- 1BRAI2N, Section of Neurosurgery, Department of Surgical Sciences, Dunedin School of Medicine, University of Otago, Dunedin, New Zealand
- 2School of Behavioral and Brain Sciences, University of Texas at Dallas, Richardson, TX, USA
- 3Department of Psychiatry and Psychotherapy, University of Regensburg, Regensburg, Germany
- 4Department of Neuroscience and Physiology, New York University School of Medicine, New York, NY, USA
Tinnitus is the perception of a sound in the absence of a corresponding external sound source. Pathophysiologically it has been attributed to bottom-up deafferentation and/or top-down noise-cancelling deficit. Both mechanisms are proposed to alter auditory thalamocortical signal transmission, resulting in thalamocortical dysrhythmia (TCD). In deafferentation, TCD is characterized by a slowing down of resting state alpha to theta activity associated with an increase in surrounding gamma activity, resulting in persisting cross-frequency coupling between theta and gamma activity. Theta burst-firing increases network synchrony and recruitment, a mechanism, which might enable long-range synchrony, which in turn could represent a means for finding the missing thalamocortical information and for gaining access to consciousness. Theta oscillations could function as a carrier wave to integrate the tinnitus-related focal auditory gamma activity in a consciousness enabling network, as envisioned by the global workspace model. This model suggests that focal activity in the brain does not reach consciousness, except if the focal activity becomes functionally coupled to a consciousness enabling network, aka the global workspace. In limited deafferentation, the missing information can be retrieved from the auditory cortical neighborhood, decreasing surround inhibition, resulting in TCD. When the deafferentation is too wide in bandwidth, it is hypothesized that the missing information is retrieved from theta-mediated parahippocampal auditory memory. This suggests that based on the amount of deafferentation TCD might change to parahippocampocortical persisting and thus pathological theta–gamma rhythm. From a Bayesian point of view, in which the brain is conceived as a prediction machine that updates its memory-based predictions through sensory updating, tinnitus is the result of a prediction error between the predicted and sensed auditory input. The decrease in sensory updating is reflected by decreased alpha activity and the prediction error results in theta–gamma and beta–gamma coupling. Thus, TCD can be considered as an adaptive mechanism to retrieve missing auditory input in tinnitus.
Introduction
Non-pulsatile tinnitus is the perception of a sound in the absence of a corresponding external sound source. As an auditory phantom phenomenon, it has been conceptualized to be either linked to bottom-up deafferentation (see Figure 1, green box) with or without behaviorally measurable hearing loss, or a deficient top-down noise-cancelling mechanism (see Figure 1, blue box) or a combination of both.
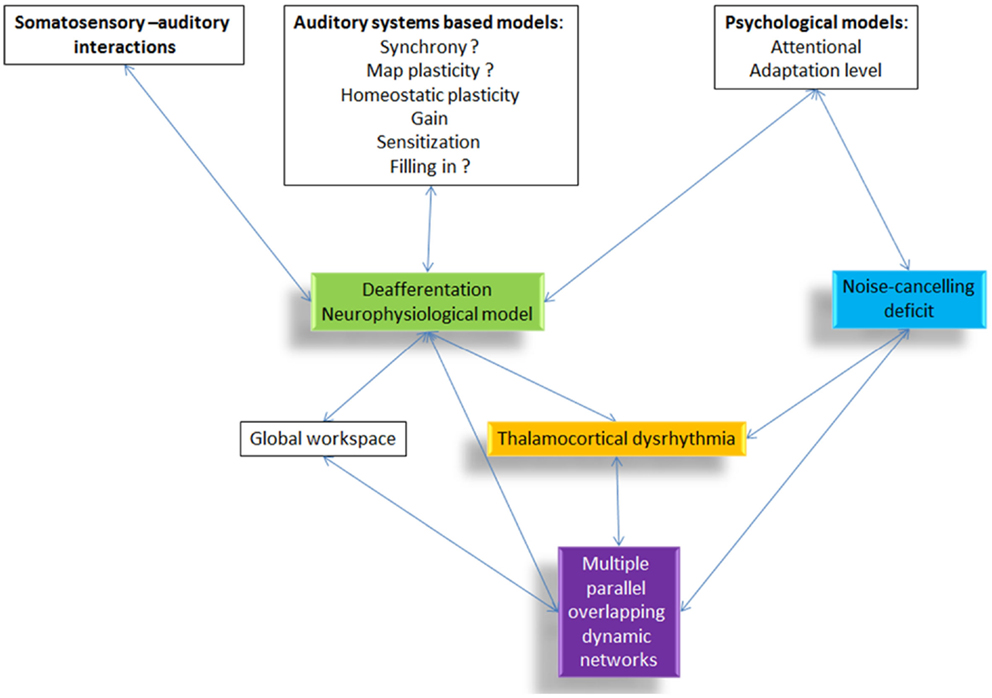
Figure 1. Overview of the different tinnitus models and how they are related to each other. All proposed pathophysiological models include or relate to thalamocortical dysrhythmia.
Based on phenomenological similarities it has been proposed that tinnitus is the auditory analog of phantom pain (1–4) with auditory deafferentation representing its causative factor. Many subsequent models are refinements of this concept and limit themselves to changes in the auditory system, zooming in on the specific aspects linked to auditory deprivation, such as hyperactivity (5), an increase in gain (6), plasticity (7), including map plasticity (8) and homeostatic plasticity (9–11), synchrony (5, 12), filling the missing information (3, 13, 14), or sensitization (15). Many of these models are still a matter of debate, such as the involvement of synchrony (16), or whether changes in gain explain really tinnitus and not only hyperacusis. Also, the memory-based filling in mechanism is still a rather speculative model with limited experimental evidence. Moreover, some models focus on somatosensory compensatory mechanisms in the dorsal cochlear nucleus (17), which in itself has been proposed to be a core region in the development of tinnitus (18).
A second complementary concept proposes that deafferentiation-induced alterations in the central auditory pathways are not sufficient for the conscious perception of tinnitus. Tinnitus only arises, if auditory deafferentiation is accompanied by a deficient inhibitory top-down mechanism (19, 20), analogous to the anti-nociceptive system deficit linked to some forms of spontaneous pain such as fibromyalgia (21).
Recently, the concept has been forwarded that tinnitus might be the expression of a global workspace hyperactivity rather than being limited to the auditory system (22), which has been extended in a heuristic model integrating the deafferentiation model, the noise-cancelling deficit, and the global workspace model. The global workspace model was first proposed by Baars (23) as a model for conscious cognitive processing. It is a model for the interface between multiple unconscious, parallel processing modules on one side, and conscious experience on the other side, proposing how different sources of information are integrated into one percept with internally consistent content. The global workspace model suggests that multiple, highly specialized; quasi-independent input processors compete for access to a broadcasting capability by which the winning processor can disseminate its information globally throughout the brain. Based on the global workspace concept, Dehaene and colleagues (24) have proposed a neuronal implementation of a global workspace architecture, the so-called “neuronal global workspace.” In this model, sensory stimuli mobilize excitatory neurons with long-range cortico-cortical axons, leading to the genesis of a global activity pattern among workspace neurons, which are widely distributed throughout the brain. Any such global pattern can inhibit alternative activity patterns among workspace neurons, thus preventing the conscious processing of alternative stimuli and enabling a uniform conscious percept. Moreover, top-down attentional amplification is the mechanism by which modular processes can be temporarily mobilized and made available to the global workspace, and therefore to consciousness. This heuristic model is based on recent studies in consciousness research (25–27) and the philosophical concept of emergence in complex adaptive systems (3, 28–30). The emergence of a unified percept relies on the coordination of scattered mosaics of functionally specialized brain regions (31). This is proposed to be related to oscillatory activity and connectivity within the brain (32). Large-scale integration has been proposed to bind the distributed anatomical and functional organization of brain activity enabling the emergence of coherent behavior and cognition. Although the mechanisms involved in large-scale integration are still largely unknown, it has been convincingly argued that the most plausible candidate is the formation of dynamic links mediated by synchrony over multiple frequency bands (31). Even though within-frequency phase synchronization may support the binding of anatomically distributed processing, it cannot coordinate neuronal processing distributed into distinct time windows or frequency bands (33). Thus, cross-frequency coupling might be important for large-scale integration via low-frequency coherence of distributed geographically focal high-frequency activity (34). This could be carried out by nested oscillations (phase–amplitude interactions) or by phase–phase interactions, such as n:m phase synchrony, at discrete frequencies (33), as shown in both invasive human recordings (35) and with MEG and EEG recordings related to sensory awareness (36). This notion is supported by very recent results demonstrating tinnitus-related cross-frequency-coupling (37). Multiple forms of cross-frequency coupling exist. The most commonly studied forms are phase–amplitude and phase–phase cross-frequency coupling, but amplitude–amplitude, phase–frequency cross-frequency coupling exist (38). In tinnitus, intra-area cross-frequency coupling is known as thalamocortical dysrhythmia (TCD), and inter-area phase–amplitude coupling between anterior cingulate and auditory cortex and dorsolateral prefrontal cortex has been shown as well (37). The other cross-frequency couplings have not been investigated yet.
What all models, the deafferentation neurophysiological model, the noise-cancelling deficit, and the multiple parallel overlapping dynamical network model have in common, is that they rely on a final common pathway called TCD (39) (see Figure 1, yellow box). However, the question arises, how to theoretically integrate TCD in the setting of consciousness as an emergent property of network activity (31, 40) and more specifically in tinnitus (41).
Tinnitus is perceived as a unified percept, incorporating a sound, which can be different in quality, location, loudness, constancy, duration, as well as affective components such as mood and distress. It is assumed that each of these characteristics is related to a separate subnetwork, but linked by hubs that are part of multiple subnetworks (41). Furthermore, there are common associated symptoms in tinnitus, such as hearing loss, which can also generate distress (42, 43), and as distress is generated by a non-specific brain network (3, 44–49) this can be perceived or confounded as tinnitus distress. The lateralization of the tinnitus percept depends on gamma-band activity in the parahippocampal area, associated with functional connectivity between the parahippocampal area and auditory cortex.
Tinnitus has been likened to pain, both pathophysiologically, clinically, and treatment-wise (1–3, 39, 50–52). However, whereas different subtypes of pain have been clearly discerned, e.g., nociceptive versus neuropathic pain, this has not been as obvious for tinnitus. It is likely that different forms or subtypes of tinnitus exist, each with a different pathophysiology, which could explain the interindividual differences seen in recent electrophysiological studies (53, 54).
Gamma-Band Activity and Consciousness
Gamma-band activity (>30 Hz) is important for motor control (55) and processing of sensory stimuli, whether in the olfactory bulb (56), visual (57), auditory (58), or somatosensory cortex (59). Synchronization of separate gamma-band activities (>30), present in different thalamocortical columns (60), is proposed to bind (57, 61) distributed neural gamma activity into one coherent auditory percept (58, 62–66). Sound intensity is also reflected by the amount of gamma-band activity (67). This could suggest that gamma reflects a difference, a prediction error (68), a detection of change to the reference (69), or status quo, the latter, which would be reflected by beta activity (70). In general, gamma-band activity is present only in locally restricted areas of the cortex for short periods of time (34, 35, 71, 72). Whereas normally gamma activity waxes and wanes, related to the presence of a novel external stimulus, persisting gamma activity localized in one brain area has been considered pathological (39) (see Figure 2). However, in contrast to the waxing and waning of gamma-band activity, recent studies related to language processing do demonstrate there are also spontaneous endogenous longer lasting gamma rhythms present at rest in monkeys (73) and humans (74). These are likely generated by inhibitory interneurons (75) or interactions between pyramidal cells and interneurons (76, 77) and might reflect attentional processes (76, 77). These complement the exogenous stimulus driven gamma-band activity, which usually increases gamma-band activity in sensory areas, presumably clustering spiking activity that is propagated to higher hierarchic processing stages (74). Thus, it has been envisaged that these spontaneous gamma oscillations mechanistically might optimize the extraction of relevant sensory input in time (74). This intriguiging finding could potentially be applied to tinnitus. It has been proposed that oscillations correspond to the alternation of phases of high- and low-neuronal excitability, which temporally constrain sensory processing (78). This means that gamma oscillations, which have a period of approximately 25 ms, provide a 10- to 15-ms window for integrating spectrotemporal information followed by a 10- to 15-ms window for propagating the output (74). However, because the average length of a phoneme is about 50 ms, a 10- to 15-ms window might be too short for integrating this information, but a phoneme can correctly be represented by three gamma cycles, which act as a three-bit code (74). Further studies should evaluate whether tinnitus could be represented by such a 3-bit gamma code. In the visual system, stimuli that reach consciousness and those which do not reach consciousness are characterized by a similar increase of local gamma oscillations in the visual cortex (79, 80). Thus, gamma-band activity, per se, is not related to conscious perception, but could be a condition sine qua non, an essential prerequisite, for conscious perception (30). This is further confirmed by animal studies. Animals lacking gamma-band thalamocortical activation, due to the absence of P/Q-type Ca++ channels [CaV2.1-null (i.e., _-1A KO)], lacks a cognitive response to sensory stimulation (81). Only when focal gamma activity is embedded in a larger network of long-range functional connectivity, this will lead to the emergence of a conscious percept (41) (see Figure 2).
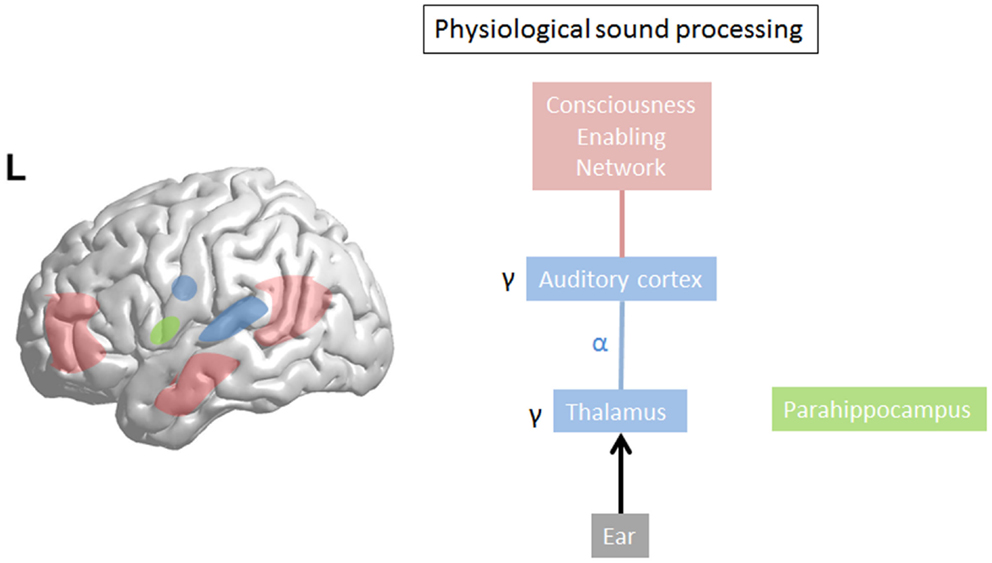
Figure 2. In physiological sound processing, sound triggers gamma-band activity in the auditory cortex. The externally presented sound only reaches consciousness if consciousness enabling network (=global workspace) is co-activated with sound perception. The last sound presentation is stored in the (para)hippocampal area as a reference for future input to calculate the prediction error.
Thalamocortical Dysrhythmia and Tinnitus
Based on the principle that gamma-band activity is associated with the conscious percept of auditory stimuli (58, 66, 82), Llinas proposed that tinnitus, as a conscious percept, should be associated with persistent gamma-band activity in the auditory cortex (39) confirmed in latter Ca V2.1-null experiments (81). Indeed, it has been shown both by EEG (83), MEG (39, 84, 85), and intracranial recordings (46) that tinnitus is related to gamma-band activity in the auditory cortex and nested on theta activity (39, 46, 84). Whereas, initially, it was thought that the gamma-band activity was contralateral (83, 84), it has been shown that gamma-band activity is increased in both auditory cortices, even in unilateral tinnitus (86). The amount of gamma-band activity has been shown to be related to the subjectively perceived tinnitus loudness (83). A decrease in tinnitus loudness has been found to be associated with a decrease in gamma-band activity in the auditory cortex (87–89) and a worsening in tinnitus loudness with an increase in auditory cortex gamma-band activity (90), even if such a relationship has been called into question by a recent study (54). In this paper (54), gamma-band activity increases and decreases were noted associated with an increase in tinnitus loudness after residual inhibition or a decrease in loudness after residual excitation, respectively. This could reflect that gamma represents a homeostatic change in loudness per se, on the condition that the tinnitus state has become the reference, or it could potentially be attributed to other factors, such as associated hearing loss in these patients.
An explanation for the occurrence of this theta–gamma coupled activity in sensory deafferentation is provided by the concept of TCD (39, 85). This model states that, in the deafferented state, the dominant resting state alpha rhythm (8–12 Hz) decreases to theta (4–7 Hz) (39) band activity. Conceptually, this can be explained that the firing rate decreases when less information needs to be processed (91) due to the deafferentation, and that firing and oscillation rate are coupled at the thalamocortical level (85). As a result, GABAA-mediated lateral inhibition is reduced (85), inducing gamma (>30 Hz) band activity (39) surrounding the deafferented theta area, also known as the edge effect (85). Indeed, in tinnitus, a decrease in alpha power is associated with an increase in gamma power (92), and gamma goes together with theta activity (84), confirming the initial studies by Llinas et al. (39, 85). Other studies have since demonstrated the presence of both low- (delta or theta) and high-frequency activity in the auditory cortex of tinnitus patients (88, 89, 93, 94), and the coupled activity has also been confirmed on electrode recordings of implanted patients (46).
It was proposed that theta reflects the negative symptoms (hearing loss, hypoesthesia, …) and gamma the positive symptoms (tinnitus, pain, …) in diseases characterized by TCD (85, 95). Thus, the negative symptoms (e.g., hearing loss, somatosensory deprivation in amputation, …) are proposed to be linked to less information processing and therefore slowed alpha activity, as if the deafferented thalamocortical columns are “as asleep” (85). It has been proposed that this theta could then act as a long-range carrier wave (96–98) on which the tinnitus information can be nested by means of high-frequency oscillatory activity (41). This notion has been confirmed by a MEG study, which demonstrated increased coupled gamma–theta wave activity in tinnitus patients (39, 84, 85) and recently demonstrated in EEG as well (99).
How is this TCD generated at a cellular level and what mechanism can link TCD to a more widespread phenomenon in the brain?
T-Type Ca Channels, Theta Activity, Synchrony, and Network Recruitment in Thalamocortical Dysrhythmia
It has been proposed that the tectal system, with its rapid multisensory input, organizes the instantaneous orienting responses crucial to the maintenance of life (95). By contrast, the thalamocortical system, with its extensive corticothalamic recurrence and temporal binding properties, creates the complex combinatorial sensorimotor activities required for intentional behavior, characteristic of vertebrate behavior (95). Thalamic neurons, on being depolarized from resting potential levels positive to −55 mV, fire tonically. Close to the threshold level, gamma dendritic subthreshold oscillations occur supported by P/Q-type Ca channels (81). At hyperpolarized levels negative to −60 mV, de-inactivation of a Ca2+ conductance give rise to an inward current through T-type Ca channels, resulting in low-threshold burst firing. This remains for as long as the cells remain hyperpolarized, i.e., deafferented. Thus, in short, thalamic neurons have the unique property to fire both at depolarization and at hyperpolarization.
T-type calcium channels are known as low-voltage-activated (LVA) channels as they first open at more hyperpolarized membrane potentials compared to high-voltage-activated calcium channels, such as the L-, P/Q-, N-, and R-type channels (100). Thus, T-type calcium channels can be considered first-responders to small depolarization (100), generating a low-threshold Ca2+ potential upon the crest of which sodium and potassium channel-mediated action potentials fire, creating low-threshold bursts (101). Overall, the entire burst process mediated via T-type Ca channels takes approximately 100–200 ms limiting the frequency at which bursting can occur to around 5–10 Hz (102), i.e., theta frequencies. When a burst is generated in a thalamocortical neuron anywhere on the dendrite or cell body, the low-threshold Ca2+ potential is conducted not only to the soma but also back-propagates throughout the entire dendritic tree (100), generating bursts in multiple postsynaptic neurons in the projected nucleus through axonal arborization to multiple synapses. These bursting neurons will in turn recruit additional neurons in the reciprocally connected initiating nucleus and this burst amplification will progress on each cycle of the oscillation, generating synchronization in a network of reciprocally connected CNS nuclei (103, 104). This is in agreement with clinical data demonstrating that the dysrhythmia can be recorded with MEG over widespread areas (39). But the burst-firing process may also act as a neuronal frequency filter, preventing the conduction of recent incoming postsynaptic activity to the soma, and thereby preventing other activities contributing to the neuronal output (99).
In summary, in the non-bursting regions subthreshold oscillations are capable of performing a filtering task without increasing neuronal output. Contrastingly, bursting regions may spread network oscillations and recruit interconnected cortical areas, while simultaneously acting as a theta frequency filter (100). As such theta burst-firing increases network synchrony and recruitment (100), a mechanism, which might enable long-range synchrony, which in turn could represent a means for finding the missing thalamocortical information (due to deafferentation) (105). Hypothetically, the missing information may be replaced from (para)hippocampal memory. The synchrony would permit the (para)hippocampal information to be boosted to conscious perception by recruiting consciousness enabling network areas (41).
Thalamocortical Dysrhythmia Versus Physiological Cross-Frequency Coupling
Gamma-band activity in the auditory cortex is a prerequisite for auditory conscious perception and, therefore, likely also contributes to the perception of a phantom sound (Table 1). As theta–gamma coupling also exists in physiological auditory processing (34, 35, 106), the TCD state can be considered as a pathological persistence of normally waxing and waning theta–gamma-band coupled activity in specific topographic thalamocortical columns, resulting from auditory deafferentation (39). It can be conceived that the theta activity is the carrier wave connecting widespread areas (72), and that focal gamma-band activity in geographically separated brain areas is synchronized by nesting on the theta phase, as exemplified in auditory attention (106).
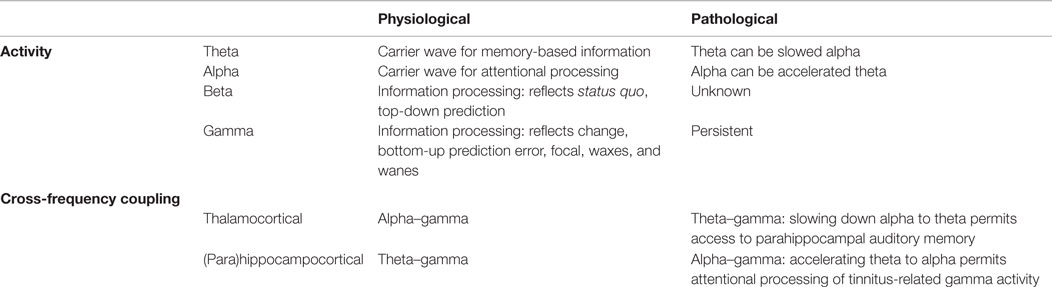
Table 1. Summary of physiological and pathological oscillatory activity and cross-frequency coupling.
It has been suggested that in physiological circumstances alpha–gamma coupling may be related to thalamocortical circuits (107, 108), whereas theta–gamma coupling has been associated with fronto–hippocampal networks (34, 108). This suggests that the theta activity in TCD is actually slowed alpha, as originally proposed (39), since it is found in thalamocortical loops. We could further speculate that alpha (lagged) phase synchronization between parahippocampal- and subgenual cortex in tinnitus distress (47, 109) may in turn represent accelerated theta activity. Indeed, heart rate variability is controlled by theta activity in the subgenual anterior cingulate cortex (110), but in distress, alpha activity is noted in this same area (44, 47, 111, 112). As distress is linked to autonomic system changes (110, 113), this suggests that the alpha in distress could actually be high theta. Thus, the difference between physiological and pathological theta–gamma coupling might not only be the transient versus persistent activity but also the generator, i.e., thalamus versus (para)hippocampus.
From its inception, it was also proposed that TCD is not restricted to the auditory system, but involves both the medial and lateral thalamic system (39). The additional involvement of the context-processing, non-specific medial thalamic nuclei, which connect to the cingulate, medial prefrontal, and orbitofrontal cortices, would provide an explanation for the affective component changes (39) present in about 20% of all people with tinnitus (114). The insula is involved in tinnitus. The anterior insula and dorsal anterior cingulate are part of a salience network, which is activated in chronic tinnitus (86, 115–117). This could reflect a paradoxical salience attributed to the tinnitus sound (3, 46–48), a reason why it remains present at a conscious level. Indeed, the right insula has been linked not only to conscious processing of cognition and emotion (118, 119) but also to the perception of tinnitus distress, reflected by sympathetic hyperactivity (113). The insula is hypothesized to integrate interoceptive and exteroceptive salient information (120), generating a feeling (118) of risk (121) and meaning (122) to the sound. The tinnitus seems to be processed by the brain as exteroceptive information as remembering a sound deactivates the insula and anterior cingulate cortex (123). Even though this concept is plausible, experimental support has only been found in the insula in distressing tinnitus (113). Most oscillatory changes in this non-specific distress network are found to occur in the alpha-frequency domain (44, 47, 111), and not in theta- or gamma-band activity. But as speculated before, this could actually reflect another form of dysrhythmia, in which theta is increased to alpha, and linked to the septal nuclei, as generator of hippocampal theta, rather than the thalamus. Indeed, septal nuclei burst at theta frequencies and fire tonically at 10–15 Hz when depolarized (124).
Thalamocortical Dysrhythmia and Error Prediction in Tinnitus
It has been proposed that tinnitus is an emergent property of network activity that fills in the deafferented thalamocortical activity in order to reduce auditory uncertainty (105). This goes back to the concept of the brain as a “prediction machine” as a fundamental characteristic of engagement with the world (125). The predictive brain requires an internal representation of the world (125), against which sensory stimuli are compared, and in which they are integrated. It incorporates the idea that a crucial function of the brain is to use stored information to imagine, simulate, and predict possible future events (126). The brain aims to minimize its prediction errors about its environment according to the free-energy principle (127). The Bayesian brain model adds to the predictive brain model that the predictions the brain makes are updated via active exploration of the environment through the senses (128). From a Bayesian statistical point of view, the brain is conceived as an inference machine that actively predicts and explains its sensations (127). Its central postulate is that percepts represent predictions or hypotheses about the external world whose degree of belief (the posterior probability) is determined by a combination of sensory evidence obtained by actively sampling the environment and background assumptions, based on a model of the world stored in contextual memory, about a priori plausible world structures (the prior) (14, 128). Subsequently, the posterior belief (=percept) becomes the next prior belief (=prediction) for a new sequence of prediction, resulting in a Markov chain Monte Carlo inference, i.e., a constantly updated hypothesis of the world. This requires some form of intentionality (129) related to self-preservation. Auditory deafferentation in a given frequency range induces a tonotopically specific prediction error. The actual auditory input is inconsistent with auditory input before the hearing loss occurred (3). In other words, the actual input is inconsistent with what is stored in memory. This inconsistency in turn signals that an information update is required (41). The oscillatory activity related to auditory predictions has been recently identified (130): delta–beta coupled oscillations underpin prediction accuracy (130), and (Bayesian) updating of the predictions is processed by the alpha-band (10–14 Hz) (130). Predicting “when” an auditory stimulus arrives predominantly involves low-frequency delta and theta oscillations, predicting “what” is processed by gamma and beta oscillations (131). Beta oscillations likely underlie a top-down flow of information, whereas gamma oscillations could be generated bottom-up (131). Whereas predictions are transmitted in a top-down “backward” manner, using mainly the beta band, prediction errors could be propagated in the gamma band in a bottom-up feed-forward manner (131). Updating of the predictions, via attention-based scanning of the environment (132), on the other hand, is linked to alpha oscillations (130, 132). Thus, transferring these findings to tinnitus, on could speculate that increased gamma activity in tinnitus would be related to a deafferentation-related (thalamocortical column specific spatial mismatch) prediction error, and the nesting on theta or delta related to its temporal prediction. In other words, gamma activity could reflect any change in the auditory environment, as this induces a prediction error. In TCD gamma activity would reflect a persistent deafferentation-based thalamocortical column specific prediction error.
One could interpret the decrease of alpha in TCD as a decrease in environmental scanning, resulting in a persistent prediction error, i.e., a persistent tinnitus percept. The slowed down alpha, resulting in theta bursting can then recruit other areas that also burst in theta such as the septal nuclei driven hippocampal–parahippocampal memory network. Thus, by decreasing its firing and oscillation rate to theta the TCD permits to access memory to fill in the missing auditory information.
In summary, the Bayesian model for tinnitus gives an alternative but complementary explanation for the oscillatory changes seen in tinnitus by adding that also other cross-frequency couplings need to be considered in the pathophysiology of tinnitus.
Thalamocortical Dysrhythmia and Deficient Inhibitory Top-Down Mechanisms
Tinnitus was proposed to be a bottom-up generated TCD, linked to thalamocortical deafferentation (39). However, from the beginning of this model, it was conceived that top-down mechanisms may also contribute to the development of TCD. This would be more related to neuropsychiatric or affective behavioral changes (133). In a normally functioning noise-cancelling mechanism alpha activity, which is the normal resting state activity in the ventral medial prefrontal cortex–pregenual anterior cingulate cortex should predominate. In a dysfunctional noise-cancelling mechanism, it is expected that delta/theta and beta would predominate. Indeed, in a study looking at selective enriched acoustic stimulation, in which overcompensation of the deafferented stimuli was used, clinical worsening of patients was related to increased beta activity in the pregenual anterior cingulate cortex, linked to increased gamma in the auditory cortex (90). This is in agreement with a study that shows that tinnitus distress is related to high beta (25 Hz) in frontal areas (44, 134). By contrast, in a study using bifrontal tDCS, improvement of tinnitus loudness and distress was associated with a decrease in gamma-band activity in the auditory cortex, mediated via increased alpha activity in the pregenual anterior cingulate cortex (87). A recent EEG study demonstrated a correlation between delta, theta, and beta1 activity in the pregenual to rostral ACC and the percentage of the time that tinnitus is perceived (135). The percentage of the time tinnitus is perceived seems, furthermore, to be inversely related to the functional connectivity between this area and the left primary auditory cortex (135). Even though these studies provide certain support for the involvement of the pregenual anterior cingulate cortex in changing auditory cortex gamma-band activity linked to tinnitus, these findings have still to be considered as preliminary and the exact mechanism how the pregenual anterior cingulated interacts with the auditory system is not yet revealed.
Thalamocortical Dysrhythmia in Tinnitus Can be Modulated
In a study evaluating electrophysiological changes associated with an improvement in tinnitus perception by means of auditory coordinated reset stimulation (136), pretreatment pathological delta and gamma-band activity was normalized only in those patients who had a benefit from the treatment (89), demonstrating that TCD is possibly causally related to the tinnitus.
MEG measurements before and after repetitive transcranial magnetic stimulation (rTMS) revealed that subjective reduction of tinnitus loudness after rTMS was related to a reduction of gamma and an increase in alpha activity (137).
Neurofeedback is a promising neuromodulation technique, based on operant conditioning, which can modulate TCD. It has been shown that tinnitus is related to a loss of alpha power, which is associated with an increase in gamma power (92), and that gamma is coupled to theta (84), the signature of TCD. Using neurofeedback in tinnitus, the alpha power can be increased in the auditory cortex (138), returning to a normal rhythmic alpha resting state.
Surgical neuromodulation treatments such as thalamic lesioning (52, 139) and auditory cortex stimulation via implanted electrodes (46, 93) have shown to reduce tinnitus in selected patients and to alter electrophysiological signatures of tinnitus-related TCD (46, 93, 139). In a tinnitus patient who was implanted with electrodes overlying the auditory cortex for tinnitus treatment, recordings from the implanted electrode revealed increased gamma and theta activity only at one single pole, whereas at the other electrode poles normal alpha activity was recorded, demonstrating a highly spatially specific TCD. Furthermore, autocorrelations demonstrated that the theta and gamma activity was coupled, giving further support to the concept of TCD. The pole with increased gamma and theta activity exactly co-localized with the hotspot of maximal blood oxygen level-dependent activation during presentation of the tinnitus tone in the fMRI scanner. Moreover, maximal tinnitus suppression was obtained when current was delivery exactly at that cortical area. Tinnitus suppression in turn was accompanied by normalization of theta and gamma spectral changes, as well as the disappearance of the coupled theta–gamma activity both on electrode and source-localized electroencephalography recordings (46). These TCD-related changes were not seen in the areas at a distance of the BOLD activation, and stimulation at the normal sites did not clinically benefit the patient.
These findings, which are still limited to a small number of cases, provide further support for a causal relationship between TCD and tinnitus.
The question arises why tinnitus is so stable, so rigid, and difficult to reverse. Even though no proven answer can be given a heuristic concept can be forwarded. For pain, it has been suggested that it should be conceived of as a homeostatic emotion (118), and tinnitus could be considered as a homeostatic emotion as well, a balance between mechanisms that increase sound processing and mechanisms that decrease sound processing (41). From a Bayesian predictive point of view, an allostatic mechanism may induce a reference resetting in chronic tinnitus, such that the tinnitus state becomes the norm instead of the silent state (48), analogous to what is described in addiction (140). There are currently no studies performed looking at oscillatory signatures for allostasis, but it can be theoretically expected that allostasis is mediated by altered high-frequency oscilliations in the pregenual anterior cingulate and dorsal ACC, resetting the balance between noise-cancelling mechanisms and areas that process loudness (99). Once the auditory homeostasis is fluctuating around a tinnitus state as reference or norm, it becomes more difficult to treat. This is confirmed in clinical data, where tinnitus becomes more difficult to treat by brain stimulation techniques such as TMS (141–143) or operative techniques (144–146).
Is Thalamocortical Dysrhythmia Present in all Tinnitus Patients?
Based on its theoretical underpinnings TCD could be absent in tinnitus patients without deafferentation. However, it has to be considered that deafferentation does not equal behaviorally measurable hearing loss (147). Indeed, one can cut part of the auditory nerve without auditory threshold changes (148). Based on the Bayesian brain model, it has been theoretically proposed that depending on the bandwidth of deafferentation different compensatory mechanisms are utilized to obtain the missing auditory information, as to reduce auditory uncertainty related to the deprivation of auditory input (105).
Limited damage to auditory receptors results in loss of functional surround inhibition in the cortex, causing unmasking of latent inputs and significantly altering neural coding. However, these changes do not lead to plasticity of the cortical map (149), as the missing information can be obtained via access of overlapping tuning curves of the neighboring cortical cells. This is in accordance with clinical data demonstrating that in patients without hearing loss, no map plasticity is noted on fMRI (150). This decreased surround inhibition is also seen in TCD, in which GABAa mediated decrease in surround inhibition is noted, surrounding the thalamocortical columns that oscillate in theta (85) (Figure 3). If the deafferentation covers a broader frequency range, the brain reacts with widening of auditory receptive fields (151), which permits to pull the missing information from the auditory cortical neighborhood. If this is not possible, due to a more extended deafferentation, dendritic and axonal rewiring can occur (152), still retrieving the missing input from the auditory cortex. If the deafferentation is too extensive for compensatory auditory cortical plasticity to fill in the missing information, it is proposed that the missing data are retrieved from auditory memory via a parahippocampal mechanism (13, 105) (Figure 4). From a clinical point of view, this suggests that tinnitus associated with mild deafferentation (<20 dB HL) should theoretically be treated by targeting the auditory cortex, whereas tinnitus with hearing loss (>20 dB) might benefit more from targeting the parahippocampal area. It needs to be mentioned that the exact cut-offs for the different compensation mechanisms (decrease in surround inhibition, widening of receptive fields, sprouting, memory retrieval) have not been investigated yet. This could also explain that when oscillatory brain activity is compared between responders and non-responders to implanted electrodes overlying the auditory cortex, the difference is in the parahippocampal area (beta3 and gamma), not in the auditory cortex, and that only those patients respond who have good functional connectivity between the auditory cortex (where the stimulating electrode is) and the parahippocampus (153). Clinically, the responders are also characterized by an exact match between the tinnitus frequency spectrum and the frequency of hearing loss, suggesting that their tinnitus is primarily caused by deafferentation (153). This suggests that in tinnitus with severe hearing loss the dysrhythmia could be centered on the parahippocampus rather than auditory cortex and thalamus. The parahippocampus’ involvement in tinnitus has been shown, both in rsfMRI (154, 155), EEG (44, 45, 47, 86, 87, 110, 111, 115, 116, 153, 156–160), SPECT (161), and PET (162, 163) studies. Parahippocampal functional interactions with auditory cortex, i.e., functional connectivity has been demonstrated both with resting state EEG (117, 153), MEG (164), and resting state fMRI (154, 155). In the parahippocampal area, 35% of cells respond to complex auditory stimuli (165) and 2% specifically and selectively to auditory stimuli (165). This area has been called the gatekeeper to the hippocampus (166), functioning as a sensory gate for incoming irrelevant or redundant auditory input (167). In other words, the posterior parahippocampal area can be considered as the main node of entry for auditory information to the medial temporal lobe memory system, where salient information is encoded into long-term memory (168). As the parahippocampal area has been hypothesized to play a central role in memory recollection, sending information from the hippocampus to the association areas, a dysfunction in this mechanism is posited as an explanation for complex auditory phantom percepts such as auditory hallucinations (169). It might therefore also be involved in the generation of simple auditory phantom percepts such as tinnitus (3). This could also explain why not in all patients with tinnitus increased auditory gamma-band activity can be detected (54) and why some studies do not find a correlation between the presence of tinnitus and gamma-band activity (94).
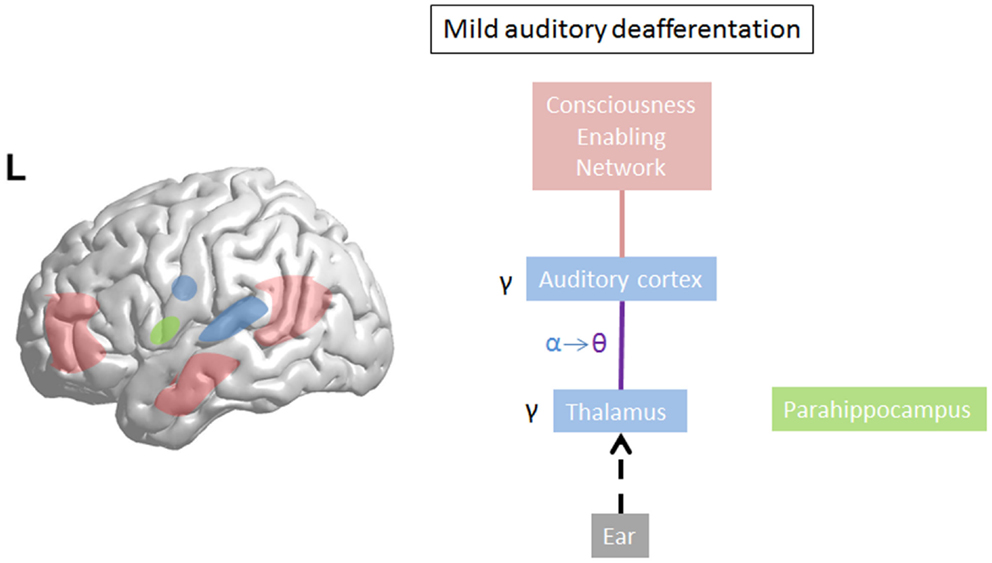
Figure 3. On limited deafferentation thalamocortical resting state alpha activity slows down to theta activity resulting in a decreased surround inhibition, so that the missing auditory information can be retrieved from the cortical neighborhood (purple line). The tinnitus sound representation is encoded by gamma. The theta–gamma coupled activity is called thalamocortical dysrhythmia.
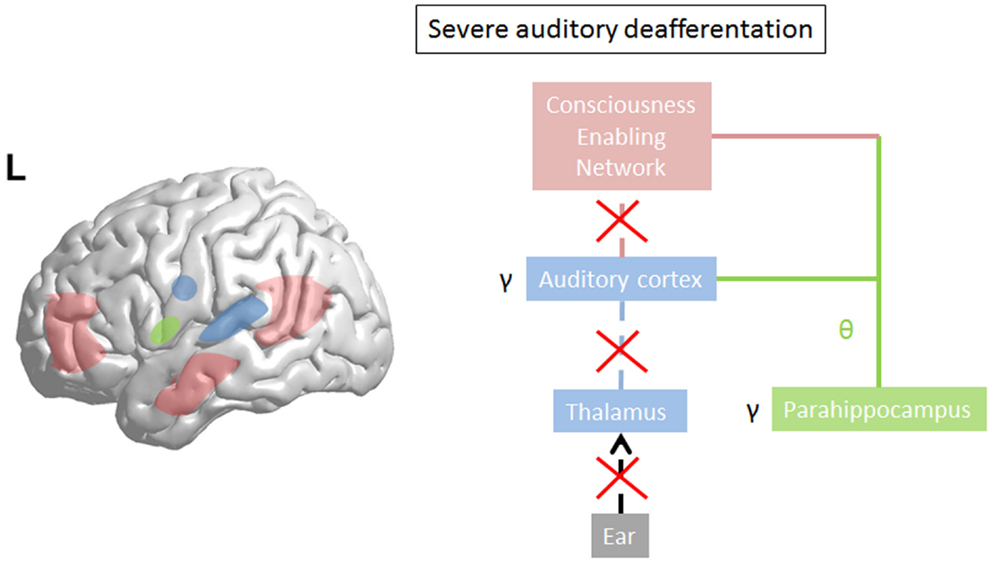
Figure 4. In severe deafferentation, the auditory cortex cannot retrieve the missing auditory input from the (auditory) cortical neighborhood. The thalamocortical bursting theta activity in the auditory cortex recruits the missing auditory input from the most recent update from parahippocampal-mediated memory processing (green line). There is no more thalamocortical theta–gamma dysrhythmia but a physiological memory related parahippocampocortical theta–gamma rhythm. The parahippocampus becomes linked to the consciousness enabling network (red line). Gamma decreases in the auditory cortex (arrow) as the prediction error disappears in comparison to what the parahippocampus predicts.
Conclusion
Thalamocortical dysrhythmia is the consequence of hyperpolarization of the thalamus, i.e., by a disconnected thalamus, due to deafferentation. The deafferentation results in alpha activity to slow down to theta activity, i.e., in decreased information input from externally, but permits to obtain the missing information from the auditory cortex neighborhood due to decrease in surround inhibition. Mechanistically, this is mediated by de-inactivation of T-type Ca2+ channels, and the generation of low-threshold bursting, normally only present during sleep. However, in case of severe deafferentation, when the missing information cannot be found in the auditory cortex neighborhood, the bursting theta activity acts as a carrier wave to access (=synchronize) (para)hippocampal theta activity-related memory processes. Persisting deafferentation could result in attentional hyperactivity and increased salience (looking for missing input), possibly resulting in accelerating theta to alpha activity, associated with tinnitus distress. Thus, TCD can be considered as an adaptive mechanism to retrieve missing auditory input and gaining access to consciousness enabling networks in tinnitus. This is in accordance with the Bayesian brain concept that states that tinnitus is an attempt to decrease auditory deprivation related uncertainty. TCD might not be present in patients without deafferentation, as in this subgroup a noise-cancelling mechanism might underly the generation of tinnitus. The exact mechanism of how the noise-cancelling mechanism modulates thalamocortical activity is unknown.
Thalamocortical dysrhythmic cross-frequency coupled activity per se is not sufficient for conscious awareness of tinnitus, and neither is parahippocampocortical cross-frequency coupled activity. For it to reach consciousness, the cross-frequency coupled activity needs to be functionally coupled with a consciousness enabling network, which is mediated via the theta oscillation as a carrier wave for the percept and alpha for attentional processing. As such, tinnitus is an emergent property of auditory (mild deafferentation) or parahippocampal (severe deafferentation) gamma-band activity connected to a consciousness enabling network via low-frequency carrier waves. From a Bayesian point of view, the gamma activity of the TCD represents a bottom-up transmitted prediction error.
Conflict of Interest Statement
The authors declare that the research was conducted in the absence of any commercial or financial relationships that could be construed as a potential conflict of interest.
References
1. Tonndorf J. The analogy between tinnitus and pain: a suggestion for a physiological basis of chronic tinnitus. Hear Res (1987) 28:271–275. doi: 10.1016/0378-5955(87)90054-2
3. De Ridder D, Elgoyhen AB, Romo R, Langguth B. Phantom percepts: tinnitus and pain as persisting aversive memory networks. Proc Natl Acad Sci U S A (2011) 108:8075-8080. doi:10.1073/pnas.1018466108
4. Jastreboff PJ. Phantom auditory perception (tinnitus): mechanisms of generation and perception. Neurosci Res (1990) 8:221–254. doi:10.1016/0168-0102(90)90031-9
5. Eggermont JJ, Roberts LE. The neuroscience of tinnitus. Trends Neurosci (2004) 27:676-682. doi:10.1016/j.tins.2004.08.010
6. Norena AJ. An integrative model of tinnitus based on a central gain controlling neural sensitivity. Neurosci Biobehav Rev (2011) 35:1089-1109. doi:10.1016/j.neubiorev.2010.11.003
7. Moller AR. The role of neural plasticity in tinnitus. Prog Brain Res (2007) 166:37–45. doi:10.1016/S0079-6123(07)66003-8
8. Muhlnickel W, Elbert T, Taub E, Flor H. Reorganization of auditory cortex in tinnitus. Proc Natl Acad Sci U S A (1998) 95:10340–10343. doi:10.1073/pnas.95.17.10340
9. Yang S, Weiner BD, Zhang LS, Cho SJ, Bao S. Homeostatic plasticity drives tinnitus perception in an animal model. Proc Natl Acad Sci U S A (2011) 108:14974–14979. doi:10.1073/pnas.1107998108
10. Schaette R, Kempter R. Development of tinnitus-related neuronal hyperactivity through homeostatic plasticity after hearing loss: a computational model. Eur J Neurosci (2006) 23:3124–3138. doi:10.1111/j.1460-9568.2006.04774.x
11. Schaette R, Kempter R. Computational models of neurophysiological correlates of tinnitus. Front Syst Neurosci (2012) 6:34. doi:10.3389/fnsys.2012.00034
12. Tass PA, Popovych OV. Unlearning tinnitus-related cerebral synchrony with acoustic coordinated reset stimulation: theoretical concept and modelling. Biol Cybern (2012) 106:27–36. doi:10.1007/s00422-012-0479-5
13. De Ridder D, Fransen H, Francois O, Sunaert S, Kovacs S, Van De Heyning P. Amygdalohippocampal involvement in tinnitus and auditory memory. Acta Otolaryngol (2006) 556:50–53. doi:10.1080/03655230600895580
14. De Ridder D, Vanneste S, Freeman W. The Bayesian brain: phantom percepts resolve sensory uncertainty. Neurosci Biobehav Rev (2012) 44:4–15. doi:10.1016/j.neubiorev.2012.04.001
15. Zenner HP, Pfister M, Birbaumer N. Tinnitus sensitization: sensory and psychophysiological aspects of a new pathway of acquired centralization of chronic tinnitus. Otol Neurotol (2006) 27:1054–1063. doi:10.1097/01.mao.0000231604.64079.77
16. Engineer ND, Riley JR, Seale JD, Vrana WA, Shetake JA, Sudanagunta SP, et al. Reversing pathological neural activity using targeted plasticity. Nature (2011) 470:101–104. doi:10.1038/nature09656
17. Shore SE, Zhou J. Somatosensory influence on the cochlear nucleus and beyond. Hear Res (2006) 216–217:90–99. doi:10.1016/j.heares.2006.01.006
18. Kaltenbach JA. Summary of evidence pointing to a role of the dorsal cochlear nucleus in the etiology of tinnitus. Acta Otolaryngol (2006) 556:20–26. doi:10.1080/03655230600895309
19. Rauschecker JP, Leaver AM, Muhlau M. Tuning out the noise: limbic-auditory interactions in tinnitus. Neuron (2010) 66:819–826. doi:10.1016/j.neuron.2010.04.032
20. De Ridder D, Vanneste S, Menovsky T, Langguth B. Surgical brain modulation for tinnitus: the past, present and future. J Neurosurg Sci (2012) 56:323–340.
22. Schlee W, Weisz N, Bertrand O, Hartmann T, Elbert T. Using auditory steady state responses to outline the functional connectivity in the tinnitus brain. PLoS One (2008) 3:e3720. doi:10.1371/journal.pone.0003720
24. Dehaene S, Kerszberg M, Changeux JP. A neuronal model of a global workspace in effortful cognitive tasks. Proc Natl Acad Sci U S A (1998) 95:14529–14534. doi:10.1073/pnas.95.24.14529
25. Boly M, Faymonville ME, Peigneux P, Lambermont B, Damas F, Luxen A, et al. Cerebral processing of auditory and noxious stimuli in severely brain injured patients: differences between VS and MCS. Neuropsychol Rehabil (2005) 15:283–289. doi:10.1080/09602010443000371
26. Boly M, Faymonville ME, Peigneux P, Lambermont B, Damas P, Del Fiore G, et al. Auditory processing in severely brain injured patients: differences between the minimally conscious state and the persistent vegetative state. Arch Neurol (2004) 61:233–238. doi:10.1001/archneur.61.2.233
27. Laureys S, Faymonville ME, Degueldre C, Fiore GD, Damas P, Lambermont B, et al. Auditory processing in the vegetative state. Brain (2000) 123(Pt 8):1589–1601. doi:10.1093/brain/123.8.1589
29. Freeman WJ. The emergence of mind and emotion in the evolution of neocortex. Riv Psichiatr (2011) 46:281–287. doi:10.1708/1009.10972
30. De Ridder D, Vanneste S, Weisz N, Londero A, Schlee W, Elgoyhen AB, et al. An integrative model of auditory phantom perception: tinnitus as a unified percept of interacting separable subnetworks. Neurosci Biobehav Rev (2013) 44:16–32. doi:10.1016/j.neubiorev.2013.03.021
31. Varela F, Lachaux JP, Rodriguez E, Martinerie J. The brainweb: phase synchronization and large-scale integration. Nat Rev Neurosci (2001) 2:229–239. doi:10.1038/35067550
32. Llinas RR. The intrinsic electrophysiological properties of mammalian neurons: insights into central nervous system function. Science (1988) 242:1654–1664. doi:10.1126/science.3059497
33. Palva S, Palva JM. Discovering oscillatory interaction networks with M/EEG: challenges and breakthroughs. Trends Cogn Sci (2012) 16:219–230. doi:10.1016/j.tics.2012.02.004
34. Lisman JE, Jensen O. The theta-gamma neural code. Neuron (2013) 77:1002–1016. doi:10.1016/j.neuron.2013.03.007
35. Canolty RT, Edwards E, Dalal SS, Soltani M, Nagarajan SS, Kirsch HE, et al. High gamma power is phase-locked to theta oscillations in human neocortex. Science (2006) 313:1626–1628. doi:10.1126/science.1128115
36. Monto S, Palva S, Voipio J, Palva JM. Very slow EEG fluctuations predict the dynamics of stimulus detection and oscillation amplitudes in humans. J Neurosci (2008) 28:8268–8272. doi:10.1523/JNEUROSCI.1910-08.2008
37. Adamchic I, Langguth B, Hauptmann C, Tass PA. Abnormal cross-frequency coupling in the tinnitus network. Front Neurosci (2014) 8:284. doi:10.3389/fnins.2014.00284
38. Jensen O, Colgin LL. Cross-frequency coupling between neuronal oscillations. Trends Cogn Sci (2007) 11:267–269. doi:10.1016/j.tics.2007.05.003
39. Llinas RR, Ribary U, Jeanmonod D, Kronberg E, Mitra PP. Thalamocortical dysrhythmia: a neurological and neuropsychiatric syndrome characterized by magnetoencephalography. Proc Natl Acad Sci U S A (1999) 96:15222–15227. doi:10.1073/pnas.96.26.15222
40. Laureys S. Eyes open, brain shut. Sci Am (2007) 296:84–89. doi:10.1038/scientificamerican0507-84
41. De Ridder D, Vanneste S, Weisz N, Londero A, Schlee W, Elgoyhen AB, et al. An integrative model of auditory phantom perception: tinnitus as a unified percept of interacting separable subnetworks. Neurosci Biobehav Rev (2014) 44C:16–32. doi:10.1016/j.neubiorev.2013.03.021
42. Ratnayake SA, Jayarajan V, Bartlett J. Could an underlying hearing loss be a significant factor in the handicap caused by tinnitus? Noise Health (2009) 11(44): 156–60. doi:10.4103/1463-1741.53362
43. Oishi N, Shinden S, Kanzaki S, Saito H, Inoue Y, Ogawa K. Influence of depressive symptoms, state anxiety, and pure-tone thresholds on the tinnitus handicap inventory in Japan. Int J Audiol (2011) 50(7): 491–5. doi:10.3109/14992027.2011.560904
44. Vanneste S, Plazier M, der Loo E, de Heyning PV, Congedo M, De Ridder D. The neural correlates of tinnitus-related distress. Neuroimage (2010) 52:470–480. doi:10.1016/j.neuroimage.2010.04.029
45. Vanneste S, Plazier M, van der Loo E, Van de Heyning P, De Ridder D. The differences in brain activity between narrow band noise and pure tone tinnitus. PLoS One (2010) 5:e13618. doi:10.1371/journal.pone.0013618
46. De Ridder D, van der Loo E, Vanneste S, Gais S, Plazier M, Kovacs S, et al. Theta-gamma dysrhythmia and auditory phantom perception. J Neurosurg (2011) 114:912–921. doi:10.3171/2010.11.JNS10335
47. De Ridder D, Vanneste S, Congedo M. The distressed brain: a group blind source separation analysis on tinnitus. PLoS One (2011) 6:e24273. doi:10.1371/journal.pone.0024273
48. De Ridder D. A heuristic pathophysiological model of tinnitus. In: Moller A, Langguth B, De Ridder D, Kleinjung T, editors. Textbook of Tinnitus. New York, NY: Springer (2011). p. 171–198.
49. Moulton EA, Elman I, Pendse G, Schmahmann J, Becerra L, Borsook D. Aversion-related circuitry in the cerebellum: responses to noxious heat and unpleasant images. J Neurosci (2011) 31(10):3795–804. doi:10.1523/JNEUROSCI.6709-10.2011
50. De Ridder D, De Mulder G, Menovsky T, Sunaert S, Kovacs S. Electrical stimulation of auditory and somatosensory cortices for treatment of tinnitus and pain. Prog Brain Res (2007) 166:377–388. doi:10.1016/S0079-6123(07)66036-1
51. De Ridder D, Van de Heyning P. The Darwinian plasticity hypothesis for tinnitus and pain. Prog Brain Res (2007) 166:55–60. doi:10.1016/S0079-6123(07)66005-1
52. Jeanmonod D, Magnin M, Morel A. Low-threshold calcium spike bursts in the human thalamus. Common physiopathology for sensory, motor and limbic positive symptoms. Brain (1996) 119(Pt 2):363–375. doi:10.1093/brain/119.2.363
53. Sedley W, Cunningham MO. Do cortical gamma oscillations promote or suppress perception? An under-asked question with an over-assumed answer. Front Hum Neurosci (2013) 7:595. doi:10.3389/fnhum.2013.00595
54. Sedley W, Teki S, Kumar S, Barnes GR, Bamiou DE, Griffiths TD. Single-subject oscillatory gamma responses in tinnitus. Brain (2012) 135:3089–3100. doi:10.1093/brain/aws220
55. Jasper HH, Andrews HL. Brain potentials and voluntary muscle activity in man. J Neurophysiol (1938) 1:87–100.
56. Bressler SL, Freeman WJ. Frequency analysis of olfactory system EEG in cat, rabbit, and rat. Electroencephalogr Clin Neurophysiol (1980) 50:19–24. doi:10.1016/0013-4694(80)90319-3
57. Gray CM, Konig P, Engel AK, Singer W. Oscillatory responses in cat visual cortex exhibit inter-columnar synchronization which reflects global stimulus properties. Nature (1989) 338:334–337. doi:10.1038/338334a0
58. Joliot M, Ribary U, Llinas R. Human oscillatory brain activity near 40 Hz coexists with cognitive temporal binding. Proc Natl Acad Sci U S A (1994) 91:11748–11751. doi:10.1073/pnas.91.24.11748
59. Palva S, Linkenkaer-Hansen K, Naatanen R, Palva JM. Early neural correlates of conscious somatosensory perception. J Neurosci (2005) 25:5248–5258. doi:10.1523/JNEUROSCI.0141-05.2005
60. Steriade M. Corticothalamic resonance, states of vigilance and mentation. Neuroscience (2000) 101:243–276. doi:10.1016/S0306-4522(00)00353-5
61. Gray CM, Singer W. Stimulus-specific neuronal oscillations in orientation columns of cat visual cortex. Proc Natl Acad Sci U S A (1989) 86:1698–1702. doi:10.1073/pnas.86.5.1698
62. Tiitinen H, Sinkkonen J, Reinikainen K, Alho K, Lavikainen J, Naatanen R. Selective attention enhances the auditory 40-Hz transient response in humans. Nature (1993) 364:59–60. doi:10.1038/364059a0
63. Llinas R, Ribary U, Contreras D, Pedroarena C. The neuronal basis for consciousness. Philos Trans R Soc Lond B Biol Sci (1998) 353:1841–1849. doi:10.1098/rstb.1998.0336
64. Llinas R, Ribary U, Joliot M, Wang X. Content and context in temporal thalamocortical binding. In: Buzsaki G, Llinas R, Singer W, editors. Temporal Coding in the Brain. Berlin: Springer-Verlag (1994). p. 251–272.
65. Ribary U, Ioannides AA, Singh KD, Hasson R, Bolton JP, Lado F, et al. Magnetic field tomography of coherent thalamocortical 40-Hz oscillations in humans. Proc Natl Acad Sci U S A (1991) 88:11037–11041. doi:10.1073/pnas.88.24.11037
66. Crone NE, Boatman D, Gordon B, Hao L. Induced electrocorticographic gamma activity during auditory perception. Brazier award-winning article, 2001. Clin Neurophysiol (2001) 112:565–582. doi:10.1016/S1388-2457(00)00545-9
67. Schadow J, Lenz D, Thaerig S, Busch NA, Frund I, Herrmann CS. Stimulus intensity affects early sensory processing: sound intensity modulates auditory evoked gamma-band activity in human EEG. Int J Psychophysiol (2007) 65:152–161. doi:10.1016/j.ijpsycho.2007.04.006
68. Arnal LH, Wyart V, Giraud AL. Transitions in neural oscillations reflect prediction errors generated in audiovisual speech. Nat Neurosci (2011) 14:797–801. doi:10.1038/nn.2810
69. Kaiser J, Hertrich I, Ackermann H, Lutzenberger W. Gamma-band activity over early sensory areas predicts detection of changes in audiovisual speech stimuli. Neuroimage (2006) 30:1376–1382. doi:10.1016/j.neuroimage.2005.10.042
70. Engel AK, Fries P. Beta-band oscillations – signalling the status quo? Curr Opin Neurobiol (2010) 20:156–165. doi:10.1016/j.conb.2010.02.015
71. Buzsaki G, Draguhn A. Neuronal oscillations in cortical networks. Science (2004) 304:1926–1929. doi:10.1126/science.1099745
72. von Stein A, Sarnthein J. Different frequencies for different scales of cortical integration: from local gamma to long range alpha/theta synchronization. Int J Psychophysiol (2000) 38:301–313. doi:10.1016/S0167-8760(00)00172-0
73. Fukushima M, Saunders RC, Leopold DA, Mishkin M, Averbeck BB. Spontaneous high-gamma band activity reflects functional organization of auditory cortex in the awake macaque. Neuron (2012) 74:899–910. doi:10.1016/j.neuron.2012.04.014
74. Arnal LH, Poeppel D, Giraud AL. Temporal coding in the auditory cortex. Handb Clin Neurol (2015) 129:85–98. doi:10.1016/B978-0-444-62630-1.00005-6
75. Tiesinga P, Sejnowski TJ. Cortical enlightenment: are attentional gamma oscillations driven by ING or PING? Neuron (2009) 63:727–732. doi:10.1016/j.neuron.2009.09.009
76. Borgers C, Epstein S, Kopell NJ. Gamma oscillations mediate stimulus competition and attentional selection in a cortical network model. Proc Natl Acad Sci U S A (2008) 105:18023-18028. doi:10.1073/pnas.0809511105
77. Borgers C, Epstein S, Kopell NJ. Background gamma rhythmicity and attention in cortical local circuits: a computational study. Proc Natl Acad Sci U S A (2005) 102:7002–7007. doi:10.1073/pnas.0502366102
78. Schroeder CE, Lakatos P. Low-frequency neuronal oscillations as instruments of sensory selection. Trends Neurosci (2009) 32:9–18. doi:10.1016/j.tins.2008.09.012
79. Gaillard R, Dehaene S, Adam C, Clemenceau S, Hasboun D, Baulac M, et al. Converging intracranial markers of conscious access. PLoS Biol (2009) 7:e61. doi:10.1371/journal.pbio.1000061
80. Melloni L, Molina C, Pena M, Torres D, Singer W, Rodriguez E. Synchronization of neural activity across cortical areas correlates with conscious perception. J~Neurosci (2007) 27:2858–2865. doi:10.1523/JNEUROSCI.4623-06.2007
81. Llinas RR, Choi S, Urbano FJ, Shin HS. Gamma-band deficiency and abnormal thalamocortical activity in P/Q-type channel mutant mice. Proc Natl Acad Sci U S A (2007) 104:17819–17824. doi:10.1073/pnas.0707945104
82. Gurtubay IG, Alegre M, Labarga A, Malanda A, Artieda J. Gamma band responses to target and non-target auditory stimuli in humans. Neurosci Lett (2004) 367:6–9. doi:10.1016/j.neulet.2004.05.104
83. van der Loo E, Gais S, Congedo M, Vanneste S, Plazier M, Menovsky T, et al. Tinnitus intensity dependent gamma oscillations of the contralateral auditory cortex. PLoS One (2009) 4:e7396. doi:10.1371/journal.pone.0007396
84. Weisz N, Muller S, Schlee W, Dohrmann K, Hartmann T, Elbert T. The neural code of auditory phantom perception. J Neurosci (2007) 27:1479–1484. doi:10.1523/JNEUROSCI.3711-06.2007
85. Llinas R, Urbano FJ, Leznik E, Ramirez RR, van Marle HJ. Rhythmic and dysrhythmic thalamocortical dynamics: GABA systems and the edge effect. Trends Neurosci (2005) 28:325–333. doi:10.1016/j.tins.2005.04.006
86. Vanneste S, Plazier M, van der Loo E, Van de Heyning P, De Ridder D. The difference between uni- and bilateral auditory phantom percept. Clin Neurophysiol (2011) 122:578–587. doi:10.1016/j.clinph.2010.07.022
87. Vanneste S, De Ridder D. Bifrontal transcranial direct current stimulation modulates tinnitus intensity and tinnitus-distress-related brain activity. Eur J Neurosci (2011) 34:605–614. doi:10.1111/j.1460-9568.2011.07778.x
88. Adamchic I, Hauptmann C, Tass PA. Changes of oscillatory activity in pitch processing network and related tinnitus relief induced by acoustic CR neuromodulation. Front Syst Neurosci (2012) 6:18. doi:10.3389/fnsys.2012.00018
89. Adamchic I, Toth T, Hauptmann C, Tass PA. Reversing pathologically increased EEG power by acoustic coordinated reset neuromodulation. Hum Brain Mapp (2014) 35:2099–2118. doi:10.1002/hbm.22314
90. Vanneste S, van Dongen M, De Vree B, Hiseni S, van der Velden E, Strydis C, et al. Does enriched acoustic environment in humans abolish chronic tinnitus clinically and electrophysiologically? A double blind placebo controlled study. Hear Res (2012) 296:141–148. doi:10.1016/j.heares.2012.10.003
91. Borst A, Theunissen FE. Information theory and neural coding. Nat Neurosci (1999) 2:947–957. doi:10.1038/4508
92. Lorenz I, Muller N, Schlee W, Hartmann T, Weisz N. Loss of alpha power is related to increased gamma synchronization-A marker of reduced inhibition in tinnitus? Neurosci Lett (2009) 453:225–228. doi:10.1016/j.neulet.2009.02.028
93. Ramirez RR, Kopell BH, Butson CR, Gaggl W, Friedland DR, Baillet S. Neuromagnetic source imaging of abnormal spontaneous activity in tinnitus patient modulated by electrical cortical stimulation. Conf Proc IEEE Eng Med Biol Soc (2009) 1:1940–1944. doi:10.1109/IEMBS.2009.5333457
94. Adjamian P, Sereda M, Zobay O, Hall DA, Palmer AR. Neuromagnetic indicators of tinnitus and tinnitus masking in patients with and without hearing loss. J~Assoc Res Otolaryngol (2012) 13:715–731. doi:10.1007/s10162-012-0340-5
95. Llinas RR, Steriade M. Bursting of thalamic neurons and states of vigilance. J~Neurophysiol (2006) 95:3297–3308. doi:10.1152/jn.00166.2006
96. Freeman WJ. The wave packet: an action potential for the 21st century. J Integr Neurosci (2003) 2:3–30. doi:10.1142/S0219635203000214
97. Freeman WJ. A field-theoretic approach to understanding scale-free neocortical dynamics. Biol Cybern (2005) 92:350–359. doi:10.1007/s00422-005-0563-1
98. Freeman WJ, Rogers LJ. Fine temporal resolution of analytic phase reveals episodic synchronization by state transitions in gamma EEGs. J Neurophysiol (2002) 87:937–945.
99. De Ridder D, Congedo M, Vanneste S. The neural correlates of subjectively perceived and passively matched loudness perception in auditory phantom perception. Brain Behav (2015) 5(5): e00331. doi:10.1002/brb3.331
100. Cain SM, Snutch TP. T-type calcium channels in burst-firing, network synchrony, and epilepsy. Biochim Biophys Acta (2013) 1828:1572–1578. doi:10.1016/j.bbamem.2012.07.028
101. Jahnsen H, Llinas R. Voltage-dependent burst-to-tonic switching of thalamic cell activity: an in vitro study. Arch Ital Biol (1984) 122:73–82.
102. Cain SM, Snutch TP. Contributions of T-type calcium channel isoforms to neuronal firing. Channels (2010) 4:475–482. doi:10.4161/chan.4.6.14106
103. Landry P, Deschenes M. Intracortical arborizations and receptive fields of identified ventrobasal thalamocortical afferents to the primary somatic sensory cortex in the cat. J Comp Neurol (1981) 199:345–371. doi:10.1002/cne.901990304
104. Destexhe A, Bal T, McCormick DA, Sejnowski TJ. Ionic mechanisms underlying synchronized oscillations and propagating waves in a model of ferret thalamic slices. J Neurophysiol (1996) 76:2049-2070.
105. De Ridder D, Vanneste S, Freeman W. The Bayesian brain: phantom percepts resolve sensory uncertainty. Neurosci Biobehav Rev (2014) 44C:4–15. doi:10.1016/j.neubiorev.2012.04.001
106. Doesburg SM, Green JJ, McDonald JJ, Ward LM. Theta modulation of inter-regional gamma synchronization during auditory attention control. Brain Res (2012) 1431:77–85. doi:10.1016/j.brainres.2011.11.005
107. Saalmann YB, Pinsk MA, Wang L, Li X, Kastner S. The pulvinar regulates information transmission between cortical areas based on attention demands. Science (2012) 337:753–756. doi:10.1126/science.1223082
108. Roux F, Uhlhaas PJ. Working memory and neural oscillations: alpha-gamma versus theta-gamma codes for distinct WM information? Trends Cogn Sci (2014) 18:16–25. doi:10.1016/j.tics.2013.10.010
109. Vanneste S, Congedo M, De Ridder D. Pinpointing a highly specific pathological functional connection that turns phantom sound into distress. Cereb Cortex (2013) 24(9):2268–2282. doi:10.1093/cercor/bht068
110. Vanneste S, De Ridder D. Brain areas controlling heart rate variability in tinnitus and tinnitus-related distress. PLoS One (2013) 8:e59728. doi:10.1371/journal.pone.0059728
111. Joos K, Vanneste S, De Ridder D. Disentangling depression and distress networks in the tinnitus brain. PLoS One (2012) 7:e40544. doi:10.1371/journal.pone.0040544
112. Vanneste S, Joos K, Langguth B, To WT, De Ridder D. Neuronal correlates of maladaptive coping: an EEG-study in tinnitus patients. PLoS One (2014) 9:e88253. doi:10.1371/journal.pone.0088253
113. van der Loo E, Congedo M, Vanneste S, De Heyning PV, De Ridder D. Insular lateralization in tinnitus distress. Auton Neurosci (2011) 165:191–194. doi:10.1016/j.autneu.2011.06.007
114. Axelsson A, Ringdahl A. Tinnitus – a study of its prevalence and characteristics. Br J Audiol (1989) 23:53–62. doi:10.3109/03005368909077819
115. Vanneste S, de Heyning PV, Ridder DD. Contralateral parahippocampal gamma-band activity determines noise-like tinnitus laterality: a region of interest analysis. Neuroscience (2011) 199:481–490. doi:10.1016/j.neuroscience.2011.07.067
116. Vanneste S, Focquaert F, Van de Heyning P, De Ridder D. Different resting state brain activity and functional connectivity in patients who respond and not respond to bifrontal tDCS for tinnitus suppression. Exp Brain Res (2011) 210:217–227. doi:10.1007/s00221-011-2617-z
117. Vanneste S, van de Heyning P, De Ridder D. The neural network of phantom sound changes over time: a comparison between recent-onset and chronic tinnitus patients. Eur J Neurosci (2011) 34:718–731. doi:10.1111/j.1460-9568.2011.07793.x
118. Craig AD. A new view of pain as a homeostatic emotion. Trends Neurosci (2003) 26:303–307. doi:10.1016/S0166-2236(03)00123-1
119. Uddin LQ. Salience processing and insular cortical function and dysfunction. Nat Rev Neurosci (2015) 16(1): 55–61. doi:10.1038/nrn3857
120. Simmons WK, Rapuano KM, Kallman SJ, Ingeholm JE, Miller B, Gotts SJ, et al. Category-specific integration of homeostatic signals in caudal but not rostral human insula. Nat Neurosci (2013) 16(11): 1551–2. doi:10.1038/nn.3535
121. Bossaerts P. Risk and risk prediction error signals in anterior insula. Brain Struct Funct (2010) 214(5–6): 645–53. doi:10.1007/s00429-010-0253-1
122. Bamiou DE, Musiek FE, Luxon LM. The insula (Island of Reil) and its role in auditory processing. Literature review. Brain Res Brain Res Rev (2003) 42(2): 143–54. doi:10.1016/S0165-0173(03)00172-3
123. Rinne T, Koistinen S, Salonen O, Alho K. Task-dependent activations of human auditory cortex during pitch discrimination and pitch memory tasks. J Neurosci (2009) 29(42): 13338–43. doi:10.1523/JNEUROSCI.3012-09.2009
124. Steriade M, McCarley R. Intrinsic Electrophysiological Properties of Brainstem and Forebrain Neurons, BRain Control of Wakefulnessand Sleep. New York, NY: Springer (2005). p. 155–209.
125. Llinas RR, Roy S. The ‘prediction imperative’ as the basis for self-awareness. Philos Trans R Soc Lond B Biol Sci (2009) 364:1301–1307. doi:10.1098/rstb.2008.0309
126. Schacter DL, Addis DR, Buckner RL. Remembering the past to imagine the future: the prospective brain. Nat Rev Neurosci (2007) 8:657–661. doi:10.1038/nrn2213
127. Friston K. The free-energy principle: a unified brain theory? Nat Rev Neurosci (2010) 11:127–138. doi:10.1038/nrn2787
128. Knill DC, Pouget A. The Bayesian brain: the role of uncertainty in neural coding and computation. Trends Neurosci (2004) 27:712–719. doi:10.1016/j.tins.2004.10.007
129. Freeman WJ. Neurodynamic models of brain in psychiatry. Neuropsychopharmacology (2003) 28(Suppl 1):S54–S63. doi:10.1038/sj.npp.1300147
130. Arnal LH, Doelling KB, Poeppel D. Delta-beta coupled oscillations underlie temporal prediction accuracy. Cereb Cortex (2014). doi:10.1093/cercor/bhu103
131. Arnal LH, Giraud AL. Cortical oscillations and sensory predictions. Trends Cogn Sci (2012) 16:390–398. doi:10.1016/j.tics.2012.05.003
132. Palva S, Palva JM. New vistas for alpha-frequency band oscillations. Trends Neurosci (2007) 30:150–158. doi:10.1016/j.tins.2007.02.001
133. Schulman JJ, Cancro R, Lowe S, Lu F, Walton KD, Llinas RR. Imaging of thalamocortical dysrhythmia in neuropsychiatry. Front Hum Neurosci (2011) 5:69. doi:10.3389/fnhum.2011.00069
134. Meyer M, Luethi MS, Neff P, Langer N, Büchi S. Disentangling tinnitus distress and tinnitus presence by means of EEG power analysis. Neural Plast (2014) 2014:1–13. doi:10.1155/2014/468546
135. Song JJ. Dysfunctional noise cancelling of the rostral anterior cingulate cortex in tinnitus patients. PLoS One (2015) 10:e0123538. doi:10.1371/journal.pone.0123538
136. Tass PA, Adamchic I, Freund HJ, von Stackelberg T, Hauptmann C. Counteracting tinnitus by acoustic coordinated reset neuromodulation. Restor Neurol Neurosci (2012) 30(2):137–59. doi:10.3233/RNN-2012-110218
137. Muller N, Lorenz I, Langguth B, Weisz N. rTMS induced tinnitus relief is related to an increase in auditory cortical alpha activity. PLoS One (2013) 8:e55557. doi:10.1371/journal.pone.0055557
138. Hartmann T, Lorenz I, Muller N, Langguth B, Weisz N. The effects of neurofeedback on oscillatory processes related to tinnitus. Brain Topogr (2014) 27(1): 149–57. doi:10.1007/s10548-013-0295-9
139. Jeanmonod D, Schulman J, Ramirez R, Cancro R, Lanz M, Morel A, et al. Neuropsychiatric thalamocortical dysrhythmia: surgical implications. Neurosurg Clin N Am (2003) 14:251–265. doi:10.1016/S1042-3680(02)00116-X
140. Koob GF, Le Moal M. Drug addiction, dysregulation of reward, and allostasis. Neuropsychopharmacology (2001) 24:97–129. doi:10.1016/S0893-133X(00)00195-0
141. De Ridder D, Verstraeten E, Van der Kelen K, De Mulder G, Sunaert S, Verlooy J, et al. Transcranial magnetic stimulation for tinnitus: influence of tinnitus duration on stimulation parameter choice and maximal tinnitus suppression. Otol Neurotol (2005) 26:616–619. doi:10.1097/01.mao.0000178146.91139.3c
142. Kleinjung T, Steffens T, Sand P, Murthum T, Hajak G, Strutz J, et al. Which tinnitus patients benefit from transcranial magnetic stimulation? Otolaryngol Head Neck Surg (2007) 137:589–595. doi:10.1016/j.otohns.2006.12.007
143. Khedr EM, Rothwell JC, Ahmed MA, El-Atar A. Effect of daily repetitive transcranial magnetic stimulation for treatment of tinnitus: comparison of different stimulus frequencies. J Neurol Neurosurg Psychiatry (2008) 79:212–215. doi:10.1136/jnnp.2007.127712
144. De Ridder D, Vanneste S, Adriaenssens I, Lee AP, Plazier M, Menovsky T, et al. Microvascular decompression for tinnitus: significant improvement for tinnitus intensity without improvement for distress. A 4-year limit. Neurosurgery (2010) 66:656–660. doi:10.1227/01.NEU.0000366110.87836.53
145. Moller MB, Moller AR, Jannetta PJ, Jho HD. Vascular decompression surgery for severe tinnitus: selection criteria and results. Laryngoscope (1993) 103:421–427. doi:10.1002/lary.5541030410
147. Weisz N, Hartmann T, Dohrmann K, Schlee W, Norena A. High-frequency tinnitus without hearing loss does not mean absence of deafferentation. Hear Res (2006) 222:108–114. doi:10.1016/j.heares.2006.09.003
149. Rajan R. Receptor organ damage causes loss of cortical surround inhibition without topographic map plasticity. Nat Neurosci (1998) 1:138–143. doi:10.1038/388
150. Langers DR, de Kleine E, van Dijk P. Tinnitus does not require macroscopic tonotopic map reorganization. Front Syst Neurosci (2012) 6:2. doi:10.3389/fnsys.2012.00002
151. Chen L, Trautwein PG, Shero M, Salvi RJ. Tuning, spontaneous activity and tonotopic map in chicken cochlear ganglion neurons following sound-induced hair cell loss and regeneration. Hear Res (1996) 98:152–164. doi:10.1016/0378-5955(96)00086-X
152. Hsieh CY, Hong CT, Cramer KS. Deletion of EphA4 enhances deafferentation-induced ipsilateral sprouting in auditory brainstem projections. J Comp Neurol (2007) 504:508–518. doi:10.1002/cne.21465
153. De Ridder D, Vanneste S. Targeting the parahippocampal area by auditory cortex stimulation in tinnitus. Brain Stimul (2014) 7(5):709–717. doi:10.1016/j.brs.2014.04.004
154. Maudoux A, Lefebvre P, Cabay JE, Demertzi A, Vanhaudenhuyse A, Laureys S, et al. Connectivity graph analysis of the auditory resting state network in tinnitus. Brain Res (2012) 1485:10–21. doi:10.1016/j.brainres.2012.05.006
155. Maudoux A, Lefebvre P, Cabay JE, Demertzi A, Vanhaudenhuyse A, Laureys S, et al. Auditory resting-state network connectivity in tinnitus: a functional MRI study. PLoS One (2012) 7:e36222. doi:10.1371/journal.pone.0036222
156. Moazami-Goudarzi M, Michels L, Weisz N, Jeanmonod D. Temporo-insular enhancement of EEG low and high frequencies in patients with chronic tinnitus. QEEG study of chronic tinnitus patients. BMC Neurosci (2010) 11:40. doi:10.1186/1471-2202-11-40
157. Song JJ, Vanneste S, Schlee W, Van de Heyning P, De Ridder D. Onset-related differences in neural substrates of tinnitus-related distress: the anterior cingulate cortex in late-onset tinnitus, and the frontal cortex in early-onset tinnitus. Brain Struct Funct (2015) 220:571–584. doi:10.1007/s00429-014-0708-x
158. Vanneste S, De Ridder D. The auditory and non-auditory brain areas involved in tinnitus. An emergent property of multiple parallel overlapping subnetworks. Front Syst Neurosci (2012) 6:31. doi:10.3389/fnsys.2012.00031
159. Vanneste S, De Ridder D. The use of alcohol as a moderator for tinnitus-related distress. Brain Topogr (2012) 25:97–105. doi:10.1007/s10548-011-0191-0
160. Vanneste S, Joos K, De Ridder D. Prefrontal cortex based sex differences in tinnitus perception: same tinnitus intensity, same tinnitus distress, different mood. PLoS One (2012) 7:e31182. doi:10.1371/journal.pone.0031182
161. Laureano MR, Onishi ET, Bressan RA, Castiglioni ML, Batista IR, Reis MA, et al. Memory networks in tinnitus: a functional brain image study. PLoS One (2014) 9:e87839. doi:10.1371/journal.pone.0087839
162. Schecklmann M, Landgrebe M, Poeppl TB, Kreuzer P, Manner P, Marienhagen J, et al. Neural correlates of tinnitus duration and distress: a positron emission tomography study. Hum Brain Mapp (2013) 34:233–240. doi:10.1002/hbm.21426
163. Song JJ, De Ridder D, Van de Heyning P, Vanneste S. Mapping tinnitus-related brain activation: an activation-likelihood estimation metaanalysis of PET studies. J Nucl Med (2012) 53(10):1550–1557. doi:10.2967/jnumed.112.102939
164. Schlee W, Mueller N, Hartmann T, Keil J, Lorenz I, Weisz N. Mapping cortical hubs in tinnitus. BMC Biol (2009) 7:80. doi:10.1186/1741-7007-7-80
165. Desimone R, Gross CG. Visual areas in the temporal cortex of the macaque. Brain Res (1979) 178:363–380. doi:10.1016/0006-8993(79)90699-1
166. Tulving E, Markowitsch HJ. Memory beyond the hippocampus. Curr Opin Neurobiol (1997) 7:209–216. doi:10.1016/S0959-4388(97)80009-8
167. Boutros NN, Mears R, Pflieger ME, Moxon KA, Ludowig E, Rosburg T. Sensory gating in the human hippocampal and rhinal regions: regional differences. Hippocampus (2008) 18:310–316. doi:10.1002/hipo.20388
168. Engelien A, Stern E, Isenberg N, Engelien W, Frith C, Silbersweig D. The parahippocampal region and auditory-mnemonic processing. Ann N Y Acad Sci (2000) 911:477–485. doi:10.1111/j.1749-6632.2000.tb06750.x
Keywords: thalamocortical dysrhythmia, theta, gamma, EEG, MEG, tinnitus, cross-frequency coupling, Bayes
Citation: De Ridder D, Vanneste S, Langguth B and Llinas R (2015) Thalamocortical dysrhythmia: a theoretical update in tinnitus. Front. Neurol. 6:124. doi: 10.3389/fneur.2015.00124
Received: 09 January 2015; Accepted: 14 May 2015;
Published: 09 June 2015
Edited by:
Arnaud Norena, Université de Provence, FranceReviewed by:
Martin Meyer, University of Zurich, SwitzerlandPeyman Adjamian, MRC Institute of Hearing Research, UK
Audrey Maudoux, Georgetown University, USA
Copyright: © 2015 De Ridder, Vanneste, Langguth and Llinas. This is an open-access article distributed under the terms of the Creative Commons Attribution License (CC BY). The use, distribution or reproduction in other forums is permitted, provided the original author(s) or licensor are credited and that the original publication in this journal is cited, in accordance with accepted academic practice. No use, distribution or reproduction is permitted which does not comply with these terms.
*Correspondence: Dirk De Ridder, BRAI2N, Section of Neurosurgery, Department of Surgical Sciences, Dunedin School of Medicine, University of Otago, Dunedin Public Hospital, Great King Street, Dunedin Central, Dunedin, New Zealand,ZGlyay5kZXJpZGRlckBvdGFnby5hYy5ueg==http://www.brai2n.org