- 1Non-invasive Brain Stimulation and Neuroplasticity Laboratory, Department of Physiotherapy, School of Primary and Allied Health Care, Faculty of Medicine, Nursing and Health Science, Monash University, Melbourne, VIC, Australia
- 2Discipline of Physiotherapy, School of Health, Federation University Australia, Churchill, VIC, Australia
Exercise performance (EP) is affected by a combination of factors including physical, physiological, and psychological factors. This includes factors such as peripheral, central, and mental fatigue, external peripheral factors such as pain and temperature, and psychological factors such as motivation and self-confidence. During the last century, numerous studies from different fields of research were carried out to improve EP by modifying these factors. During the last two decades, the focus of research has been mainly moved toward the brain as a dynamic ever-changing organ and the ways changes in this organ may lead to improvements in physical performance. Development of centrally-acting performance modifiers such as level of motivation or sleep deprivation and the emergence of novel non-invasive brain stimulation (NIBS) techniques such as transcranial magnetic stimulation (TMS) and transcranial direct current stimulation (tDCS) are the key motives behind this move. This article includes three sections. Section Introduction provides an overview of the mechanisms behind the reduction of EP. The main focus of the Effects of tDCS on EP section is to provide a brief description of the effects of tDCS on maximal and submaximal types of exercise and finally, the section Mechanisms Behind the Effects of tDCS on EP provides description of the mechanisms behind the effects of tDCS on EP.
Introduction
Enhancing exercise performance (EP) portrays the everyday goal for many healthy young persons. In the context of sports, athletes are forced to push their bodily limits to run or swim quicker, lift heavier weights, perform some tasks better or jump higher or further. Therefore, competitors from all different athletic events are encouraged to use innovative approaches to increase their performance. EP is affected by a combination of factors including physical, physiological, and psychological factors. During the last century, numerous researches from different fields of studies were carried out to increase physical performance by modifying these factors (Schubert and Astorino, 2013). Therefore, much of the modern-day literature has ignored the significance of the brain in the regulation of physical performance.
Introduction and development of new non-invasive techniques such as neuroimaging techniques and non-invasive brain stimulation techniques, shed light on the role of the central nervous system on human performance during exercise. As the first step, neuroimaging techniques such as functional magnetic resonance imaging (fMRI) shed light on the role of specific brain areas during simple tasks involving a group of muscles in a single joint or multiple groups of muscles during a more complex task involving multiple parts of the body.
Furthermore, non-invasive brain stimulation techniques (NIBS) such as transcranial direct current stimulation (tDCS) or the centrally-acting performance modifiers, i.e. level of motivation or sleep deprivation are used to modulate these brain areas. incidentally, emerging literature indicates the possibility of influencing performance outcomes following stimulation of specific brain areas. Therefore, during the last two decades, the focus of research has been mainly moved toward the brain as a dynamic ever-changing organ and the ways changes in this organ may lead to improvements in EP.
Altogether, research studies on the use of tDCS provide remarkable understandings about the possible mechanisms behind the effects of tDCS on cortical neurons which finally led to enhancement of EP in healthy individuals. TDCS generates low intensity electric field within the brain (Datta et al., 2009; Edwards et al., 2013; Stagg et al., 2018; Truong and Bikson, 2018). There are two related mechanisms of tDCS that support its use for improvement of EP. The first mechanism is modulation of neuronal excitability and the second one is plasticity (Jackson et al., 2016). Traditionally, it has been established in animal and human studies that application of tDCS can induce polarity-specific changes in neuronal excitability. According to these studies “anodal” tDCS depolarizes neurons and increases neuronal firing frequency and “cathodal” tDCS hyperpolarizes neurons and decreases their firing rates (Creutzfeldt et al., 1962) (Figure 2). It should be noted that, this notion is an oversimplification. The whole neuron does not uniformly depolarize or hyperpolarize in response to the polarity of the applied current. Instead, every neuron has a number of compartments which some of them are depolarizing and others are simultaneously hyperpolarized during application of direct currents (Radman et al., 2009; Rahman et al., 2013). Indeed, the compartments nearer the cathode hyperpolarizing and the ones nearer the anode depolarizing. The polarization in these compartments will be reverses by changes in the polarity of the stimulation. Evidence also supports the non-linear dose-response relationships between tDCS application and the induced changes in corticospinal excitability. A number of recent studies indicate that anodal tDCS may also reduce or cathodal tDCS may also increase the corticospinal excitability (Batsikadze et al., 2013; Monte-Silva et al., 2013; Lopez-Alonso et al., 2014; Tremblay et al., 2016).
When the length of tDCS application increases and passes several minutes, both animal (Bindman et al., 1964; Reato et al., 2015) and human studies using TMS (Nitsche and Paulus, 2000) have confirmed changes in neuronal excitability that remains for minutes or hours after termination of stimulation. Animal models have further linked long lasting variations in excitability of the cortical/brain areas with synaptic plasticity. Long-term potentiation (LTP) and depression (LTD) are examples of these changes (Gartside, 1968; Kronberg et al., 2017; Yu et al., 2019). LTP is a process by which synaptic connections between neurons become stronger with frequent activation (Bliss and Cooke, 2011). On the other hand, LTD is a process by which synaptic connections between neurons become weaker with frequent activation (Bliss and Cooke, 2011). The changes in brain excitability, measured during or immediately after tDCS, and plasticity based on indicators of LTP or LTD are related.
The main objectives of this review are: to provide an overview of different models of fatigue as the underlying mechanisms behind the reduction of EP, a brief description of the effects of tDCS on maximal and submaximal types of exercise, and finally a description of the mechanisms behind the effects of tDCS on EP.
The Mechanisms Behind the Reduction of EP
Fatigue could be considered as factor for reduction of EP. Traditionally, different discipline of sport science provided different definition for fatigue. These definitions are developed to best suit the individual disciplines. For example, an expert in biomechanics may define fatigue as a reduction in the force output generated by a muscle (Allman and Rice, 2002; Millet et al., 2003) and an expert in psychology may define fatigue as a “sensation” of tiredness (Kayser, 2003) on the other hand an expert in physiology may describe fatigue as the malfunction of a specific physiological system (Green, 1997).
Our ambiguity about the understanding of fatigue is may be related to the viewpoint that fatigue is an unfortunate event which due to some involuntary peripheral physiological or biochemical factors reduces EP (Damasio et al., 2000; Noakes and St Clair Gibson, 2004; St Clair Gibson and Noakes, 2004; Lambert et al., 2005; Datta et al., 2009; Dantzer et al., 2014). It may also relate to the use of a reductionist or cause-and-effect approach by the experts from different disciplines of sport which tried to shed light on different underlying mechanisms behind fatigue (St Clair Gibson and Noakes, 2004). Consequently, several direct cause-and-effect models have been introduced to describe fatigue (Noakes, 2000). These models include:
Cardiovascular/Anaerobic Model
In this model, cardiovascular system failure in delivery of oxygen and removal of waste products to and from the active muscles is considered as the main reason behind the fatigue (Noakes, 2000; Noakes et al., 2001).
Energy Supply/Energy Depletion Model
In this model, failure to supply sufficient ATP via different metabolic pathways to the active muscles (Noakes, 2000; Allman and Rice, 2002) is considered as the main reason behind the fatigue.
Neuromuscular Model
In this model, the factors affecting muscle excitation, recruitment and contraction are considered as the main factors behind the fatigue (Noakes, 2000; Millet et al., 2002).
Muscle Trauma Model
This model suggests that muscle damage due to prolonged muscle activity may led to a reduction in capacity of the active muscles to produce power (Gollhofer et al., 1987; del Aguila et al., 1999).
Biomechanical Model
This model suggests that efficiency of movement patterns during muscle activity is main reason behind the fatigue. A more efficient movement pattern will lead to: drop the required VO2 (Gissane et al., 1991), to reduce energy storage (Hahn and Gore, 2001), to hinder the build-up of the metabolites and finally reduces the rise of core body temperature.
Thermoregulatory Model
This model suggests that a critical core body temperature is a main reason behind the reduction or termination of the exercise Coyle and Montain, 1992; Kay et al., 1999).
Psychological/Motivational Model
In this model a lack of enthusiasm or interest in EP is considered as the main factor for reduction or termination of muscle performance (Hargreaves and Febbraio, 1998; Nybo and Nielsen, 2001). This model is usually merged with the neuromuscular model of fatigue.
Central Governor Model
In this model, the main assumption is that the attenuation or termination of EP is controlled by a constant feedback system (Noakes et al., 2001). This system includes a central controller which receive afferent somatosensory pathways and send information on force, displacement, time and muscular metabolism toward the active muscles.
Complex System Model
This model is an extension of the central governor model (Figure 1) and postulated that skeletal muscle fatigue is not influenced by any one of the aforementioned single linear models. According to this model, EP is continuously controlled by the interaction of multiple physiological systems checked by continuous feed-forward and feedback mechanisms (St Clair Gibson and Noakes, 2004; Lambert et al., 2005).
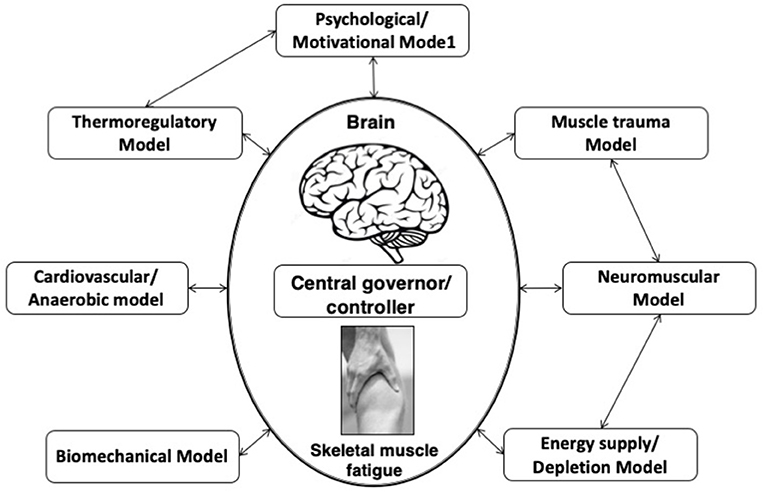
Figure 1. Complex systems model of fatigue. Interaction of developed fatigue models. In this complex systems model of fatigue peripheral feedback originating from numerous linear models of fatigue are integrated by the brain, along with centrally located senses.
The idea of the central nervous system as contributing factor in the development of fatigue is back to the early work by Mosso (1904) which concluded a decreased capacity to perform repeated muscle contractions after a prolonged period of demanding cognitive activity, lead to the development of a psychobiological state which is called psychological or mental model of fatigue (Figure 2). It has implications for many activities of daily living including physical performance.
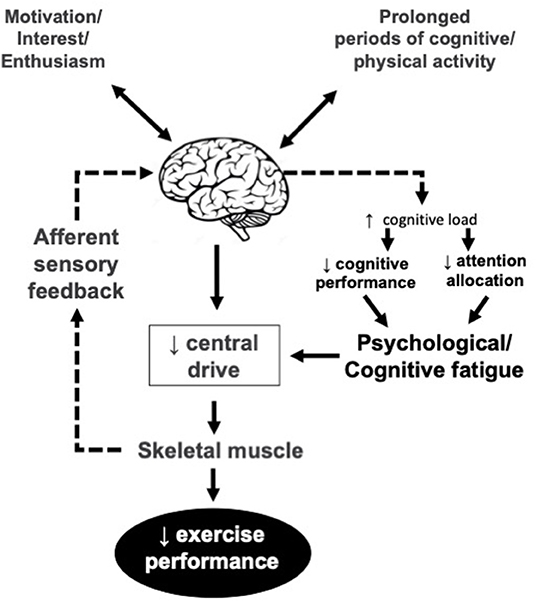
Figure 2. Psychological/mental model of fatigue. Central drive is reduced due to lower motivation, interest and/or enthusiasm and lack of cognitive resources for the EP. The reduced enthusiasm may or may not be related to afferent sensory feedback.
Psychological or mental model of fatigue is related to lack of energy, motivation, and alertness increased fatigability, and feelings of tiredness (Dantzer et al., 2014), and a reason behind the increased risk of error in the workplace (McCormick et al., 2012). From a neurophysiological viewpoint, mental fatigue inhibits athletes' performance by enhancing perceived exertion (Ishii et al., 2014) and also by deactivation of the mechanisms responsible for neurofacilitation that normally encourages athletes toward action (Hallett, 2007). It decreases physical performance even during long-duration exercise which seems less dependent on cognitive functioning (Marcora et al., 2009; Elferink-Gemser and Hettinga, 2017; Van Cutsem et al., 2017). Unlike endurance exercise, mental fatigue seems not to affect athletes' maximal strength, explosive power, and anaerobic work (Boksem et al., 2006; Dantzer et al., 2014; Martin et al., 2015; Van Cutsem et al., 2017).
Different fatigue mechanisms and adaptation of power outputs in long and short duration tasks is the reason behind this difference (Gandevia, 2001). Short duration, anaerobic types of exercises are mainly affected by peripheral fatigue (Coggan and Coyle, 1991), while long duration, aerobic (endurance) types of exercises, are affected by a decline in central motor drive and central fatigue (Amann, 2011). Hence, while during endurance exercise, the brain decides when to stop, during short-term exercise, the muscles are the main decision-makers (Gandevia, 2001). In this regard, Mental fatigue may disrupt the decision-making process involved in choosing an optimal pacing strategy (Martin et al., 2018).
The Effects of tDCS on EP
The literature on EP provides exciting understandings on the effects of tDCS on EP in healthy individuals (Figure 3). In these studies, the effects of tDCS were investigated on maximal (explosive) and submaximal (endurance) performances. This is important because these different exercise intensities require completely different metabolic, cardiorespiratory, and psychological demands, and therefore affect the brain activity differently (Sidhu et al., 2013).
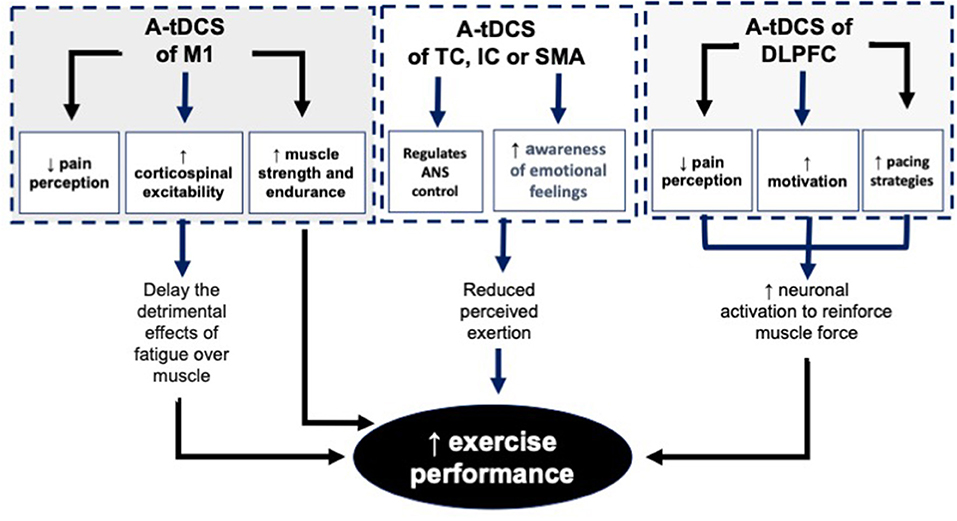
Figure 3. The mechanisms behind the effects of A-tDCS of M1, TC, IC, SMA and DLPFC on EP. A-tDCS, Anodal transcranial direct current stimulation; M1, Primary motor cortex; TC, Temporal cortex; IC, Insular cortex; SMA, Supplementary motor area; DLPFC, Dorsolateral.
How tDCS of M1 Affects Maximal Force Capacities
Despite the methodological differences in study design, experimental tasks, tDCS parameters, and montages in different studies, the literature indicates that a single session of unilateral tDCS over the dominant M1 failed to improve anaerobic maximal types of exercise (Cogiamanian et al., 2007; Kan et al., 2013; Williams et al., 2013; Angius et al., 2015, 2016a,b; Baldari and Buzzachera, 2018; Romero-Arenas et al., 2021). da Silva Machado et al. (2021), in a single session tDCS study compared the effects of conventional tDCS of M1 (2 mA, 20 min) with high definition tDCS (2.4 mA, 20 min) on exercise performance (time to exhaustion at 80% peak power) on a cycle simulator. They concluded, a single session of neither HD-tDCS nor conventional tDCS changed exercise performance and psychophysiological responses in athletes.
The reason behind this failure may lie in the fact that under maximal force conditions, muscles are already functioning maximally and all motor units within the involved muscles are already recruited and therefore the ceiling effects do not allow tDCS to show any further effects.
However, unlike the findings in these studies, recently, Lattari et al. (2020) concluded that 2 mA, 20 min bilateral tDCS of M1 significantly increases power during power-related tasks such as the vertical jumping ability. Codella et al. (2021), is also showed that bilateral tDCs of M1, enhance power in lower limb muscles.
How tDCS of M1 Affects Submaximal Force Capacities
Having said that, the same literature (listed in the previous section) indicates positive effects of a single session of tDCS on submaximal intensity tasks in the majority of the studies (Cogiamanian et al., 2007; Williams et al., 2013; Angius et al., 2015, 2016a,b, 2018; Vitor-Costa Okuno et al., 2015; Abdelmoula et al., 2016; Lattari et al., 2018; Huang et al., 2019; Codella et al., 2021).
How tDCS of DLPFC or TC Affects EP
The number of tDCS studies on other cortical sites of the brain such as dorsolateral prefrontal cortex (DLPFC) or temporal cortex (TC) is very low.
To investigate the effect of a single session tDCS of DLPFC (2 mA for 15 min) on the force-velocity relationship, strength training volume, movement velocity, and PRE in healthy non-professional participants, Alix-Fages et al. (2020), showed an increase in training volume, preservation of higher movement velocities, and reduction of ratings of perceived exertion (RPE) values. Lattari et al. (2018) applied tDCS over the left DLPFC (2 mA for 20 min) before a time to exhaustion (TTE) test (100% of peak power) in 11 moderately active women and found longer TTE compared to sham. Angius et al. (2019), used a similar protocol, and applied a single session of tDCS over the left DLPFC (2 mA) but with a longer duration (30 min) in 12 trained participants before a TTE test (70% of peak power). Participants were able to cycle for longer durations after tDCS, with lower HR and RPE compared to sham.
It is important to note that despite the positive results previously reported, some studies, however, have found no improvement using relatively similar protocols to the previous studies. Holgado et al. (2019) (n = 36), investigated the effects of tDCS of DLPFC (2 mA for 20 min) on power output, heart rate, RPE, and electroencephalography at baseline and during a 20-min time-trial self-paced exercise. They concluded neither power output, heart rate, RPE nor electroencephalography activity were affected by tDCS.
Similarly, the two studies on the effects of tDCS of TC on EP, are also concluded opposite conflicting findings on EP. Evidence shows that a single session tDCS (20 min, 2 mA) of TC, targeting the left insular cortex (IC), enhanced cycling performance in professional cyclists. They also showed that tDCS of TC decreases heart rate and increased delay in parasympathetic vagal withdrawal and RPE in submaximal exercise intensities (Okano et al., 2015). Unlike the positive findings in the above study, Okano et al. (2017) evaluated the effects of a single session tDCS of left TC targeting the left IC (20 min, 2 mA), on physiological and psychological responses during 30 min of vigorous exercise with a constant load (80% heart rate). The findings of this study suggest that tCDS of TC does not modulate either heart rate at rest or heart rate, RPE, and affective responses during exercise.
How Direct Current Stimulation (DCS) of the Spinal Cord Affect EP
Sasada et al. (2017), investigated the effects of a single session of direct current stimulation (DCS) of the spinal cord (2 mA for 20 min) using the Halo Sport device on physical fitness indicators of healthy, physically active, men (n = 17). All participants underwent either stimulation or sham, before a vertical jump, sit & reach, and endurance running tests. The results suggest that DCS of spinal cord using a Halo Sport system can enhance the output in these three physical fitness measures in physically active participants.
How Bilateral tDCS of M1 Using a Halo Sport Device Affect EP
The Halo Sport device is a commercial brain stimulation device that contains a headset like a usual headphone. This device uses weak direct currents below 2–3 mA (tDCS) which can be applied bilaterally over the scalp through surface electrodes. The main objective of this application is the induction of changes in motor cortex in both sides of the brain.
Huang et al. (2019), investigated the effects of a single session bilateral tDCS of M1 (2 mA for 20 min) using the Halo Sport device on repeated sprint cycling ability (n = 9). Peak and mean power output were measured for 5 × 6-s sprints interspersed with 24 s of active recovery on a cycle ergometer. The results suggest that tDCS with the Halo Sport system can enhance mean power output in physically active participants. In another study, Codella et al. (2021), investigated the effects of a single session bilateral tDCS of M1 (2 mA for 20 min) using the Halo Sport device on physical fitness indicators of healthy, physically active, men (n = 17). All participants underwent either stimulation or sham, before a vertical jump, sit and reach, and endurance running tests. The results suggest that tDCS with the Halo Sport system can enhance the output in these three physical fitness measures in physically active participants.
Mechanisms Behind the Effects of tDCS on EP
The underlying mechanisms behind the positive effects of tDCS of M1 on EP are not fully understood yet. Literature indicates the following mechanisms behind the effects of a-tDCS on the enhancement of EP:
Facilitation of M1 and Enhancement of Corticospinal Excitability
TDCS depends on the parameters used, may facilitate the M1, and therefore enhancing corticospinal excitability during exercise (Cogiamanian et al., 2007; Williams et al., 2013). This hypothesis is challenged by Abdelmoula et al. (2016) which concluded lack of relationship between the improvement in corticospinal excitability and EP. It should be noted that the M1 is not the only active brain site during exercise, therefore well-designed double-blinded studies to establish the relationship between the cortical/corticospinal changes and EP is necessary.
Reduction of the Fatigue Through Affecting the Central Governor/Controller
TDCS of M1 may also lead to a reduction of fatigue (Cogiamanian et al., 2007; Williams et al., 2013; Vitor-Costa Okuno et al., 2015). A neural pathway that connects a large number of brain areas, including, the spinal cord, thalamus, secondary somatosensory cortex, medial IC, posterior cingulate cortex, anterior cingulate cortex, premotor area, supplementary motor area, and M1 represents the inhibitory network which leads to fatigue. The balance between inhibitory and facilitatory mechanisms in the M1 optimize the cortical excitability and therefore increase the magnitude of EP. Application of tDCS may induces facilitatory effects to increase motor output from the M1 helps to overcome the existing central fatigue (Vitor-Costa Okuno et al., 2015).
Reduction of Psychological or Mental Fatigue
Decision making-process during pacing and cognitive control necessary to choose an optimal pacing strategy may be disrupted by mental fatigue (Martin et al., 2018). This disruption is much more evident in long-term aerobic types of exercise. TDCS of DLPFC is a non-invasive technique for the reduction of mental fatigue and therefore enhancement of PE (Nikooharf Salehi et al., 2021). It should be noted that this reduction is only affecting submaximal endurance type of exercise. Literature indicates that mental fatigue does not affect athletes' maximal strength and anaerobic work (Boksem et al., 2006; Dantzer et al., 2014; Martin et al., 2015; Van Cutsem et al., 2017). The difference in the adaptation of athletes for their power output during these long and short duration tasks could be the reason behind this difference (Gandevia, 2001).
Reduction of RPE
Reduction of RPE following application of tDCS is considered as one of the other reasons behind increased EP in several studies (Okano et al., 2015; Angius et al., 2016a,b). Modulation of sensory perception of effort plays a crucial role in the control of motor output commands (Okano et al., 2015). Overall, the amount of motor commands from M1 or premotor area is considered as the reason behind the changes in RPE (de Morree et al., 2012, 2014; Goodall et al., 2013; Takarada et al., 2014; Zénon et al., 2015).
Modulation of Autonomic Nervous System Activity
Literature indicates that the autonomic nervous system (ANS) has an important role in the regulation of EP (Okano et al., 2015). Literature supports the association between TC and IC, with ANS control. Therefore, tDCS can modulate the cortical areas directly under the electrodes related to ANS.
ANS is highly related to the mechanisms behind EP and fatigue. It controls homeostatic mechanisms (Damasio et al., 2000; Craig, 2003), especially during PE which requires high metabolic demands (Tulppo et al., 1998; Williamson, 2010). Indeed, ANS responses are linked to EP in healthy individuals (Tanaka et al., 2009). Individuals with higher fitness levels (high aerobic capacity) usually have significantly greater heart rate variability, which is controlled by vagal modulation of the heart rate, compared to individuals with lower fitness levels (low aerobic capacity) (Tulppo et al., 1998).
Reduction of Perceived Pain
The effects of pain-inducing substances suggest that perception of pain is one of the important regulators of exertion level during fatiguing exercise. Literature shows that a common analgesic such as Acetaminophen increases cycling performance (Mauger et al., 2010). Recent literature indicates that endogenous inhibitory responses, which normally act to decrease nociceptive input and reduce the perception of pain, could be increased following application of tDCS over M1 (Flood et al., 2016). TDCS stimulates descending regions associated with endogenous pain inhibition, enhancing central pain inhibitory responses and causing widespread analgesia (Flood et al., 2016). The association between the pain inhibitory networks and regulation of EP is challenged by the study of Flood et al. (2017) which did not show any significant increase in maximal force production or muscular endurance following application of a single session of high definition tDCS.
Suggestions for Future Research
There are a number of issues regarding the studies reported in this review which may have implications for future research:
1. Almost all studies reported in this review used single session tDCS. Multiple session tDCS studies are recommended because of its accumulating effects.
2. Majority of studies, used unilateral tDCS of M1. New studies using bilateral tDCS of M1 for both upper limbs, trunk and lower limb muscles are recommended specially in cases that the activity involve trunk and all extremities.
3. Single site tDCS of a brain site was used in almost all of the included studies. It should be noted that multiple sites of the brain never working in isolation. Multi-site application of tDCS is recommended for future studies.
4. In almost all of the included studies, large tDCS electrodes (5 x 7 or 4–6 cm2) were used for modulation of single sites of the brain. New tDCS studies using small electrodes is recommended to increase focality of the effects.
In summary, different mechanisms such as facilitation of M1 which causes enhancement of corticospinal excitability, reduction of the supraspinal fatigue, reduction of the psychological/mental fatigue, reduction of PRE, modulation of ANS activity, and reduction of perceived pain play important roles in the enhancement of EP. Literature indicates that modulation of M1, DLPFC, TC, IC, and SMA using unilateral or bilateral tDCS techniques enables us to benefit from these mechanisms.
Author Contributions
SJ and MZ contributed to all steps including the design of the review to writing of the first and other drafts of the review. Both authors contributed to the article and approved the submitted version.
Conflict of Interest
The authors declare that the research was conducted in the absence of any commercial or financial relationships that could be construed as a potential conflict of interest.
Publisher's Note
All claims expressed in this article are solely those of the authors and do not necessarily represent those of their affiliated organizations, or those of the publisher, the editors and the reviewers. Any product that may be evaluated in this article, or claim that may be made by its manufacturer, is not guaranteed or endorsed by the publisher.
References
Abdelmoula, A., Baudry, S., and Duchateau, J. (2016). Anodal transcranial direct current stimulation enhances time to task failure of a submaximal contraction of elbow flexors without changing corticospinal excitability. Neuroscience 322, 94–103. doi: 10.1016/j.neuroscience.2016.02.025
Alix-Fages, C., García-Ramos, A., Calderón-Nadal, G., Colomer-Poveda, D., and Romero-Arenas, S. (2020). Anodal transcranial direct current stimulation enhances strength training volume but not the force-velocity profile. Eur. J. Appl. Physiol. 120, 1881–1891. doi: 10.1007/s00421-020-04417-2
Allman, B. L., and Rice, C. L. (2002). Neuromuscular fatigue and aging: central and peripheral factors. Muscle Nerve 25, 785–796. doi: 10.1002/mus.10116
Amann, M. (2011). Central and peripheral fatigue: interaction during cycling exercise in humans. Med. Sci. Sports Exerc. 43, 2039–2045. doi: 10.1249/MSS.0b013e31821f59ab
Angius, L., Marcora, S. M., Hopker, J. G., and Mauger, A. R. (2016a). Transcranial direct current stimulation improves cycling performance in healthy individuals. Proc. Physiol. Soc. 35, C03.
Angius, L., Mauger, A. R., Hopker, J., Pascual-Leone, A., Santarnecchi, E., and Marcora, S. M. (2018). Bilateral extracephalic transcranial direct current stimulation improves endurance performance in healthy individuals. Brain Stimul. 11, 108–117. doi: 10.1016/j.brs.2017.09.017
Angius, L., Pageaux, B., Hopker, J., Marcora, S. M., and Mauger, A. R. (2015). Transcranial direct current stimulation improves isometric time to exhaustion performance of lower limbs. Proc. Physiol. Soc. 34, C54. Available online at: https://kar.kent.ac.uk/id/eprint/56787
Angius, L., Pageaux, B., Hopker, J., Marcora, S. M., and Mauger, A. R. (2016b). Transcranial direct current stimulation improves isometric time to exhaustion of the knee extensors. Neuroscience 339, 363–375. doi: 10.1016/j.neuroscience.2016.10.028
Angius, L., Santarnecchi, E., Pascual-Leone, A., and Marcora, S. M. (2019). Transcranial direct current stimulation over the left dorsolateral prefrontal cortex improves inhibitory control and endurance performance in healthy individuals. Neuroscience 419, 34–45. doi: 10.1016/j.neuroscience.2019.08.052
Baldari, C., Buzzachera, C. F., Vitor-Costa, M., Gabardo, J. M., Bernardes, A. G., Altimari, L. R., et al. (2018). Effects of transcranial direct current stimulation on psychophysiological responses to maximal incremental exercise test in recreational endurance runners. Front. Psychol. 9, 1867. doi: 10.3389/fpsyg.2018.01867
Batsikadze, G., Moliadze, V., Paulus, W., Kuo, M. F., and Nitsche, M. A. (2013). Partially non-linear stimulation intensity-dependent effects of direct current stimulation on motor cortex excitability in humans. J. Physiol. 591, 1987–2000. doi: 10.1113/jphysiol.2012.249730
Bindman, L. J., Lippold, O. C., and Redfearn, J. W. (1964). The action of brief polarizing currents on the cerebral cortex of the rat (1). during current flow and (2). in the production of long-lasting after-effects. J. Physiol. Lond. 172, 369–382. doi: 10.1113/jphysiol.1964.sp007425
Bliss, T. V. P., and Cooke, S. F. (2011). Long-term potentiation and long-term depression: a clinical perspective. Clinics (São Paulo) 66(Suppl. 1), 3–17. doi: 10.1590/S1807-59322011001300002
Boksem, M. A., Meijman, T. F., and Lorist, M. M. (2006). Mental fatigue, motivation and action monitoring. Biol. Psychol. 72, 123–132. doi: 10.1016/j.biopsycho.2005.08.007
Codella, R., Alongi, R., Filipas, L., and Luzi, L. (2021). Ergogenic effects of bihemispheric transcranial direct current stimulation on fitness: a randomized cross-over trial. Int. J. Sports Med. 42, 66–73. doi: 10.1055/a-1198-8525
Coggan, A. R., and Coyle, E. F. (1991). Carbohydrate ingestion during prolonged exercise: effects on metabolism and performance. Exerc. Sports Sci. Rev. 19, 1–40. doi: 10.1249/00003677-199101000-00001
Cogiamanian, F., Marceglia, S., Ardolino, G., Barbieri, S., and Priori, A. (2007). Improved isometric force endurance after transcranial direct current stimulation over the human motor cortical areas. Eur. J. Neurosci. 26, 242–249. doi: 10.1111/j.1460-9568.2007.05633.x
Coyle, E. F., and Montain, S. J. (1992). Carbohydrate, and fluid ingestion during exercise: are there trade-offs? Med. Sci. Sports Exerc. (1992) 24, 671–678. doi: 10.1249/00005768-199206000-00009
Craig, A. D. (2003). Interception: the sense of the physiological condition of the body. Curr. Opin. Neurobiol. 13, 500–505. doi: 10.1016/S0959-4388(03)00090-4
Creutzfeldt, O. D., Fromm, G. H., and Kapp, H. (1962). Influence of transcortical d-c currents on cortical neuronal activity. Exp Neurol. 5, 436–452. doi: 10.1016/0014-4886(62)90056-0
da Silva Machado, D. G., Bikson, M., Datta, A., et al. (2021). Acute effect of high-definition and conventional tDCS on exercise performance and psychophysiological responses in endurance athletes: a randomized controlled trial. Sci. Rep. 11, 13911. doi: 10.1038/s41598-021-92670-6
Damasio, A. R., Grabowski, T. J., Bechara, A., Damasio, H., Ponto, L. L., Parvizi, J., et al. (2000). Subcortical and cortical brain activity during the feeling of self-generated emotions. Nat. Neurosci. 3, 1049–1056. doi: 10.1038/79871
Dantzer, R., Heijnen, C. J., Kavelaars, A., Laye, S., and Capuron, L. (2014). The neuroimmune basis of fatigue. Trends Neurosci. 37, 39–46. doi: 10.1016/j.tins.2013.10.003
Datta, A., Bansal, V., Diaz, J., Patel, J., Reato, D., and Bikson, M. (2009). Gyri-precise head model of transcranial direct current stimulation: improved spatial focality using a ring electrode versus conventional rectangular pad. Brain Stimulat. 2:201–207. doi: 10.1016/j.brs.2009.03.005
de Morree, H. M., Klein, C., and Marcora, S. M. (2012). Perception of effort reflects central motor command during movement execution. Psychophysiology 49, 1242–1253. doi: 10.1111/j.1469-8986.2012.01399.x
de Morree, H. M., Klein, C., and Marcora, S. M. (2014). Cortical substrates of the effects of caffeine and time-on-task on perception of effort. J Appl Physiol. 117, 1514–23. doi: 10.1152/japplphysiol.00898.2013
del Aguila, L. F., Claffey, K. P., and Kirwan, J. P. (1999). TNF-alpha impairs insulin signaling and insulin stimulation of glucose uptake in C2C12 muscle cells. Am. J. Physiol. 276, E849–855. doi: 10.1152/ajpendo.1999.276.5.E849
Edwards, D., Cortes, M., Datta, A., Minhas, P., Wassermann, E. M., and Bikson, M. (2013). Physiological and modeling evidence for focal transcranial electrical brain stimulation in humans: a basis for high-definition tDCS. Neuroimage 74, 266–275. doi: 10.1016/j.neuroimage.2013.01.042
Elferink-Gemser, M. T., and Hettinga, F. J. (2017). Pacing and self-regulation: important skills for talent development in endurance sports. Int. J. Sports Physiol. Perform. 12, 831–835. doi: 10.1123/ijspp.2017-0080
Flood, A., Waddington, G., and Cathcart, S. (2016). High-definition transcranial direct current stimulation enhances conditioned pain modulation in healthy volunteers: a randomized trial. J. Pain 17, 600–605. doi: 10.1016/j.jpain.2016.01.472
Flood, A., Waddington, G., and Keegan, R. J. (2017). The effects of elevated pain inhibition on endurance exercise performance. PeerJ 5, e3028. doi: 10.7717/peerj.3028
Gandevia, S. C. (2001). Spinal and supraspinal factors in human muscle fatigue. Physiol. Rev. 81, 1725–1789. doi: 10.1152/physrev.2001.81.4.1725
Gartside, I. B. (1968). Mechanisms of sustained increases of firing rate of neurones in the rat cerebral cortex after polarization: role of protein synthesis. Nature 220, 383–384. doi: 10.1038/220383a0
Gissane, C., Corrigan, D. L., and White, J. A. (1991). Gross efficiency responses to exercise conditioning in adult males of various ages. J. Sports Sci. 9, 383–391. doi: 10.1080/02640419108729898
Gollhofer, A., Komi, P.V., Fujitsuka, N., and Miyashita, M. (1987). Fatigue during stretch-shortening cycle exercises II: changes in neuromuscu- lar activation patterns of human skeletal muscle. Int. J. Sports Med. (1987) 8, 38–47. doi: 10.1055/s-2008-1025644
Goodall, S., St Clair Gibson, A., Voller, B., Lomarev, M., Howatson, G., Dang, N., et al. (2013). Repetitive transcranial magnetic stimulation attenuates the perception of force output production in non-exercised hand muscles after unilateral exercise. PLoS ONE 8, e80202. doi: 10.1371/journal.pone.0080202
Green, H. J. (1997). Mechanisms of muscle fatigue in intense exercise. J. Sports Sci. 15, 247–256. doi: 10.1080/026404197367254
Hahn, A. G., and Gore, C. J. (2001). The effects of altitude on cycling performance. Sports Med. 31, 533–557. doi: 10.2165/00007256-200131070-00008
Hallett, M. (2007). Volitional control of movement: the physiology of free will. Clin. Neurophysiol. 118, 1179–1192. doi: 10.1016/j.clinph.2007.03.019
Hargreaves, M., and Febbraio, M. (1998). Limits to exercise performance in the heat. Int. J. Sports Med. 19 Suppl.2, S115–S116. doi: 10.1055/s-2007-971973
Holgado, D., Zandonai, T., Ciria, L. F., Zabala, M., Hopker, J., and Sanabria, D. (2019). Transcranial direct current stimulation (tDCS) over the left prefrontal cortex does not affect time-trial self-paced cycling performance: evidence from oscillatory brain activity and power output. PLoS ONE 14, e0210873. doi: 10.1371/journal.pone.0210873
Huang, L., Deng, Y., Zheng, X., and Liu, Y. (2019). Transcranial direct current stimulation with halo sport enhances repeated sprint cycling and cognitive performance. Front. Physiol. 10, 1118. doi: 10.3389/fphys.2019.00118
Ishii, A., Tanaka, M., and Watanabe, Y. (2014). Neural mechanisms of mental fatigue. Rev. Neurosci. 25, 469–479. doi: 10.1515/revneuro-2014-0028
Jackson, M. P., Rahman, A., Lafon, B., Kronberg, G., Ling, D., Parra, L. C., et al. (2016). Animal models of transcranial direct current stimulation: methods and mechanisms. Clin. Neurophysiol. 127, 3425–3454. doi: 10.1016/j.clinph.2016.08.016
Kan, B., Dundas, J. E., and Nosaka, K. (2013). Effect of transcranial direct current stimulation on elbow flexor maximal voluntary isometric strength and endurance. Appl. Physiol. Nutr. Metab. 38, 734–739. doi: 10.1139/apnm-2012-0412
Kay, D., Taaffe, D. R., and Marino, F. E. (1999). Whole-body pre-cooling and heat storage during self-paced cycling performance in warm humid conditions. J. Sports Sci. 17, 937–944. doi: 10.1080/026404199365326
Kayser, B. (2003). Exercise starts and ends in the brain. Eur. J. Appl. Physiol. 90, 441–449. doi: 10.1007/s00421-003-0902-7
Kronberg, G., Bridi, M., Abel, T., Bikson, M., and Parra, L. C. (2017). Direct current stimulation modulates LTP and LTD: activity dependence and dendritic effects. Brain Stimulat. 10, 51–58. doi: 10.1016/j.brs.2016.10.001
Lambert, M., St Clair Gibson, A., and Noakes, T. D. (2005). Complex systems model of fatigue: integrative homeostatic control of peripheral physiological systems during exercise in humans. Br. J. Sports Med. 39, 52–62. doi: 10.1136/bjsm.2003.011247
Lattari, E., Campos, C., Lamego, M. K., Legey, S., Neto, G. M., Rocha, N. B., et al. (2020). Can transcranial direct current stimulation improve muscle power in individuals with advanced weight-training experience? J. Strength Cond. Res. 34, 97–103. doi: 10.1519/JSC.0000000000001956
Lattari, E., de Oliveira, B. S., Oliveira, B. R. R., de Mello Pedreiro, R. C., Machado, S., and Neto, G. A. M. (2018). Effects of transcranial direct current stimulation on time limit and ratings of perceived exertion in physically active women. Neurosci. Lett. 662, 12–16. doi: 10.1016/j.neulet.2017.10.007
Lopez-Alonso, V., Cheeran, B., Rio-Rodriguez, D., and Fernandez-Del-Olmo, M. (2014). Inter-individual variability in response to non-invasive brain stimulation paradigms. Brain Stimul. 7, 372–380. doi: 10.1016/j.brs.2014.02.004
Marcora, S. M., Staiano, W., and Manning, V. (2009). Mental fatigue impairs physical performance in humans. J. Appl. Physiol. 106, 857–864. doi: 10.1152/japplphysiol.91324.2008
Martin, K., Meeusen, R., Thompson, K. G., Keegan, R., and Rattray, B. (2018). Mental fatigue impairs endurance performance: a physiological explanation. Sports Med. 48, 2041–2051. doi: 10.1007/s40279-018-0946-9
Martin, K., Thompson, K. G., Keegan, R., Ball, N., and Rattray, B. (2015). Mental fatigue does not affect maximal anaerobic exercise performance. Eur. J. Appl. Physiol. 115, 715–725. doi: 10.1007/s00421-014-3052-1
Mauger, A. R., Jones, A. M., and Williams, C. A. (2010). Influence of acetaminophen on performance during time trial cycling. J. Appl. Physiol. 108, 98–104. doi: 10.1152/japplphysiol.00761.2009
McCormick, F., Kadzielski, J., Landrigan, C. P., Evans, B., Herndon, J. H., and Rubash, H. E. (2012). Surgeon fatigue: a prospective analysis of the incidence, risk, and intervals of predicted fatigue-related impairment in residents. Arch Surg. 147, 430–435. doi: 10.1001/archsurg.2012.84
Millet, G. Y., Lepers, R., Maffiuletti, N. A., Babault, N., Martin, V., and Lattier, G. (2002). Alterations of neuromuscular function after an ultramarathon. J. Appl. Physiol. 92, 486–492. doi: 10.1152/japplphysiol.00122.2001
Millet, G. Y., Millet, G. P., Lattier, G., Maffiuletti, N. A., and Candau, R. (2003). Alteration of neuromuscular function after a prolonged road cycling race. Int. J. Sports Med. 24, 190–194. doi: 10.1055/s-2003-39088
Monte-Silva, K., Kuo, M. F., Hessenthaler, S., Fresnoza, S., Liebetanz, D., Paulus, W., et al. (2013). Induction of late LTP-like plasticity in the human motor cortex by repeated non-invasive brain stimulation. Brain Stimul. 6, 424–432. doi: 10.1016/j.brs.2012.04.011
Nikooharf Salehi, E., Jaydari Fard, S., Jaberzadeh, S., and Zoghi, M. (2021). Transcranial direct current stimulation reduces the negative impact of mental fatigue on swimming performance. J. Motor Behav. 25, 1–10. doi: 10.1080/00222895.2021.1962238
Nitsche, M. A., and Paulus, W. (2000). Excitability changes induced in the human motor cortex by weak transcranial direct current stimulation. J. Physiol. 527, 633–639. doi: 10.1111/j.1469-7793.2000.t01-1-00633.x
Noakes, T. D. (2000). Physiological models to understand exercise fatigue and the adaptations that predict or enhance athletic performance. Scand. J. Med. Sci. Sports. 10, 123–145. doi: 10.1034/j.1600-0838.2000.010003123.x
Noakes, T. D., Peltonen, J. E., and Rusko, H. K. (2001). Evidence that a central governor regulates exercise performance during acute hypoxia and hyperoxia. J. Exp. Biol. 204(Pt 18), 3225–3234. doi: 10.1242/jeb.204.18.3225
Noakes, T. D., and St Clair Gibson, A. (2004). Logical limitations to the “catastrophe” models of fatigue during exercise in humans. Br. J. Sports Med. 38, 648–649. doi: 10.1136/bjsm.2003.009761
Nybo, L., and Nielsen, B. (2001). Hyperthermia and central fatigue during prolonged exercise in humans. J. Appl. Physiol. 91, 1055–1060. doi: 10.1152/jappl.2001.91.3.1055
Okano, A. H., Fontes, E. B., Montenegro, R. A., Farinatti Pde, T., Cyrino, E. S., Li, L. M., et al. (2015). Brain stimulation modulates the autonomic nervous system, rating of perceived exertion and performance during maximal exercise. Br. J. Sports Med. 49, 1213–1218. doi: 10.1136/bjsports-2012-091658
Okano, A. H., Machado, D. G. S., Neto, L. O., Farias-Junior, L.F., Agrícola, P. M. D., Arruda, A., et al. (2017). Can transcranial direct current stimulation modulate psychophysiological response in sedentary men during vigorous aerobic exercise? Int. J. Sports Med. 38, 493–500. doi: 10.1055/s-0042-121897
Radman, T., Ramos, R. L., Brumberg, J. C., and Bikson, M. (2009). Role of cortical cell type and morphology in subthreshold and suprathreshold uniform electric field stimulation in vitro. Brain Stimul. 2, 215–228. doi: 10.1016/j.brs.2009.03.007
Rahman, A., Reato, D., Arlotti, M., Gasca, F., Datta, A., Parra, L. C., et al. (2013). Cellular effects of acute direct current stimulation: somatic and synaptic terminal effects. J. Physiol. 591, 2563–2578. doi: 10.1113/jphysiol.2012.247171
Reato, D., Bikson, M., and Parra, L. C. (2015). Lasting modulation of in vitro oscillatory activity with weak direct current stimulation. J. Neurophysiol. 113, 1334–1341. doi: 10.1152/jn.00208.2014
Romero-Arenas, S., Calderón-Nadal, G., Alix-Fages, C., Jerez-Martínez, A., Colomer-Poveda, D., and Márquez, G. (2021). Transcranial direct current stimulation does not improve countermovement jump performance in young healthy men. J. Strength. Cond. Res. 35, 2918–2921. doi: 10.1519/JSC.0000000000003242
Sasada, S., Endoh, T., Ishii, T., and Komiyama, T. (2017). Polarity-dependent improvement of maximal-effort sprint cycling performance by direct current stimulation of the central nervous system. Neurosci Lett. 657, 97–101. doi: 10.1016/j.neulet.2017.07.056
Schubert, M. M., and Astorino, T. A. (2013). A systematic review of the efficacy of ergogenic aids for improving running performance. J. Strength Condit. Res. 27, 1699–1707. doi: 10.1519/JSC.0b013e31826cad24
Sidhu, S. K., Cresswell, A. G., and Carroll, T. J. (2013). Corticospinal responses to sustained locomotor exercises: moving beyond single-joint studies of central fatigue. Sports Med. 43, 437–449. doi: 10.1007/s40279-013-0020-6
St Clair Gibson, A., and Noakes, T. D. (2004). Evidence for complex system integration and dynamic neural regulation of skeletal muscle recruitment during exercise in humans. Br. J. Sports Med. 38, 797–806. doi: 10.1136/bjsm.2003.009852
Stagg, C. J., Antal, A., and Nitsche, M. A. (2018). Physiology of transcranial direct current stimulation. J. ECT 34, 144–152. doi: 10.1097/YCT.0000000000000510
Takarada, Y., Mima, T., Abe, M., Nakatsuka, M., and Taira, M. (2014). Inhibition of the primary motor cortex can alter one's “sense of effort”: effects of low-frequency rTMS. Neurosci. Res. 89, 54–60. doi: 10.1016/j.neures.2014.09.005
Tanaka, S., Hanakawa, T., Honda, M., and Watanabe, K. (2009). Enhancement of pinch force in the lower leg by anodal transcranial direct current stimulation. Exp. Brain. Res. 196, 459–465. doi: 10.1007/s00221-009-1863-9
Tremblay, S., Larochelle-Brunet, F., Lafleur, L. P., El Mouderrib, S., Lepage, J. F., and Théoret, H. (2016). Systematic assessment of duration and intensity of anodal transcranial direct current stimulation on primary motor cortex excitability. Eur. J. Neurosci. 44, 2184–2190. doi: 10.1111/ejn.13321
Truong, D. Q., and Bikson, M. (2018). Physics of transcranial direct current stimulation devices and their history. J. ECT 34, 137–143. doi: 10.1097/YCT.0000000000000531
Tulppo, M. P., Mäkikallio, T. H., Seppänen, T., Laukkanen, R. T., and Huikuri, H. V. (1998). Vagal modulation of heart rate during exercise: effects of age and physical fitness. Am. J. Physiol. 274, 424–429. doi: 10.1152/ajpheart.1998.274.2.H424
Van Cutsem, J., De Pauw, K., Marcora, S., et al. (2017). A caffeine-maltodextrin mouth rinse counters mental fatigue. Psychopharmacology 235, 947–958. doi: 10.1007/s00213-017-4809-0
Vitor-Costa Okuno, N. M., Bortolotti, H., Bertollo, M., Boggio, P. S., Fregni, F., and Altimari, L. R. (2015). Improving cycling performance: Transcranial direct current stimulation increases time to exhaustion in cycling. PLoS ONE 10, e0144916. doi: 10.1371/journal.pone.0144916
Williams, P. S., Hoffman, R. L., and Clark, B. C. (2013). Preliminary evidence that anodal transcranial direct current stimulation enhances time to task failure of a sustained submaximal contraction. PLoS ONE 8, e81418. doi: 10.1371/journal.pone.0081418
Williamson, J. W. (2010). The relevance of central command for the neural cardiovascular control of exercise. Exp. Physiol. 95, 1043–1048. doi: 10.1113/expphysiol.2009.051870
Yu, T. H., Wu, Y. J., Chien, M. E., and Hsu, K. S. (2019). Transcranial direct current stimulation induces hippocampal metaplasticity mediated by brain-derived neurotrophic factor. Neuropharmacology 144, 358–367. doi: 10.1016/j.neuropharm.2018.11.012
Keywords: exercise performance, transcranial direct current stimulation (tDCS), central fatigue, mental fatigue, peripheral fatigue, endurance exercise, strength exercise
Citation: Jaberzadeh S and Zoghi M (2022) Transcranial Direct Current Stimulation Enhances Exercise Performance: A Mini Review of the Underlying Mechanisms. Front. Neuroergon. 3:841911. doi: 10.3389/fnrgo.2022.841911
Received: 22 December 2021; Accepted: 18 March 2022;
Published: 26 April 2022.
Edited by:
Wei-Peng Teo, Nanyang Technological University, SingaporeReviewed by:
Alexandre Moreira, University of São Paulo, BrazilCopyright © 2022 Jaberzadeh and Zoghi. This is an open-access article distributed under the terms of the Creative Commons Attribution License (CC BY). The use, distribution or reproduction in other forums is permitted, provided the original author(s) and the copyright owner(s) are credited and that the original publication in this journal is cited, in accordance with accepted academic practice. No use, distribution or reproduction is permitted which does not comply with these terms.
*Correspondence: Shapour Jaberzadeh, c2hhcG91ci5qYWJlcnphZGVoJiN4MDAwNDA7bW9uYXNoLmVkdQ==
†These authors have contributed equally to this work and share first authorship