- 1University of Bordeaux, Centre National de la Recherche Scientifique, Institut de Neurosciences Cognitives et Intégratives d'Aquitaine, Unité Mixte de Recherche 5287, Bordeaux, France
- 2Department of Health, Safety & Environment, Bordeaux Institute of Technology, Bordeaux, France
Central motor rhythm-generating networks controlling different functions are generally considered to operate mostly independently from one another, each controlling the specific behavioral task to which it is assigned. However, under certain physiological circumstances, central pattern generators (CPGs) can exhibit strong uni- or bidirectional interactions that render them closely inter-dependent. One of the best illustrations of such an inter-CPG interaction is the functional relationship that may occur between rhythmic locomotor and respiratory functions. It is well known that in vertebrates, lung ventilatory rates accelerate at the onset of physical exercise in order to satisfy the accompanying rapid increase in metabolism. Part of this acceleration is sustained by a coupling between locomotion and ventilation, which most often results in a periodic drive of the respiratory cycle by the locomotor rhythm. In terrestrial vertebrates, the likely physiological significance of this coordination is that it serves to reduce the mechanical interference between the two motor systems, thereby producing an energetic benefit and ultimately, enabling sustained aerobic activity. Several decades of studies have shown that locomotor-respiratory coupling is present in most species, independent of the mode of locomotion employed. The present article aims to review and discuss mechanisms engaged in shaping locomotor-respiratory coupling (LRC), with an emphasis on the role of sensory feedback inputs, the direct influences between CPG networks themselves, and finally on spinal cellular candidates that are potentially involved in the coupling of these two vital motor functions.
Coordination of locomotor and respiratory rhythms
Breathing is a continuous rhythmic motor activity that maintains the homeostasis of blood gas tension. Its level of activity is thus finely tuned when body homeostasis is challenged, such as during physical effort. First, at the onset of exercise, breathing frequency increases in order to match or to anticipate the subsequent increase in energy consumption (Krogh and Lindhard, 1913; Dejours, 1959). Secondly, and in the specific context of locomotor activity, walking or running at increasing speeds results in an increase in respiratory frequency rates. Although the breathing frequency can be progressively and continuously adjusted during locomotion through the combined activation of peripheral and central chemoreceptors and mechanoreceptors located in joints and muscles, data collected across the vertebrate phylum have shown that specific coordination patterns between locomotor and respiratory activities can also occur in several species (Stickford and Stickford, 2014). This phenomenon, reported for fish, birds and mammals (Bramble and Carrier, 1983) is referred to as locomotor-respiratory coupling (LRC). One typical example of LRC is the 1:1 coupling that preferentially emerges in most mammals, notably during gallop (Figure 1). In this case, each locomotor cycle is associated with a coordinated respiratory motor sequence. LRC can also be favored by the contribution of multiple factors including external stimuli such as auditory cues (Bernasconi and Kohl, 1993) and training (Bramble and Carrier, 1983). But in some species, the synchronization of breaths to strides can be either absent [for example in adult mice (Hérent et al., 2020)], or differently expressed, as is the case in running or flying birds. Therefore, many different harmonic couplings (2:1, 3:1, 4:1, 5:2, etc.) have been reported in birds during flight (Butler and Woakes, 1980; Funk et al., 1992b; Boggs et al., 1997), and also in running quadrupeds and humans (Bechbache and Duffin, 1977; Bramble and Carrier, 1983; Perségol et al., 1991; Banzett et al., 1992; Bernasconi and Kohl, 1993). Finally, and regardless of the different coupling patterns that may occur, LRC has been suggested to produce a number of important physiological benefits by reducing biomechanical interferences between the respiratory and locomotor effector systems in order to assist breathing from forces produced during locomotion (Bramble and Carrier, 1983; Brown, 1999) or by decreasing the energetic cost of lung ventilation (Funk et al., 1992a). It has also been proposed that in running humans, LRC may reduce work in breathing by minimizing fatigue in the respiratory muscles that are essential for endurance aerobic activity (Daley et al., 2013).
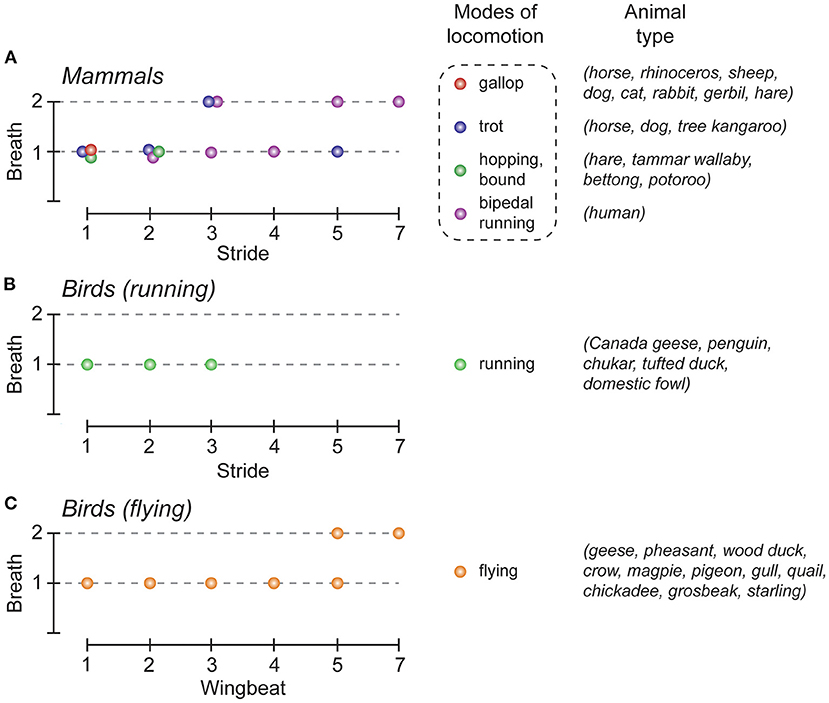
Figure 1. Locomotor-respiratory coupling (LRC) ratios in the animal kingdom. (A–C), Graphical representation of the most common LRC ratios observed in mammals (A), and birds (B,C). The colored dots indicate the LRC ratios commonly observed, depending on the mode of locomotion used, in mammals (red, gallop; blue, trot; green, hopping/bond; purple, bipedal running), and birds (green, running; orange, flying). Note that for the same gait either one (e.g. gallop) or several (e.g., trot) LRC can be observed. Adapted from Boggs (2002); Stickford and Stickford (2014).
Biomechanical mechanisms
LRC can be ascribed to several underlying, and possibly redundant, mechanisms, the respective contributions of which may vary between animal species and might operate in parallel. Among these mechanisms, the biomechanical characteristics of the individual can be involved. Firstly, the “visceral piston” theory has been proposed to explain the 1:1 LRC observed in galloping quadrupeds. This idea proposes that the mechanical forward/backward movement of the internal organs during galloping physically affects movement of the diaphragm and contributes to the emergence of a 1:1 coupling between the locomotor and respiratory cycles (Bramble and Carrier, 1983; Young et al., 1992; Bramble and Jenkins, 1993). A similar mechanism could also operate in hopping animals that also express such coupling. Secondly, 1:1 LRC could result from changes in volume and pressure within the rib cage that occur when ground contact is made in each cycle. In galloping mammals, for example, the impact of the forelimbs with the ground results in an external loading on the thorax that compresses the rib cage. The resultant decrease in thoracic volume and the accompanying increase in pulmonary pressure thereby facilitates exhalation (Bramble and Carrier, 1983; Boggs, 2002). Finally, it has been postulated that 1:1 coupling could also emerge from changes in volume and pressure within the thoracic cavity associated with dorsiflexion of the lumbo-pelvic region. Mainly observed in fast running mammals such as the cheetah, the alternating lumbosacral flexions/extensions contribute to the rhythmic displacement of the visceral mass, which in turn facilitates exhalation and inspiration, respectively (Bramble, 1989; Stickford and Stickford, 2014).
Neurogenic mechanisms
The above biomechanical mechanisms cannot explain either the emergence of harmonic LRC (e.g., 2:1, 3:2, 4:1, etc.) observed in birds and bipeds, or the progressive changes in the breathing rhythm that is observed during or even before the onset of locomotor episodes (Tobin et al., 1986; Gravel et al., 2007), implying that other mechanisms are also involved (Viala, 1997). On the one hand, neurogenic coupling processes, mainly involving feedforward mechanisms (Gariépy et al., 2010, 2012), have also been proposed to play a critical role in the regulation of breathing frequency during exercise. Indeed, feedforward pathways originating from the hypothalamus (Eldridge et al., 1981; Horn and Waldrop, 1998), the mesencephalic locomotor region (Horn and Waldrop, 1998; Ryczko and Dubuc, 2013; Opris et al., 2019), or medullary structures (Romaniuk et al., 1994), are likely to simultaneously influence the level of excitability of both the respiratory and locomotor rhythm generating networks. On the other hand, direct interactions between neuronal groups controlling locomotor and respiratory activities can also account for LRC. For instance, generation of the rhythmic locomotor and respiratory motor patterns both rely on so-called central pattern generator networks (CPG) located in the spinal cord (Viala and Vidal, 1978; Yamaguchi, 1992; Cazalets et al., 1995; Kiehn and Kjaerulff, 1996; Ballion et al., 2001; Kiehn, 2016) and the brainstem (Smith et al., 1991; Gray et al., 2001; Del Negro et al., 2018), respectively. Central pathway-mediated interactions between the locomotor and respiratory CPGs have been described in different animal models ranging from decerebrated paralyzed rabbits (Viala et al., 1987; Perségol et al., 1988; Corio et al., 1993), cats (Kawahara et al., 1989a,b), birds (Funk et al., 1992a,b), and ex vivo preparations of rodent central nervous systems (Morin and Viala, 2002; Le Gal et al., 2020). In all cases, experimental data have clearly indicated that the spinal locomotor CPGs can adjust the level of activity of the respiratory network through purely central mechanisms. In the following sections of this review, we will focus on the neurogenic mechanisms that subserve LRC (Figure 2A), or in a different manner, the ability of the locomotor neural system to regulate breathing frequency (Figure 2B).
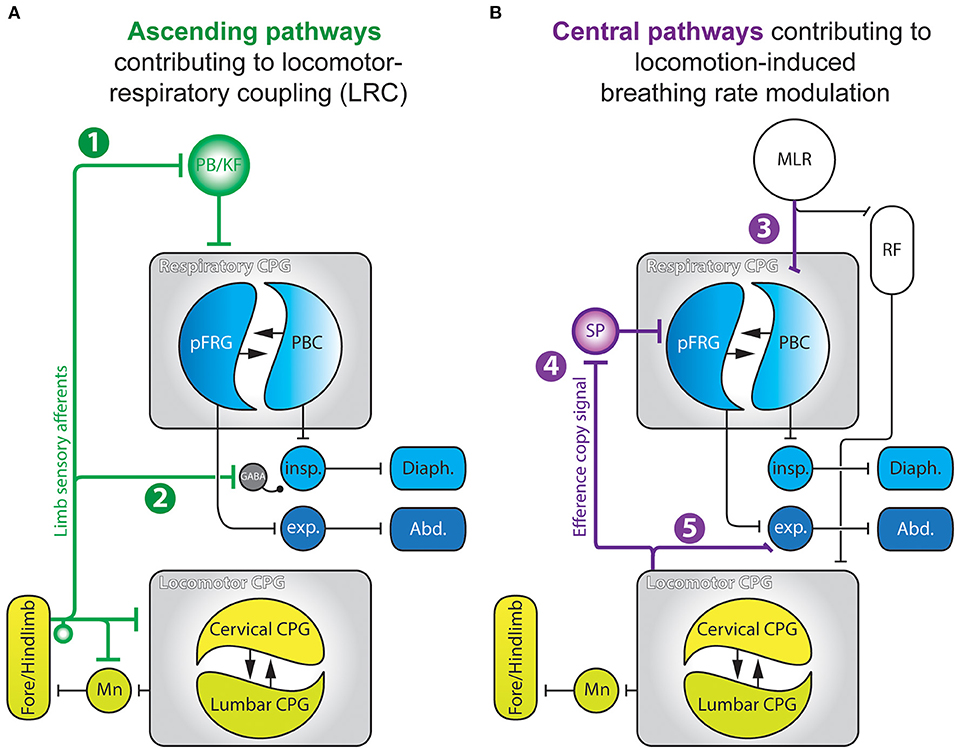
Figure 2. Summary schematics of the neurogenic pathways contributing to locomotor-respiratory coupling (A) and locomotion-derived breathing rate modulation (B). 1, Ascending projections from limb sensory afferents to the PB/KF complex; 2, Pathway enabling spinal proprioceptive afferents to modulate the excitability of spinal inspiratory motoneurons via a GABA-releasing relay; 3, Parallel descending drives from the MLR to the spinal locomotor CPG and to the PBC; 4, Efference copy signals conveyed by ascending glutamatergic projections from the spinal locomotor CPG to respiratory CPG networks through an SP-releasing relay; 5, Propriospinal pathway enabling the locomotor CPG to modulate activity of spinal expiratory neurons. Abd., abdominal muscles; CPG, central pattern generator; Diaph., diaphragm; exp., expiratory neurons; insp., inspiratory neurons; MLR, mesencephalic locomotor region; Mn, motoneurons; PBC, preBötzinger Complex; PB/KF, parabrachial/Kölliker-Fuse nucleus; pFRG, parafacial respiratory group; RF, reticular formation; SP, substance P. Adapted from Morin and Viala (2002); Giraudin et al. (2008, 2012); Le Gal et al. (2016, 2020).
Peripheral feedback signals for locomotor-respiratory coupling
In different species, passive mobilization of the limbs modulates breathing activity and, in some cases, is able to entrain the breathing rhythm in a 1:1 manner (Bramble and Carrier, 1983; Viala, 1997; Boggs, 2002). During locomotion, limb sensory receptors are rhythmically activated (Prochazka and Gorassini, 1998a,b) and play an important role in the transition between the swing and stance phases of each movement cycle (Orlovsky et al., 1999; Duysens et al., 2000). This afferent control of the locomotor pattern timing is mediated through a direct feedback action on the spinal locomotor CPGs themselves (Conway et al., 1987; Kiehn et al., 1992; Sqalli-Houssaini et al., 1993; Perreault et al., 1995; Iizuka et al., 1997; Pearson et al., 1998; Schomburg et al., 1998; Juvin et al., 2012). Interestingly, fore and hindlimb sensory afferents also have access to the respiratory CPG where they are also likely to influence the latter's operation (Figure 2A) (Iscoe and Polosa, 1976; Bramble and Carrier, 1983; Palisses et al., 1988; Funk et al., 1992a). Indeed, passively-activated or electrically-stimulated limb sensory pathways are able to reset and entrain respiratory activity in a 1:1 manner in a large array of species and experimental preparations (Iscoe and Polosa, 1976; Bramble and Carrier, 1983; Funk et al., 1992a; Morin and Viala, 2002; Potts et al., 2005; Giraudin et al., 2008, 2012). For instance, in an in situ preparation of young rats (Potts et al., 2005), electrical stimulation of somatic afferents can reset the respiratory pattern cycle through a mechanism that does not rely on an intercalated intraspinal reflex-like mechanism, but rather via a direct action on the respiratory CPG itself. This was indicated by the finding that such resetting induced by somatic afferents involves the generation of a complete respiratory-like pattern, comprising the pre-inspiratory, inspiratory and expiratory phases of each cycle (Giraudin et al., 2008). In addition, repeated sensory stimulations are able to entrain the respiratory rhythm in a 1:1 manner, albeit within a specific range of stimulation frequencies (from 0.2 up to 0.4 Hz), which would not be expected from the involvement of a reflex-like mechanism (Potts et al., 2005). Similar results were reported from an ex vivo brainstem/spinal cord preparation of the newborn rat, where rhythmical electrical stimulation of spinal dorsal roots resets and entrains the ongoing respiratory rhythm over a limited range of stimulus frequencies [0.125 up to 0.25 Hz, (Morin and Viala, 2002)]. Moreover, this entrainment seems to be phase-dependent, since respiratory rhythm resetting appears to be facilitated when activation of limb somatic afferents are applied during expiration (Morin and Viala, 2002; Potts et al., 2005), producing a significant increase in the firing rate of medullary expiratory neurons (Potts et al., 2005). When stimulated, therefore, the somatic afferents cause initiation of inspiration via the initial activation of expiratory neurons. This respiratory phase transition, termed an “inspiratory on-switching” mechanism operating in the ponto-medullary region, relies strongly on the involvement of the parabrachial/Kölliker-Fuse (PB/KF) nucleus (see Figure 2A, pathway 1) (Cohen, 1971; Miura and Takayama, 1991; Chamberlin and Saper, 1994; Dutschmann and Herbert, 2006; Mörschel and Dutschmann, 2009). Indeed, several findings have indicated that the integrity of the PB/KF nucleus is necessary for respiratory entrainment during LRC. Firstly, calcium-imaging revealed that a cell population located within the PB/KF elicits calcium transients in response to individual spinal dorsal root stimulations (Giraudin et al., 2012). However, a reversible pharmacological inhibition of the PB/KF complex (Potts et al., 2005) or its bilateral lesion (Giraudin et al., 2012), prevent both the resetting and entrainment of the respiratory activity induced by limb sensory pathway stimulation. Although the exact role of the PB/KF complex in LRC remains open to debate, this pontine relay could be of primary importance during locomotion in gating out inappropriately timed sensory information resulting from antagonistic (flexor/extensor) movements of each of the four limbs. Such filtering would therefore ensure that the remaining ascending signals provide effective descending commands sent to medullary interneurons engaged in the cycle-by-cycle regulation of respiratory rhythmogenesis. The excitability of medullary respiratory neurons (Potts et al., 2005) and, ultimately, phrenic cervical motoneurons (Figure 2A, pathway 2) (Morin and Viala, 2002) would be modulated in such a way as to “prepare” these cell ensembles for responding preferentially to the premature respiratory command triggered by the spinal sensory afferent activation.
Finally, the exact nature and relative contributions of the different sensory afferent populations to the operation of the respiratory CPGs still remain uncertain, but virtually all types of fibers could be involved (Bruce et al., 2019). So far, small myelinated and non-myelinated fibers from groups III and IV muscle afferents have been found to contribute to the modulation of respiratory activity (Senapati, 1966; Decherchi et al., 2007; Amann et al., 2010). In contrast, the contribution of Ia and Ib primary afferents still remains a matter of debate in light of contradictory observations reported in the literature, with studies supporting (Bessou et al., 1959; Koizumi et al., 1961; Senapati, 1966; Carcassi et al., 1983; Morin and Viala, 2002; Potts et al., 2005; Giraudin et al., 2008, 2012) and others excluding their contribution (Waldrop et al., 1984). Further work is therefore needed to unravel whether these discrepancies arise from significant differences between experimental protocols or to species differences in the relative contributions of the different afferent populations.
Central feedforward influences for breathing rate modulation
Moving at low or moderate pace triggers an elevation of the breathing frequency which is not directly coupled to the locomotor cycle frequency (Kawahara et al., 1989a; Hérent et al., 2020), especially in humans where LRC is observed during running but only sporadically during walking (Hill et al., 1988). This is also the case in the decerebrated cat, for instance, during locomotor episodes induced by stimulation of the mesencephalic locomotor region (MLR). At moderate locomotor pace, such as during trotting, the frequency of breathing activity increases, but LRC emerges only at the most rapid locomotor pace, especially during galloping (Kawahara et al., 1989a). The elevation of the ventilatory rate observed at the initiation of locomotion, or even before the onset of the physical effort (Tobin et al., 1986; Gravel et al., 2007) cannot only rely on sensory feedback mechanisms, including peripheral chemoreception, since blood gas homeostasis is maintained at the onset of exercise (Mateika and Duffin, 1995; Haouzi et al., 1997). On this basis, therefore, the anticipatory respiratory response must rely on central feedforward mechanisms that are different from those involved in direct locomotor–respiratory coupling (Figure 2B) (Tobin et al., 1986; Bell, 2006; Gravel et al., 2007; Gariépy et al., 2010). Several brain regions involved in the initiation of locomotor episodes, including the hypothalamus (Eldridge et al., 1981; DiMarco et al., 1983; Waldrop and Iwamoto, 2006), the MLR (Gariépy et al., 2012), and the ponto-medullary reticular formation have been shown to produce modulation of the breathing frequency. In the unanesthetized decorticated cat, electrical stimulation of the sub-thalamic locomotor region (SLR) triggers episodes of locomotion. Significantly, the initiation of locomotor movement is preceded by a rapid modulation of the breathing frequency, with breathing amplitude correlated to the intensity of the locomotor activity (Eldridge et al., 1981). These anticipatory responses were also observed in the paralyzed cat where phasic sensory feedback from limb afferents was lacking due to the absence of muscle contractions (Eldridge et al., 1981). In the cat and the lamprey, stimulation of the MLR also triggers a rapid elevation of respiratory rate concomitant with the initiation of locomotor activity (Figure 2B, pathway 3) (Kawahara et al., 1989b; Gariépy et al., 2012). In the lamprey model furthermore, these authors reported that the MLR projects directly to the respiratory CPG network, and that stimulations of the MLR that fail to trigger locomotion can nonetheless increase the respiratory frequency. Thus, an activation of supra-spinal locomotor structures is capable of eliciting an integrated response in both the respiratory and locomotor systems. Such a feedforward mechanism would serve to adapt ongoing respiratory activity in order to anticipate and minimize the metabolic changes induced by locomotion. However, the nature and relative contribution of the different locomotor regions in the feedforward regulation of the respiratory network remains to be fully described.
Other CNS regions are also involved in the modulation of the respiratory CPG network, especially the spinal cord where the locomotor CPGs controlling the limbs are located. In the ex vivo neonatal rat brainstem-spinal cord preparation, both locomotor and respiratory motor outputs can be recorded from specific spinal motor roots (Smith and Feldman, 1987). In this isolated preparation, the motor commands normally sent to the limb muscle effectors were monitored by electroneurograms, and the recorded activities referred to as “fictive” respiration and locomotion due to the inevitable absence of effective movement. In this preparation, we have shown that the specific activation of the spinal locomotor CPGs induces a rapid increase in the frequency of spontaneous fictive respiration (Morin and Viala, 2002; Le Gal et al., 2014). Similar to the activation of the supra-spinal locomotor regions, a slowly increasing pharmacological stimulation of the spinal locomotor CPGs evokes an increase in fictive respiration frequency that precedes the onset of fictive locomotion (Le Gal et al., 2020). Moreover, both the lumbar and cervical locomotor CPGs controlling the hindlimbs and forelimbs, respectively (Cazalets et al., 1995; Ballion et al., 2001) can modulate respiratory activity (Le Gal et al., 2020), with this ascending central influence being integrated at the level of the parafacial respiratory group (pFRG) via an intercalated substance P-releasing relay (Figure 2B, pathway 4). Both the bilateral removal of the pFRG or the pharmacological blockade of brainstem substance P receptors abolishes the ascending modulation of the respiratory network by the spinal locomotor CPGs (Le Gal et al., 2014). However, blocking spinal thoracic circuitry interposed between the brainstem respiratory network and the lumbar locomotor CPG does not affect the ascending modulatory action, indicating the involvement of long ascending projection pathways originating in the lumbar spinal area (Le Gal et al., 2020). In order to identify the nature of the neurons involved in this modulation, we performed optogenetic stimulation of glutamatergic Vglut2-positive neurons previously shown to participate in the generation of fictive locomotion (Hägglund et al., 2010). Using this stimulus method, we found a concomitant increase in the rate of fictive respiration (Le Gal et al., 2020). Moreover, spinal V2 interneurons themselves are likely to be a source of the long ascending projections to the respiratory CPG, since firstly, they are known to interconnect the lumbar and cervical locomotor networks to strengthen interlimb coordination (Ruder et al., 2016), and second, they are rhythmically active during fictive locomotion (Dougherty and Kiehn, 2010; Zhong et al., 2010; Dougherty et al., 2013). Additionally, a subset of glutamatergic V2 neurons (V2a) have been found to project from the spinal cord to the brainstem where they convey an internal efference copy of the ongoing motor command sent to the forelimb muscles during skilled reaching (Brockett et al., 2013; Azim et al., 2014).
Finally, the spinal locomotor CPGs also influence respiratory motor output at the level of the spinal cord itself. In the isolated brainstem–spinal cord preparation from neonatal rat, the activity of expiratory, but not inspiratory, motoneurons and interneurons is rhythmically modulated during fictive locomotion (Figure 2B, pathway 5) (Le Gal et al., 2016). Similar results were obtained when recording simultaneously from the spinal ventral roots, motor nerves and limb and trunk muscles in semi-intact preparation of newborn rat (Iizuka et al., 2022). From a functional perspective, this latter observation is relevant to the existence of bi-functional muscles, as is the case for the abdominal muscles, which participate in both respiration and locomotion (Saunders et al., 2004; Iizuka et al., 2022). Indeed, axial musculature, which is involved in expiration in mammals (Koterba et al., 1988; De Troyer et al., 1990; Deban and Carrier, 2002), is also engaged in locomotion (Grillner et al., 1978; Puckree et al., 1998; Deban and Carrier, 2002; Reilly et al., 2009). It has been proposed that this influence of the lumbar locomotor generator on expiratory neurons that control trunk movements could induce bending of the spine, thereby generating a forward displacement of the pelvis, which is known to facilitate exhalation.
Concluding remarks
The spinal and supraspinal circuits involved in the interactions between locomotor and respiratory functions are distributed over several CNS regions, making their overall understanding a challenging task. Clearly, the neural networks and pathways described here represent only a portion of the circuitry involved and, besides mechanical constraints and neurogenic control processes, metabolic conditions also influence locomotor-respiratory coordination. Although it is very likely that the experimental observations thus far obtained using reduced CNS preparations have only partially revealed the mechanisms engaged in the coordination between the respiratory and locomotor networks, these data have nonetheless provided important insights into the functional relationships between these two mammalian motor functions. Already initiated in the newborn rodent (Le Gal et al., 2020), efforts to identify the cell types involved in this coupling should now be further pursued, both by using ex vivo preparations but also in the behaving animal, in particular by taking advantage of the development of transgenic models and advanced experimental tools to monitor and analyze motor activities. Ultimately, a precise knowledge of the neural mechanisms and cellular pathways involved in the coordination between these two vital behaviors should have important implications for developing rehabilitation procedures after an incomplete spinal cord injury (Sutor et al., 2022).
Author contributions
LJ, EC, GB, MT-B, and DM wrote and edited the manuscript text. All authors contributed to the article and approved the submitted version.
Funding
This work was supported by an Equipe FRM (Fondation pour la Recherche Médicale) funding (DEQ20170336764) to MT-B.
Acknowledgments
We warmly thank Dr. John Simmers for valuable comments on the manuscript and for English revision.
Conflict of interest
The authors declare that the research was conducted in the absence of any commercial or financial relationships that could be construed as a potential conflict of interest.
Publisher's note
All claims expressed in this article are solely those of the authors and do not necessarily represent those of their affiliated organizations, or those of the publisher, the editors and the reviewers. Any product that may be evaluated in this article, or claim that may be made by its manufacturer, is not guaranteed or endorsed by the publisher.
References
Amann, M., Blain, G. M., Proctor, L. T., Sebranek, J. J., Pegelow, D. F., Dempsey, J. A., et al. (2010). Group III and IV muscle afferents contribute to ventilatory and cardiovascular response to rhythmic exercise in humans. J. Appl. Physiol. Bethesda Md. 109, 966–976. doi: 10.1152/japplphysiol.00462.2010
Azim, E., Jiang, J., Alstermark, B., and Jessell, T. M. (2014). Skilled reaching relies on a V2a propriospinal internal copy circuit. Nature 508, 357–363. doi: 10.1038/nature13021
Ballion, B., Morin, D., and Viala, D. (2001). Forelimb locomotor generators and quadrupedal locomotion in the neonatal rat. Eur. J. Neurosci. 14, 1727–1738. doi: 10.1046/j.0953-816x.2001.01794.x
Banzett, R. B., Mead, J., Reid, M. B., and Topulos, G. P. (1992). Locomotion in men has no appreciable mechanical effect on breathing. J. Appl. Physiol. 72, 1922–1926. doi: 10.1152/jappl.1992.72.5.1922
Bechbache, R. R., and Duffin, J. (1977). The entrainment of breathing frequency by exercise rhythm. J. Physiol. 272, 553–561. doi: 10.1113/jphysiol.1977.sp012059
Bell, H. J. (2006). Respiratory control at exercise onset: an integrated systems perspective. Respir. Physiol. Neurobiol. 152, 1–15. doi: 10.1016/j.resp.2006.02.005
Bernasconi, P., and Kohl, J. (1993). Analysis of co-ordination between breathing and exercise rhythms in man. J. Physiol. 471, 693–706. doi: 10.1113/jphysiol.1993.sp019923
Bessou, P., Dejours, P., and Laporte, Y. (1959). Reflex ventilatory action of largediameter afferent fibers of muscular origin in the cat. J. Physiol. 51, 400–401.
Boggs, D., Seveyka, J., Kilgore, D., and Dial, K. (1997). Coordination of respiratory cycles with wingbeat cycles in the black-billed magpie (Pica pica). J. Exp. Biol. 200, 1413–1420. doi: 10.1242/jeb.200.9.1413
Boggs, D. F. (2002). Interactions between locomotion and ventilation in tetrapods. Comput. Biochem. Physiol. Part A Mol. Integr. Physiol. 133, 269–288. doi: 10.1016/S1095-6433(02)00160-5
Bramble, D. M. (1989). Axial-appendicular dynamics and the integration of breathing and gait in mammals. Am. Zool. 29, 171–186. doi: 10.1093/icb/29.1.171
Bramble, D. M., and Carrier, D. R. (1983). Running and breathing in mammals. Science 219, 251–256. doi: 10.1126/science.6849136
Bramble, D. M., and Jenkins, F. A. (1993). Mammalian locomotor-respiratory integration: implications for diaphragmatic and pulmonary design. Science 262, 235–240. doi: 10.1126/science.8211141
Brockett, E. G., Seenan, P. G., Bannatyne, B. A., and Maxwell, D. J. (2013). Ascending and descending propriospinal pathways between lumbar and cervical segments in the rat: evidence for a substantial ascending excitatory pathway. Neuroscience 240, 83–97. doi: 10.1016/j.neuroscience.2013.02.039
Brown, R. E. (1999). “Avian respiration: isolation of ventilation and flight,” in Proceedings of the 22nd International Ornithological Congress. NJ Adams and RH Slotow, Eds. (BirdLife South Africa: Johannesburg) 114–123.
Bruce, R. M., Jolley, C., and White, M. J. (2019). Control of exercise hyperpnoea: Contributions from thin-fibre skeletal muscle afferents. Exp. Physiol. 104, 1605–1621. doi: 10.1113/EP087649
Butler, P. J., and Woakes, A. J. (1980). Heart rate, respiratory frequency and wing beat frequency of free flying barnacle geese, Branta leucopsis. J. Exp. Biol. 85, 213–226. doi: 10.1242/jeb.85.1.213
Carcassi, A. M., Concu, A., Decandia, M., Onnis, M., Orani, G. P., Piras, M. B., et al. (1983). Respiratory responses to stimulation of large fibers afferent from muscle receptors in cats. Pflugers Arch. 399, 309–314. doi: 10.1007/BF00652758
Cazalets, J. R., Borde, M., and Clarac, F. (1995). Localization and organization of the central pattern generator for hindlimb locomotion in newborn rat. J. Neurosci. Off. J. Soc. Neurosci. 15, 4943–4951. doi: 10.1523/JNEUROSCI.15-07-04943.1995
Chamberlin, N. L., and Saper, C. B. (1994). Topographic organization of respiratory responses to glutamate microstimulation of the parabrachial nucleus in the rat. J. Neurosci. Off. J. Soc. Neurosci. 14, 6500–6510. doi: 10.1523/JNEUROSCI.14-11-06500.1994
Cohen, M. I. (1971). Switching of the respiratory phases and evoked phrenic responses produced by rostral pontine electrical stimulation. J. Physiol. 217, 133–158. doi: 10.1113/jphysiol.1971.sp009563
Conway, B. A., Hultborn, H., and Kiehn, O. (1987). Proprioceptive input resets central locomotor rhythm in the spinal cat. Exp. Brain Res. 68, 643–656. doi: 10.1007/BF00249807
Corio, M., Palisses, R., and Viala, D. (1993). Origin of the central entrainment of respiration by locomotion facilitated by MK 801 in the decerebrate rabbit. Exp. Brain Res. 95, 84–90. doi: 10.1007/BF00229657
Daley, M. A., Bramble, D. M., and Carrier, D. R. (2013). Impact loading and locomotor-respiratory coordination significantly influence breathing dynamics in running humans. PLoS ONE 8, e70752. doi: 10.1371/journal.pone.0070752
De Troyer, D., Estenne, A., Ninane, M., Van Gansbeke, V. D., and Gorini, M. (1990). Transversus abdominis muscle function in humans. J. Appl. Physiol. Bethesda Md. 68, 1010–1016. doi: 10.1152/jappl.1990.68.3.1010
Deban, S. M., and Carrier, D. R. (2002). Hypaxial muscle activity during running and breathing in dogs. J. Exp. Biol. 205, 1953–1967. doi: 10.1242/jeb.205.13.1953
Decherchi, P., Dousset, E., and Jammes, Y. (2007). Respiratory and cardiovascular responses evoked by tibialis anterior muscle afferent fibers in rats. Exp. Brain Res. 183, 299–312. doi: 10.1007/s00221-007-1044-7
Dejours, P. (1959). Regulation of ventilation during muscular exercise in man. J. Physiol. 51 163–261.
Del Negro, C. A., Funk, G. D., and Feldman, J. L. (2018). Breathing matters. Nat. Rev. Neurosci. 19, 351–367. doi: 10.1038/s41583-018-0003-6
DiMarco, A. F., Romaniuk, J. R., Von Euler, C., and Yamamoto, Y. (1983). Immediate changes in ventilation and respiratory pattern associated with onset and cessation of locomotion in the cat. J. Physiol. 343, 1–16. doi: 10.1113/jphysiol.1983.sp014878
Dougherty, K. J., and Kiehn, O. (2010). Firing and cellular properties of V2a interneurons in the rodent spinal cord. J. Neurosci. Off. J. Soc. Neurosci. 30, 24–37. doi: 10.1523/JNEUROSCI.4821-09.2010
Dougherty, K. J., Zagoraiou, L., Satoh, D., Rozani, I., Doobar, S., Arber, S., et al. (2013). Locomotor rhythm generation linked to the output of spinal shox2 excitatory interneurons. Neuron 80, 920–933. doi: 10.1016/j.neuron.2013.08.015
Dutschmann, M., and Herbert, H. (2006). The Kölliker-Fuse nucleus gates the postinspiratory phase of the respiratory cycle to control inspiratory off-switch and upper airway resistance in rat. Eur. J. Neurosci. 24, 1071–1084. doi: 10.1111/j.1460-9568.2006.04981.x
Duysens, J., Clarac, F., and Cruse, H. (2000). Load-regulating mechanisms in gait and posture: comparative aspects. Physiol. Rev. 80, 83–133. doi: 10.1152/physrev.2000.80.1.83
Eldridge, F. L., Millhorn, D. E., and Waldrop, T. G. (1981). Exercise hyperpnea and locomotion: parallel activation from the hypothalamus. Science 211, 844–846. doi: 10.1126/science.7466362
Funk, G. D., Milsom, W. K., and Steeves, J. D. (1992a). Coordination of wingbeat and respiration in the Canada goose. I. Passive wing flapping. J. Appl. Physiol. Bethesda Md. 73, 1014–1024. doi: 10.1152/jappl.1992.73.3.1014
Funk, G. D., Steeves, J. D., and Milsom, W. K. (1992b). Coordination of wingbeat and respiration in birds. II. “Fictive” flight. J. Appl. Physiol. Bethesda Md. 73, 1025–1033. doi: 10.1152/jappl.1992.73.3.1025
Gariépy, J.-F., Missaghi, K., Chevallier, S., Chartré, S., Robert, M., Auclair, F., et al. (2012). Specific neural substrate linking respiration to locomotion. Proc. Natl. Acad. Sci. U.S.A. 109, E84–92. doi: 10.1073/pnas.1113002109
Gariépy, J.-F., Missaghi, K., and Dubuc, R. (2010). The interactions between locomotion and respiration. Prog. Brain Res. 187, 173–188. doi: 10.1016/B978-0-444-53613-6.00012-5
Giraudin, A., Cabirol-Pol, M.-J., Simmers, J., and Morin, D. (2008). Intercostal and abdominal respiratory motoneurons in the neonatal rat spinal cord: spatiotemporal organization and responses to limb afferent stimulation. J. Neurophysiol. 99, 2626–2640. doi: 10.1152/jn.01298.2007
Giraudin, A., Le Bon-Jégo, M., Cabirol, M.-J., Simmers, J., and Morin, D. (2012). Spinal and pontine relay pathways mediating respiratory rhythm entrainment by limb proprioceptive inputs in the neonatal rat. J. Neurosci. Off. J. Soc. Neurosci. 32, 11841–11853. doi: 10.1523/JNEUROSCI.0360-12.2012
Gravel, J., Brocard, F., Gariépy, J.-F., Lund, J. P., and Dubuc, R. (2007). Modulation of respiratory activity by locomotion in lampreys. Neuroscience 144, 1120–1132. doi: 10.1016/j.neuroscience.2006.10.019
Gray, P. A., Janczewski, W. A., Mellen, N., McCrimmon, D. R., and Feldman, J. L. (2001). Normal breathing requires preBötzinger complex neurokinin-1 receptor-expressing neurons. Nat. Neurosci. 4, 927–930. doi: 10.1038/nn0901-927
Grillner, S., Nilsson, J., and Thorstensson, A. (1978). Intra-abdominal pressure changes during natural movements in man. Acta Physiol. Scand. 103, 275–283. doi: 10.1111/j.1748-1716.1978.tb06215.x
Hägglund, M., Borgius, L., Dougherty, K. J., and Kiehn, O. (2010). Activation of groups of excitatory neurons in the mammalian spinal cord or hindbrain evokes locomotion. Nat. Neurosci. 13, 246–252. doi: 10.1038/nn.2482
Haouzi, P., Hirsch, J. J., Marchal, F., and Huszczuk, A. (1997). Ventilatory and gas exchange response during walking in severe peripheral vascular disease. Respir. Physiol. 107, 181–190. doi: 10.1016/S0034-5687(96)02508-X
Hérent, C., Diem, S., Fortin, G., and Bouvier, J. (2020). Absent phasing of respiratory and locomotor rhythms in running mice. Elife 9, e61919. doi: 10.7554/eLife.61919.sa2
Hill, A. R., Adams, J. M., Parker, B. E., and Rochester, D. F. (1988). Short-term entrainment of ventilation to the walking cycle in humans. J. Appl. Physiol. Bethesda Md. 65, 570–578. doi: 10.1152/jappl.1988.65.2.570
Horn, E. M., and Waldrop, T. G. (1998). Suprapontine control of respiration. Respir. Physiol. 114, 201–211. doi: 10.1016/S0034-5687(98)00087-5
Iizuka, M., Kiehn, O., and Kudo, N. (1997). Development in neonatal rats of the sensory resetting of the locomotor rhythm induced by NMDA and 5-HT. Exp. Brain Res. 114, 193–204. doi: 10.1007/PL00005628
Iizuka, M., Ruangkittisakul, A., and Ballanyi, K. (2022). Expiratory abdominal muscle nerve is active at flexor phase, while inspiratory phrenic nerve is not active during locomotion evoked by 5-HT and NMDA in the neonatal rat. Neurosci. Res. 174, 9–18. doi: 10.1016/j.neures.2021.07.004
Iscoe, S., and Polosa, C. (1976). Synchronization of respiratory frequency by somatic afferent stimulation. J. Appl. Physiol. 40, 138–148. doi: 10.1152/jappl.1976.40.2.138
Juvin, L., Le Gal, J.-P., Simmers, J., and Morin, D. (2012). Cervicolumbar coordination in mammalian quadrupedal locomotion: role of spinal thoracic circuitry and limb sensory inputs. J. Neurosci. Off. J. Soc. Neurosci. 32, 953–965. doi: 10.1523/JNEUROSCI.4640-11.2012
Kawahara, K., Kumagai, S., Nakazono, Y., and Miyamoto, Y. (1989a). Coupling between respiratory and stepping rhythms during locomotion in decerebrate cats. J. Appl. Physiol. Bethesda Md. 67, 110–115. doi: 10.1152/jappl.1989.67.1.110
Kawahara, K., Nakazono, Y., Yamauchi, Y., and Miyamoto, Y. (1989b). Coupling between respiratory and locomotor rhythms during fictive locomotion in decerebrate cats. Neurosci. Lett. 103, 326–330. doi: 10.1016/0304-3940(89)90121-3
Kiehn, O. (2016). Decoding the organization of spinal circuits that control locomotion. Nat. Rev. Neurosci. 17, 224–238. doi: 10.1038/nrn.2016.9
Kiehn, O., Iizuka, M., and Kudo, N. (1992). Resetting from low threshold afferents of N-methyl-D-aspartate-induced locomotor rhythm in the isolated spinal cord-hindlimb preparation from newborn rats. Neurosci. Lett. 148, 43–46. doi: 10.1016/0304-3940(92)90800-M
Kiehn, O., and Kjaerulff, O. (1996). Spatiotemporal characteristics of 5-HT and dopamine-induced rhythmic hindlimb activity in the in vitro neonatal rat. J. Neurophysiol. 75, 1472–1482. doi: 10.1152/jn.1996.75.4.1472
Koizumi, K., Ushiyama, J., and Brooks, C. M. (1961). Muscle afferents and activity of respiratory neurons. Am. J. Physiol. 200, 679–684. doi: 10.1152/ajplegacy.1961.200.4.679
Koterba, A. M., Kosch, P. C., Beech, J., and Whitlock, T. (1988). Breathing strategy of the adult horse (Equus caballus) at rest. J. Appl. Physiol. Bethesda Md. 64, 337–346. doi: 10.1152/jappl.1988.64.1.337
Krogh, A., and Lindhard, J. (1913). The regulation of respiration and circulation during the initial stages of muscular work. J. Physiol. 47, 112–136. doi: 10.1113/jphysiol.1913.sp001616
Le Gal, J.-P., Colnot, E., Cardoit, L., Bacqué-Cazenave, J., Thoby-Brisson, M., Juvin, L., et al. (2020). Modulation of respiratory network activity by forelimb and hindlimb locomotor generators. Eur. J. Neurosci. 52, 3181–3195. doi: 10.1111/ejn.14717
Le Gal, J.-P., Juvin, L., Cardoit, L., and Morin, D. (2016). Bimodal respiratory-locomotor neurons in the neonatal rat spinal cord. J. Neurosci. Off. J. Soc. Neurosci. 36, 926–937. doi: 10.1523/JNEUROSCI.1825-15.2016
Le Gal, J.-P., Juvin, L., Cardoit, L., Thoby-Brisson, M., and Morin, D. (2014). Remote control of respiratory neural network by spinal locomotor generators. PLoS ONE 9, e89670. doi: 10.1371/journal.pone.0089670
Mateika, J. H., and Duffin, J. (1995). A review of the control of breathing during exercise. Eur. J. Appl. Physiol. 71, 1–27. doi: 10.1007/BF00511228
Miura, M., and Takayama, K. (1991). Circulatory and respiratory responses to glutamate stimulation of the lateral parabrachial nucleus of the cat. J. Auton. Nerv. Syst. 32, 121–133. doi: 10.1016/0165-1838(91)90062-8
Morin, D., and Viala, D. (2002). Coordinations of locomotor and respiratory rhythms in vitro are critically dependent on hindlimb sensory inputs. J. Neurosci. Off. J. Soc. Neurosci. 22, 4756–4765. doi: 10.1523/JNEUROSCI.22-11-04756.2002
Mörschel, M., and Dutschmann, M. (2009). Pontine respiratory activity involved in inspiratory/expiratory phase transition. Philos. Trans. R. Soc. Lond., B. Biol. Sci. 364, 2517–2526. doi: 10.1098/rstb.2009.0074
Opris, I., Dai, X., Johnson, D. M. G., Sanchez, F. J., Villamil, L. M., Xie, S., et al. (2019). Activation of brainstem neurons during mesencephalic locomotor region-evoked locomotion in the cat. Front. Syst. Neurosci. 13, 69. doi: 10.3389/fnsys.2019.00069
Orlovsky, G., Deliagina, T. G., and Grillner, S. (1999). Neuronal Control of Locomotion: From Mollusc to Man. Oxford: Oxford University Press. doi: 10.1093/acprof:oso/9780198524052.001.0001
Palisses, R., Persegol, L., Viala, D., and Viala, G. (1988). Reflex modulation of phrenic activity through hindlimb passive motion in decorticate and spinal rabbit preparation. Neuroscience 24, 719–728. doi: 10.1016/0306-4522(88)90364-8
Pearson, K. G., Misiaszek, J. E., and Fouad, K. (1998). Enhancement and resetting of locomotor activity by muscle afferents. Ann. N. Y. Acad. Sci. 860, 203–215. doi: 10.1111/j.1749-6632.1998.tb09050.x
Perreault, M. C., Angel, M. J., Guertin, P., and McCrea, D. A. (1995). Effects of stimulation of hindlimb flexor group II afferents during fictive locomotion in the cat. J. Physiol. 487, 211–220. doi: 10.1113/jphysiol.1995.sp020872
Perségol, L., Jordan, M., and Viala, D. (1991). Evidence for the entrainment of breathing by locomotor pattern in human. J. Physiol. 85 38–43.
Perségol, L., Jordan, M., Viala, D., and Fernandez, C. (1988). Evidence for central entrainment of the medullary respiratory pattern by the locomotor pattern in the rabbit. Exp. Brain Res. 71, 153–162. doi: 10.1007/BF00247530
Potts, J. T., Rybak, I. A., and Paton, J. F. R. (2005). Respiratory rhythm entrainment by somatic afferent stimulation. J. Neurosci. Off. J. Soc. Neurosci. 25, 1965–1978. doi: 10.1523/JNEUROSCI.3881-04.2005
Prochazka, A., and Gorassini, M. (1998a). Ensemble firing of muscle afferents recorded during normal locomotion in cats. J. Physiol. 507, 293–304. doi: 10.1111/j.1469-7793.1998.293bu.x
Prochazka, A., and Gorassini, M. (1998b). Models of ensemble firing of muscle spindle afferents recorded during normal locomotion in cats. J. Physiol. 507, 277–291. doi: 10.1111/j.1469-7793.1998.277bu.x
Puckree, T., Cerny, F., and Bishop, B. (1998). Abdominal motor unit activity during respiratory and nonrespiratory tasks. J. Appl. Physiol. Bethesda Md. 84, 1707–1715. doi: 10.1152/jappl.1998.84.5.1707
Reilly, S. M., McElroy, E. J., and White, T. D. (2009). Abdominal muscle function in ventilation and locomotion in new world opossums and basal eutherians: breathing and running with and without epipubic bones. J. Morphol. 270, 1014–1028. doi: 10.1002/jmor.10735
Romaniuk, J. R., Kasicki, S., Kazennikov, O. V., and Selionov, V. A. (1994). Respiratory responses to stimulation of spinal or medullary locomotor structures in decerebrate cats. Acta Neurobiol. Exp. 54, 11–17.
Ruder, L., Takeoka, A., and Arber, S. (2016). Long-distance descending spinal neurons ensure quadrupedal locomotor stability. Neuron 92, 1063–1078. doi: 10.1016/j.neuron.2016.10.032
Ryczko, D., and Dubuc, R. (2013). The multifunctional mesencephalic locomotor region. Curr. Pharm. Des. 19, 4448–4470. doi: 10.2174/1381612811319240011
Saunders, S. W., Rath, D., and Hodges, P. W. (2004). Postural and respiratory activation of the trunk muscles changes with mode and speed of locomotion. Gait Posture 20, 280–290. doi: 10.1016/j.gaitpost.2003.10.003
Schomburg, E. D., Petersen, N., Barajon, I., and Hultborn, H. (1998). Flexor reflex afferents reset the step cycle during fictive locomotion in the cat. Exp. Brain Res. 122, 339–350. doi: 10.1007/s002210050522
Senapati, J. M. (1966). Effect of stimulation of muscle afferents on ventilation of dogs. J. Appl. Physiol. 21, 242–246. doi: 10.1152/jappl.1966.21.1.242
Smith, J. C., Ellenberger, H. H., Ballanyi, K., Richter, D. W., and Feldman, J. L. (1991). Pre-Bötzinger complex: a brainstem region that may generate respiratory rhythm in mammals. Science 254, 726–729. doi: 10.1126/science.1683005
Smith, J. C., and Feldman, J. L. (1987). In vitro brainstem-spinal cord preparations for study of motor systems for mammalian respiration and locomotion. J. Neurosci. Methods 21, 321–333. doi: 10.1016/0165-0270(87)90126-9
Sqalli-Houssaini, Y., Cazalets, J. R., and Clarac, F. (1993). Oscillatory properties of the central pattern generator for locomotion in neonatal rats. J. Neurophysiol. 70, 803–813. doi: 10.1152/jn.1993.70.2.803
Stickford, A. S. L., and Stickford, J. L. (2014). Ventilation and locomotion in humans: mechanisms, implications, and perturbations to the coupling of these two rhythms. Springer Sci. Rev. 2, 95–118. doi: 10.1007/s40362-014-0020-4
Sutor, T. W., Fuller, D. D., and Fox, E. J. (2022). Locomotor-respiratory coupling in ambulatory adults with incomplete spinal cord injury. Spinal Cord Ser. Cases 8, 49. doi: 10.1038/s41394-022-00515-9
Tobin, M. J., Perez, W., Guenther, S. M., D'Alonzo, G., and Dantzker, D. R. (1986). Breathing pattern and metabolic behavior during anticipation of exercise. J. Appl. Physiol. Bethesda Md. 60, 1306–1312. doi: 10.1152/jappl.1986.60.4.1306
Viala, D. (1997). “Coordination of locomotion and respiration,” in Neural Control of the Respiratory Muscles (New York, NY: CRC Press).
Viala, D., Persegol, L., and Palisses, R. (1987). Relationship between phrenic and hindlimb extensor activities during fictive locomotion. Neurosci. Lett. 74, 49–52. doi: 10.1016/0304-3940(87)90049-8
Viala, D., and Vidal, C. (1978). Evidence for distinct spinal locomotion generators supplying respectively fore- and hindlimbs in the rabbit. Brain Res. 155, 182–186. doi: 10.1016/0006-8993(78)90322-0
Waldrop, T. G., and Iwamoto, G. A. (2006). Point: supraspinal locomotor centers do contribute significantly to the hyperpnea of dynamic exercise. J. Appl. Physiol. Bethesda Md. 100, 1077–1079. doi: 10.1152/japplphysiol.01528.2005
Waldrop, T. G., Rybicki, K. J., and Kaufman, M. P. (1984). Chemical activation of group I and II muscle afferents has no cardiorespiratory effects. J. Appl. Physiol. 56, 1223–1228. doi: 10.1152/jappl.1984.56.5.1223
Yamaguchi, T. (1992). Activity of cervical neurons during forelimb fictive locomotion in decerebrate cats. Jpn. J. Physiol. 42, 501–514. doi: 10.2170/jjphysiol.42.501
Young, I. S., Warren, R. D., and Altringham, J. D. (1992). Some properties of the mammalian locomotory and respiratory systems in relation to body mass. J. Exp. Biol. 164, 283–294. doi: 10.1242/jeb.164.1.283
Zhong, G., Droho, S., Crone, S. A., Dietz, S., Kwan, A. C., Webb, W. W., et al. (2010). Electrophysiological characterization of V2a interneurons and their locomotor-related activity in the neonatal mouse spinal cord. J. Neurosci. Off. J. Soc. Neurosci. 30, 170–182. doi: 10.1523/JNEUROSCI.4849-09.2010
Keywords: breathing rate modulation, locomotor-respiratory coupling, neural network interactions, lumbar glutamatergic neurons, limb proprioceptive inputs
Citation: Juvin L, Colnot E, Barrière G, Thoby-Brisson M and Morin D (2022) Neurogenic mechanisms for locomotor-respiratory coordination in mammals. Front. Neuroanat. 16:953746. doi: 10.3389/fnana.2022.953746
Received: 26 May 2022; Accepted: 06 July 2022;
Published: 28 July 2022.
Edited by:
Marie-Claude Perreault, Emory University, United StatesReviewed by:
Ying Zhang, Dalhousie University, CanadaKimberly J. Dougherty, Drexel University, United States
Copyright © 2022 Juvin, Colnot, Barrière, Thoby-Brisson and Morin. This is an open-access article distributed under the terms of the Creative Commons Attribution License (CC BY). The use, distribution or reproduction in other forums is permitted, provided the original author(s) and the copyright owner(s) are credited and that the original publication in this journal is cited, in accordance with accepted academic practice. No use, distribution or reproduction is permitted which does not comply with these terms.
*Correspondence: Didier Morin, ZGlkaWVyLm1vcmluQHUtYm9yZGVhdXguZnI=