- 1Department of Neurology, University Hospitals, Case Western Reserve University, Cleveland, OH, United States
- 2Daroff-Dell'Osso Ocular Motility Laboratory, Cleveland VA Medical Center, Cleveland, OH, United States
- 3NeuroMetrology Lab, Nuffield Department of Clinical Neurosciences, University of Oxford, Oxford, United Kingdom
- 4Nuffield Department of Surgical Sciences, University of Oxford, Oxford, United Kingdom
- 5Cole Eye Institute, Cleveland Clinic, Cleveland, OH, United States
Discovery of inter-latching circuits in the basal ganglia and invention of deep brain stimulation (DBS) for their modulation is a breakthrough in basic and clinical neuroscience. The DBS not only changes the quality of life of hundreds of thousands of people with intractable movement disorders, but it also offers a unique opportunity to understand how the basal ganglia interacts with other neural structures. An attractive yet less explored area is the study of DBS on eye movements and vestibular function. From the clinical perspective such studies provide valuable guidance in efficient programming of stimulation profile leading to optimal motor outcome. From the scientific standpoint such studies offer the ability to assess the outcomes of basal ganglia stimulation on eye movement behavior in cognitive as well as in motor domains. Understanding the influence of DBS on ocular motor function also leads to analogies to interpret its effects on complex appendicular and axial motor function. This review focuses on the influence of globus pallidus, subthalamic nucleus, and thalamus DBS on ocular motor and vestibular functions. The anatomy and physiology of basal ganglia, pertinent to the principles of DBS and ocular motility, is discussed. Interpretation of the effects of electrical stimulation of the basal ganglia in Parkinson's disease requires understanding of baseline ocular motor function in the diseased brain. Therefore we have also discussed the baseline ocular motor deficits in these patients and how the DBS changes such functions.
Introduction
Deep brain stimulation (DBS) is the standard of care in treatment of movement disorders including Parkinson's disease (PD), essential tremor, and dystonia. In addition to the compelling clinical benefits seen in the over 100,000 movement disorders patients treated worldwide, DBS also offers an opportunity to study the effects of electrical stimulation of the basal ganglia on the physiology of motor, sensory, or cognitive systems. The focus of this review is to discuss the effects of DBS of globus pallidus internus (GPi), subthalamic nucleus (STN), and thalamus on ocular motor and vestibular functions. While interpreting the effects of DBS on any aspect of physiology, it is critical to appreciate that such surgery is by definition performed on diseased brains and the effects of stimulation are conflated with the effects of the condition being treated. Nevertheless with a good understanding of the baseline eye movement abnormalities in the patient population concerned, and suitable healthy controls where needed, it is possible to gain insights into normal physiology, disease pathophysiology, and how DBS affects them (1).
How Does DBS Work?
Although DBS can dramatically improve the motor symptoms of PD, essential tremor, and dystonia, its physiological mechanism of action remains unclear. Because stimulation and lesional surgery at the same sites produce similar beneficial effects in PD, it was previously believed that DBS produced a “physiological ablation” of the target. This is consistent with a simple physiological model of the basal ganglia and PD, where symptoms are due to increased activity of the STN and GPi. It was, therefore, hypothesized that DBS improves clinical symptoms by suppressing the outflow of the basal ganglia.
This simple view is no longer tenable, but the mechanism of action of DBS remains poorly understood with several possible explanations (2–4). Stimulation almost certainly causes neuronal excitation, most likely of axons, which have lower firing thresholds than neuronal somata. The consequence of this is likely not to be simply the up-regulation or down-regulation of one or more nuclei, but rather the disruption of some form of pathological network activity. Contemporary studies suggest hyper-synchronization of spontaneous neural activity as a cause of tremor and rigidity. It follows that de-synchronization of such activity might resolve these symptoms. De-synchronization is possible by lesioning the parts of hyper-synchronized circuit (e.g., pallidotomy or thalamotomy), or electrically stimulating (thereby suppressing) the circuit (e.g., DBS) (5, 6). Another possible type of pathological activity is “neural noise” due to excessive random firing, as is seen in the medium spiny neurons of the striatum in PD. Such noise can interfere with information flow within the basal ganglia and it has been suggested that pallidal DBS might act to dampen the noise (7).
Applied Anatomy, Physiology, and Pathophysiology of Basal Ganglia in Ocular Motor Control
The frontal eye field (FEF), supplementary eye field (SEF), dorsolateral prefrontal cortex (DLPFC), and the parietal eye fields project to the basal ganglia, which then relay this input to the superior colliculus (8, 9). The cortical areas project to the caudate nucleus which sends direct inhibitory fibers to the substantia nigra pars reticulata (SNr) and an indirect projection to the STN via the external segment of the globus pallidus (Figure 1A) [The figure modified with permission from (10)]. The SNr maintains tonic GABAergic inhibition on the superior colliculus (11, 12); timely and transient cessation of such inhibitory control leads to timely saccade initiation (9, 13, 14). Lesions of the caudate nucleus decrease the velocity and the amplitude of saccades (15). Pharmacological inhibition of the SNr after muscimol injection results in saccadic intrusions and contralaterally directed spontaneous saccades (16). Electrical stimulation of the SNr results in reduced latency and hypometric memory or visually guided saccades (17). In pathological states such as PD, the superior colliculus remains in an inhibited state due to hyperactivity of the SNr (See thick arrow Figure 1B) [the figure modified with permission from (10)].
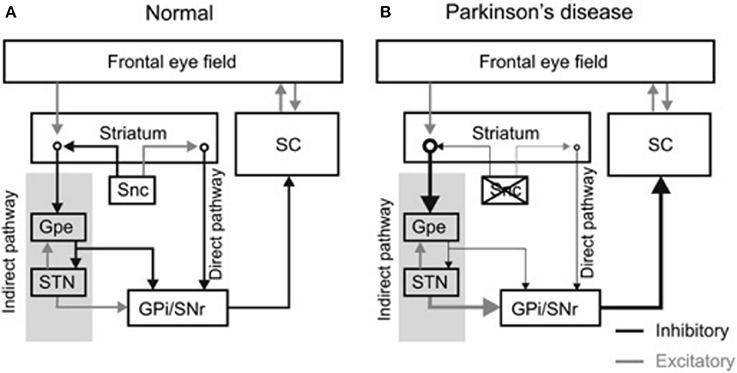
Figure 1. Diagram summarizes organization of the basal ganglia nuclei and their projections. (A) The substantia nigra pars compacta (Snc) sends excitatory and inhibitory projections to the striatum. Latter subsequently projects to the basal ganglia outflow nuclei—the globus pallidum internus (GPi) and substantia nigra pars reticulata (SNr) via, parallel, direct and indirect pathways. The direct pathway neurons in the striatum receive excitatory projections from the SNc and sends direct inhibitory projections to GPi and SNr, while inhibitory projections from SNc are sent to the striatal indirect pathway. The indirect pathway, via globus pallidum externus (GPe) and subthalamic nucleus (STN), influences the SNr and GPi. As a result of these connections the dopaminergic neuronal degeneration, as seen in Parkinson's disease, leads to disinhibition of GPi and SNr. Such dysinhibtion leads to inappropriate inhibition of the superior colliculus (SC) (relative thickness of the arrows represents the strength of projection (B). [Figure and corresponding legend modified with permission from (10)].
Eye Movement Abnormalities in PD
PD is a common neurodegenerative disorder with a complex and variable mixture of clinical features including slowness of movements (bradykinesia), resting tremor, shuffling gait, mask-like facies, and increased muscle tone. A wide range of eye movement abnormalities have been reported in PD, affecting smooth pursuit, vergence, fixation, saccades, and gaze holding (10, 18–23). Abnormalities in smooth pursuit eye movements include a reduction in the eye velocity relative to target movement, i.e., decreased smooth pursuit gain. The reduction in gain worsens as the disease progresses (21). Insufficiency of both convergence and divergence has been described (24–26). A reduction in smooth pursuit gain, mild restriction in upgaze (27), and convergence insufficiency is also seen in elderly who are otherwise healthy, but is more marked in PD. Subjects with PD have saccadic intrusions during attempted steady gaze fixation, followed by return; these movements are called square-wave jerks (10, 20, 28). While square-waves [see Figures 2A,C; the figure reproduced with permission from 10] are often notable in healthy individuals (29), their hypometric characteristic followed by a catch up saccade, just like hypometric visually guided saccades (see Figure 2B), (“staircase square-waves”) is a unique feature of PD [see Figures 2A,D; the figure reproduced with permission from (10)].
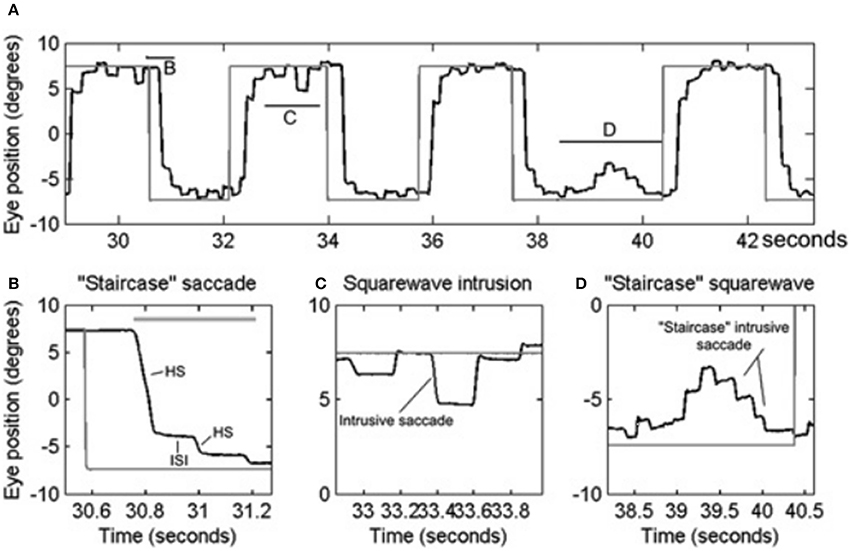
Figure 2. Example of saccadic and fixation deficit in one patient with early Parkinson's disease. Horizontal eye positions are depicted with black trace; the gray trace represents the visual target position. (A) Saccade and fixation abnormality is summarized from one patient. There is hypometria of initial saccade and it is then followed by several hypometric corrective saccades (HS). Latter brings the gaze to the target. Horizontal lines in (A) depict the portion of the eye movement trace that is temporally zoomed in (B–D). Thick, horizontal, gray line is the target acquisition time. (C) Depicts an example of square-wave jerk (SWJ), while (D) shows “staircase” square-wave jerk. ISI, inter-saccadic interval. [Figure and legend reproduced with permission from (10)].
Several studies have shown abnormalities in visually guided saccades in subjects with PD. Visually guided prosaccades (saccades toward a novel target) show increased latency (30–34), which progresses over time. Analyzing the distribution of latencies over multiple trials can help in the differential diagnosis of PD and other parkinsonian syndromes (35). Surprisingly, treatment with levodopa, despite alleviating motor symptoms, further increases latency (36). Patients with PD also often have difficulty in inhibiting the reflexive prosaccadic response or initiating a voluntary response in the opposite direction during the antisaccade task. This leads to a higher than normal antisaccadic error rate (AER) (37, 38).
Saccades in parkinsonian patients show an increased prevalence of hypometria, compared to healthy controls (10, 22). Asymmetry of hypometria can be seen in subjects with asymmetric PD, the saccades being more hypometric on the more symptomatic side (39). Series of hypometric saccades that ultimately leads to shift the gaze to its target appears like a “staircase” (40, 41). While staircases increase the time taken to reach the target of interest, this does not imply reduction in the saccade velocity (10). Although there is increased variability in the peak velocity, only the subjects with advanced PD have saccade slowing (21, 28, 42). Correlation between eye movement abnormalities and freezing of gait has also been observed in PD (20, 43, 44). PD patients with freezing of gait have increased antisaccade latency and variability in the saccade velocity and accuracy (44).
Effects of DBS on Saccades
DBS is known to influence some saccade abnormalities; for example, prosaccade latency is reduced by the STN or GPi DBS (45–47). DBS is unique in doing this: both levodopa treatment and lesional surgery do the opposite, despite the fact that both of these treatments produce symptomatic improvement. This is further evidence that the physiological mechanism of action of DBS is not simply a physiological ablation. The STN not only facilitates saccades, but also plays a critical role in their planning. Recordings of local field potentials from STN DBS electrodes show event related desynchronizations in the beta band immediately prior to saccades (48) that are qualitatively similar to those seen in motor planning of limb movement.
It has been proposed that the basal ganglia comprise a network that is capable of performing Bayesian statistics for movement selection (49, 50). In this scheme the STN feedback assures that increase in probability of one action is linked with reduction in the probability of other available options (51). Studies examining the effects of STN DBS on saccade generation have provided evidence for this concept. PD patients were asked to make horizontal saccades to a target that appeared either to the left or to the right. The investigators periodically changed the probability of the target appearing in each location and found that saccadic latencies shortened as the target location became more probable, or vice versa. When DBS was turned on, the latency for the more probable target location remained shortened, but the reaction time for less probable locations failed to increase. Disrupting the STN output interfered with the normalized representation of prior probabilities (7, 52).
Both STN and GPi DBS improve prosaccadic latencies. Interestingly GPi stimulation (but not STN stimulation) also reduces the AER (53). While changes in prosaccade latency could be accounted for exclusively by effects within the basal ganglia, the antisaccade task involves higher level functions including inhibition of a reflexive prosaccade and the subsequent volitional generation of a saccade in the opposite direction, both of which are functions of the prefrontal cortex. The results of this study imply that GPi DBS can improve deficits in higher control of lower motor functions (53).
Effects of DBS on Smooth Pursuit Eye Movements
Video-oculography in 34 patients with PD revealed decreased smooth pursuit eye movement velocity compared to the velocity of a pursued target (i.e., reduced smooth pursuit gain) (54). There is conflicting literature on the effects of DBS on smooth pursuit eye movements. In one study including 14 STN DBS patients, stimulation did not affect pursuit eye movements (54), while in another study of 9 DBS patients both smooth pursuit velocity and accuracy were significantly increased with stimulation (55). Globus pallidus, via thalamic relay, projects to the smooth pursuit areas of the frontal eye fields (56, 57). It is therefore possible that basal ganglia outflow modulates the extended cortical network responsible for smooth pursuit (55).
Effects of DBS on Gaze Holding
Square-wave jerks are defined by spontaneous intrusive saccades that takes the gaze away from the target followed by a return saccade within 200 ms intersaccadic interval (58). Although square-wave jerks are common in atypical parkinsonism such as multiple system atrophy and progressive supranuclear palsy; approximately 20% of patients with early idiopathic PD (10, 20, 23). Square-wave jerks in early PD are often interrupted, giving the appearance of a “staircase” [see Figures 2A,D; the figure reproduced with permission from (10)]. We hypothesized that enhanced inhibition of the superior colliculus in the parkinsonian state may lead to reduced inhibition of presaccadic activity FEF activity leading to its phasic increase and subsequently the square-wave jerk (10). Interruptions of the large intrusive saccades comprising the square-wave jerk, due to phasic SNr inhibition, leads to its “staircase” pattern [see Figures 2A,D] (10) [The figure reproduced with permission from (10)].
Pallidotomy increases the frequency of square wave jerks in patients with PD (59). As an explanation, it was proposed that the disinhibition of the ascending thalamocortical loops can reactivate the prefrontal cortex creating imbalance in the activity of the saccade-related prefrontal structures such as the frontal eye fields and supplementary motor eye fields (59). In a study examining the effects of STN DBS on square-wave jerks, the authors found reduction in the frequency of intrusive saccades after bilateral DBS (60).
Single-Unit Activity in Human Basal Ganglia and Eye Movements
The functional changes in the ocular motor system resulting from pallidal and subthalamic DBS prompt a fundamental question—is the STN or pallidum directly related to ocular motor control, i.e., do eye movement sensitive neurons exist in the STN or pallidum, or does the stimulation indirectly affect downstream ocular motor sensitive areas such as the SNr or superior colliculus to manifest its effects? Intraoperative microelectrode recordings and single channel electrooculography in 19 PD patients addressed this question. Intraoperatively, while simultaneously sampling single unit activity and oculography, the patients were asked to view a series of colored pictures and perform a visually guided saccade task (61). About one-fifth of neurons isolated from the SNr, globus pallidus, and STN had direct eye movement sensitivity (61).
DBS and Eyelid Motor Control
DBS in a rodent model of parkinsonism was recently used to study blink hyper-reflexia, impaired reflex blink plasticity, and reduced spontaneous blink rate (62). A hyper-synchronized beta-band in the basal ganglia output was found to be associated with such blink abnormalities. High-frequency DBS of the STN affected blink hyper-reflexia and blink reflex plasticity; however there were no effects on the abnormal rate and rhythm of the spontaneous blinks (62). It is possible that that the stimulated area of STN was away from that controlling spontaneous blink rate and pattern. It is also possible that DBS parameters used in the experiments were not suitable to change the spontaneous blink abnormality. Finally it can also be speculated that DBS does not affect the discharge pattern of the basal ganglia activity, but it simply reduces the neural response gain. A clinical study showed that pallidal DBS in humans with tardive as well as axial dystonia improves forced eyelid closures (blepharospasm) (63, 64).
Effects of DBS on the Vestibular System
The central vestibular system facilitates stable gaze holding, the motion perception, and orientation. The brainstem, under the cerebellar guidance, modulates these functions. In support of this theory, contemporary investigations identified the disorders of human brain where motion perception is selectively affected (65–76). The discovery of non-eye movement sensitive brainstem and cerebellar vestibular neurons further supported this concept (65, 67–69, 77, 78). The non-eye movement sensitive brainstem and cerebellar neurons may have specialized role in encoding a central representation of the gravitational force (65, 67, 70), heading direction (66, 70, 79). The cerebellum sends direct projections, via the vestibulo-thalamic track (fibers that are adjacent to the medial lamniscus but medial and then dorso-medial to the STN), to the ventro-posterior and ventro-lateral thalamus (77, 78, 80). It is therefore predicted that inadvertent stimulation of the vestibulo-thalamic projections can lead to abnormal interpretation of gravitational force. A recent study investigated the effects of DBS of the nucleus ventralis intermedius of the thalamus revealed change in sense of visual verticality (subjective visual vertical) when electrical stimulation was turned on. The patients felt a tilt at 1.4 ± 0.4 degrees on the contraversive side when the stimulator was on; in contrast when stimulator was turned off, the contraversive tilt was 4.4 ± 3.0 degrees (81).
Inadvertent stimulation of medial longitudinal fasciculus or the interstitial nucleus of Cajal can lead to isolated ipsilateral head tilt (82). It is also possible that altered perception of visual vertical (orientation of self in relation to the gravity), due to inadvertent stimulation of the vestibulo-thalamic fibers, have led to reactive head tilt. Recent study investigated the effects of STN stimulation through the medial and caudal DBS electrode contact in five PD subjects (83). Imaging and electrode location from one subject is depicted in Figure 3 [The figure modified with permission from (83)]. Perception of rotational motion in the plane of horizontal semicircular canal was most commonly reported by these patients, one patient also felt as if she was riding a swing. Latter form of complex perception could be due to the combined stimulation of the vestibulo-thalamic fibers conducting vertical semicircular canals and otolith derived signals, i.e., combination of pitch and fore-aft motion respectively. These serendipitous findings brought new insight into counter-intuitively implementing DBS for the treatment of vertigo and imbalance due to abnormal motion perception.
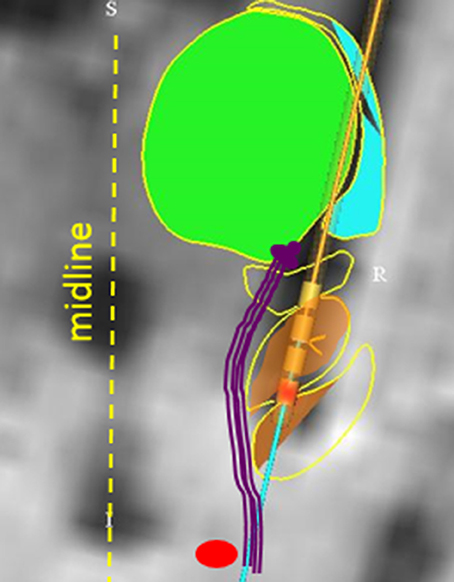
Figure 3. Anatomical model that reconstructed the basal ganglia subnuclei and coordinates of DBS electrode placed in STN. The green area is the model fitted to the thalamus, orange area (yellow arrow) is fitted to STN. The red circle depicts red nucleus. Vestibulo-thalamic fibers (schematized with purple lines) are medial to the STN before they course on the medio-dorsal border of STN to enter the thalamus. DBS electrode leads are depicted with four cylinder shapes (black arrow); red colored cylinder is contact #0 on the implanted lead (Medtronic 3389). [Figure and corresponding legend modified with permission from (83)].
Conclusions
Although DBS is predominantly viewed as modulating appendicular and axial motor function, tremor, and dystonia, it also has a substantial influence on ocular motor and vestibular function. Clinically, knowledge of how these systems may be affected by stimulation, together with knowledge of the anatomical locations of motor structures relative to ocular motor regions, is critical for safe and effective programming of stimulation parameters. The underlying mechanism of action of DBS is unclear, and because the neurophysiology of eye movements is better understood, the studies of DBS on ocular motor function may offer analogies that help to interpret its effects on appendicular and axial motor function. We also acknowledge that on several occasions the DBS studies have resulted in inconsistent effects on ocular motor function. Such incoherencies could result from institutional (minor) variabilities in DBS electrode placements as well as the variabilities in tested therapeutic electrode contacts. The next generation of studies correlating the outcome of DBS on ocular motor parameters and electrical tissue activation models are desperately needed.
Author Contributions
AS conceptualized the manuscript, authored manuscript. CA, JF, and FG edited manuscript.
Conflict of Interest Statement
The authors declare that the research was conducted in the absence of any commercial or financial relationships that could be construed as a potential conflict of interest.
Acknowledgments
This work was supported by grants from Dystonia Coalition (AS), Dystonia Medical Research Foundation (AS), Blind Children's Foundation (FG), and RPB Unrestricted grant CCLCM-CWRU.
References
1. FitzGerald JJ, Antoniades CA. Eye movements and deep brain stimulation. Curr Opin Neurol. (2016) 29:69–73. doi: 10.1097/WCO.0000000000000276
2. Benazzouz A, Hallett M. Mechanism of action of deep brain stimulation. Neurology (2000) 55(12 Suppl. 6):S13–6.
3. Dostrovsky JO, Levy R, Wu JP, Hutchison WD, Tasker RR, Lozano AM. Microstimulation-induced inhibition of neuronal firing in human globus pallidus. J Neurophysiol. (2000) 84:570–4. doi: 10.1152/jn.2000.84.1.570
4. Montgomery E. B. Jr, Gale JT. Mechanisms of action of deep brain stimulation(DBS). Neurosci Biobehav Rev. (2008) 32:388–407. doi: 10.1016/j.neubiorev.2007.06.003
5. Herzog J, Hamel W, Wenzelburger R, Potter M, Pinsker MO, Bartussek J, et al. Kinematic analysis of thalamic versus subthalamic neurostimulation in postural and intention tremor. Brain (2007) 130:1608–25. doi: 10.1093/brain/awm077
6. Groppa S, Herzog J, Falk D, Riedel C, Deuschl G, Volkmann J. Physiological and anatomical decomposition of subthalamic neurostimulation effects in essential tremor. Brain (2014) 137:109–21. doi: 10.1093/brain/awt304
7. Antoniades CA, Rebelo P, Kennard C, Aziz TZ, Green AL, FitzGerald JJ. Pallidal deep brain stimulation improves higher control of the oculomotor system in Parkinson's disease. J Neurosci (2015) 35:13043–52. doi: 10.1523/JNEUROSCI.2317-15.2015
8. Alexander GE, DeLong MR, Strick PL. Parallel organization of functionally segregated circuits linking basal ganglia and cortex. Annu Rev Neurosci. (1986) 9:357–81. doi: 10.1146/annurev.ne.09.030186.002041
9. Hikosaka O, Takikawa Y, Kawagoe R. Role of the basal ganglia in the control of purposive saccadic eye movements. Physiol Rev. (2000) 80:953–78. doi: 10.1152/physrev.2000.80.3.953
10. Shaikh AG, Xu-Wilson M, Grill S, Zee DS. 'Staircase' square-wave jerks in early Parkinson's disease. Br J Ophthalmol. (2011) 95:705–9. doi: 10.1136/bjo.2010.179630
11. Francois C, Percheron G, Yelnik J. Localization of nigrostriatal, nigrothalamic and nigrotectal neurons in ventricular coordinates in macaques. Neuroscience (1984) 13:61–76. doi: 10.1016/0306-4522(84)90259-8
12. Fisher RS, Buchwald NA, Hull CD, Levine MS. The GABAergic striatonigral neurons of the cat: demonstration by double peroxidase labeling. Brain Res. (1986) 398:148–56. doi: 10.1016/0006-8993(86)91260-6
13. Handel A, Glimcher PW. Quantitative analysis of substantia nigra pars reticulata activity during a visually guided saccade task. J Neurophysiol. (1999) 82:3458–75. doi: 10.1152/jn.1999.82.6.3458
14. Hikosaka O. GABAergic output of the basal ganglia. Prog Brain Res. (2007) 160:209–26. doi: 10.1016/S0079-6123(06)60012-5
15. Kato M, Miyashita N, Hikosaka O, Matsumura M, Usui S, Kori A. Eye movements in monkeys with local dopamine depletion in the caudate nucleus. I. Deficits in spontaneous saccades. J Neurosci. (1995) 15:912–27.
16. Wurtz RH, Hikosaka O. Role of the basal ganglia in the initiation of saccadic eye movements. Prog Brain Res. (1986) 64:175–90. doi: 10.1016/S0079-6123(08)63412-3
17. Basso MA, Liu P. Context-dependent effects of substantia nigra stimulation on eye movements. J Neurophysiol. (2007) 97:4129–42. doi: 10.1152/jn.00094.2007
18. DeJong JD, Jones GM. Akinesia, hypokinesia, and bradykinesia in the oculomotor system of patients with Parkinson's disease. Exp Neurol. (1971) 32:58–68. doi: 10.1016/0014-4886(71)90165-8
20. Rascol O, Clanet M, Montastruc JL, Simonetta M, Soulier-Esteve MJ, Doyon B, et al. Abnormal ocular movements in Parkinson's disease. Evidence for involvement of dopaminergic systems. Brain (1989) 112 (Pt 5), 1193–1214. doi: 10.1093/brain/112.5.1193
21. Rottach KG, Riley DE, DiScenna AO, Zivotofsky AZ, Leigh RJ. Dynamic properties of horizontal and vertical eye movements in parkinsonian syndromes. Ann Neurol. (1996) 39:368–77. doi: 10.1002/ana.410390314
22. Terao Y, Fukuda H, Yugeta A, Hikosaka O, Nomura Y, Segawa M, et al. Initiation and inhibitory control of saccades with the progression of Parkinson's disease - changes in three major drives converging on the superior colliculus. Neuropsychologia (2011) 49:1794–806. doi: 10.1016/j.neuropsychologia.2011.03.002
23. Otero-Millan J, Schneider R, Leigh RJ, Macknik SL, Martinez-Conde S. Saccades during attempted fixation in parkinsonian disorders and recessive ataxia: from microsaccades to square-wave jerks. PLoS ONE (2013) 8:e58535. doi: 10.1371/journal.pone.0058535
24. Repka MX, Claro MC, Loupe DN, Reich SG. Ocular motility in Parkinson's disease. J Pediatr Ophthalmol Strabismus (1996) 33:144–147.
25. Nowacka B, Lubinski W, Honczarenko K, Potemkowski A, Safranow K. Ophthalmological features of Parkinson disease. Med Sci Monit. (2014) 20:2243–9. doi: 10.12659/MSM.890861
26. Hanuska J, Bonnet C, Rusz J, Sieger T, Jech R, Rivaud-Pechoux S, et al. Fast vergence eye movements are disrupted in Parkinson's disease: a video-oculography study. Parkinsonism Relat Disord. (2015) 21:797–9. doi: 10.1016/j.parkreldis.2015.04.014
27. Clark RA, Demer JL. Effect of aging on human rectus extraocular muscle paths demonstrated by magnetic resonance imaging. Am J Ophthalmol. (2002) 134:872–8. doi: 10.1016/S0002-9394(02)01695-1
28. White OB, Saint-Cyr JA, Tomlinson RD, Sharpe JA. Ocular motor deficits in Parkinson's disease. II. Control of the saccadic and smooth pursuit systems. Brain (1983) 106 (Pt 3), 571–87.
29. Abadi RV, Gowen E. Characteristics of saccadic intrusions. Vision Res. (2004) 44:2675–90. doi: 10.1016/j.visres.2004.05.009
30. Armstrong IT, Chan F, Riopelle RJ, Munoz DP. Control of saccades in Parkinson's disease. Brain Cogn. (2002) 49:198–201.
31. MacAskill MR, Anderson TJ, Jones RD. Adaptive modification of saccade amplitude in Parkinson's disease. Brain (2002) 125:1570–82. doi: 10.1093/brain/awf168
32. Chan F, Armstrong IT, Pari G, Riopelle RJ, Munoz DP. Deficits in saccadic eye-movement control in Parkinson's disease. Neuropsychologia (2005) 43:784–96. doi: 10.1016/j.neuropsychologia.2004.06.026
33. Chambers JM, Prescott TJ. Response times for visually guided saccades in persons with Parkinson's disease: a meta-analytic review. Neuropsychologia (2010) 48:887–99. doi: 10.1016/j.neuropsychologia.2009.11.006
34. Terao Y, Fukuda H, Ugawa Y, Hikosaka O. New perspectives on the pathophysiology of Parkinson's disease as assessed by saccade performance: a clinical review. Clin Neurophysiol. (2013) 124:1491–506. doi: 10.1016/j.clinph.2013.01.021
35. Antoniades CA, Bak TH, Carpenter RH, Hodges JR, Barker RA. Diagnostic potential of saccadometry in progressive supranuclear palsy. Biomark Med. (2007) 1:487–90. doi: 10.2217/17520363.1.4.487
36. Hood AJ, Amador SC, Cain AE, Briand KA, Al-Refai AH, Schiess MC, et al. Levodopa slows prosaccades and improves antisaccades: an eye movement study in Parkinson's disease. J Neurol Neurosurg Psychiatry (2007) 78:565–70. doi: 10.1136/jnnp.2006.099754
37. Kitagawa M, Fukushima J, Tashiro K. Relationship between antisaccades and the clinical symptoms in Parkinson's disease. Neurology (1994) 44:2285–9. doi: 10.1212/WNL.44.12.2285
38. Rivaud-Pechoux S, Vidailhet M, Brandel JP, Gaymard B. Mixing pro- and antisaccades in patients with parkinsonian syndromes. Brain (2007) 130 (Pt 1):256–64. doi: 10.1093/brain/awl315
39. Choi SM, Lee SH, Choi KH, Nam TS, Kim JT, Park MS, et al. Directional asymmetries of saccadic hypometria in patients with early Parkinson's disease and unilateral symptoms. Eur Neurol. (2011) 66:170–4. doi: 10.1159/000330671
40. Kimmig H, Haussmann K, Mergner T, Lucking CH. What is pathological with gaze shift fragmentation in Parkinson's disease? J Neurol. (2002) 249:683–92. doi: 10.1007/s00415-002-0691-7
41. Blekher T, Weaver M, Rupp J, Nichols WC, Hui SL, Gray J, et al. Multiple step pattern as a biomarker in Parkinson disease. Parkinsonism Relat Disord. (2009) 15:506–10. doi: 10.1016/j.parkreldis.2009.01.002
42. Vidailhet M, Rivaud S, Gouider-Khouja N, Pillon B, Bonnet AM, Gaymard B, et al. Eye movements in parkinsonian syndromes. Ann Neurol. (1994) 35:420–6. doi: 10.1002/ana.410350408
43. Lohnes CA, Earhart GM. Saccadic eye movements are related to turning performance in Parkinson disease. J Parkinsons Dis. (2011) 1:109–18. doi: 10.3233/JPD-2011-11019
44. Nemanich ST, Earhart GM. Freezing of gait is associated with increased saccade latency and variability in Parkinson's disease. Clin Neurophysiol. (2016) 127:2394–401. doi: 10.1016/j.clinph.2016.03.017
45. Temel Y, Visser-Vandewalle V, Carpenter RHS. Saccadic latency during electrical stimulation of the human subthalamic nucleus. Curr Biol. (2008) 18:R412–14. doi: 10.1016/j.cub.2008.03.008
46. Yugeta A, Terao Y, Fukuda H, Hikosaka O, Yokochi F, Okiyama R, et al. Effects of STN stimulation on the initiation and inhibition of saccade in Parkinson disease. Neurology (2010) 74:743–748. doi: 10.1212/WNL.0b013e3181d31e0b
47. Antoniades CA, Buttery P, FitzGerald JJ, Barker RA, Carpenter RH, Watts C. Deep brain stimulation: eye movements reveal anomalous effects of electrode placement and stimulation. PLoS ONE (2012) 7:e32830. doi: 10.1371/journal.pone.0032830
48. Yugeta A, Hutchison WD, Hamani C, Saha U, Lozano AM, Hodaie M, et al. Modulation of Beta oscillations in the subthalamic nucleus with prosaccades and antisaccades in Parkinson's disease. J Neurosci. (2013) 33:6895–904. doi: 10.1523/JNEUROSCI.2564-12.2013
49. Redgrave P, Prescott TJ, Gurney K. The basal ganglia: a vertebrate solution to the selection problem? Neuroscience (1999) 89:1009–23.
50. Obeso JA, Rodriguez-Oroz MC, Rodriguez M, Lanciego JL, Artieda J, Gonzalo N, et al. Pathophysiology of the basal ganglia in Parkinson's disease. Trends Neurosci. (2000) 23:S8–19. doi: 10.1016/S1471-1931(00)00028-8
51. Mink JW. The basal ganglia: focused selection and inhibition of competing motor programs. Prog Neurobiol. (1996) 50:381–425. doi: 10.1016/S0301-0082(96)00042-1
52. Antoniades CA, Bogacz R, Kennard C, FitzGerald JJ, Aziz T, Green AL. Deep brain stimulation abolishes slowing of reactions to unlikely stimuli. J Neurosci. (2014) 34:10844–52. doi: 10.1523/JNEUROSCI.1065-14.2014
53. Straube A, Ditterich J, Oertel W, Kupsch A. Electrical stimulation of the posteroventral pallidum influences internally guided saccades in Parkinson's disease. J Neurol. (1998) 245:101–5. doi: 10.1007/s004150050186
54. Pinkhardt EH, Jurgens R, Lule D, Heimrath J, Ludolph AC, Becker W, et al. Eye movement impairments in Parkinson's disease: possible role of extradopaminergic mechanisms. BMC Neurol. (2012) 12:5. doi: 10.1186/1471-2377-12-5
55. Nilsson MH, Patel M, Rehncrona S, Magnusson M, Fransson PA. Subthalamic deep brain stimulation improves smooth pursuit and saccade performance in patients with Parkinson's disease. J Neuroeng Rehabil. (2013) 10:33. doi: 10.1186/1743-0003-10-33
56. Tian Lynch JC. Subcortical input to the smooth and saccadic eye movement subregions of the frontal eye field in Cebus monkey. J Neurosci. (1997) 17:9233–47. doi: 10.1523/JNEUROSCI.17-23-09233.1997
57. Lynch JC, Tian JR. Cortico-cortical networks and cortico-subcortical loops for the higher control of eye movements. Prog Brain Res. (2006) 151:461–501. doi: 10.1016/S0079-6123(05)51015-X
58. Leigh RJ, Zee DS. The Neurology of Eye Movements. New York, NY; Oxford: Oxford University Press (2015).
59. Averbuch-Heller L, Stahl JS, Hlavin ML, Leigh RJ. Square-wave jerks induced by pallidotomy in parkinsonian patients. Neurology (1999) 52:185–8. doi: 10.1212/WNL.52.1.185
60. Wark HA, Garell PC, Walker AL, Basso MA. A case report on fixation instability in Parkinson's disease with bilateral deep brain stimulation implants. J Neurol Neurosurg Psychiatry (2008) 79:443–7. doi: 10.1136/jnnp.2007.117192
61. Cavanagh JF, Wiecki TV, Cohen MX, Figueroa CM, Samanta J, Sherman SJ, et al. Subthalamic nucleus stimulation reverses mediofrontal influence over decision threshold. Nat Neurosci. (2011) 14:1462–7. doi: 10.1038/nn.2925
62. Kaminer J, Thakur P, Evinger C. Effects of subthalamic deep brain stimulation on blink abnormalities of 6-OHDA lesioned rats. J Neurophysiol. (2015) 113:3038–46. doi: 10.1152/jn.01072.2014
63. Shaikh AG, Mewes K, Jinnah HA, DeLong MR, Gross RE, Triche S, et al. Globus pallidus deep brain stimulation for adult-onset axial dystonia. Parkinsonism Relat Disord. (2014) 20:1279–82. doi: 10.1016/j.parkreldis.2014.09.005
64. Shaikh AG, Mewes K, DeLong MR, Gross RE, Triche SD, Jinnah HA, et al. Temporal profile of improvement of tardive dystonia after globus pallidus deep brain stimulation. Parkinsonism Relat Disord. (2015) 21:116–9. doi: 10.1016/j.parkreldis.2014.11.013
65. Angelaki DE, Shaikh AG, Green AM, Dickman JD. Neurons compute internal models of the physical laws of motion. Nature (2004) 430:560–4. doi: 10.1038/nature02754
66. Shaikh AG, Meng H, Angelaki DE. Multiple reference frames for motion in the primate cerebellum. J Neurosci. (2004) 24:4491–7. doi: 10.1523/JNEUROSCI.0109-04.2004
67. Green AM, Shaikh AG, Angelaki DE. Sensory vestibular contributions to constructing internal models of self-motion. J Neural Eng. (2005) 2:S164–79. doi: 10.1088/1741-2560/2/3/S02
68. Shaikh AG, Ghasia FF, Dickman JD, Angelaki DE. Properties of cerebellar fastigial neurons during translation, rotation, and eye movements. J Neurophysiol. (2005) 93:853–63. doi: 10.1152/jn.00879.2004
69. Shaikh AG, Green AM, Ghasia FF, Newlands SD, Dickman JD, Angelaki DE. Sensory convergence solves a motion ambiguity problem. Curr Biol. (2005) 15:1657–62. doi: 10.1016/j.cub.2005.08.009
70. Yakusheva TA, Shaikh AG, Green AM, Blazquez PM, Dickman JD, Angelaki DE. Purkinje cells in posterior cerebellar vermis encode motion in an inertial reference frame. Neuron (2007) 54:973–85. doi: 10.1016/j.neuron.2007.06.003
71. Bertolini G, Ramat S, Laurens J, Bockisch CJ, Marti S, Straumann D, et al. Velocity storage contribution to vestibular self-motion perception in healthy human subjects. J Neurophysiol. (2011) 105:209–23. doi: 10.1152/jn.00154.2010
72. Bertolini G, Ramat S, Bockisch CJ, Marti S, Straumann D, Palla A. Is vestibular self-motion perception controlled by the velocity storage? Insights from patients with chronic degeneration of the vestibulo-cerebellum. PLoS ONE (2012) 7:e36763. doi: 10.1371/journal.pone.0036763
73. Shaikh AG, Palla A, Marti S, Olasagasti I, Optican LM, Zee DS, et al. Role of cerebellum in motion perception and vestibulo-ocular reflex-similarities and disparities. Cerebellum (2013) 12:97–107. doi: 10.1007/s12311-012-0401-7
74. Shaikh AG. Motion perception without Nystagmus–a novel manifestation of cerebellar stroke. J Stroke Cerebrovasc Dis. (2014) 23:1148–56. doi: 10.1016/j.jstrokecerebrovasdis.2013.10.005
75. Bertolini G, Wicki A, Baumann CR, Straumann D, Palla A. Impaired tilt perception in Parkinson's disease: a central vestibular integration failure. PLoS ONE (2015) 10:e0124253. doi: 10.1371/journal.pone.0124253
76. Yousif N, Bhatt H, Bain PG, Nandi D, Seemungal BM. The effect of pedunculopontine nucleus deep brain stimulation on postural sway and vestibular perception. Eur J Neurol. (2016) 23:668–70. doi: 10.1111/ene.12947
77. Meng H, May PJ, Dickman JD, Angelaki DE. Vestibular signals in primate thalamus: properties and origins. J Neurosci. (2007) 27:13590–602. doi: 10.1523/JNEUROSCI.3931-07.2007
78. Meng H, Angelaki DE. Responses of ventral posterior thalamus neurons to three-dimensional vestibular and optic flow stimulation. J Neurophysiol. (2010) 103:817–26. doi: 10.1152/jn.00729.2009
79. Liu S, Yakusheva T, Deangelis GC, Angelaki DE. Direction discrimination thresholds of vestibular and cerebellar nuclei neurons. J Neurosci. (2010) 30:439–48. doi: 10.1523/JNEUROSCI.3192-09.2010
80. Hawrylyshyn PA, Rubin AM, Tasker RR, Organ LW, Fredrickson JM. Vestibulothalamic projections in man–a sixth primary sensory pathway. J Neurophysiol. (1978) 41:394–401. doi: 10.1152/jn.1978.41.2.394
81. Baier B, Vogt T, Rohde F, Cuvenhaus H, Conrad J, Dieterich M. (2015). Deep brain stimulation of the nucleus ventralis intermedius: a thalamic site of graviceptive modulation. Brain Struct Funct. 222:645–50. doi: 10.1007/s00429-015-1157-x
82. Mike A, Balas I, Varga D, Janszky J, Nagy F, Kovacs N. Subjective visual vertical may be altered by bilateral subthalamic deep brain stimulation. Mov Disord. (2009) 24:1556–7. doi: 10.1002/mds.22605
Keywords: Parkinson's disease, tremor, dystonia, neuromodulation, saccade, pursuit, gaze holding
Citation: Shaikh AG, Antoniades C, Fitzgerald J and Ghasia FF (2018) Effects of Deep Brain Stimulation on Eye Movements and Vestibular Function. Front. Neurol. 9:444. doi: 10.3389/fneur.2018.00444
Received: 12 December 2017; Accepted: 25 May 2018;
Published: 12 June 2018.
Edited by:
Caroline Tilikete, Institut National de la Santé et de la Recherche Médicale (INSERM), FranceReviewed by:
Christopher Charles Glisson, Michigan State University, United StatesTim Anderson, University of Otago, Christchurch, New Zealand
Copyright © 2018 Shaikh, Antoniades, Fitzgerald and Ghasia. This is an open-access article distributed under the terms of the Creative Commons Attribution License (CC BY). The use, distribution or reproduction in other forums is permitted, provided the original author(s) and the copyright owner are credited and that the original publication in this journal is cited, in accordance with accepted academic practice. No use, distribution or reproduction is permitted which does not comply with these terms.
*Correspondence: Aasef G. Shaikh, YWFzZWZzaGFpa2hAZ21haWwuY29t