- Department of Pharmacology and Toxicology, School of Medical Sciences, University of Otago Medical School, Dunedin, New Zealand
For decades it has been speculated that there is a close association between the vestibular system and spatial memories constructed by areas of the brain such as the hippocampus. While many animal studies have been conducted which support this relationship, only in the last 10 years have detailed quantitative studies been carried out in patients with vestibular disorders. The majority of these studies suggest that complete bilateral vestibular loss results in spatial memory deficits that are not simply due to vestibular reflex dysfunction, while the effects of unilateral vestibular damage are more complex and subtle. Very recently, reports have emerged that sub-threshold, noisy galvanic vestibular stimulation can enhance memory in humans, although this has not been investigated for spatial memory as yet. These studies add to the increasing evidence that suggests a connection between vestibular sensory information and memory in humans.
History of the Vestibular System and Spatial Memory: Animal Studies
In the neurobiological literature, the vestibular system has had a long association with memory, in particular with spatial memory. Many early animal studies of spatial navigation suggested that non-visual, idiothetic cues such as vestibular and proprioceptive information, along with external, allocentric cues, were used by animals in order to remember their way through a familiar environment (Beritoff, 1965; Potegal et al., 1977; Etienne, 1980; Mittelstaedt and Mittelstaedt, 1980; Horn et al., 1981; Potegal, 1982; Miller et al., 1983; Etienne et al., 1988). Given the importance of the hippocampus for spatial memory, it was later speculated that vestibular information must be transmitted to the hippocampus, where it would be integrated with other sensory information relevant to spatial memory (Wiener and Berthoz, 1993; Berthoz, 1996; McNaughton et al., 1996; Etienne and Jeffery, 2004). The discovery that the activity of place cells in the hippocampus that respond to specific places in the environment was modulated by vestibular stimulation, added a potential mechanism to this hypothesis (Gavrilov et al., 1995; Wiener et al., 1995), which was supported by numerous animal behavioral studies showing that the disruption of normal vestibular function resulted in spatial memory deficits (Potegal et al., 1977; Horn et al., 1981; Potegal, 1982; Miller et al., 1983; Petrosini, 1984; Mathews et al., 1988, 1989; Semenov and Bures, 1989; Chapuis et al., 1992; Ossenkopp and Hargreaves, 1993; Stackman and Herbert, 2002; Wallace et al., 2002; Russell et al., 2003a; Zheng et al., 2003, 2006, 2007, 2008, 2009a,b; Baek et al., 2010).
Stackman et al. (2002) reported that reversible, bilateral inactivation of the vestibular labyrinth, using intratympanic tetrodotoxin, resulted in a loss of the selective firing of hippocampal place cells in alert rats, a result replicated by Russell et al. (2003b) using permanent bilateral surgical lesions of the labyrinth. Russell et al. (2006) also reported that bilateral labyrinthectomy caused a disruption of theta rhythm, which is believed to coordinate the activity of hippocampal place cells (Lever et al., 2010). Taken together, these various sources of experimental data from animal studies support the view that vestibular information is fundamentally important for the generation of spatial memories in animals and humans (Wiener and Berthoz, 1993; Berthoz, 1996; McNaughton et al., 1996, 2006; Etienne and Jeffery, 2004; Smith et al., 2005, 2009, 2010).
There is still a great deal of debate as to how vestibular information reaches the hippocampus. Electrical stimulation of one vestibular labyrinth or of the vestibular nucleus has been reported to evoke field potentials and single unit activity in the bilateral CA1 region of the hippocampus, albeit with a long latency (Horii et al., 1994, 1995, 1996, 2004; Cuthbert et al., 2000). Caloric or electrical stimulation of the human labyrinth has been shown to cause activation of the hippocampus (Vitte et al., 1996; De Waele et al., 2001) and glucose uptake is reduced in the hippocampus in patients with acute vestibular neuritis (Bense et al., 2004). The thalamus is certain to be one important relay station for at least some vestibular information; however, the number of different vestibulo-hippocampal pathways and their precise nature, remains to be determined (Smith, 1997; see Shinder and Taube, 2010 for a recent review). It must also be kept in mind that the hippocampus is only one part of a highly complex system of limbic-neocortical pathways that are responsible for spatial memory (Guldin and Grusser, 1998; Hanes and McCollum, 2006; Gu et al., 2007; Shinder and Taube, 2010).
One of the important limitations of the animal studies used to investigate vestibular disorders, is that chemical or surgical lesions of the vestibular labyrinth usually involve damage to the cochlea as well, and therefore it is possible that any cognitive effects of the lesions are partly due to hearing loss. For example, auditory stimulation, including noise trauma, has been reported to affect place cell function (Sakurai, 1990, 1994; Goble et al., 2009). For this reason, in our behavioral studies, sham control animals have their tympanic membranes removed so that sound is no longer transmitted effectively to the malleus, incus and stapes. This can serve only as a partial auditory control, however, because some sound will still be transmitted to the cochlea. Nonetheless, we have consistently found that rats without vestibular lesions but with the tympanic membrane removed have performed significantly better in cognitive tasks than animals with vestibular lesions (Zheng et al., 2006, 2007, 2008, 2009a,b; Baek et al., 2010). This suggests that hearing loss is not the major cause of the spatial memory deficits in animals subjected to bilateral vestibular lesions. In addition, animal studies, using different kinds of aminoglycosides (i.e., streptomycin and neomycin) with different toxicities for the auditory and vestibular hair cells, have shown that the effects of auditory and vestibular lesions on learning and memory are different (Schaeppi et al., 1991). In a radial arm maze task, rats treated with streptomycin, which lesioned the auditory and the vestibular systems, exhibited impaired working memory; however, rats treated with neomycin, which lesioned only the auditory system, did not (Schaeppi et al., 1991). In recent studies, we have also found that rats subjected to acoustic trauma, which causes elevated auditory brainstem response thresholds and tinnitus, do not exhibit deficits in an alternating T maze or a five choice serial reaction time task that are similar to rats with vestibular lesions (Darlington et al., 2010).
Most animal studies of bilateral vestibular loss have been conducted at time points long after the original lesion and the spatial memory deficits still persist (Zheng et al., 2006, 2007, 2008, 2009a,b). In the most recent study, rats that were 14 months post-lesion were more severely impaired in a spatial memory foraging task than at 5 months post-op. (Baek et al., 2010; see Figure 1). Furthermore, the spatial memory deficits exhibited by bilateral vestibular deafferentation (BVD) rats manifested differently in different kinds of tasks and with different lesions. While oscillopsia, the blurring of the visual world due to a deficient or absent vestibulo-ocular reflex, is an obvious explanation for poor performance in cognitive tasks, there was no obvious relationship to the availability of visual cues that would allow for oscillopsia. For example, at 5–6 months post-op., rats with bilateral vestibular loss had only minimally impaired performance in a foraging task in light, but their performance deteriorated substantially in darkness (Zheng et al., 2009b). However, at 14 months post-op., rats with the same lesions were impaired in both light and darkness (Baek et al., 2010). By contrast, animals with unilateral vestibular loss showed deficits in darkness at 3 months following the lesion, but performed at levels similar to sham controls at 6 months post-op. (Zheng et al., 2006). In tasks such as the spatial alternation in a T maze in light, rats with bilateral vestibular loss exhibited some improvement in performance over time, but at 5 months post-op. their percentage of correct responses was still significantly less than normal (Zheng et al., 2007). At 6 weeks post-op., rats with bilateral vestibular lesions performed significantly worse in a radial arm maze task even in light (Russell et al., 2003). These results show that vestibular lesions have different effects in different cognitive tasks and that the deficits observed are more likely to be due to a reduced cognitive ability than simply reflex dysfunction. Furthermore, most studies of rats with bilateral vestibular lesions indicate that they are hyperactive rather than hypoactive (Russell, et al., 2003; Goddard et al., 2008; Zheng et al., 2008, 2009; Baek et al., 2010).
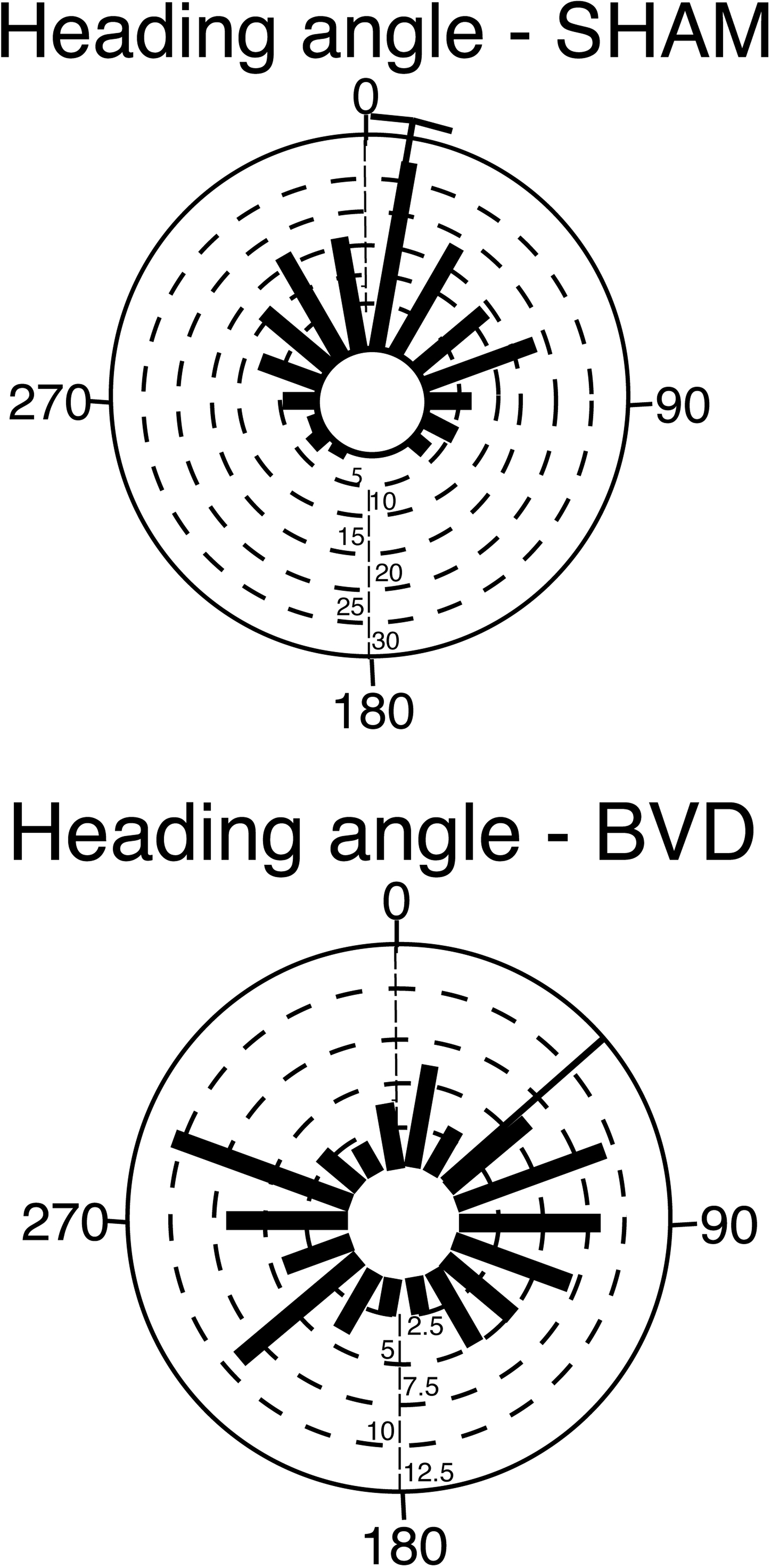
Figure 1. Rose plots of the heading angle of rats subjected to bilateral vestibular deafferentation (BVD) or sham surgery at 14 months post-op. The plots represent the animal’s direction on the homeward route in a foraging task in which they had to remember the location of a home base in darkness. In the plots, 0° represents the correct direction or heading angle. Each blue bar represents one animal and the black bars represent the mean heading angles for the group with a 95% confidence interval (for the sham group). Notice that the BVD animals’ heading angles were distributed equally around 360° and that, as a result, no 95% confidence interval could be calculated. Modified from Baek et al. (2010).
While most animal studies of bilateral vestibular loss have used some form of memory task that has a spatial component (e.g., the foraging task, radial arm maze, alternating T maze), some studies have also reported deficits in attention (Zheng et al.,2009a) and in object recognition memory that has no spatial component (Zheng et al., 2004). In a five choice serial reaction time task, which is a task commonly used to assess attention performance, rats with bilateral vestibular lesions made significantly fewer correct responses, significantly more incorrect responses, with no more omissions, and responded with a reduced latency, compared to sham controls (Zheng et al.,2009a).
The Effects of Vestibular Dysfunction on Cognition in Human Patients
The first clinical evidence that vestibular loss might affect cognition in human patients was reported by Grimm et al. (1989). They described patients with a perilymph fistular syndrome, who reported symptoms such as positional vertigo but also a variety of psychological symptoms, including memory and attention deficits. They studied a total sample of 102 patients, and more than 85% of them reported memory loss. The patients had a normal level of intellectual function; however, their performance on digit symbol, block design, paired associate learning and picture arrangement tasks, was impaired.
Spatial Memory
Following the study by Grimm et al. (1989), several studies in the 1990s examined the effects of vestibular damage on spatial navigation in humans. They showed that patients with vestibular disorders exhibited deficits in path navigation (Peruch et al., 1999; Cohen, 2000; Cohen and Kimball, 2002; Borel et al., 2004). However, because the tasks involved movement, it could be argued that the deficits resulted from a complex interaction between cognition and postural control. Other studies examined cognitive performance more directly.
In what was perhaps the most direct study of spatial memory, Schautzer et al. (2003) and Brandt et al. (2005) examined performance using a spatial memory test, the virtual Morris water maze, in 10 patients with bilateral vestibular loss and age-, sex- and education-matched controls. The task involved moving only a cursor on a computer screen, using a mouse. The patients had received bilateral vestibular neurectomies 5–10 years previously for the treatment of neurofibromatosis type 2. Importantly, only one patient had a total postoperative hearing loss. They found that the patients with bilateral vestibular loss showed impaired performance in the task when they had to remember a navigation path to a previously visible target; however, they showed no deficit when the target was visible, therefore the effect was specific to memory. Brandt et al. used the Wechsler memory test to show that the patients had normal or above normal non-spatial memory performance. Compared to the controls, the patients also exhibited a significant decrease in the volume of the hippocampus (16.9%). This effect was bilateral, specific to the hippocampus and there was no significant reduction in the total brain volume or in the volume of gray matter or white matter, only a significant increase in cerebrospinal fluid volume.
In a study of patients with unilateral vestibular deafferentation (UVD), Hufner et al. (2007) reported that the results from the virtual Morris water maze task were more complex. During place learning, males with a right UVD and females with a left UVD exhibited a significantly smaller decrease in heading error over the repeated trials compared to the control and other UVD groups. In addition, inspection of the swim paths showed that only 2 out of the 8 right UVD patients used direct paths to the platform, compared to 12 out of the 16 control subjects, a difference that was statistically significant. In the probe trial, the right UVD patients were found to have a greater heading error than the left UVD patients or control subjects. Finally, in the cued navigation, females with left UVD performed worse than the other groups in the first visible platform trial. By contrast with the study of Brandt et al. (2005) of patients with BVD, Hufner et al. (2007) found no significant differences in the hippocampal volume of UVD patients compared to controls. In a later study, Hufner et al. (2009) reported some subtle deficits in spatial memory in patients with UVD as well as an atrophy of the ipsilateral supramarginal nucleus, the postcentral and superior temporal gyrus and the MT/V5 area, as well as the contralateral thalamus and tegmentum of the mesencephalon.
By contrast, zu Eulenburg et al. (2010) have recently reported that patients who had recovered from left- or right-sided unilateral vestibular neuritis exhibited a significant decrease in the volume of the left posterior hippocampus, compared to healthy controls.
In support of the notion that the human hippocampus may increase or decrease in volume depending upon behavioral experience, Hufner et al. (2010, in press) have also reported that professional dancers and slackliners showed an increase in the volume of the posterior hippocampus and a relative atrophy of the anterior hippocampus, relative to controls. There was a negative correlation between the right anterior hippocampal volume and the degree of experience of the dancers and slackliners and a positive correlation between the right posterior hippocampal volume and their degree of experience. However, there were no significant differences in either general memory or spatial memory. To what extent the changes in hippocampal volume reflect differences in vestibular information processing as opposed to other sensory experience, is unclear. It is also important to consider the possible effect of sensory stimulation in general, on hippocampal structure. For example, it is well known that exercise and environmental stimulation can alter neurogenesis in the hippocampus via neuromodulators such as brain-derived neurotrophic factor (BDNF) (e.g., Olson et al., 2006; Clark et al., 2008; Lou et al., 2008).
As with the animal studies, it is obvious to think that any memory impairment following vestibular damage might simply be a result of vestibular reflex deficits, for example oscillopsia or the inability to move properly as a consequence of impaired vestibulo-spinal reflexes (Gizzi et al., 2003). However, studies such as those by Schautzer et al. (2003) and Brandt et al. (2005) were conducted 5–10 years following bilateral vestibular neurectomy. Although it is clear that the patients would never have recovered normal vestibulo-ocular reflex and vestibulo-spinal reflex function, by this time they would have compensated for the severe acute symptoms (Smith and Curthoys, 1989; Curthoys and Halmagyi, 1995).
Given the severity of some vestibular disorders such as Ménière’s disease, it is possible that cognitive dysfunction is an indirect consequence of symptoms such as vertigo. However, studies of patients with chronic vestibular loss without vertigo, have reported that the patients exhibit spatial memory impairment (Guidetti et al., 2008). Overall, there is increasing and convincing evidence that patients with vestibular disorders suffer from spatial memory problems (see Smith et al., 2005; Hanes and McCollum, 2006 for reviews).
Non-Spatial Memory and other Cognitive Symptoms
It is not clear whether the cognitive deficits associated with vestibular dysfunction are limited to spatial memory impairment. While some human studies have reported that other aspects of memory, attention and general intelligence are normal (Schautzer et al., 2003; Brandt et al., 2005), others have reported deficits in attention and concentration (Sang et al., 2006; Jauregui-Renaud et al., 2008a,b) and even dyscalculia (Risey and Briner, 1990; Yardley et al., 2002; Andersson et al., 2002, 2003). Black et al. (2004) found that general memory problems were common in patients with vestibular loss due to gentamicin ototoxicity. Jauregui-Renaud et al. (2008a,b)have published a number of studies reporting that patients with vestibular disorders have high rates of depersonalization/derealization symptoms, which include difficulty focussing attention and thoughts seeming blurred (Sang et al., 2006; Cheyne and Girard, 2009). Other studies have shown that as postural tasks become more challenging, patients with vestibular disorders exhibit deficits in reaction time and memory (Yardley et al., 2001, 2002; Redfern et al., 2004). In a study by Redfern et al. (2004), patients with unilateral vestibular loss showed large increases in complex and inhibitory reaction time, even while seated. A similar result was reported by Talkowski et al. (2005).
It is possible and even probable that the association between vestibular dysfunction and anxiety/depression has some connection with the observed cognitive deficits that accompany vestibular disorders (Balaban and Thayer, 2001; Balaban, 2002; Staab and Ruckenstein, 2003; Staab, 2006). There seems to be a complex, two-way interaction between vestibular function and affective state, which is also mediated in part by hippocampal function, therefore it is difficult to separate the cognitive from the emotional effects of vestibular loss. Vestibular impairment may cause a multitude of changes in cognition, emotion and personality, which are difficult if not impossible to disentangle.
Noisy Galvanic Vestibular Stimulation and Memory in Humans
The evidence that vestibular loss in humans can give rise to memory deficits, raises the interesting question of whether activating the vestibular system could enhance memory? At first, this possibility appears paradoxical. The theory behind the effects of vestibular loss on spatial memory is that during movement, the hippocampus uses vestibular information in order to form memories concerning the spatial environment (Wiener and Berthoz, 1993; Berthoz, 1996; McNaughton et al., 1996; Etienne and Jeffery, 2004; Smith et al., 2005, 2009, 2010; McNaughton et al., 2006). Activating the vestibular system in the absence of self-motion would seem, if anything, to be likely to confuse the brain regarding spatial memory.
Galvanic vestibular stimulation (GVS) involves stimulation of the vestibular system by means of small galvanic (DC) currents, often passed transcutaneously through the mastoid processes to the vestibular system (Goldberg et al., 1984; Kim and Curthoys, 2004; see Utz et al., 2010 in press for a recent review). Neurophysiological studies involving single neuron recordings from the vestibular nerve have confirmed that GVS directly affects the discharge of Scarpa’s ganglion neurons in the vestibular nerve (Goldberg et al., 1984; Courjon et al., 1987; Kim and Curthoys, 2004; Kim, 2009). GVS modulates the spontaneous firing of vestibular afferents in a manner which either increases (cathodal stimulation) or decreases (anodal stimulation) the afferents’ firing frequency (Lowenstein, 1955; Goldberg et al., 1984; Courjon et al., 1987; Kim and Curthoys, 2004; Kim, 2009). Although it is commonly believed that cathodal GVS works by activating the spike trigger site of the vestibular nerve (Goldberg et al., 1984), the fact that it does not elicit a vestibulo-ocular reflex in patients with gentamicin vestibulotoxicity, in whom the vestibular hair cells are missing but the vestibular nerve is intact, suggests that it may work via the hair cells themselves (Aw et al., 2008).
In humans, functional magnetic resonance imaging (fMRI) has revealed that areas of significant activation by GVS include the posterior insula, the retroinsular regions, the superior temporal gyrus, parts of the inferior parietal lobule, the intraparietal sulcus, the post-central and pre-central gyrus, the anterior insular, the inferior frontal gyrus, the anterior cingulate gyrus, the precuneus and the hippocampus (Lobel et al., 1998; see Karnath and Dieterich, 2006 for a review). Activation of cortical networks during GVS is not symmetrical; it is stronger in the non-dominant hemisphere, in the hemisphere ipsilateral to the stimulated ear, and in the hemisphere ipsilateral to the fast phase of vestibular nystagmus (see Karnath and Dieterich, 2006 for a review). The overlaying of a noise signal on a galvanic stimulus, termed “noisy GVS”, is a concept based upon the principle of stochastic resonance, in which a sub-threshold sensory stimulus can be made to exceed a fixed threshold if a Gaussian noise signal, with a frequency much higher than the sub-threshold stimulus, is superimposed upon it (Moss et al., 2004). It has been shown in the visual, auditory and tactile sensory systems that stochastic resonance increases the ability of the CNS to extract signals (see Moss et al., 2004 for a review). Soma et al. (2003) suggested the hypothesis that 1/f noise might optimize the brain’s responsiveness, since, when coupled with GVS, it was more effective at inducing the baroreflex at lower intensities than white noise (Soma et al., 2003).
A small literature has emerged on the use of low amplitude, noisy GVS to enhance memory in humans. This work is related to a number of intriguing studies in human patients in which caloric vestibular stimulation (CVS) and noisy GVS have been used to treat patients with various neurological disorders, ranging from prosopagnosia (Wilkinson et al., 2005), hemi-spatial neglect (Rorsman et al., 1999), akinesia (Pan et al., 2008) and autonomic dysfunction (Yamamoto et al., 2005), to neuropathic pain (Ramachandran et al., 2007a,b;McGeoch and Ramachandran, 2008; McGeoch et al., 2008, 2009). In one of the earliest studies, Rorsman et al. (1999) demonstrated that subliminal GVS could reduce visuo-spatial neglect in patients with stroke.
Batchtold et al. (2001) first reported that CVS could improve verbal and spatial memory in humans, and the effect was greater for right ear irrigation. However, CVS induces vestibular reflexes and therefore it is difficult to separate those effects from cognitive performance. Wilkinson et al. (2008) investigated whether noisy, low intensity, bipolar GVS, which was sub-threshold for the activation of the vestibular reflexes, would affect memory for faces. Wilkinson et al. (2008) found that the mean reaction time was shorter for the GVS groups compared to the sham controls, but that the largest reduction was for the anode on the left ear and the cathode on the right ear. They concluded that GVS can improve memory for faces, perhaps by increasing blood flow to the right temporal and parietal cortices. This effect of GVS on memory was unlikely to be due to non-specific arousal since the electrical stimulation was sub-threshold, the memory improvement was greater for the anode on the left, and improvement was found only when a noise signal was added to the GVS. Wilkinson et al. (2005)have also found that noisy GVS can attenuate prosopagnosia and figure copying deficits (Wilkinson et al., 2010).
In other studies, air puffs have been applied to the ear as a treatment for Ménière’s disease (Densert and Sass, 2001). It would be interesting to investigate whether this form of stimulation has any effect on memory.
At present, there is very little evidence relating to how noisy GVS affects memory in humans. It is not clear whether the effect has any relationship to the effects of vestibular lesions on spatial memory. One possibility is that any beneficial effect of GVS is merely a result of the fact that vestibular information reaches many regions of the neocortex (Bense et al., 2001) and that GVS will probably cause a change in the integration of sensory information (Wilkinson et al., 2008). However, there are other possibilities. Given the importance of vestibular information to spatial memory, even sub-threshold GVS may somehow “prime” the brain to process and store new information that may be important for survival. In this respect it is important to recognize the evolutionary age of the vestibular system, and the possibility that vestibular input into multiple brain circuits has evolved so that all sensory processing and motor planning is carried out within the framework of the perception of gravitational vertical, especially given the demands of bipedalism (Indovina et al., 2005; Skoyles, 2006; Smith et al., 2010).
Future Directions
The studies linking vestibular disorders with cognitive impairment in humans that have been conducted to date, suggest that various types of dysfunction of the vestibular system can lead to memory deficits and perhaps the most striking, are deficits in spatial memory following bilateral vestibular loss (Brandt et al., 2005). On the other hand, studies are emerging that suggest that specific types of sub-threshold, noisy GVS may enhance some kinds of memory, perhaps through the mechanism of stochastic resonance. Whether these two lines of evidence are directly related remains to be seen, and one interesting question is whether noisy GVS could be used to resolve spatial memory deficits in cases where the vestibular nerves are still intact. Some progress has been made in understanding the mechanisms by which vestibular damage results in spatial memory deficits, in terms of the disruption of hippocampal place cell firing (Stackman et al., 2002; Russell et al., 2003) and theta rhythm (Russell et al., 2006). However, our understanding of precisely how the hippocampus might use vestibular information and through which pathways, remains incomplete. By contrast, we know very little about why sub-threshold, noisy GVS might enhance memory. Whether it has specific effects on areas of the brain involved in memory remains to be determined. It will be important for animal studies to investigate this using neuronal recording from limbic and neocortical regions. Nonetheless, the current literature relating to memory, vestibular lesions and noisy GVS, supports the view that memory and the vestibular system are inextricably linked.
Conflict of Interest Statement
The authors declare that the research was conducted in the absence of any commercial or financial relationships that could be construed as a potential conflict of interest.
Acknowledgments
This research was supported by a grant from the Marsden Fund of the Royal Society of New Zealand, to PS, YZ and CD. LG was supported by a Post-Doctoral Fellowship from the Marsden Fund. JB was supported by a University of Otago PhD Scholarship.
References
Andersson, G., Hagman, J., Talianzadeh, R., Svedberg, A., and Larsen, H. C. (2002). Effects of cognitive load on postural control. Brain Res. Bull. 58, 135–139.
Andersson, G., Hagman, J., Talianzadeh, R., Svedberg, A., and Larsen, H. C. (2003). Dual task study of cognitive and postural interference in patients with vestibular disorders. Otol. Neurotol. 24, 289–293.
Aw, S. T., Todd, M. J., Aw, G. E., Weber, K. P., and Halmagyi, G. M. (2008). Gentamicin vestibulotoxicity impairs human electrically evoked vestibulo-ocular reflex. Neurology 71, 1776–1782.
Baek, J.-H., Zheng, Y., Darlington, C. L., and Smith, P. F. (2010). Evidence that spatial memory deficits in rats following bilateral vestibular loss are probably permanent. Neurobiol. Learn. Mem. 94, 402–413.
Balaban, C. D. (2002). Neural substrates linking balance control and anxiety. Physiol. Behav. 77, 469–475.
Balaban, C. D., and Thayer, J. F. (2001). Neurological bases for balance-anxiety links. J. Anxiety Dis. 15, 53–79.
Batchtold, D., Baumann, T., Sandor, P. S., Kritos, M., Regard, M., and Brugger, P. (2001). Spatial- and verbal-memory improvement by cold water caloric stimulation in healthy subjects. Exp. Brain Res. 136, 128–132.
Bense, S., Bartenstein, P., Lochmann, M., Schlindwein, P., Brandt, T., and Dieterich, M. (2004). Metabolic changes in the vestibular and visual cortices in acute vestibular neuritis. Ann. Neurol. 56, 624–630.
Bense, S., Stephan, T., Yousry, T. A., Brandt, T., and Dieterich, M. (2001). Multisensory cortical signal increases and decreases during vestibular galvanic stimulation (fMRI). J. Neurophysiol. 85, 886–899.
Berthoz, A. (1996) “How does the cerebral cortex process and utilize vestibular signals?” in Disorders of the Vestibular System, eds R. Baloh and G. M. Halmagyi (New York-Oxford: Oxford University Press), 113–125.
Black, F. O., Pesznecker, S., and Stallings, V. (2004). Permanent gentamicin vestibulotoxicity. Otol. Neurotol. 25, 559–569.
Borel, L., Harlay, F., Loopez, C., Magnan, J., Chays, A., and Lacour, M. (2004). Walking performance in vestibular-defective patients before and after unilateral vestibular neurrectomy. Behav. Brain Res. 150, 191–200.
Brandt, T., Schautzer, F., Hamilton, D. A., Bruning, R., Markowitsch, H., Kalla, R., Darlington, C. L., Smith, P. F., and Strupp, M. (2005). Vestibular loss causes hippocampal atrophy and impaired spatial memory in humans. Brain 128, 2732–2741.
Chapuis, N., Krimm, M., de Waele, C., Vibert, N., and Berthoz, A. (1992). Effect of post-training unilateral labyrinthectomy in a spatial orientation task by guinea pigs. Behav. Brain Res. 51, 115–126.
Cheyne, J. A., and Girard, T. A. (2009). The body unbound: vestibular-motor hallucinations and out-of-body experiences. Cortex 45, 201–215.
Clark, P. J., Brzezinska, W. J., Thomas, M. W., Ryzhenko, N. A., Toshkov, S. A., and Rhodes, J. S. (2008). Intact neurogenesis is required for benefits of exercise on spatial memory but not motor performance or contextual fear conditioning in C57BL/6J mice. Neuroscience 155, 1048–1058.
Cohen, H. S. (2000). Vestibular disorders and impaired path integration along a linear trajectory. J. Vestib. Res. 10, 7–15.
Cohen, H. S., and Kimball, K. T. (2002). Improvements in path integration after vestibular rehabilitation. J. Vestib. Res. 12, 47–51.
Courjon, J. H., Precht, W., and Sirkin, D. W. (1987). Vestibular nerve and nuclei unit responses and eye movement responses to repetitive galvanic stimulation of the labyrinth in the rat. Exp. Brain Res. 66, 41–48.
Curthoys, I. S, and Halmagyi, G. M. (1995). Vestibular compensation: a review of the ocular motor, neural and clinical consequences of unilateral vestibular loss. J. Vestib. Res. 5, 67–107.
Cuthbert, P. C., Gilchrist, D. P., Hicks, S. L., MacDougall, H. G., and Curthoys, I. S. (2000). Electrophysiological evidence for vestibular activation of the guinea pig hippocampus. Neuroreport 11, 1443–1447.
Darlington, C. L., Hamilton, E., Stiles, L., Smith, P. F., and Zheng, Y. (2010). Effects of tinnitus induced by acoustic trauma on performance in a spatial forced alternating T maze task. J. Vestib. Res. 20, 176.
Densert, B., and Sass, K. (2001). Control of symptoms in patients with Ménière’s disease using middle ear pressure applications: two years follow-up. Acta Otolaryngol. 121, 616–621.
De Waele, C., Baudonniere, P. M., Lepecq, J. C., Tran Ba Huy, P., and Vidal, P. P. (2001). Vestibular projections in the human cortex. Exp. Brain Res. 141, 541–551.
Etienne, A. S. (1980). The orientation of the golden hamster to its nest-site after the elimination of various sensory cues. Experientia 36, 1048–1050.
Etienne, A. S., Maurer, R., and Saucy, F. (1988). Limitations in the assessment of path dependent information. Behaviour 106, 81–111.
Gavrilov, V. V., Wiener, S. I., and Berthoz, A. (1995). Enhanced hippocampal theta EEG during whole body rotations in awake restrained rats. Neurosci. Lett. 197, 239–241.
Gizzi, M., Zlotnick, M., Cicerone, K., and Riley, E. (2003). Vestibular disease and cognitive dysfunction: no evidence for a causal connection. J. Head Trauma Rehabil. 18, 398–407.
Goble, T. J, Møller, A. R., and Thompson, L. T. (2009). Acute high-intensity sound exposure alters responses of place cells in hippocampus. Hear. Res. 253(1-2), 52–59.
Goddard, M. Y., Zheng, C. L., Darlington, and Smith, P. F. (2008). Locomotor and exploratory behaviour in the rat following bilateral vestibular deafferentation. Behav. Neurosci. 122, 448–459.
Goldberg, J. M., Smith, C. E., and Fernandez, C. (1984). Relation between discharge regularity and responses to externally applied galvanic currents in vestibular nerve afferents of the squirrel monkey. J. Neurophysiol. 51, 1236–1256.
Grimm, R. J., Hemenway, W. G., Lebray, P. R., and Black, F. O. (1989). The perilymph fistula syndrome defined in mild head trauma. Acta Otolaryngol. Suppl. 464, 1–40.
Gu, Y., DeAngelis, G. C., and Angelaki, D. E. (2007). A functional link between area MSTd and heading perception based on vestibular signals. Nat. Neurosci. 10, 1038–1047.
Guidetti, G., Monzani, D., Trebbi, M., and Rovatti, V. (2008). Impaired navigation skills with psychological distress and chronic peripheral vestibular hypofunction without vertigo. Acta Otorhinolarngol. Ital. 28, 21–25.
Guldin, W. O., and Grusser, O. J. (1998). Is there a vestibular cortex? Trends Neurosci. 21, 254–259.
Hanes, D. A., and McCollum, G. (2006). Cognitive-vestibular interactions: a review of patient difficulties and possible mechanisms. J. Vestib. Res. 16, 75–91.
Horii, A., Russell, N., Smith, P. F., Darlington, C. L., and Bilkey, D. K. (2004). Vestibular influences on CA1 neurons in the rat hippocampus: an electrophysiological study in vivo. Exp. Brain Res. 155, 245–250.
Horii, A., Takeda, N., Mochizuki, T., Okakura-Mochizuki, K., Yamamoto, Y., and Yamatodani, A. (1994). Effects of vestibular stimulation on acetylcholine release from rat hippocampus: an in vivo microdialysis study. J. Neurophysiol. 72, 605–611.
Horii, A., Takeda, N., Mochizuki, T., Okakura-Mochizuki, K., Yamamoto, Y., Yamatodani, A., and Kubo, T. (1995). Vestibular modulation of the septo-hippocampal cholinergic system of rats. Acta Otolaryngol. 520, 395–398.
Horii, A., Takeda, N., Yamatodani, T. A., and Kubo, T. (1996). Vestibular influences on the histaminergic and cholinergic systems in the rat brain. Ann. N. Y. Acad. Sci. 781, 633–634.
Horn, K. M., DeWitt, J. R., and Nielson, H. C. (1981). Behavioral assessment of sodium arsanilate induced vestibular dysfunction in rats. Physiol. Psychol. 9, 371–378.
Hufner, K., Binetti, C., Hamilton, D. A., Stephan, T., Flanagin, V. L., Linn, J., Labudda, K., Markowitsch, H. J., Glasauer, S., Jahn, K., Strupp, M., and Brandt, T. (2010) Structural and functional plasticity of the hippocampal formation in professional dancers and slackliners. Hippocampus. (inpress)
Hufner, K., Hamilton, D. A., Kalla, R., Stephan, T., Glasauer, S., Ma, J., Bruning, R., Markowitsch, H. J., Labudda, K., Scichor, C., Strupp, M., and Brandt, T. (2007). Spatial memory and hippocampal volume in humans with unilateral vestibular deafferentation. Hippocampus 17, 471–485.
Hüfner, K., Stephan, T., Hamilton, D. A., Kalla, R., Glasauer, S., Strupp, M., and Brandt, T. (2009). Gray-matter atrophy after chronic complete unilateral vestibular deafferentation. Ann. N. Y. Acad. Sci. 1164, 350–352.
Indovina, I., Maffei,V., Bosco, G., Zago, M., Macaluso, E., and Lacquaniti, F. (2005). Representation of visual gravitational vertical in the human vestibular cortex. Science 308, 416–419.
Jauregui-Renaud, K., Ramos-Toledo, V., Aguilar-Bolanos, M., Montano-Velazquez, B., and Pliego-Maldonado, A. (2008a). Symptoms of detachment from the self or from the environment in patients with an acquired deficiency of the special senses. J. Vestib. Res. 18, 129–137.
Jauregui-Renaud, K., Sang, F. Y., Gresty, M. A., Green, D. A, and Bronstein, A. M. (2008b). Depersonalisation/derealisation symptoms and updating orientation in patients with vestibular disease. J. Neurol. Neurosurg. Psychiatr. 79, 276–283.
Kim, J. (2009). Short-term habituation of eye-movement responses induced by galvanic vestibular stimulation (GVS) in the alert guinea pig. Brain Res. Bull. 79, 1–5.
Kim, J., and Curthoys, I. S. (2004). Responses of primary vestibular neurons to galvanic vestibular stimulation (GVS) in the anaesthetised guinea pig. Brain Res. Bull. 64, 265–271.
Lever, C., Burton, S., Jeewajee, A., Wills, T. J., Cacucci, F., Burgess, N., and O’Keefe, J. (2010). Environmental novelty elicits a later theta phase of firing in CA1 but not subiculum. Hippocampus 20, 229–234.
Lobel, E., Kleine, J. F., Bihan, D. L., Leroy-Willig, A., and Berthoz, A. (1998). Functional MRI of galvanic vestibular stimulation. J. Neurophysiol. 80, 2699–2709.
Lou, S. J., Liu, J. Y., Chang, H., and Chen, P. J. (2008). Hippocampal neurogenesis and gene expression depend on exercise intensity in juvenile rats. Brain Res. 1210, 48–55.
Lowenstein, O. (1955). The effect of galvanic polarization on the impulse discharge from sense endings in the isolated labyrinth of the thornback ray (Raja clavata). J. Physiol. 127, 104–117.
Mathews, B. L., Campbell, K. A., and Deadwyler, S. A. (1988). Rotational stimulation disrupts spatial learning in fornix-lesioned rats. Behav. Neurosci. 102, 35–42.
Mathews, B. L., Ryu, J. H., and Bockaneck, C. (1989). Vestibular contribution to spatial orientation. Evidence of vestibular navigation in an animal model. Acta Otolaryngol. 468, 149–154.
McGeoch, P. D., and Ramachandran, V. S. (2008). Vestibular stimulation can relieve central pain of spinal origin. Spinal Cord 46, 756–757.
McGeoch, P. D., Williams, L. E., Song, T., Lee, R. R., Huang, M., and Ramachandran, V. S. (2008). in press. Post-stroke tactile allodynia and its modulation by vestibular stimulation: a MEG case study. Acta Neurol. Scand. 119, 404–409.
McGeoch, P. D., Williams, L. E., Song, T., Lee, R. R., Huang, M., and Ramachandran, V. S. (2009). Post-stroke tactile allodynia and its modulation by vestibular stimulation: a MEG case study. Acta Neurol. Scand. 119, 404–409.
McGeoch, P. D., Williams, L. E., Song, T., Lee, R. R., and Ramachandran, V. S. (2008). Behavioural evidence for vestibular stimulation as a treatment for post-stroke pain. J. Neurol. Neurosurg. Psychiatr. 79, 1298–1301.
McNaughton, B., Battaglia, F. P., Jensen, O., Moser, E. I., and Moser, M. B. (2006). Path integration and the neural basis for the “cognitive map”. Nat. Rev. Neurosci. 7, 663–678.
McNaughton, B. L., Barnes, C. A., Gerard, J. L., Gothard, K., Jung, M. W., Knierim, J. J., Kudrimoti, H., Qin, Y., Skaggs, W. E., Suster, M., and Weaver, K. L. (1996). Deciphering the hippocampal polyglot: the hippocampus as a path integration system. J. Exp. Biol. 199, 173–185.
Miller, S. M. Potegal, L, and Abraham, L. (1983). Vestibular involvement in a passive transport and return task. Physiol. Psychol. 11, 1–10.
Mittelstaedt, M. L., and Mittelstaedt, H. (1980). Homing by path integration in a mammal. Naturwissenschaften. 67, 556–567.
Moss, F., Ward, L. M., and Sannita, W. G. (2004). Stochastic resonance and sensory information processing: a tutorial and review of application. Clin. Neurophysiol. 115, 267–281.
Olson, A. K., Eadie, B. D., Ernst, C., and Christie, B. R. (2006). Environmental enrichment and voluntary exercise massively increase neurogenesis in the adult hippocampus via dissociable pathways. Hippocampus 16, 250–160.
Ossenkopp, K. P., and. Hargreaves, E. L. (1993). Spatial learning in an enclosed eight-arm maze in rats with sodium arsinilate-induced labyrinthectomies. Behav. Neural. Biol. 59, 253–257.
Pan, W., Soma, R., Kwak, S., and Yamamoto, Y. (2008). Improvement of motor functions by noisy vestibular stimulation in central neurodegenerative disorders. J. Neurol. 255, 1657–1661.
Peruch, P., Borel, L., Gaunet, F., Thinus-Blanc, G., Magnan, J., and Lacour, M. (1999). Spatial performance of unilateral vestibular defective patients in nonvisual versus visual navigation. J. Vestib. Res. 9, 37–47.
Petrosini, L. (1984). Task-dependent rate of recovery from hemilabyrinthectomy: an analysis of swimming and locomotor performances. Physiol. Behav. 33, 799–804.
Potegal, M. (1982) “Vestibular and neostriatal contributions to spatial orientation.” in Spatial Abilities: Development and Physiological Foundation, ed. M. Potegal (New York: Academic Press), 361–387.
Potegal, M., Day, M. J., and Abraham, L. (1977). Maze orientation, visual and vestibular cues in two-maze spontaneous alternation of rats. Physiol. Psychol. 5, 414–420.
Ramachandran, V. S., McGeoch, P. D., and Williams, L. (2007a) Can vestibular caloric stimulation be used to treat Dejerine–Roussy Syndrome? Med. Hypotheses 69, 486–488.
Ramachandran, V. S., McGeoch, P. D., Williams, L., and Arcilla, G. (2007b). Rapid relief of thalamic pain syndrome induced by vestibular caloric vestibular stimulation. Neurocase 13, 185–188.
Redfern, M. S., Talkowski, M. E., Jennings, J. R., and Furman, J. M. (2004). Cognitive influences in postural control of patients with unilateral vestibular loss. Gait Posture 19, 105–114.
Risey, J., and Briner, W. (1990-1991) Dyscalculia in patients with vertigo. J. Vestib. Res. 1, 31–37.
Rorsman, L., Magnusson, M., and Johansson, B. B. (1999) Reduction of visuospatial neglect with vestibular galvanic stimulation. Scand. J. Rehabil. Med. 31, 117–124.
Russell, N., Horii, A., Smith, P. F., Darlington, C. L., and Bilkey, D. (2003). Effects of bilateral vestibular deafferentation on radial arm maze performance. J. Vestib. Res. 13, 9–16.
Russell, N., Horii, A., Smith, P. F., Darlington, C. L., and Bilkey, D. (2003). The long-term effects of permanent vestibular lesions on hippocampal spatial firing. J. Neurosci. 23, 6490–6498.
Russell, N., Horii, A., Smith, P. F., Darlington, C. L., and Bilkey, D. (2006). Lesions of the vestibular system disrupt hippocampal theta rhythm in the rat. J. Neurophysiol. 96, 4–14.
Sakurai, Y. (1990). Hippocampal cells have behavioral correlates during the performance of an auditory working memory task in the rat. Behav. Neurosci. 104, 253–263.
Sakurai, Y. (1994). Involvement of auditory cortical and hippocampal neurons in auditory working memory and reference memory in the rat. J. Neurosci. 14, 2606–2623.
Sang, F. Y., Jauregui-Renaud, K., Green, D. A., Bronstein, D. A., and Gresty, M. A. (2006). Depersonalisation/derealisation symptoms in vestibular disease. J. Neurol. Neurosurg. Psychiatry 77, 760–766.
Schaeppi, U. G., Krinke, R. E., FitzGerald, and Classen, W. (1991). Impaired tunnel-maze behavior in rats with sensory lesions: vestibular and auditory systems. Neurotoxicology 12, 445–454.
Schautzer, F., Hamilton, D., Kalla, R., Strupp, M., and Brandt, T. (2003). Spatial memory deficits in patients with chronic bilateral vestibular failure. Ann. N. Y. Acad. Sci. 1004, 316–324.
Semenov, L. V., and Bures, J. (1989). Vestibular stimulation disrupts acquisition of place navigation in the Morris water tank task. Behav. Neural Biol. 51, 346–363.
Shinder, M. E., and Taube, J. S. (2010). Differentiating ascending vestibular pathways to the cortex involved in spatial cognition. J. Vestib. Res. 20, 3–23.
Skoyles, J. R. (2006) Human balance, the evolution of bipedalism and disequilibrium syndrome. Med. Hypothses 66, 1060–1068.
Smith, P. F., and Curthoys, I. S. (1989). Mechanisms of recovery following peripheral vestibular lesions: a review. Brain Res. Rev. 14, 155–180.
Smith, P. F., Brandt, T., Strupp, M., Darlington, C. L., and Zheng, Y. (2009). Balance before reason in rats and humans. Ann. N. Y. Acad. Sci. 1164, 127–133.
Smith, P. F., Darlington, C. L., and Zheng, Y. (2010). Move it or lose it: is stimulation of the vestibular system necessary for normal spatial memory? Hippocampus. 20, 36–43.
Smith, P. F., Horii, A., Russell, N., Bilkey, D., Zheng, Y., Liu, P., Kerr, D. S., and Darlington, C. L. (2005). The effects of vestibular damage on hippocampal function in rats. Prog. Neurobiol. 75, 391–405.
Smith, P. F., Zheng, Y., Horii, A., and Darlington, C. L. (2005). Does vestibular damage cause cognitive dysfunction in humans? J. Vestib. Res. 15, 1–9.
Soma, R., Nozaki, D., Kwak, S., and Yamamoto, Y. (2003). 1/f noise outperforms white noise in sensitizing baroreflex function in the human brain. Phys. Rev. Lett. 91, 078101.
Staab, J. P., and Ruckenstein, M. J. (2003). Which comes first? Psychogenic dizziness versus otogenic anxiety? Laryngoscope 113, 1714–1718.
Staab, J. P. (2006). Chronic dizziness: the interface between psychiatry and neuro-otology. Curr. Opin. Neurol. 19, 41–48.
Stackman, R. W., and Herbert, A. M. (2002). Rats with lesions of the vestibular system require a visual landmark for spatial navigation. Behav. Brain Res. 128, 27–40.
Stackman, R. W., Clark, A. S., and Taube, J. S. (2002). Hippocampal spatial representations require vestibular input. Hippocampus 12, 291–303.
Talkowski, M. E., Redfern, M. S., Jennings, J. R., and Furman, J. M. (2005). Cognitive requirements for vestibular and ocular motor processing in healthy adults and patients with unilateral vestibular lesions. J. Cogn. Neurosci. 17, 1432–1441.
Utz, K. S, Dimova, V., Oppenländer, K., and Kerkhoff, G. (2010). Electrified minds: transcranial direct current stimulation (tDCS) and galvanic vestibular stimulation (GVS) as methods of non-invasive brain stimulation in neuropsychology – a review of current data and future implications. Neuropsychologia 48, 2789–2810.
Vitte, E., Derosier, C., Caritu, Y., Berthoz, A., Hasboun, D., and Soulie, D. (1996). Activation of the hippocampal formation by vestibular stimulation: a functional magnetic resonance imaging study. Exp. Brain Res. 112, 523–526.
Wallace, D. G., Hines, D. J., Pellis, S. M., and Whishaw, I. Q. (2002). Vestibular information is required for dead reckoning in the rat. J. Neurosci. 15, 10009–10017.
Wiener, S. I. and Berthoz, A. (1993) “Forebrain structures mediating the vestibular contribution during navigation.” in Multisensory Control of Movement, ed. A. Berthoz (Oxford: Oxford University Press) 427–456.
Wiener, S. I., Korshunov, V. A., Garcia, R., and Berthoz, A. (1995). Inertial, substratal and landmark cue control of hippocampal CA1 place cell activity. Eur. J. Neurosci. 7, 2206–2219.
Wilkinson, D, Ko, P, Kilduff, P, McGlinchey, R, and Milberg, W. (2005). Improvement of a face perception deficit via subsensory galvanic vestibular stimulation. J. Int. Neuropsychol. Soc. 11, 925–929.
Wilkinson, D., Nicholls, S., Pattenden, C., Kilduff, P., and Milberg, W. (2008). Galvanic vestibular stimulation speeds visual memory recall. Exp. Brain Res. 189, 243–248.
Wilkinson, D., Zubko, O., DeGutis, J., Milberg, W., and Potter, J. (2010). Improvement of a figure copying deficit during subsensory galvanic vestibular stimulation. J. Neuropsychol. 4, 107–118.
Yamamoto, Y., Struzik, Z. R., Soma, R., Ohashi, K., and Kwak, S. (2005). Noisy vestibular stimulation improves autonomic and motor responsiveness in central neurodegenerative disorders. Ann. Neurol. 58, 175–181.
Yardley, L., Gardner, M., Bronstein, A., Davies, R., Buckwell, D., and Luxon, L. (2001). Interference between postural control and mental task performance in patients with vestibular disorders and healthy controls. J. Neurol. Neurosurg. Psychiatr. 71, 48–542.
Yardley, L., Papo, D., Bronstein, A., Gresty, M., Gardner, M., Lavie, N., and Luxon, L. (2002). Attentional demands of continuously monitoring orientation using vestibular information. Neuropsychologia 40, 373–383.
Zheng, Y., Darlington, C. L., and Smith, P. F. (2004). Bilateral vestibular deafferentation impairs object recognition in rat. Neuroreport 15, 1913–1916.
Zheng, Y., Darlington, C. L., and Smith, P. F. (2006). Impairment and recovery on a food foraging task following unilateral vestibular deafferentation in rat. Hippocampus 16, 368–378.
Zheng, Y., Goddard, M., Darlington, C. L., and Smith, P. F. (2007). Bilateral vestibular deafferentation impairs performance in a spatial forced alternation task in rats. Hippocampus 17, 253–256.
Zheng, Y., Goddard, M., Darlington, C. L., and Smith, P. F. (2008). The effects of bilateral vestibular deafferentation on anxiety-related behaviours in Wistar rats. Behav. Brain Res. 193, 55–62.
Zheng, Y., Balabhadrapatruni, S., Munn, O., Masumura, C., Darlington, C. L., and Smith, P. F. (2009). Evidence for deficits in a 5 choice serial reaction time task in rats with bilateral vestibular deafferentation. Behav. Brain Res. 203, 113–117.
Zheng, Y., Goddard, M., Darlington, C. L., and Smith, P. F. (2009). Long-term deficits on a foraging task after bilateral vestibular deafferentation in rats. Hippocampus 19, 480–486.
Zheng, Y., Pearce, J. M., Vann, S. D., Good, M., Trish, A., Smith, P. F., and Aggleton, J. (2003). Using idiothetic cues to learn a swim path with a fixed trajectory and distance: involvement of the hippocampus but not the retrosplenial cortex. Behav. Neurosci. 117, 1363–1377.
Keywords: vestibular system, memory, spatial memory, hippocampus, galvanic vestibular stimulation
Citation: Smith PF, Geddes LH, Baek J-H, Darlington CL and Zheng Y (2010) Modulation of memory by vestibular lesions and galvanic vestibular stimulation. Front. Neur. 1:141. doi: 10.3389/fneur.2010.00141
Received: 26 July 2010;
Paper pending published: 26 August 2010;
Accepted: 13 October 2010;
Published online: 17 November 2010
Edited by:
G. M. Halmagyi, Royal Prince Alfred Hospital, AustraliaReviewed by:
Antonella Palla, Zurich University Hospital, SwitzerlandKonrad Weber, Zurich University Hospital, Switzerland
Aage Moller, University of Texas at Dallas, USA
Copyright: © 2010 Smith, Geddes, Baek, Darlington and Zheng. This is an open-access article subject to an exclusive license agreement between the authors and the Frontiers Research Foundation, which permits unrestricted use, distribution, and reproduction in any medium, provided the original authors and source are credited.
*Correspondence: Paul F. Smith, Department of Pharmacology and Toxicology, School of Medical Sciences, University of Otago Medical School, Dunedin, New Zealand. e-mail:cGF1bC5zbWl0aEBzdG9uZWJvdy5vdGFnby5hYy5ueg==