:320–5. doi: 10.2967/jnumed.116.178996
83. Lagarde J, Sarazin M, Bottlaender M. In vivo PET imaging of neuroinflammation in Alzheimer's disease. J Neural Transm (2018) 125:847–67. doi: 10.1007/s00702-017-1731-x
84. Donat CK, Mirzaei N, Tang SP, Edison P, Sastre M. Imaging of microglial activation in Alzheimer's Disease by [(11)C]PBR28 PET. Methods Mol Biol. (2018) 1750:323–39. doi: 10.1007/978-1-4939-7704-8_22
85. Hamelin L, Lagarde J, Dorothee G, Potier MC, Corlier F, Kuhnast B, et al. Distinct dynamic profiles of microglial activation are associated with progression of Alzheimer's disease. Brain (2018) 141:1855–70. doi: 10.1093/brain/awy079
86. Rapic S, Backes H, Viel T, Kummer MP, Monfared P, Neumaier B, et al. Imaging microglial activation and glucose consumption in a mouse model of Alzheimer's disease. Neurobiol Aging (2013) 34:351–4. doi: 10.1016/j.neurobiolaging.2012.04.016
87. Mirzaei N, Tang SP, Ashworth S, Coello C, Plisson C, Passchier J, et al. In vivo imaging of microglial activation by positron emission tomography with [(11)C]PBR28 in the 5XFAD model of Alzheimer's disease. Glia (2016) 64:993–1006. doi: 10.1002/glia.22978
88. Walker MD, Dinelle K, Kornelsen R, Lee NV, Miao Q, Adam M, et al. [11C]PBR28 PET imaging is sensitive to neuroinflammation in the aged rat. J Cereb Blood Flow Metab. (2015) 35:1331–8. doi: 10.1038/jcbfm.2015.54
89. James ML, Belichenko NP, Nguyen TV, Andrews LE, Ding Z, Liu H, et al. PET imaging of translocator protein (18 kDa) in a mouse model of Alzheimer's disease using N-(2,5-dimethoxybenzyl)-2-18F-fluoro-N-(2-phenoxyphenyl)acetamide. J Nucl Med. (2015) 56:311–6. doi: 10.2967/jnumed.114.141648
90. Liu B, Le KX, Park MA, Wang S, Belanger AP, Dubey S, et al. In Vivo detection of age- and disease-related increases in neuroinflammation by 18F-GE180 TSPO MicroPET imaging in wild-type and alzheimer's transgenic mice. J Neurosci. (2015) 35:15716–30. doi: 10.1523/JNEUROSCI.0996-15.2015
91. Brendel M, Probst F, Jaworska A, Overhoff F, Korzhova V, Albert NL, et al. Glial activation and glucose metabolism in a transgenic amyloid mouse model: a triple-Tracer PET Study. J Nucl Med. (2016) 57:954–60. doi: 10.2967/jnumed.115.167858
92. Lopez-Picon FR, Snellman A, Eskola O, Helin S, Solin O, Haaparanta-Solin M, et al. Neuroinflammation appears early on PET imaging and then plateaus in a mouse model of Alzheimer disease. J Nucl Med. (2018) 59:509–15. doi: 10.2967/jnumed.117.197608
93. James ML, Belichenko NP, Shuhendler AJ, Hoehne A, Andrews LE, Condon C, et al. [(18)F]GE-180 PET detects reduced microglia activation after LM11A-31 therapy in a mouse model of Alzheimer's Disease. Theranostics (2017) 7:1422–36. doi: 10.7150/thno.17666
94. Maeda J, Zhang MR, Okauchi T, Ji B, Ono M, Hattori S, et al. In vivo positron emission tomographic imaging of glial responses to amyloid-beta and tau pathologies in mouse models of Alzheimer's disease and related disorders. J Neurosci. (2011) 31:4720–30. doi: 10.1523/JNEUROSCI.3076-10.2011
95. Shukuri M, Mawatari A, Ohno M, Suzuki M, Doi H, Watanabe Y, et al. Detection of Cyclooxygenase-1 in activated microglia during amyloid plaque progression: PET Studies in Alzheimer's disease model mice. J Nucl Med. (2016) 57:291–6. doi: 10.2967/jnumed.115.166116
96. Teodoro R, Moldovan RP, Lueg C, Gunther R, Donat CK, Ludwig FA, et al. Radiofluorination and biological evaluation of N-aryl-oxadiazolyl-propionamides as potential radioligands for PET imaging of cannabinoid CB2 receptors. Org Med Chem Lett. (2013) 3:11. doi: 10.1186/2191-2858-3-11
97. Zimmer ER, Leuzy A, Benedet AL, Breitner J, Gauthier S, Rosa-Neto P. Tracking neuroinflammation in Alzheimer's disease: the role of positron emission tomography imaging. J Neuroinflammation (2014) 11:120. doi: 10.1186/1742-2094-11-120
98. Moldovan RP, Hausmann K, Deuther-Conrad W, Brust P. Development of highly affine and selective fluorinated cannabinoid type 2 receptor ligands. ACS Med Chem Lett. (2017) 8:566–71. doi: 10.1021/acsmedchemlett.7b00129
99. Han J, Liu H, Liu C, Jin H, Perlmutter JS, Egan TM, et al. Pharmacologic characterizations of a P2X7 receptor-specific radioligand, [11C]GSK1482160 for neuroinflammatory response. Nucl Med Commun. (2017) 38:372–82. doi: 10.1097/MNM.0000000000000660
100. Roberts JC, Friel SL, Roman S, Perren M, Harper A, Davis JB, et al. (2009) Autoradiographical imaging of PPARgamma agonist effects on PBR/TSPO binding in TASTPM mice. Exp Neurol. (2009) 216:459–70.
101. Haris M, Singh A, Cai K, Nath K, Crescenzi R, Kogan F, et al. MICEST: a potential tool for non-invasive detection of molecular changes in Alzheimer's disease. J Neurosci Methods (2013) 212:87–93. doi: 10.1016/j.jneumeth.2012.09.025
102. Crescenzi R, DeBrosse C, Nanga RP, Reddy S, Haris M, Hariharan H, et al. In vivo measurement of glutamate loss is associated with synapse loss in a mouse model of tauopathy. Neuroimage (2014) 101:185–92. doi: 10.1016/j.neuroimage.2014.06.067
103. Wells JA, O'Callaghan JM, Holmes HE, Powell NM, Johnson RA, Siow B, et al. In vivo imaging of tau pathology using multi-parametric quantitative MRI. Neuroimage (2015) 111:369–78. doi: 10.1016/j.neuroimage.2015.02.023
104. Johansson A, Engler H, Blomquist G, Scott B, Wall A, Aquilonius SM, et al. Evidence for astrocytosis in ALS demonstrated by [11C](L)-deprenyl-D2 PET. J Neurol Sci. (2007) 255:17–22. doi: 10.1016/j.jns.2007.01.057
105. Ruiz J, Martin I, Callado LF, Meana JJ, Barturen F, Garcia-Sevilla JA. Non-adrenoceptor [3H]idazoxan binding sites (I2-imidazoline sites) are increased in postmortem brain from patients with Alzheimer's disease. Neurosci Lett. (1993) 160:109–12.
106. Kawamura K, Naganawa M, Konno F, Yui J, Wakizaka H, Yamasaki T, et al. Imaging of I2-imidazoline receptors by small-animal PET using 2-(3-fluoro-[4-11C]tolyl)-4,5-dihydro-1H-imidazole ([11C]FTIMD). Nucl Med Biol. (2010) 37:625–35. doi: 10.1016/j.nucmedbio.2010.02.013
107. Kawamura K, Maeda J, Hatori A, Okauchi T, Nagai Y, Higuchi M, et al. In vivo and in vitro imaging of I(2) imidazoline receptors in the monkey brain. Synapse (2011) 65:452–5. doi: 10.1002/syn.20897
108. Kealey S, Turner EM, Husbands SM, Salinas CA, Jakobsen S, Tyacke RJ, et al. Imaging imidazoline-I2 binding sites in porcine brain using 11C-BU99008. J Nucl Med. (2013) 54:139–44. doi: 10.2967/jnumed.112.108258
109. Tyacke RJ, Fisher A, Robinson ES, Grundt P, Turner EM, Husbands SM, et al. Evaluation and initial in vitro and ex vivo characterization of the potential positron emission tomography ligand, BU99008 (2-(4,5-dihydro-1H-imidazol-2-yl)-1- methyl-1H-indole), for the imidazoline2 binding site. Synapse (2012) 66:542–51. doi: 10.1002/syn.21541
110. Parker CA, Nabulsi N, Holden D, Lin SF, Cass T, Labaree D, et al. Evaluation of 11C-BU99008, a PET ligand for the imidazoline2 binding sites in rhesus brain. J Nucl Med. (2014) 55:838–44. doi: 10.2967/jnumed.113.131854
111. Tyacke RJ, Myers JFM, Venkataraman A, Mick I, Turton S, Passchier J, et al. Evaluation of (11)C-BU99008, a positron emission tomography ligand for the Imidazoline2 binding site in human brain. J Nucl Med. (2018). doi: 10.2967/jnumed.118.208009. [Epub ahead of print].
112. Rodriguez-Vieitez E, Ni R, Gulyas B, Toth M, Haggkvist J, Halldin C, et al. Astrocytosis precedes amyloid plaque deposition in Alzheimer APPswe transgenic mouse brain: a correlative positron emission tomography and in vitro imaging study. Eur J Nucl Med Mol Imaging (2015) 42:1119–32. doi: 10.1007/s00259-015-3047-0
113. Tamguney G, Francis KP, Giles K, Lemus A, DeArmond SJ, Prusiner SB. Measuring prions by bioluminescence imaging. Proc Natl Acad Sci USA. (2009) 106:15002–6. doi: 10.1073/pnas.0907339106
114. Watts JC, Giles K, Grillo SK, Lemus A, DeArmond SJ, Prusiner SB. Bioluminescence imaging of Abeta deposition in bigenic mouse models of Alzheimer's disease. Proc Natl Acad Sci USA. (2011) 108:2528–33. doi: 10.1073/pnas.1019034108
115. Sydow A, Hochgrafe K, Konen S, Cadinu D, Matenia D, Petrova O, et al. Age-dependent neuroinflammation and cognitive decline in a novel Ala152Thr-Tau transgenic mouse model of PSP and AD. Acta Neuropathol Commun. (2016) 4:17. doi: 10.1186/s40478-016-0281-z
Keywords: inflammation, TSPO, Alzheimer's disease, positron emission tomography (PET), microglia, astrocyte, imaging
Citation: Edison P, Donat CK and Sastre M (2018) In vivo Imaging of Glial Activation in Alzheimer's Disease. Front. Neurol. 9:625. doi: 10.3389/fneur.2018.00625
Received: 21 May 2018; Accepted: 10 July 2018;
Published: 07 August 2018.
Edited by:
Beatriz Gomez Perez-Nievas, King's College London, United KingdomReviewed by:
Alberto Lleo, Hospital de la Santa Creu i Sant Pau, SpainNadia Canu, Università degli Studi di Roma Tor Vergata, Italy
Copyright © 2018 Edison, Donat and Sastre. This is an open-access article distributed under the terms of the Creative Commons Attribution License (CC BY). The use, distribution or reproduction in other forums is permitted, provided the original author(s) and the copyright owner(s) are credited and that the original publication in this journal is cited, in accordance with accepted academic practice. No use, distribution or reproduction is permitted which does not comply with these terms.
*Correspondence: Magdalena Sastre, bS5zYXN0cmVAaW1wZXJpYWwuYWMudWs=