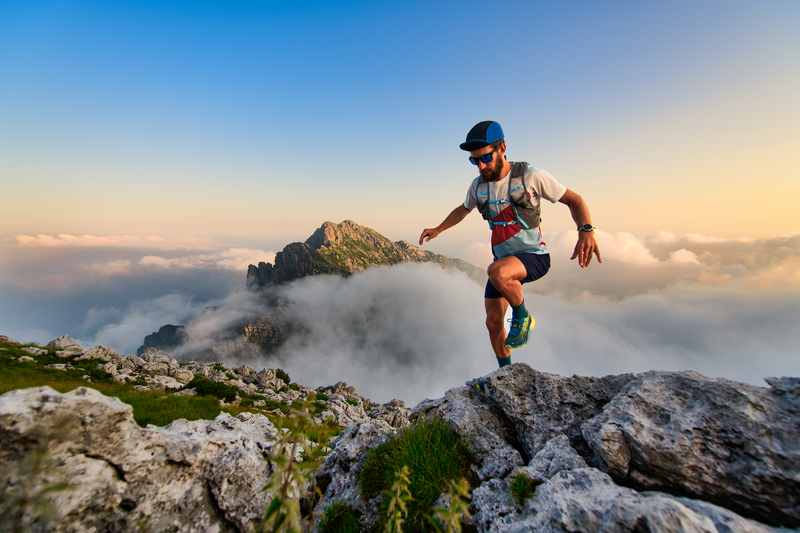
95% of researchers rate our articles as excellent or good
Learn more about the work of our research integrity team to safeguard the quality of each article we publish.
Find out more
ORIGINAL RESEARCH article
Front. Neurol. , 04 July 2018
Sec. Neurotrauma
Volume 9 - 2018 | https://doi.org/10.3389/fneur.2018.00425
This article is part of the Research Topic An Update on Cognitive Neuroscience and Neurorehabilitation: From Bench to Clinic View all 6 articles
Olfactory bulb (OB) plays an important role in protecting against harmful substances via the secretion of antioxidant and detoxifying enzymes. Environmental enrichment (EE) is a common rehabilitation method and known to have beneficial effects in the central nervous system. However, the effects of EE in the OB still remain unclear. At 6 weeks of age, CD-1® (ICR) mice were assigned to standard cages or EE cages. After 2 months, we performed proteomic analysis. Forty-four up-regulated proteins were identified in EE mice compared to the control mice. Gene Ontology analysis and Kyoto Encyclopedia of Genes and Genomes Pathway demonstrated that the upregulated proteins were mainly involved in metabolic pathways against xenobiotics. Among those upregulated proteins, 9 proteins, which participate in phase I or II of the xenobiotic metabolizing process and are known to be responsible for ROS detoxification, were validated by qRT-PCR. To explore the effect of ROS detoxification mediated by EE, glutathione activity was measured by an ELISA assay. The ratio of reduced glutathione to oxidized glutathione was significantly increased in EE mice. Based on a linear regression analysis, GSTM2 and UGT2A1 were found to be the most influential genes in ROS detoxification. For further analysis of neuroprotection, the level of iNOS and the ratio of Bax to Bcl-2 were significantly decreased in EE mice. While TUNEL+ cells were significantly decreased, Ki67+ cells were significantly increased in EE mice, implicating that EE creates an optimal state for xenobiotic metabolism and antioxidant activity. Taken together, our results suggested that EE protects olfactory layers via the upregulation of glutathione-related antioxidant and xenobiotic metabolizing enzymes, eventually lowering ROS-mediated inflammation and apoptosis and increasing neurogenesis. This study may provide an opportunity for a better understanding of the beneficial effects of EE in the OB.
The olfactory bulb (OB) is a region of brain that plays a critical role in health and pathological behaviors such as the detection of hazards in the environment, food selection, olfactory memory formation, mating, and maintenance of mood (1–4). Tight communication between the OB and other olfactory systems can transfer xenobiotics to the brain by the active axonal transport of olfactory neurons, bypassing the blood brain barrier (5). To maintain cellular integrity and homeostasis of the brain from environmental chemicals, it is necessary to have active xenobiotic metabolism during the olfactory transport (6).
Xenobiotic metabolism is a metabolism pathway that modifies the chemical structure of foreign, either exogenous or endogenous, substances so that maintain homeostasis and cellular integrity. Xenobiotic metabolism process includes three steps; Phase I modifies xenobiotics via hydrolysis, oxidation, and reduction and thereby produces the active metabolites. Phase II further conjugates the phase I product with transferase enzymes to yield hydrophilic products. Lastly, Phase III excretes the final modified product out of the cells via various transporters (6). Namely, metabolizing enzymes such as the cytochrome P450 (CYPs) family, the UDP-glucuronosyltransferases (UGT) family, glutathione-transferases (GST) family and the aldehyde dehydrogenase (ADH) family are involved in the detoxification process (6, 7). Especially, the phase II enzymes such as UGT and GST family plays an important role in determining the rate of xenobiotic metabolism and ROS detoxification (8).
Previous studies have shown olfactory bulbectomy in rodents leads to cortical and hippocampal cell death (9, 10), suggesting that the OB is related to other brain regions. Moreover, the OB is a special nervous structure that changes its neural cells over lifetime. Several studies have shown that newly generated cells in the subventricular zone (SVZ) of adult mouse migrate into the OB through the rostral migratory stream (RMS), forming functional granule cells in the OB and constituting the network of the OB to other brain regions (11, 12). Since the OB constantly interacts with external environment and encounters harmful air-borne chemicals, the above ongoing process is important for proper OB function (13).
Environmental enrichment (EE) is a rehabilitation strategy for rodents and the enhancement of the brain by surroundings that include various objects such as running wheels, novel toys and allow large social interactions to experience the effects of a complex combination of physical, cognitive, and social stimulation in the brain (14–16). In many studies, EE has been implicated in improvement of the brain functions such as neuroprotection and neurogenesis in an animal model of aging (17–20), ischemic brain injury (14), and neurodegenerative diseases (14, 21). These diseases and brain injuries, accompanied with an increase in oxidative stress and olfactory impairment, eventually lead to a clinically significant problem (22, 23). However, this event can be reversed by exercise via the increased expression of antioxidant enzymes (17, 24).
Previous studies have investigated the effect of EE, odor enrichment, and xenobiotic inhalant on the OB of normal mice (25–27). Allowing xenobiotic inhalants or various odorants in a timely fashion, it could induce changes in gene expressions and odor perception (25, 26). Several studies have reported the beneficial effect of EE on the OB (25, 27). In contrast to the previous studies, our study focuses on the effect of EE on the OB of normal mice without utilizing any inhalant or timely-exposure odorants.
The aim of this study was to determine whether there are alterations in physiological responses after long-term exposure to EE in the intact OB of normal mice. Here, we showed the expression of metabolizing enzymes, which were predominated in the OB of EE mice. Moreover, we found that EE enhances neurogenesis and ROS detoxification, resulting in the reduction of inflammation and apoptotic process. These results imply that EE has beneficial effects on the OB through setting an optimal status for antioxidant and xenobiotic metabolism.
At 6 weeks of age, a total of CD-1® (ICR) mice were assigned randomly to either environmental enrichment (EE) or standard cage (SC) group. For 2 months, EE mice were housed in a huge cage (86 × 76 × 31 cm3) containing novel objects, such as tunnels, shelters, toys, and running wheels for voluntary exercise, and allowing for large social interaction (12–15 mice/cage) (Figure 1A) while control mice were housed in standard cages (27 × 22.5 × 14 cm3) without any novel objects (4–5 mice/cage) (Figures 1B,C) (14). All animals were housed in a facility accredited by the Association for Assessment and Accreditation of Laboratory Animal Care (AAALAC) and were given ad libitum access to food and water under alternating 12-h light/dark cycles. Used mice of this study were all female mice and purchased from the Jackson Laboratory.
Figure 1. Experimental design for environmental enrichment. (A) Mice in an enriched environment accessed freely not only to water and food but also to tunnels, shelters, toys, running wheels, and the large space of social interaction. (B,C) Mice in a standard cage freely accessed to water and food. (D) A schematic time schedule for the experiment. Mice of 6 weeks of age were randomly assigned and reared in either a control cage or EE cage for 2 months. After the housing conditions, brain tissues were harvested for proteomic analysis to see differentially expressed proteins. Long-term exposure to EE during adulthood is known to induce the beneficial effects on brain health with cellular and molecular alterations.
All procedures were reviewed and approved by the Animal Care and Use Committee of the Yonsei University College of Medicine (Identification code: 2014-0125-3). All procedures were in accordance with the guidelines of the National Institutes of Health's Guide for the Care and Use of Laboratory Animals. These regulations, notifications, and guidelines originated and were modified from the Animal Protection Law (2008), the Laboratory Animal Act (2008), and the Eighth Edition of the Guide for the Care and Use of Laboratory Animals (NRC 2011). They were sacrificed at 8 weeks after the housing conditions under ketamine (100 mg/kg) and xylazine (10 mg/kg) anesthesia by intraperitoneal injection. Thereafter, they were given an intracardial perfusion of 4% paraformaldehyde, and the brain tissues were harvested for histological assessments. All efforts were made to minimize animal suffering.
In this study, a total of 28 CD-1® (ICR) female mice at 6 weeks of age were randomly housed either in environmental enrichment (N = 14) or standard cages (N = 14). Among the subjects, 3 mice per group were recruited for the proteomics analysis after housing for 2 months (N = 6). To validate the results of proteomics and measure iNOS levels, 4 mice per group were further recruited at 8 weeks post-conditions (N = 8). Moreover, 3 mice per group were recruited for an ELISA assay to measure glutathione activity and apoptosis process (N = 6). Finally, 4 mice per group were further recruited for histological assessments (N = 8). A schematic timeline for this study is described in Figure 1D.
Olfactory bulb of mouse brain tissues was homogenized in 200 μl of cold RIPA buffer (50 mM Tris-HCl, pH 7.5, 1% Triton X-100, 150 mM NaCl, 0.1% sodium dodecyl sulfate (SDS), 1% sodium deoxycholate) with a protease inhibitor cocktail (Sigma-Aldrich, St. Louis, MO, USA) in the ice. The tissue lysates were centrifugated for 20 min at 13,000 g at 4°C. The supernatant containing the protein extracts were stored at −80°C until use. The protein concentration of the supernatant was determined using Bradford assay, according to the manufacturer's instructions.
Protein samples were digested by in-solution method after quantified using a bicinchoninic acid (BCA) assay kit (Thermo Scientific, Foster City, CA, USA). Briefly, samples were resolved in a digestion solution of 6 M urea (Sigma-Aldrich) and 50 mM tris-HCl in water. Protein reduction was performed with 10 mM DTT (Sigma-Aldrich) for 1 h at 37°C. Additionally, 55 mM iodoacetamide (Sigma-Aldrich) were treated for 30 min at room temperature in darkness for alkylation of cysteine residues. The resulting samples were digested by sequencing-grade modified trypsin (Promega, Madison, WI, USA) overnight at 37°C. The digested peptides were desalted using C18 Spin-Column (Thermo Scientific).
For TMT labeling, digested peptides were resuspended in 200 mM triethyl ammonium bicarbonate (TEAB, Sigma-Aldrich). TMT™ Isobaric Mass Tagging reagent (Thermo Scientific) were dissolved in anhydrous acetonitrile and added to each sample. After incubation for 1 h at room temperature, the reaction was quenched with 5% hydroxylamine. The labeled samples were pooled into one tubes and separated with an Agilent 3100 OFF-GEL fractionator system (Agilent Technologies, Santa Clara, CA, USA) according to manufacturer's instructions. Finally, resulting samples were desalted using C18 Spin-Column (Thermo Scietific).
Prepared samples were analyzed using Q Exactive mass spectrometer (Thermo Finnigan, San Jose, CA, USA) coupled with EASY-nLC 1000 (Thermo Finnigan). The tryptic peptides were loaded into a trap column (3 μm sized C18 resin into 75 μm × 2 cm) and separated by the analytical column (3 μm sized C18 resin into 75 μm × 50 cm) at a flow rate of 300 nL/min. The separated peptides were eluted by solvent A (0.1% formic acid in water) and solvent B (0.1% formic acid in acetonitrile) with a linear gradient for 60 min. Each MS scan was followed by 8 MS/MS scans of the most abundant peak to the eighth-most abundant peak of the MS scan in data-dependent method with dynamic exclusion options (repeat duration was 30 s and repeat count was set to 1). The normalized collision energy for MS/MS was 27%. To identify peptides, TurboSEQUEST (Thermo Electron, San Jose, CA, USA) was used to search MS/MS spectra against the Uniprot database for forward sequences (17098 Mus musculus protein sequence entries as of July 15, 2014). The mass tolerance was set to 10 ppm for precursor ions, 0.8 Da for fragment ions. Carbamidomethylation of cysteine (+57.0215 Da) and oxidation of methionine (+15.9949 Da) were set as the static modifications and variable modifications, respectively. Scaffold Q+S (version 4.3.4, Proteome Software Inc., OR, USA) was used to compute probability of proteins and peptides (28). Search results were validated using the protein and peptide cut-off probabilities with >99% and 95% probability, respectively. Spectral counts were calculated by Scaffold software after normalization by total spectral counts of each MS run for an estimate of expression fold change between the experimental groups (29). An absorbance spectra peak for each interested protein is provided in Figure S1.
To identify protein function of the differentially expressed genes, gene ontology (GO) was used for further analysis. GO annotation analysis with the Database for Annotation, Visualization and Integrated Discovery (DAVID) annotation tool describes protein function with three basic categories: associated biological process (BP), molecular function (MF) and cellular components (CC). This categorization gives an overview of the interested protein functions. Moreover, pathway enrichment analysis was performed using the Kyoto Encyclopedia of Genes and Genomes (KEGG) pathway. Pathways that are up-regulated in EE mice compared to control mice were identified. Within the identified pathways, genes that are involved in metabolism, drug metabolism, and xenobiotic metabolism were sorted out for further validation (Tables 1– 3).
Total RNA was extracted from tissue samples as previously described (30). Agilent 2100 Bioanalyzer (Agilent Technologies, Palo Alto, CA, USA) with the A260/A280 ratio for quality control analysis was used to evaluate purity of RNA extracted from olfactory bulb tissues, and visual valuation of the 28S:18S rRNA ratio using gel electrophoresis was used to evaluate the RNA integrity.
To further validate proteomic analysis, qRT-PCR for interested differentially expressed genes was performed in triplicate. One microgram of purified total RNA was used for reverse transcription into cDNA using a ReverTra Ace® qPCR TR master Mix with gDNA remover (TOYOBO). The expression of each gene of interest was profiled using a LightCycler 480 (Roche Applied Science, Mannheim, Germany) using the LightCycler 480 SYBR Green master mix (Roche Applied Science). The validated genes are as follows: subfamily A7 (ALDH1A7); Carboxylesterase 1D (CES1D); Cytochrome P450, family 2, subfamily a, polypeptide 5 (CYP2A5); Serum paraoxonase 1 (PON1); Cytochrome P450, family 1, subfamily a, polypeptide 2 (CYP1A2); Dihydropyrimidinase (DPYS); Alcohol dehydrogenase 1 (ADH1); Alcohol dehydrogenase [NADP(+)] (AKR1A1); Crystalline, lambda1 (CRYL1), forward 5′-3′ and reverse 5′-3′; UDP-glucuronosyltransferase 2 family, polypeptide A1 (UGT2A1); Glutathione S-transferase, alpha3 (GSTA3); Glutathione S-transferase Mu 2 (GSTM2); Epoxide hydrolase 1 (EPHX1); Thiosulfate sulfurtransferase (TST). To confirm detoxifying activity by EE, we also identified iNOS level. And glyceraldehyde-3-phosphate dehydrogenase (GADPH) was used as the loading control. The expression level of each gene of interest was obtained using the 2−ΔΔCt method (31). Target-gene expression was normalized relative to the expression of GAPDH and represented as fold change relative to the control. The primer sequences are described in Table S1.
The concentration of total glutathione, oxidized glutathione, ratio of reduced glutathione to oxidized glutathione were measured in OB extracts using the glutathione detection kit from Enzo Life Sciences (Enzo Life Sciences, East Farmingdale, NY, USA, ADI-900-160) following the manufacturer's instructions. The values were obtained at 1:40 dilution and expressed as pmol/well and each well contained the total volume of 200 μl.
To measure the expression of apoptotic neuronal cells, the protein was extracted as described above. The equal amounts of protein samples (40 μg) were denatured and separated by 4–12% Bis-Tris gels in 1× NuPage MES SDS Running Buffer (Invitrogen, Eugene, OR, USA). Proteins were transferred at 4°C onto a polyvinylidene difluoride membrane (Invitrogen) in NuPage Transfer Buffer (Invitrogen) with 20% (vol/vol) methanol. Membranes were blocked and then incubated overnight at 4°C with the following antibodies: Anti-Bax (1: 1,000 dilution, Santa Cruz Biotechnology), Anti-Bcl-2 (1:1,000 dilution, Santa Cruz Biotechnology), and Anti-Actin (1:1,000 dilution, Santa Cruz Biotechnology). The next day, blots were washed three times with TBS plus Tween 20 and incubated at room temperature for 1 hr with horseradish peroxidase-conjugated secondary antibodies (1:3,000 dilution, Santa Cruz). An enhanced chemiluminescence detection system (Amersham Pharmacia Biotech, Little Chalfont, UK) was used to visualize the protein. The intensity of the protein bands was recorded with a Fujifilm LAS-3000 imager.
Immunohistochemistry was performed as previously described (32). The animals were sacrificed and perfused with 4% paraformaldehyde. The harvested brain tissues were cryosectioned at 16-μm thickness along the sagittal plane, and immunohistochemistry staining was performed on four sections over a range of >128 μm. Sections were stained with primary antibodies against Ki67 (1:200; Abcam), and secondary antibodies such as Alexa Fluor 488 goat anti-Rabbit (1:400; Invitrogen) and mounted on glass slides with fluorescent mounting medium containing 4′,6-diamidino-2-phenylindole (Vectorshield, Vector, Burlingame, CA). The stained sections were analyzed using confocal microscopy (LSM700; Zeiss, Gottingen, Germany). Ki67+ cell area (μm2) with respect to DAPI area (/μm2) was evaluated using ZEN Imaging Software (Blue edition; Zeiss).
For analysis of apoptosis, the selected areas of the RMS were analyzed for each group. Fluorometric TUNEL assay (Promega, Madison, WI, USA) was conducted according to the manufacturer's protocol. Images of apoptotic cells were taken using a fluorescent microscopy (LSM700), and positive apoptotic cells (μm2) with respect to DAPI area (/μm2) were quantified using ZEN Imaging Software (Blue edition; Zeiss).
Statistical analyses were performed using Statistical Package for Social Sciences (SPSS) software (IBM Corporation, Armonk, NY, USA; version 23.0). All data were expressed as median and interquartile range (25th−75th percentiles). Mann–Whitney U-tests were used to compare the difference between groups. Linear regression analysis was conducted to identify the relationship between xenobiotic metabolizing proteins and ROS detoxification (glutathione activity) and a univariate analysis was also used identify relationships between EE and xenobiotic metabolizing proteins and glutathione activity. A P < 0.05 was considered statistically significant.
After placing mice in either SC cage or EE cage for 2 months, the mice were sacrificed according to the time schedule (Figure 1D). The proteomic analysis revealed that 44 up-regulated proteins were identified in EE mice compared with control mice with a cut-off fold change >1.5 (p < 0.05) (Table 1). The up-regulated proteins in the OB of mice exposed to EE were categorized based on biological processes (BP), molecular functions (MF), and cellular components (CC) (Table 2). BP based GO analysis identified the up-regulated proteins were related to “oxidation-reduction process” (GO:0055114), “response to toxic substance” (GO:0009636), “epoxygenase P450 pathway” (GO:0019373), “metabolic process” (GO:0008152), “short-chain fatty acid catabolic process” (GO:0019626), “aromatic compound catabolic process” (GO:0019439), “ethanol catabolic process” (GO:0006068), “cellular aromatic compound metabolic process” (GO:0006725), “cellular response to cadmium ion” (GO:0071276), “retinoic acid metabolic process” (GO:0042573), and “drug metabolic process” (GO:0017144). Moreover, in Kyoto Encyclopedia of Genes and Genomes (KEGG) pathway, the most highlighted cluster in the up-regulated genes contains overall themes for metabolic pathways and xenobiotic metabolism (Table 3).
Table 2. Gene ontology with Database for Annotation, Visualization, and Integrated Discovery (DAVID) generated by EE up-regulated genes.
Table 3. Kyoto Encyclopedia of Genes and Genomes (KEGG) pathways identified to be significantly up-regulated from EE using Database for Annotation, Visualization, and Integrated Discovery (DAVID) software.
After 8 weeks of the exposure to EE or SC, mice were sacrificed, and the olfactory bulbs were extracted and lysed for proteomic and qPCR analysis. The expression level of 44 identified proteins were significantly different in terms of the expression level between control group and EE group. The up-regulated proteins via pathway analysis were validated using qRT-PCR analysis. Xenobiotic metabolizing phase I enzymes, such as ALDH1A7, DPYS, PON1, CRYL1, AKR1A1, CYP1A2, and xenobiotic metabolizing phase II enzymes, such as UGT2A1, GSTM2, GSTA3 were significantly increased in EE mice compared with control mice (Figure 2; p < 0.05).
Figure 2. Validation of the up-regulated proteins associated with xenobiotic metabolism. Among the 44 up-regulated proteins, which were associated with xenobiotic metabolism and detoxifying enzymes, a total of 9 genes, phase I (ALDH1A7, DPYS, PON1, CRYL1, AKR1A1, CYP1A2) (A) and phase II (UGT2A1, GSTIM2, GSTA3) (B), were significantly increased in EE mice compared to control mice (*p < 0.05, by Mann-Whitney U test). Values are median (interquartile range) and a red tick line indicates the relative expression of control mice.
Glutathione activity is important for the removal of ROS, which generated from endogenous environment and during xenobiotic metabolism, and glutathione redox status can be a marker for oxidative stress. The number of oxidized glutathione was significantly lower in EE mice compared to control mice (Figure 3A; p < 0.05), and the ratio of reduced glutathione (GSH) to oxidized glutathione (GSSG) was significantly higher in EE mice compared to control mice (Figure 3B; p < 0.05), and no significant difference in the number of total glutathione was observed in between EE mice and control mice (Data not shown).
Figure 3. Glutathione activity. The number of oxidized glutathione in EE mice was significantly decreased compared to control mice (A), and GSH/GSSG ratio was significantly increased in EE mice compared with control mice (B). Values are median (interquartile range). An asterisk indicates significant difference (*p < 0.05, by Mann–Whitney U-test).
Linear regression analysis was conducted to identify which xenobiotic metabolizing enzymes mostly contribute to glutathione activity (Table 4). In the analysis of oxidized glutathione, GSTM2 (p = 0.008), GSTA3 (p = 0.022), CYP2A5 (p = 0.004), ALDH1A7 (p = 0.009), and UGT2A1 (p = 0.002) were found to be significantly correlated. In the analysis of GSH/GSSG, GSTM2 (p = 0.003), GSTA3 (p = 0.008), CYP2A5 (p = 0.029), ALDH1A7 (p = 0.004), UGT2A1 (p = 0.003), CRYL1 (p = 0.049), AKR1A1 (p = 0.024), and DPYS (p = 0.016) were found to be significantly correlated. The final stepwise multiple regression model revealed a significant association between oxidized glutathione and UGT2A1 (p = 0.002), and significant associations between GSH/GSSG and GSTM2 (p = 0.024) and GSH/GSSG and UGT2A1 (p = 0.029). EE was significantly associated with GSTM2 (Coefficient (SE) = 0.625 (0.072); reference = SC; p < 0.001 and UGT2A1 (Coefficient (SE) = 2.688 (0.685); p < 0.001), individually. Also, EE was significantly correlated with glutathione activity-related factors including oxidized glutathione [Coefficient (SE) = −1.186 (0.319); p = 0.002] and the ratio of GSH to GSSG [Coefficient (SE) = 26.218 (5.403); p < 0.001], respectively.
Table 4. Linear regression analysis of xenobiotic metabolism-related genes for predicting glutathione activity.
The expression of iNOS is highly up-regulated when the cells are in oxidative stress, and the relationship between apoptotic markers (Bax and Bcl-2) is important for regulating cell apoptosis. To measure the expression of iNOS, RT-PCR was performed with the referenced primers (Table S1) (33). The representative figures of RT-PCR for the iNOS expression between EE and control group were shown in Figure 4A. The expression of iNOS in EE mice was significantly lower than that of control mice (Figure 4B; p < 0.05). To further evaluate the apoptotic process and neuroprotection in the OB, western blot was conducted. The representative figures of WB for Bax, Bcl-2, and Actin between EE and control group were shown in Figure 4C. The ratio of Bax, a pro-apoptosis marker to Bcl-2, an anti-apoptosis marker was significantly decreased in EE mice compared to control mice (Figure 4D; p < 0.05). Taken together, the upregulation of xenobiotic metabolizing enzymes mediated by EE induces glutathione-mediated ROS detoxification, eventually reducing oxidative stress and apoptosis process. IHC was performed to evaluate neuroprotection and cell proliferation in the RMS. The RMS is a structure that have a full of neuroblasts, which originated from the SVZ. These neuroblasts further migrate along the RMS into the OB, resulting in the replacement of OB interneurons. In the analysis of TUNEL assay, apoptotic cells were significantly decreased in the RMS of EE mice compared to control mice (Figures 5A–G; p < 0.05) In the analysis of newly generated cells, Ki67+ cells in the RMS were significantly increased in EE mice compared with control mice (Figures 5H–N; p < 0.05).
Figure 4. iNOS expression and apoptotic process. The expression of iNOS in the OB of EE mice were significantly decreased compared to control mice (A,B). Moreover, the ratio of Bax to Bcl-2 in EE mice was significantly decreased compared to control mice (C,D). Values are median (interquartile range). An asterisk indicates significant difference (*p < 0.05, by Mann–Whitney U-test).
Figure 5. Histological assessments for neuroprotection and cell proliferation in the RMS. TUNEL+ cells were indicated in green (A–F). TUNEL+ cells in EE mice were significantly decreased in the RMS compared to control mice (G; p < 0.05). Ki67+ cells were indicated in green (H–M). Ki67+ cells in EE mice were significantly increased in the RMS compared to control mice (N; p < 0.05). Values are median (interquartile range). An asterisk indicates significant difference (*p < 0.05, by Mann–Whitney U-test). Scale bars for (A,C,H,L) represent 100 μm, scale bars for (B,D,I,M) represent 20 μm, and scale bars for (E,F,J,K) represent 10 μm.
In this study, we provided evidences that EE has the protective effects on the OB through setting an optimal status for antioxidant and xenobiotic metabolism. The proteomic analysis revealed the DEPs in the OB of EE mice compared with control mice. GO analysis was conducted to identify protein function with DAVID 6.8 beta annotation tool and KEGG pathways. In the KEGG pathways, the most highlighted themes were general metabolism and xenobiotic metabolism within several biological processes.
To validate the expression of the up-regulated proteins, qRT-PCR was performed. Among the phase I metabolizing enzymes, ALDH1A7, DPYS, PON1, CRYL1, AKR1A1, CYP1A2 were found to be significantly increased in EE mice compared with control mice and among the phase II metabolizing enzymes, UGT2A1, GSTM2, GSTA3 were found to be significantly increased in EE mice compared with control mice. The activation ratio of phase I and phase II xenobiotic metabolism is important because the product of phase I could be more deleterious than the original form of xenobiotics. The phase-II genes encode for a battery of enzymes that are essential in the antioxidation and detoxification of xenobiotics and endogenous reactive electrophilic compounds (34). The transcriptional regulation of the phase II genes is achieved by the binding of nuclear factor erythroid2- related factor (Nrf2) to the consensus antioxidant response element (ARE) sequence in the promoter regions.
Nrf2 has been shown to regulate the expression of a group of genes encoding cytoprotective enzymes in response to cell exposure to certain toxicants, including reactive oxygen species (ROS), electrophiles, as well as dithiolethiones, isothiocyanates, and triterpenoids (35). The target genes include ones encoding such enzymes as antioxidative, catalyzing electrophile conjugation, glutathione homeostasis, producing reducing equivalents, proteasome function and other. Among others, glutathione reductase catalyzes the reduction of glutathione disulfide (GSSG) to the sulfhydryl form glutathione (GSH), which is a critical molecule in resisting oxidative stress and maintaining the reducing environment of the cell (36).
To further analyze the rate of phase II enzyme activity, one of the phase II enzymes, glutathione, the GSH/GSSG detection assay was performed. The GSH/GSSG ratio in the OB of EE mice was significantly higher than in the OB of control mice, and oxidized glutathione was significantly decreased in EE mice compared to control mice. However, the level of total glutathione was not significantly different between the groups. These results suggest that EE has beneficial effects on the OB of the mouse brain by setting an optimal status for getting rid of ROS via enhancement of antioxidant and detoxifying enzyme activity.
To further investigate the relationship between glutathione-mediated ROS detoxification and the validated up-regulated metabolizing enzymes, the regression analyses were conducted to identify whether the genes predict the glutathione activity. While GSTM2, GSTA3, CYP2A5, ALDH1A7, UGT2A1 were correlated in oxidized glutathione, GSTM2, GSTA3, CYP2A5, ALDH1A7, UGT2A1, CRYL1, AKR1A1, DPYS were correlated in GSH/GSSG ratio. The stepwise multiple linear regression indicated that UGT2A1 was the most influential gene in oxidized glutathione, and GSTM2 and UGT2A1 were the most influential genes in the GSH/GSSG ratio.
GSTM2 stabilizes reactive metabolites formed by CYPs, UGT, and ST, and the proper activity of GST isoenzymes is important for olfactory acuity (37). A fluctuation in the GST activity was observed in striatal neurons of Huntington's diseases, reflecting its protective role for oxidative damage to DNA, proteins, and lipids (38). The expression of GST activity is highly expressed in rat olfactory bulb compared to that of the cerebral cortex (37). Interestingly, the transcriptional level of Nrf2 is positively correlated to GST activities in most cases (39). GSTM2 also takes an important role in glutathione-mediated antioxidant activity and clearance of odorants (40).
UGTs, consisting of genetically variable family of enzymes, detoxify various exogenous xenobiotics from air pollutants to food and endogenous lipids (41). These enzymes involved in all three phases of xenobiotic metabolism (42) and are detectable in olfactive mucosa and olfactory bulb (43). Various isoforms of UGTs are highly expressed in the olfactory area. Their expression is highly related to the transfer rate of xenobiotics via the nasal pathway to the brain, the modulation of olfactory perception, and the detoxification of exogenous or endogenous compounds, which are important for physiological olfactory processing in the system (44).
During xenobiotic metabolism, the numerous amounts of ROS and reactive nitrogen species generate in the membrane of the cell and mitochondria. These reactive species can exert deleterious effects on DNA, protein, and membrane of the cell (44). Furthermore, excessive ROS generation or oxidative stress activates stress-mediated MAPKs, ultimately leading to cell death (37). The accumulation of endogenous ROS can be alleviated by either enzymatic or non-enzymatic antioxidants defense (45). In RT-PCR and WB analysis, the significant reductions of the iNOS expression and apoptotic process were observed in EE mice compared with control mice. This neuroprotective effect may be due to the up-regulation of glutathione activity and the reduction of ROS.
The OB relays various information from the olfactory epithelium, which has a direct contact with external environment, to the olfactory cortex (43, 46). When encountering and metabolizing xenobiotics, continuous neural regeneration and synaptic plasticity occur in sensory cell axons and mitral cells of the OB (6). Most of the progenitor cells from the subventricular zone that migrate along the migratory stream to the OB differentiate into granule cells (GC) (47). These cells are inhibitory interneurons, and their activity is extremely important to modulate mitral cell activity and to accurately shape the output from the OB to higher olfactory centers (38, 40). Notably, olfactory interneurons are continuously generated throughout adulthood and their integration in the OB circuits is regulated by sensory experience (48). Several studies have shown that exposing animals to an olfactory enrichment increases survival of adult-born neurons in the OB (49, 50). In the presence of powerful neurogenic stimulus, such as voluntary exercise, the proliferative capability of SVZ stem cells turns out to be more plastic (51). Moreover, EE increases neurogenesis in the granular cell layer (GCL) of the adult OB (52). Consistent with the previous studies, our results showed that EE significantly reduced the apoptosis of newly regenerated cells in the RMS.
Oxidative stress in brain is related with many factors such as environmental toxin, aging, and diseases. Previous studies have shown oxidative stress could be alleviated by environmental enrichment (17, 24, 53), and the dramatic effect can be shown in the aging rat brain (17, 24). EE also mediates various functional improvements via the increased expression of growth factors such as FGF-2 in the hypoxic ischemic mice (14). Moreover, EE also induces behavioral and functional improvement associated with synaptic plasticity and neurogenesis (54–57). However, the mechanisms underlying the effect of EE on xenobiotic metabolism in the OB are less clear. As indicated in the study of Yokota et al., EE during the perinatal period can significantly modulate the gene expression related to inflammation in the OB via the proper activation of xenobiotic metabolism, eventually resulting in neuroprotection (25). Consistent with the previous study, our results showed the beneficial effects of EE that can alleviate endogenous oxidative stress via the glutathione-mediated ROS detoxification in the adult mouse brain and can also mediate neuroprotection, resulting in more newly regenerated cells in the RMS and modulation of the OB circuits.
Previous studies suggested that the complexity of the environment is the key feature of EE that can provide a wide range of opportunities for stimulation in various brain regions (21, 58–60). In our study, we provided larger space with the items varying in size, shape, smell, and texture in EE. The active interaction between the mice and items available in the environment may lead to dramatic results in endogenous ROS detoxification of normal adult mice. However, since the activity response of antioxidant enzymes in the brain is probably dependent on various factors such as the duration of EE, sex, and strain of mice, further prospective studies to evaluate the effect of EE based on these factors are needed.
Since there is little information available for the effect of EE on the intact OB of normal mice, our study may provide a possible protective mechanism of EE. In the present study, the proteomic analysis revealed the DEPs, which are associated with metabolic pathways and xenobiotic metabolism, in EE mice compared with control mice. EE can induce the neuroprotective effects via the up-regulation of glutathione-associated antioxidant enzymes in the OB. EE also creates the optimal state against ROS by the up-regulation of GSH/GSSG ratio, down-regulation of iNOS expression and apoptotic process, and up-regulation of cell proliferation.
JHS and SP equally contributed to this study. JHS performed most of the experiments, analyzed data, wrote the manuscript, and contributed to the study concept and design. SP performed molecular study, analyzed data, wrote the manuscript, and contributed to English editing. Y-KS conducted statistical analysis. B-GN managed animals. JWK and KPK conducted proteomic analysis and LC-MS analysis. HYL and S-RC wrote the manuscript, developed the study concept, and supervised the project. All authors read and approved the manuscript.
The authors declare that the research was conducted in the absence of any commercial or financial relationships that could be construed as a potential conflict of interest.
This study was supported by grants from the National Research Foundation (NRF-5-2017-A0154-00395), the Ministry of Science and Technology, Republic of Korea, the Korean Health Technology R&D Project (HI16C1012), Ministry of Health & Welfare, Republic of Korea.
The Supplementary Material for this article can be found online at: https://www.frontiersin.org/articles/10.3389/fneur.2018.00425/full#supplementary-material
1. Song C, Leonard BE. The olfactory bulbectomised rat as a model of depression. Neurosci Biobehav Rev. (2005) 29:627–47. doi: 10.1016/j.neubiorev.2005.03.010
2. Moberg PJ, Turetsky BI. Scent of a disorder: olfactory functioning in schizophrenia. Curr Psychiatry Rep. (2003) 5:311–9. doi: 10.1007/s11920-003-0061-x
3. Keverne EB. Odor here, odor there: chemosensation and reproductive function. Nat Neurosci. (2005) 8:1637–8. doi: 10.1038/nn1205-1637
4. Yoon H, Enquist LW, Dulac C. Olfactory inputs to hypothalamic neurons controlling reproduction and fertility. Cell (2005) 123:669–82. doi: 10.1016/j.cell.2005.08.039
5. Sasajima H, Miyazono S, Noguchi T, Kashiwayanagi M. Intranasal administration of rotenone in mice attenuated olfactory functions through the lesion of dopaminergic neurons in the olfactory bulb. Neurotoxicology (2015) 51:106–15. doi: 10.1016/j.neuro.2015.10.006
6. Heydel JM, Coelho A, Thiebaud N, Legendre A, Le Bon AM, Faure P, et al. Odorant-binding proteins and xenobiotic metabolizing enzymes: implications in olfactory perireceptor events. Anat Rec. (2013) 296:1333–45. doi: 10.1002/ar.22735
7. Gachon F, Olela FF, Schaad O, Descombes P, Schibler U. The circadian PAR-domain basic leucine zipper transcription factors DBP, TEF, and HLF modulate basal and inducible xenobiotic detoxification. Cell Metab. (2006) 4:25–36. doi: 10.1016/j.cmet.2006.04.015
8. Bhattacharyya S, Sinha K, Sil PC. Cytochrome P450s: mechanisms and biological implications in drug metabolism and its interaction with oxidative stress. Curr Drug Metab. (2014) 15:719–42. doi: 10.2174/1389200215666141125121659
9. Hozumi S, Nakagawasai O, Tan-No K, Niijima F, Yamadera F, Murata A, et al. Characteristics of changes in cholinergic function and impairment of learning and memory-related behavior induced by olfactory bulbectomy. Behav Brain Res. (2003) 138:9–15. doi: 10.1016/S0166-4328(02)00183-3
10. Moriguchi S, Han F, Nakagawasai O, Tadano T, Fukunaga K. Decreased calcium/calmodulin-dependent protein kinase II and protein kinase C activities mediate impairment of hippocampal long-term potentiation in the olfactory bulbectomized mice. J Neurochem. (2006) 97:22–9. doi: 10.1111/j.1471-4159.2006.03710.x
11. Imayoshi I, Sakamoto M, Ohtsuka T, Takao K, Miyakawa T, Yamaguchi M, et al. Roles of continuous neurogenesis in the structural and functional integrity of the adult forebrain. Nat Neurosci. (2008) 11:1153–61. doi: 10.1038/nn.2185
12. Kelsch W, Sim S, Lois C. Watching synaptogenesis in the adult brain. Annu Rev Neurosci. (2010) 33:131–49. doi: 10.1146/annurev-neuro-060909-153252
13. Baker H, Franzen L, Stone D, Cho JY, Margolis FL. Expression of tyrosine hydroxylase in the aging, rodent olfactory system. Neurobiol Aging (1995) 16:119–28.
14. Seo JH, Yu JH, Suh H, Kim MS, Cho SR. Fibroblast growth factor-2 induced by enriched environment enhances angiogenesis and motor function in chronic hypoxic-ischemic brain injury. PLoS ONE (2013) 8:e74405. doi: 10.1371/journal.pone.0074405
15. Vannucci RC, Connor JR, Mauger DT, Palmer C, Smith MB, Towfighi J, et al. Rat model of perinatal hypoxic-ischemic brain damage. J Neurosci Res. (1999) 55:158–63. doi: 10.1002/(SICI)1097-4547(19990115)55:2<158::AID-JNR3>3.0.CO;2-1
16. Yager JY. Animal models of hypoxic-ischemic brain damage in the newborn. Semin Pediatr Neurol. (2004) 11:31–46. doi: 10.1016/j.spen.2004.01.006
17. Ravikiran T, Sowbhagya R, Anupama SK, Anand S, Bhagyalakshmi D. Age-related changes in the brain antioxidant status: modulation by dietary supplementation of Decalepis hamiltonii and physical exercise. Mol Cell Biochem. (2016) 419:103–13. doi: 10.1007/s11010-016-2754-6
18. Asl NA, Sheikhzade F, Torchi M, Roshangar L, Khamnei S. Long-term regular exercise promotes memory and learning in young but not in older rats. Pathophysiology (2008) 15:9–12. doi: 10.1016/j.pathophys.2007.10.002
19. Fordyce DE, Farrar RP. Enhancement of spatial learning in F344 rats by physical activity and related learning-associated alterations in hippocampal and cortical cholinergic functioning. Behav Brain Res. (1991) 46:123–33.
20. van Praag H, Christie BR, Sejnowski TJ, Gage FH. Running enhances neurogenesis, learning, and long-term potentiation in mice. Proc Natl Acad Sci USA. (1999) 96:13427–31.
21. Nithianantharajah J, Hannan AJ. Enriched environments, experience-dependent plasticity and disorders of the nervous system. Nat Rev Neurosci. (2006) 7:697–709. doi: 10.1038/nrn1970
22. Attems J, Walker L, Jellinger KA. Olfactory bulb involvement in neurodegenerative diseases. Acta Neuropathol. (2014) 127:459–75. doi: 10.1007/s00401-014-1261-7
23. Patel RM, Pinto JM. Olfaction: anatomy, physiology, and disease. Clin Anat. (2014) 27:54–60. doi: 10.1002/ca.22338
24. Devi SA, Kiran TR. Regional responses in antioxidant system to exercise training and dietary vitamin E in aging rat brain. Neurobiol Aging (2004) 25:501–8. doi: 10.1016/S0197-4580(03)00112-X
25. Yokota S, Hori H, Umezawa M, Kubota N, Niki R, Yanagita S, et al. Gene expression changes in the olfactory bulb of mice induced by exposure to diesel exhaust are dependent on animal rearing environment. PLoS ONE (2013) 8:e70145. doi: 10.1371/journal.pone.0070145
26. Mandairon N, Didier A, Linster C. Odor enrichment increases interneurons responsiveness in spatially defined regions of the olfactory bulb correlated with perception. Neurobiol Learn Mem. (2008) 90:178–84. doi: 10.1016/j.nlm.2008.02.008
27. Hendriksen H, Meulendijks D, Douma TN, Bink DI, Breuer ME, Westphal KG, et al. Environmental enrichment has antidepressant-like action without improving learning and memory deficits in olfactory bulbectomized rats. Neuropharmacology (2012) 62:270–7. doi: 10.1016/j.neuropharm.2011.07.018
28. Kang JW, Lee NY, Cho KC, Lee MY, Choi DY, Park SH, et al. Analysis of nitrated proteins in Saccharomyces cerevisiae involved in mating signal transduction. Proteomics (2015) 15:580–90. doi: 10.1002/pmic.201400172
29. Mueller LN, Brusniak MY, Mani DR, Aebersold R. An assessment of software solutions for the analysis of mass spectrometry based quantitative proteomics data. J Proteome Res. (2008) 7:51–61. doi: 10.1021/pr700758r
30. Won YH, Lee MY, Choi YC, Ha Y, Kim H, Kim DY, et al. Elucidation of relevant neuroinflammation mechanisms using gene expression profiling in patients with amyotrophic lateral sclerosis. PLoS ONE (2016) 11:e0165290. doi: 10.1371/journal.pone.0165290
31. Livak KJ, Schmittgen TD. Analysis of relative gene expression data using real-time quantitative PCR and the 2(-Delta Delta C(T)) Method. Methods (2001) 25:402–8. doi: 10.1006/meth.2001.1262
32. Im SH, Yu JH, Park ES, Lee JE, Kim HO, Park KI, et al. Induction of striatal neurogenesis enhances functional recovery in an adult animal model of neonatal hypoxic-ischemic brain injury. Neuroscience (2010) 169:259–68. doi: 10.1016/j.neuroscience.2010.04.038
33. Tomita K, Freeman BL, Bronk SF, LeBrasseur NK, White TA, Hirsova P, et al. CXCL10-mediates macrophage, but not other innate immune cells-associated inflammation in murine nonalcoholic steatohepatitis. Sci Rep. (2016) 6:28786. doi: 10.1038/srep28786
34. Ha KN, Chen Y, Cai JY, Sternberg P. Increased glutathione synthesis through an ARE-Nrf2-dependent pathway by zinc in the RPE: implication for protection against oxidative stress. Invest Ophth Vis Sci. (2006) 47:2709–15. doi: 10.1167/iovs.05-1322
35. Talalay P, Dinkova-Kostova AT, Holtzclaw WD. Importance of phase 2 gene regulation in protection against electrophile and reactive oxygen toxicity and carcinogenesis. Adv Enzyme Regul. (2003) 43:121–34. doi: 10.1016/S0065-2571(02)00038-9
36. Deponte M. Glutathione catalysis and the reaction mechanisms of glutathione-dependent enzymes. Biochim Biophys Acta (2013) 1830:3217–66. doi: 10.1016/j.bbagen.2012.09.018
37. Naik E, Dixit VM. Mitochondrial reactive oxygen species drive proinflammatory cytokine production. J Exp Med. (2011) 208:417–20. doi: 10.1084/jem.20110367
38. Gage FH. Mammalian neural stem cells. Science (2000) 287:1433–8. doi: 10.1126/science.287.5457.1433
39. Yuan C, Li M, Zheng Y, Zhou Y, Wu F, Wang Z. Accumulation and detoxification dynamics of Chromium and antioxidant responses in juvenile rare minnow, Gobiocypris rarus. Aquat Toxicol. (2017) 190:174–80. doi: 10.1016/j.aquatox.2017.07.005
40. Alonso M, Lepousez G, Sebastien W, Bardy C, Gabellec MM, Torquet N, et al. Activation of adult-born neurons facilitates learning and memory. Nat Neurosci. (2012) 15:897–904. doi: 10.1038/nn.3108
41. Heydel J, Leclerc S, Bernard P, Pelczar H, Gradinaru D, Magdalou J, et al. Rat olfactory bulb and epithelium UDP-glucuronosyltransferase 2A1 (UGT2A1) expression: in situ mRNA localization and quantitative analysis. Brain Res Mol Brain Res. (2001) 90:83–92. doi: 10.1016/S0169-328X(01)00080-8
42. Heydel JM, Holsztynska EJ, Legendre A, Thiebaud N, Artur Y, Le Bon AM. UDP-glucuronosyltransferases (UGTs) in neuro-olfactory tissues: expression, regulation, and function. Drug Metab Rev. (2010) 42:74–97. doi: 10.3109/03602530903208363
43. Minn A, Leclerc S, Heydel JM, Minn AL, Denizcot C, Cattarelli M, et al. Drug transport into the mammalian brain: the nasal pathway and its specific metabolic barrier. J Drug Target (2002) 10:285–96. doi: 10.1080/713714452
44. Zorov DB, Juhaszova M, Sollott SJ. Mitochondrial reactive oxygen species (ROS) and ROS-induced ROS release. Physiol Rev. (2014) 94:909–50. doi: 10.1152/physrev.00026.2013
45. Valko M, Leibfritz D, Moncol J, Cronin MT, Mazur M, Telser J. Free radicals and antioxidants in normal physiological functions and human disease. Int J Biochem Cell Biol. (2007) 39:44–84. doi: 10.1016/j.biocel.2006.07.001
46. Zhang Q, Yan W, Bai Y, Zhu Y, Ma J. Repeated formaldehyde inhalation impaired olfactory function and changed SNAP25 proteins in olfactory bulb. Int J Occup Environ Health (2014) 20:308–12. doi: 10.1179/2049396714Y.0000000079
47. Lazarini F, Lledo PM. Is adult neurogenesis essential for olfaction? Trends Neurosci. (2011) 34:20–30. doi: 10.1016/j.tins.2010.09.006
48. Ming GL, Song H. Adult neurogenesis in the mammalian brain: significant answers and significant questions. Neuron (2011) 70:687–702. doi: 10.1016/j.neuron.2011.05.001
49. Bovetti S, Veyrac A, Peretto P, Fasolo A, De Marchis S. Olfactory enrichment influences adult neurogenesis modulating GAD67 and plasticity-related molecules expression in newborn cells of the olfactory bulb. PLoS ONE (2009) 4:e6359. doi: 10.1371/journal.pone.0006359
50. Bonzano S, Bovetti S, Fasolo A, Peretto P, De Marchis S. Odour enrichment increases adult-born dopaminergic neurons in the mouse olfactory bulb. Eur J Neurosci. (2014) 40:3450–7. doi: 10.1111/ejn.12724
51. Mastrorilli V, Scopa C, Saraulli D, Costanzi M, Scardigli R, Rouault JP, et al. Physical exercise rescues defective neural stem cells and neurogenesis in the adult subventricular zone of Btg1 knockout mice. Brain Struct Funct. (2017) 222:2855–76. doi: 10.1007/s00429-017-1376-4
52. Monteiro BM, Moreira FA, Massensini AR, Moraes MF, Pereira GS. Enriched environment increases neurogenesis and improves social memory persistence in socially isolated adult mice. Hippocampus (2014) 24:239–48. doi: 10.1002/hipo.22218
53. ernandez CI, Collazo J, Bauza Y, Castellanos MR, Lopez O. Environmental enrichment-behavior-oxidative stress interactions in the aged rat: issues for a therapeutic approach in human aging. Ann N Y Acad Sci. (2004) 1019:53–7. doi: 10.1196/annals.1297.012
54. Mohammed AH, Zhu SW, Darmopil S, Hjerling-Leffler J, Ernfors P, Winblad B, et al. Environmental enrichment and the brain. Prog Brain Res. (2002) 138:109–33. doi: 10.1016/S0079-6123(02)38074-9
55. Kolb B, Gorny G, Soderpalm AH, Robinson TE. Environmental complexity has different effects on the structure of neurons in the prefrontal cortex versus the parietal cortex or nucleus accumbens. Synapse (2003) 48:149–53. doi: 10.1002/syn.10196
56. Kempermann G, Gast D, Gage FH. Neuroplasticity in old age: sustained fivefold induction of hippocampal neurogenesis by long-term environmental enrichment. Ann Neurol. (2002) 52:135–43. doi: 10.1002/ana.10262
57. Mizutani K, Sonoda S, Karasawa N, Yamada K, Shimpo K, Chihara T, et al. Effects of exercise after focal cerebral cortex infarction on basal ganglion. Neurol Sci. (2013) 34:861–7. doi: 10.1007/s10072-012-1137-3
58. Gaskill BN, Pritchett-Corning KR. The effect of cage space on behavior and reproduction in Crl:CD1(Icr) and C57BL/6NCrl laboratory mice. PLos ONE (2015) 10:e0127875. doi: 10.1371/journal.pone.0127875
59. Sha JC, Ismail R, Marlena D, Lee JL. Environmental complexity and feeding enrichment can mitigate effects of space constraints in captive callitrichids. Lab Anim. (2016) 50:137–44. doi: 10.1177/0023677215589258
Keywords: environmental enrichment, olfactory bulb, metabolizing enzymes, antioxidant, detoxification, glutathione
Citation: Seo JH, Pyo S, Shin Y-K, Nam B-G, Kang JW, Kim KP, Lee HY and Cho S-R (2018) The Effect of Environmental Enrichment on Glutathione-Mediated Xenobiotic Metabolism and Antioxidation in Normal Adult Mice. Front. Neurol. 9:425. doi: 10.3389/fneur.2018.00425
Received: 30 November 2017; Accepted: 22 May 2018;
Published: 04 July 2018.
Edited by:
Firas H. Kobeissy, University of Florida, United StatesReviewed by:
Melissa Cavallin Johnson, University of West Georgia, United StatesCopyright © 2018 Seo, Pyo, Shin, Nam, Kang, Kim, Lee and Cho. This is an open-access article distributed under the terms of the Creative Commons Attribution License (CC BY). The use, distribution or reproduction in other forums is permitted, provided the original author(s) and the copyright owner(s) are credited and that the original publication in this journal is cited, in accordance with accepted academic practice. No use, distribution or reproduction is permitted which does not comply with these terms.
*Correspondence: Hoo Young Lee, cmFwaGFlbGxhcG1yQGdtYWlsLmNvbQ==
Sung-Rae Cho, c3JjaG85MThAeXVocy5hYw==
†These authors have contributed equally to this study.
Disclaimer: All claims expressed in this article are solely those of the authors and do not necessarily represent those of their affiliated organizations, or those of the publisher, the editors and the reviewers. Any product that may be evaluated in this article or claim that may be made by its manufacturer is not guaranteed or endorsed by the publisher.
Research integrity at Frontiers
Learn more about the work of our research integrity team to safeguard the quality of each article we publish.