- 1Instituto de Neurociencias, Consejo Superior de Investigaciones Científicas, Universidad Miguel Hernández de Elche, Elche, Spain
- 2Departamento de Anatomía, Biología Celular y Zoología, Facultad de Ciencias, Universidad de Extremadura, Badajoz, Spain
The thalamocortical projections are part of the most important higher level processing connections in the vertebrates and follow a highly ordered pathway from their origin in the thalamus to the cerebral cortex. Their functional complexities are not only due to an extremely elaborate axon guidance process but also due to activity-dependent mechanisms. Gli2 is an intermediary transcription factor in the Sonic hedgehog (Shh) pathway. During neural early development, Shh has an important role in dorsoventral patterning, diencephalic anteroposterior patterning, and many later developmental processes, such as axon guidance and cell migration. Using a Gli2 knockout mouse line, we have studied the role of Shh signaling mediated by Gli2 in the development of the thalamocortical projections during embryonic development. In wild-type brains, we have described the normal trajectory of the thalamocortical axons into the context of the prosomeric model. Then, we have compared it with the altered thalamocortical axons course in Gli2 homozygous embryos. The thalamocortical axons followed different trajectories and were misdirected to other territories probably due to alterations in the Robo/Slit signaling mechanism. In conclusion, the alteration of Gli2-mediated Shh signaling produces an erroneous specification of several territories related with the thalamocortical axons. This is translated into a huge modification in the pathfinding signaling mechanisms needed for the correct wiring of the thalamocortical axons.
Introduction
The thalamus (Th) is a neuronal nuclei complex located in the diencephalon. Its functions involve sensory processing and regulation of motor functions as well as the control of sleep and awake states of consciousness (Jones, 2007). Also, as increasingly recognized, the Th acts as a link between distinct cortical areas (Theyel et al., 2010) and it is related to the cortical states control in a behaviorally relevant way (Cruikshank et al., 2010; Minlebaev et al., 2011; Poulet et al., 2012).
It develops from the alar portion neuroepithelium of prosomere 2 (Puelles et al., 1987; Puelles and Rubenstein, 1993, Puelles and Rubenstein, 2003) between embryonic days (E) E10.5 and E16.5 (Angevine, 1970; Puelles and Rubenstein, 1993, Puelles and Rubenstein, 2003). Primarily, it generates five pronuclei, which further differentiate into specific thalamic nuclei gradually building a complex nested structure (Jones, 2007). This structure is defined by the expression of the homeobox gene Gbx2, a transcription factor required for thalamic development (Miyashita-Lin et al., 1999). This region is a highly subdivided structure in terms of its nuclear organization. In mammals, this structure is constituted by dozens of morphologically and functionally distinct nuclei (Jones, 2007). In parallel with the process of progressive nuclear differentiation within the Th, there is a morphological and neurochemical differentiation of relay neurons, interneurons, and neuroglial cells, and finally, afferent and efferent connections are established (Jones, 2007). Its development is a model of the generation of an intricate, three-dimensional brain region from a two-dimensional neuroepithelium (Haddad-Tóvolli et al., 2012).
The thalamocortical projection constitutes one of the most prominent higher level processing connections in the mammalian brain. This projection conveys inputs to the sensory and motor cerebral cortex, where the processing of this information leads to perception and the organization of valid responses to internal and external stimuli. The innervation of the cerebral cortex by thalamic axons is a late event in brain development, but the thalamic axons growth toward their cortical targets begins quite early in embryogenesis even before the establishment of subcortical afferent connections to the Th (Molnár et al., 2012).
The thalamocortical projection functional complexity is due to a complex elaborate axon guidance process, linking the various thalamic nuclei with specific cortical regions (Castillo-Paterna et al., 2015; Mitsogiannis et al., 2017; López-Bendito, 2018; Nakagawa, 2019; Quintana-Urzainqui et al., 2020).
Around the second and third week of gestation (E12–E18 in mice), the cortex and Th start to link with each other through precise and reciprocal connections. Thalamocortical and corticothalamic projections have to cross diverse regions and morphogenetic boundary zones, including the diencephalic–prosencephalic, hypothalamic-telencephalic, and the pallial–subpallial boundaries, to reach their ultimate target cells. The thalamocortical axons (TCAs) follow a highly organized pathway from their Th origin to the cerebral cortex (López-Bendito and Molnár, 2003; Molnár et al., 2003, Molnár et al., 2012; Garel and Rubenstein, 2004). They move rostrally toward the alar peduncular hypothalamus (PHy), make a sharp turn dorsally at the alar PHy-telencephalic boundary to enter the mantle region of the medial ganglionic eminence (MGE), where they enter the internal capsule by E13 in mice, and then advance through the striatum, cross the pallial–subpallial boundaries, and finally reach the developing cortex.
It remains unclear how different thalamocortical projecting neural groups are topographically specified, and which transcription factors regulate the growth of their axons (López-Bendito and Molnár, 2003; Shimogori and Grove, 2005; Price et al., 2006; Quintana-Urzainqui et al., 2020). Numerous transcription factors are expressed in distinct but often overlapping patterns in the Th, suggesting that they must cooperate to control the specification and differentiation of thalamic nuclei and cell types. The zona limitans intrathalamica is the main organizer of the Th (Martinez-Ferre and Martinez, 2012). Its morphogenetic signal is Sonic Hedgehog (Shh; Scholpp and Lumsden, 2010). This morphogen induces the expression of transcription factors such as Gbx2, which is expressed broadly and early in the Th (Bulfone et al., 1993), and later it is required for the differentiation of a subset of nuclei and the development of TCA projections (Miyashita-Lin et al., 1999).
The Hedgehog family of secreted signaling molecules plays crucial roles in many aspects of embryonic development and adult tissue homeostasis [reviewed by Ingham and McMahon (2001) and Logan and Nusse (2004)]. Its role in the specification and differentiation of the diencephalic, an especially of the Th (Kiecker and Lumsden, 2004; Vieira et al., 2005; Vue et al., 2009; Jeong et al., 2011; Epstein, 2012; Ding et al., 2017).
Canonical Shh signaling occurs via a derepression mechanism involving the multiple-pass transmembrane receptor Patched (Ptch) and the G-protein-coupled receptor-like molecule Smoothened (Smo). Shh binding to Ptch relieves this repression, allowing Smo to translocate to the primary cilium where it activates intracellular signaling pathways. This activation of Smo culminates can activate or repress Shh target genes (Martí and Bovolenta, 2002).
In vertebrates, three Gli transcription factors (Gli1–Gli3) have been described, all of which are expressed in the developing neural tube (Hui et al., 1994; Lee et al., 1997; Ruiz i Altaba, 1998; Bai et al., 2002; Jacob and Briscoe, 2003). While Gli2 and Gli3 have transcriptional activator and repressor functions, Gli1 is solely a transcriptional activator [reviewed by Ruiz i Altaba et al. (2007)]. Each Gli family member responds in a different way to Shh. In the absence of Shh, Gli2 is fully degraded, while Gli3 is converted (via proteolytic processing of its C-terminus) to a repressor of transcription (Gli3R). When Shh is present, Gli1 expression is induced and proteolysis of Gli2 and Gli3 is inhibited, allowing full-length activator forms of the GLI proteins to accumulate. The Gli transcriptional activators then induce the expression of Shh pathway target genes (Dai et al., 1999; Ruiz I Altaba, 1999; Aza-Blanc et al., 2000; Pan et al., 2006). The Gli2 loss of function analyzed in the spinal cord demonstrated a strong reduction in the generation the ventral fated neurons (Matise et al., 1998).
Our aim is to decipher the role of the Shh-induced mechanism mediated by Gli2 in the development of the TCAs during embryonic development.
Materials and Methods
Animals
The transgenic mouse line Gli2–/– was generated as previously described (Mo et al., 1997). The day when the vaginal plug was detected was considered as embryonic day 0.5 (E0.5). The embryos were fixed in phosphate buffered saline (PBS) 1x (NaCl 13 mM, Sigma S3014; KCl 0.3 mM, Sigma P9541; Na2HPO4 1 mM, Sigma S3264 and KH2PO4 0.2 mM, Sigma P9791) with 4% paraformaldehyde (PFA, Panreac 141451.1211) overnight at 4°C. The embryos were washed in PBS 1x, embedded in 4% agarose (Pronadisa 8008), and sectioned in 100 μm vibratome sections. For wax-embedded sections, the embryos were completely dehydrated, washed twice in 100% butanol (Panreac 14.682.1211) wax embedded (GemCut emerald paraffin, Spiele no. 24364-1), and then sectioned in parallel series (7 μm). All mouse experiments were performed according to the protocols approved by the Universidad Miguel Hernández de Elche OIR Committee (ref. 2014/VSC/PEA/0055).
In situ Hybridization
The technique was realized as previously described (Martinez-Lopez et al., 2015). The RNA probes were obtained from constructions and were kindly provided by Dr. O. Marin (Robo1 and Slit2), Dr. J. Rubenstein (Dlx2), and Dr. O. Reiner (Ntn1). Each experiment was reproduced five times.
Immunohistochemistry
The technique was realized as previously described (Martinez-Lopez et al., 2015).
The antibodies used were α-Calbindin (1:100, Swant #CB-48), α-Calretinin (1:200, Swant 7699/4), α-deleted in colon carcinoma (DCC; 1:100, Santa Cruz sc6535), α-ISLET1 (1:100, Hybridoma Bank 39.4D5), and α-NKX2.1 (1:500, Biopat PA 0100). Each experiment was reproduced five times.
Axonal Tracing
For axonal tracing, embryonic brains or slices were fixed overnight or for 30 min in 4% PFA, respectively. Small DiI crystals (1,1′-dioctadecyl 3,3,3′,3′ tetramethylindocarbocyanine perchlorate; Molecular Probes) or DiA crystals (4-4-dihexadecyl aminostyryl N-methylpyridinium iodide; Molecular Probes) were inserted into the Th of the brains or into the slices previously dissected into 250 μm sections, diffused at 37°C in 4% PFA until the tracers had diffused sufficiently, and then, the brains were cut on a vibratome into 100 μm sections. DAPI (4′, 6-Diamidino-2-Phenylindole, dihydrochloride; Sigma #D9542) was used as a fluorescent nuclear counterstain, diluted in PBS at 0.001%, and incubated 10 min at room temperature. Each experiment was reproduced five times.
Area Quantification
We have used the measure tool of the Fiji program on a representative section of each experiment. The statistical analysis was realized by an unpaired t-test with the GraphPad Prism 8.0.2.
Results
Abnormal Thalamocortical Projections in Gli2 Mutant Mice
At E18.5, in the wild type, the TCAs, labeled by the detection of calretinin, are observed to be crossing the diencephalic territory. Once the Phy is reached, they turned dorsally, crossed the alar PHy-telencephalic boundary, and reached the basal ganglia. Finally, they arrived at the Cx, the final destination of the TCAs (Figure 1A). In the mutant embryos, at the same stage, the TCAs displayed a striking abnormal trajectory. Once the axons reached the boundary between the prethalamus (pTh) and the Phy, they appeared strongly defasciculated and were misdirected into aberrant directions (Figures 1B–D). Some TCAs headed toward caudal regions (arrow in Figure 1D), others toward ventral regions of the hypothalamus (Hy) (arrowhead in Figure 1D), and the rest toward rostral regions. Some axons of this last group entered the telencephalic vesicle stopping at the presumptive lateral ganglionic eminence (LGE; arrows in Figure 1D).
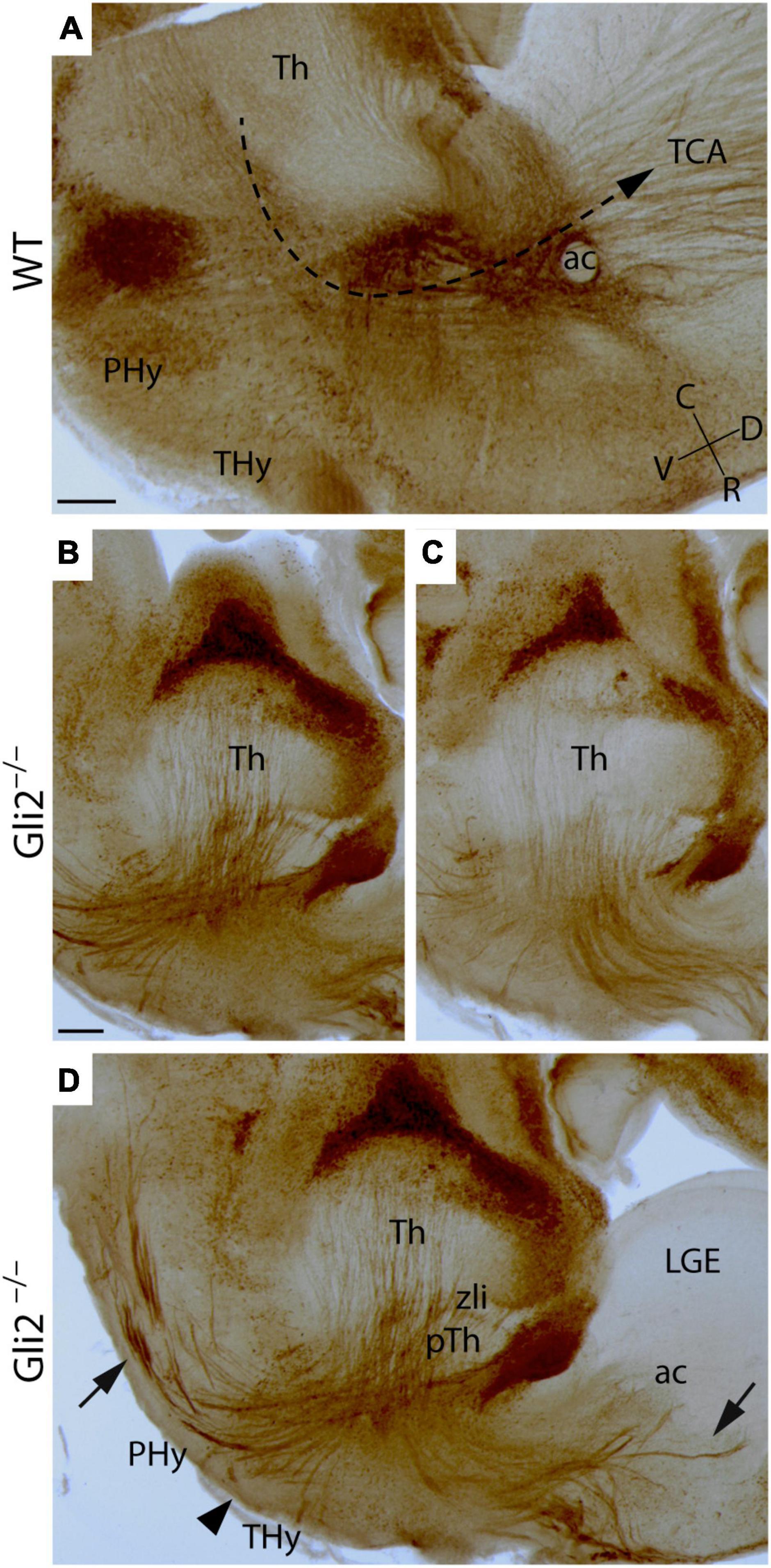
Figure 1. Thalamocortical axonal trajectory in Gli2 mutant mice embryos. Sagittal sections through wild-type WT (A) and Gli2 mutant (B–D) E18.5 embryos with a immunohistochemistry against calretinin. (D) Is a merge of images displayed in (B,C) to better illustrate the TCAs dispersion. In (A), the dotted arrow indicates the direction of the TCAs trajectory in the WT. The arrows indicate the aberrant trajectory of TCAs to the caudal and rostral region. The arrowhead indicates the axons directed toward the hypothalamus. ac, anterior commissure; C, caudal; D, dorsal; Hy, hypothalamus; LGE, lateral ganglionic eminence; PHy, peduncular hypothalamus; pTh, prethalamus; R, rostral; THy, terminal hypothalamus; TCA, thalamocortical axons; Th, thalamus; V, ventral; zli, zona limitans. Scale bar (A): 300 μm; (B–D): 200 μm.
In order to better describe the TCAs anomalous trajectory, we also analyzed mutant and wild-type embryos in a transversal plane of section at the same stage. In the wild type, the DCC (receptor of Netrin1) positive axons are detected to be crossing the diencephalic territory through the pTh (Figure 2A). In the mutant brain, the TCAs followed the same route but already displayed some alterations in their distribution (arrow in Figure 2B). In the wild type, once they reached the PHy, following their normal course, they bended dorsally to enter into the telencephalic vesicle (Figure 2C). Meanwhile, in the mutant, the TCAs maintained a straight trajectory toward the hypothalamic territory (arrow in Figure 2D). In the wild type, a section through the basal terminal hypothalamus (THy) confirmed the absence of calretinin positive axons in this territory (Figure 2E). In the mutant, we confirmed the presence of aberrant trajectory and showed its course into the Hy (Figure 2F). The TCAs can be followed into the basal territory of the THy (arrows in Figure 2F). They seemed to reach the ventral midline located in the floor plate. This strong alteration in the TCAs trajectory observed in a late embryonic stage prompted us to analyze mid-stage embryos with an aim to understand the possible causes.
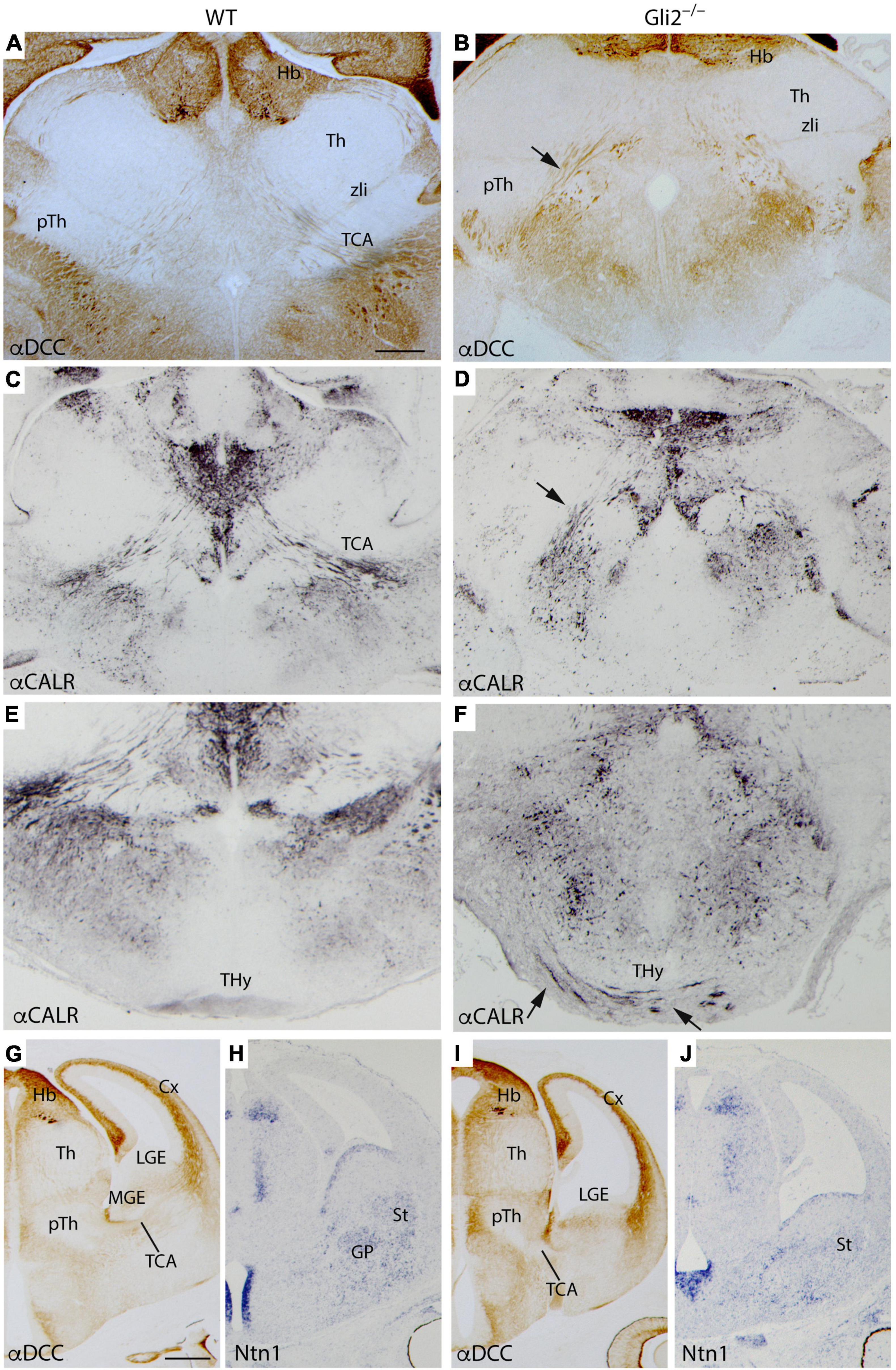
Figure 2. TCAs aberrant trajectory in late- and mid-stage mutant embryos. Selected transversal sections through the forebrain of E18.5 WT (A,C,E) and Gli2 mutant (B,D,F) showing immunohistochemistry for α-DCC (A,B) revealed with DAB and α-Calr revealed with DAB plus nickel (C–F). The mutant TCAs followed an aberrant trajectory crossing the PHy and the THy [arrow in (B,D)] reaching the midline in the basal hypothalamus [arrows in (F)]. Coronal sections of E12.5 WT (G,H) and Gli2 mutant (I,J) labeled by immunohistochemistry against DCC (G,I) and by in situ hybridization for Ntn1 (H,J). Cx, cortex; DCC, Deleted in colon carcinoma; Hb, Habenula; THy, terminal hypothalamus; GP, globus pallidus; LGE, lateral ganglionic eminence; MGE, medial ganglionic eminence; pTh, prethalamus; St, striatum; TCA, thalamocortical axons; Th, thalamus; zli, zona limitans. (A–F) Scale bar: 200 μm; (G–J) scale bar: 300 μm.
At E14.5 embryos, we labeled transverse sections by immunohistochemistry against DCC and with Netrin1 in situ hybridization. Netrin1-Dcc signaling mechanism plays an important role in TCAs guidance (Braisted et al., 2000; Powell et al., 2008). The gap of the TCAs was observed already in the corridor of the MGE, crossing the LGE, and reaching the cortex (Figure 2G). The Ntn1 appeared distributed in the ventricular layer of MGE and LGE and in its mantle layers, globus pallidus in the MGE, and striatum in the LGE (Figure 2H). In the mutant embryos, we found that once the TCAs arrived at the PHy-telencephalic boundary, they were blocked (Figure 2I). The ganglionic eminences also displayed an abnormal morphology. The distribution of Ntn1 appeared scattered along the mantle layer of the presumptive ganglionic territory (Figure 2J).
Finally, in order to confirm the thalamic origin of the aberrant axons described in the mutant embryos, we located the crystals of DiI/DiA into the Th of E18.5 Gli2–/– mutant and wild-type fixed embryos. In sagittal sections, the DiI crystal located in the wild-type Th allowed us to follow the normal TCAs trajectory. After entering the pTh, they bend dorsally and enter the telencephalic vesicle, where they reach the cortex after they cross the ganglionic eminences (Figures 3A,A’). In the mutant brains, we observed the strong TCAs defasciculation (Figures 3B,B’) and corroborated their aberrant trajectories into caudal and ventral directions (arrow and arrowhead in Figure 3B). The samples sectioned in a transversal plane verified the abnormal TCAs that entered the basal Thy by the application of DiI (red color) and DiA (green color). The left- and right-sided TCAs merged at the THy floor plate (arrow in Figures 3C,C’).
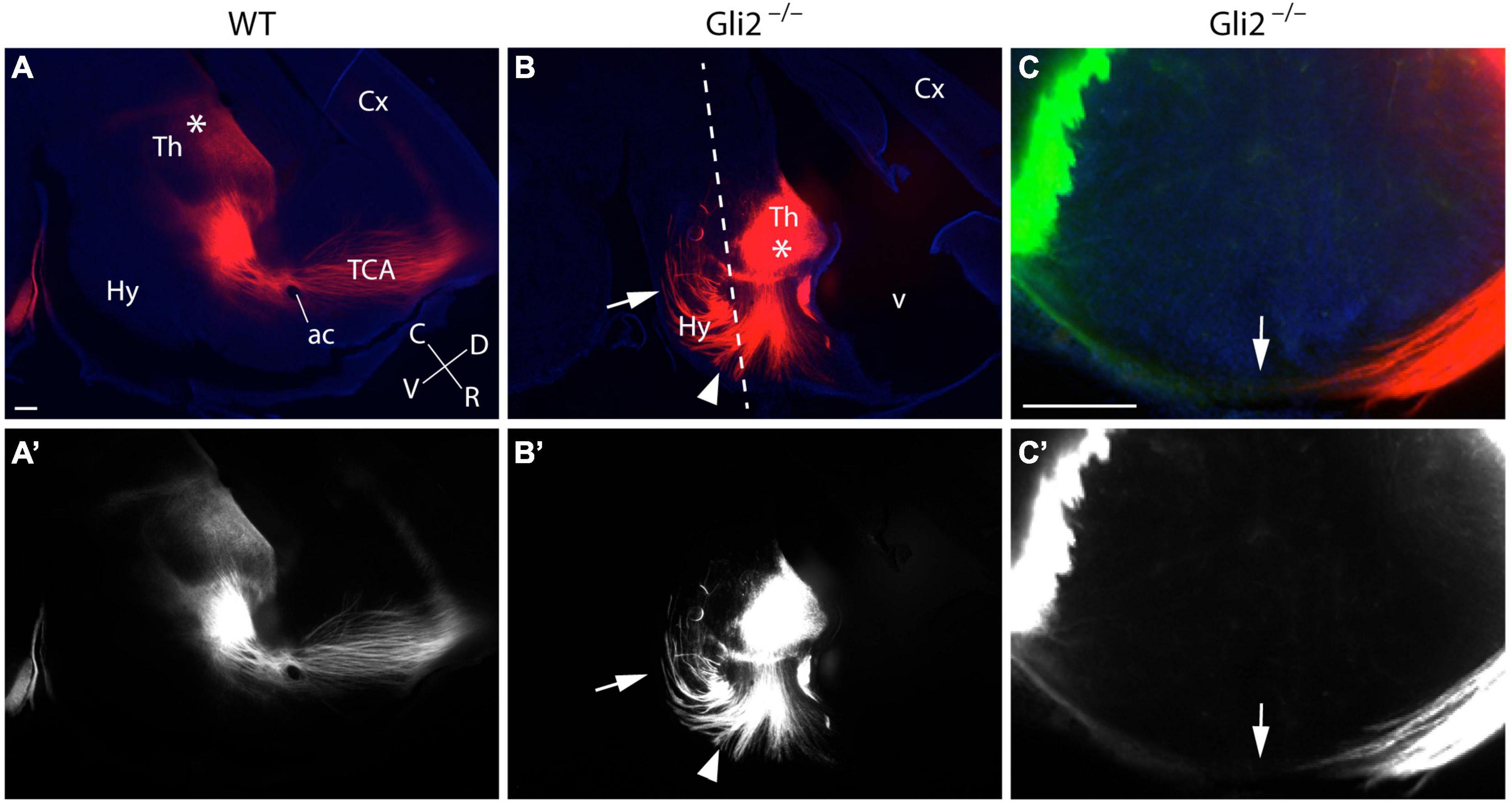
Figure 3. DiI/DiA labeling of thalamocortical axons (TCAs) in Gli2 mutant mice. Selected sagittal vibratome sections (100 μm) through the forebrain of E18.5 embryos WT (A,A’) and Gli2–/– (B,B’) labeled with DiI (red color). Transversal sections (C,C’) through the forebrain of E18.5 embryos Gli2 mutant labeled with DiI (red color) and DiA (green color). DiI implanted in wild-type Th labeled the normal route of the TCAs along the forebrain until they reach the cortex (Cx; A,A’). DiI placed in the Th of Gli2–/– brains labeled bundles of axons misrouted rostrally, caudally (arrow), and ventrally toward the THy (arrowhead; B,B’). Samples sectioned in a transversal plane of section to the hypothalamus [dotted line in (B)] to show the final destination of the axons in the Thy, the arrow indicates the floor midline of the Thy (C,C’). Asterisks in (A,B) indicate the DiI/A crystal placement sites. The images displayed in (A–C) are shown in black and white (A’–C’) to facilitate the observation of the labeled axons. ac, anterior commissure; C, caudal; Cx, cortex; D, dorsal; Hy, hypothalamus; R, rostral; TCA, thalamocortical axon; Th, thalamus; V, ventral. Scale bar: 300 μm.
Morphological Description of Main Forebrain Territories Involved in Thalamocortical Projections in Gli2 Mutant Mice
We hypothesized that the aberrant trajectory found in the Gli2–/– mutant has two possible origins. First, it could be due to an alteration in the specification of the territories crossed by the tract or second, it could be due to an alteration in the signaling mechanisms.
To pursue the identification of the origin of this aberrant trajectory, we analyzed different molecular markers that could allow us to describe the territories related to TCAs guidance.
First of all, the TCAs leave the Th rostrally and cross the pTh in the diencephalon. We studied the expression of Gbx2, thalamic-specific homeodomain transcription factor, and Dlx2, homeobox gene of the distal-less family expressed in the pTh. In the wild type, we found that Gbx2 expression was localized in the paraventricular thalamic nucleus, lateral geniculate nucleus, central thalamic nucleus, and ventromedial thalamic nucleus in the Th (Figure 4A). The Gbx2 expression in Gli2–/– Th was slightly abnormal when compared with wild-type embryos (Figure 4B). In the pTh, the Dlx2 staining was localized in the zona incerta and reticular nucleus in wild-type embryos (Figure 4C). This distribution was similar in the Gli2–/– mutants (Figure 4D) with some minor alterations. The areas occupied by the Th and pTh in the mutant did not display a significative difference with the wild type.
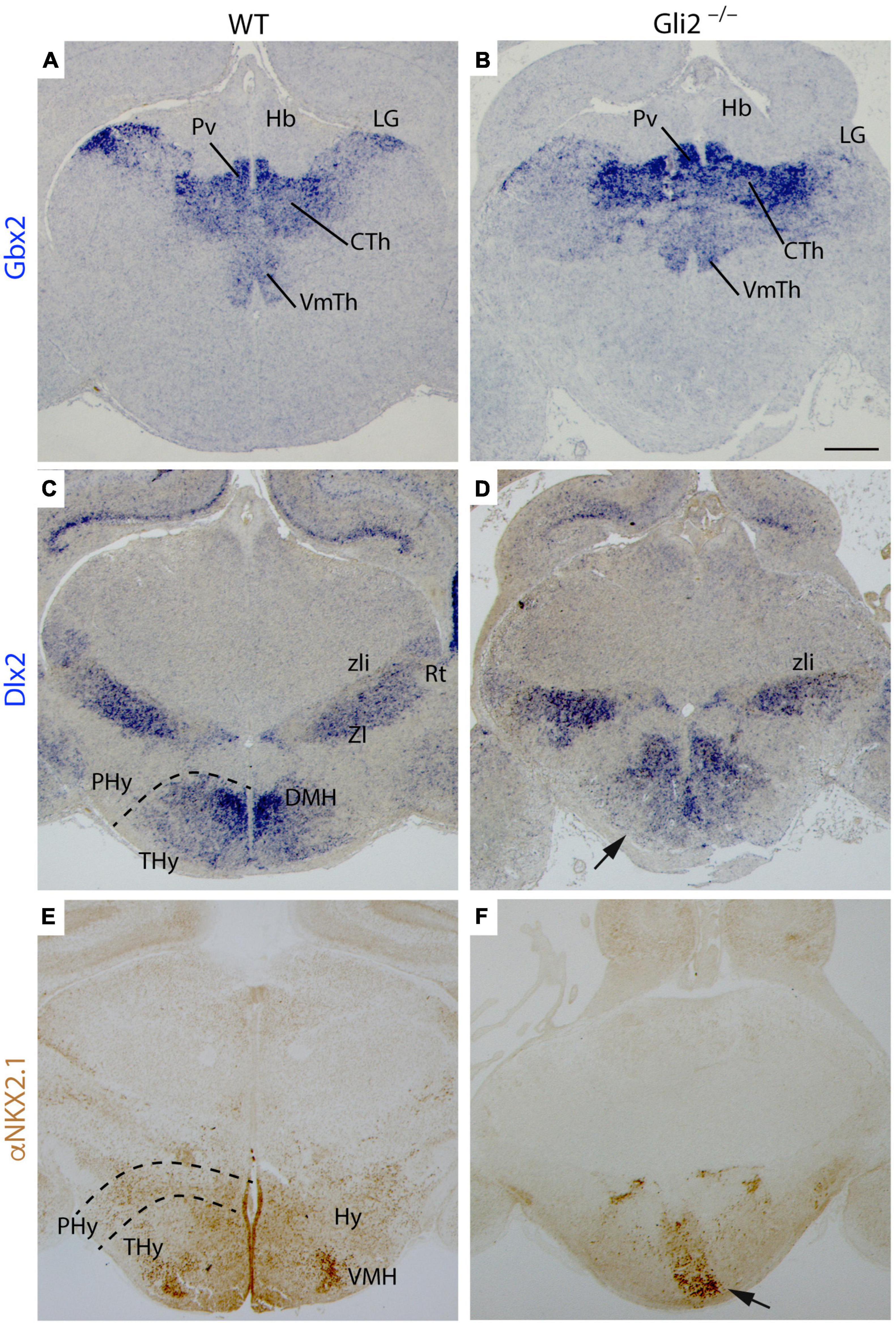
Figure 4. Specification of diencephalon and secondary prosencephalon territories in Gli2 mutant mice. Frontal sections through wild-type and E18.5 Gli2–/– embryos. (A,B) Gbx2 in situ hybridization. (C,D) Dlx2 in situ hybridization. (E,F) α-NKX2.1 immunohistochemistry in brown. The dotted lines indicate the alar-basal boundary. The Gbx2 expression in the LG, CTH, and PvTh nucleus of Gli2–/– thalamus is similar to the one observed in the control (A,B). Dlx2 labels the prethalamic ZI and Rt with a similar pattern than in the control (C,D). Dlx2 is also expressed in DMH in the basal THy of wild type although in Gli2–/–, this expression was not detected [arrow in (D)]. α-NKX2.1 had a scattered distribution in the basal THy, but presented a dense labeling of a VMH subnuclei. This distribution was strongly affected in the mutant [arrow in (F)]. CTh, Central thalamus nucleus; DMH, Dorsal medial hypothalamus nucleus; LG, Lateral geniculate nucleus; Hb, Habenula; PHy, peduncular hypothalamus; THy, terminal hypothalamus; Pv, Paraventricular Thalamic nucleus; Rt, reticular nucleus; VMH, Ventromedial hypothalamus nucleus; VmTH, ventromedial thalamic nucleus; ZI, zona incerta; zli, zona limitans. Scale bar: 300 μm.
Second, the TCAs reached the PHy in the caudal secondary prosencephalon. In this territory, they bend dorsally and reach the telencephalon. Rostrally to the PHy lays the THy, the non-permissive territory for the TCAs. We analyzed Dlx2, the homeobox gene of the distal-less family expressed in the basal PHy and THy and NKX2.1 protein distribution (specific marker of both territories). In the wild type, both markers were located in the basal THy. We were able to identify the dorsomedial hypothalamic nucleus and the ventromedial hypothalamic nucleus, being Dlx2 positive and NKX2.1 positive, respectively (Figures 4C,E). In the Gli2–/–, the basal THy displayed an aberrant distribution of both markers and we were not able to identify both hypothalamic nuclei (Figures 4D,F). The area occupied by Dlx2 expression in the mutant was 49% smaller than in the wild type. The comparison of NKX2.1 localization in the mutant displayed a reduction of 66% when compared with the wild type. These differences were statistically significative (p < 0.05).
Finally, the TCAs reach the subpallium through the MGE. This is a strongly non-permissive territory for these axons. A group of cells tangentially migrated from the LGE generates a permissive area, known as the corridor that allows the TCAs to cross through this territory (López-Bendito et al., 2006). After their navigation through the LGE, the axons reach their final destination, the cortex.
With the aim to unveil if these territories are affected in Gli2 mutant mice, we performed the analysis of Dlx2 and Ntn1 mRNA expression combined with the study of NKX2.1 and ISLET1 protein distribution in E18.5 embryos. In the wild type, the Dlx2 expression domain included the ventricular territory of the MGE and LGE (Figure 5A). In the mutant, the Dlx2 ventricular expression domain of the presumptive ganglionic eminences was strongly reduced in extension (Figure 5B). In the wild type, the NKX2.1 protein was distributed in the ventricular layer and identified the globus pallidus in the corresponding mantle layer (Figure 5C). In the mutant, we found a complete absence of NKX2.1 protein in the MGE. No positive neurons of the globus pallidus were identified (Figure 5D). The Ntn1 expression appeared was also affected when compared with the control (Figures 5C,D). In both cases, it labeled the striatal territory in the mantle layer of the LGE. But the distribution in the presumptive mutant ganglionic eminence was clearly affected. A clear gap of Ntn1 expression was located in this territory, coinciding with the area where the TCAs reach (arrow in Figure 5D). The analysis of ISLET1 protein distribution allowed us to detect the positive cells generated in the LGE that migrated radially to create the striatum. The labeling allowed us to detect the gap generated by the corridor neurons that allowed the TCAs to cross this territory (Figure 5E). In the mutant embryos, the positive cells in the striatum were also detected (Figure 5F). However, the corridor was strongly affected and was much wider than in the wild type (arrow in Figure 5F).
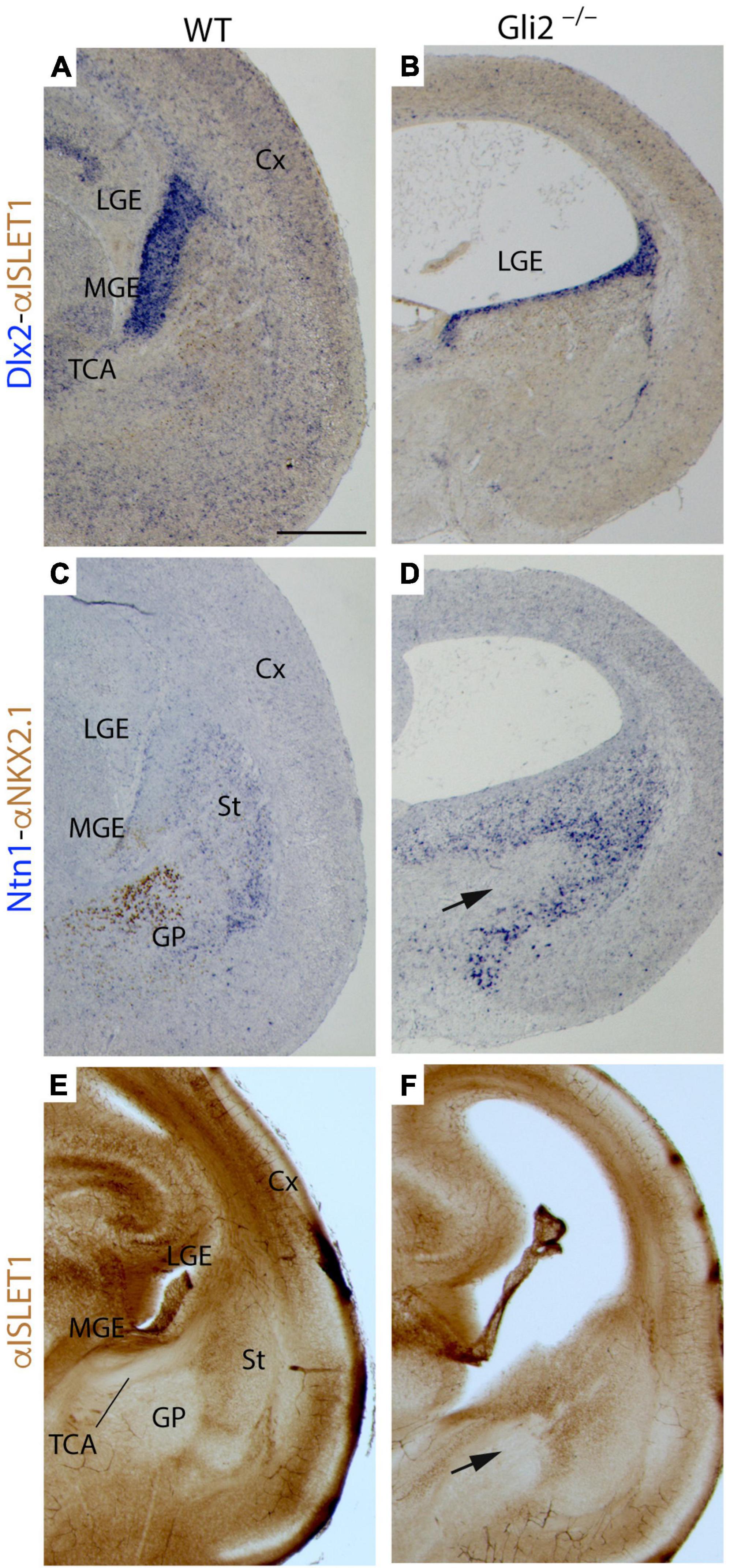
Figure 5. Territories of caudal secondary prosencephalon secondary in Gli2 mutant mice. Frontal paraffin (7 μm) (A–D) and vibratome (100 μm) sections (E,F) through wild type and Gli2–/– of E18.5 embryos with Dlx2 in situ hybridization combined with α-Islet 1 immunoreacted in brown (A,B); Ntn1 in situ hybridization combined with α-NKX2.1 immunoreacted in brown (C,D); and α- ISLET1 immunohistochemistry (E,F). In wild type, the ganglionic eminences are differentiated structures (LGE and MGE) but in the mutant, the Dlx2 expression is reduced and seems to correspond to the LGE (A,B). In the wild type, α-NKX2.1 labels the globus pallidus and ventricular MGE, meanwhile Netrin1 is expressed in the striatum [LGE mantle layer; (C)]. In the mutant, α-NKX2.1 is not detected and there is a wider Netrin1 positive territory [arrow in (D)]. In wild type, α-ISLET1 labels the striatum (E) but in Gli2–/–, these labeling is also expanded [arrow in (F)]. Note that the lateral ventricles appeared expanded in the mutant. Cx, Cortex; GE, ganglionic eminences; GP, globus pallidus; LGE, lateral ganglionic eminence; MGE, medial ganglionic eminence; St, striatum; TCA, thalamocortical axon. Scale bar: (A,C,E) 200 μm; (B,D,F) 300 μm.
Summarizing, the data mentioned above indicated a strong malformation of the MGE in the mutant embryos. The LGE was also seemed to be affected but its molecular markers were still present. This erroneous specification of the MGE could explain the incapacity of the TCAs to cross this territory and reach the cortex.
Pathfinding Signaling Alteration in Thalamocortical Projections of the Gli2 Mutant Mice
Once we analyzed the specification of the different territories involved in the trajectory of the TCAs, we decided to address the second plausible hypothesis for the alterations detected. This is an alteration in the signaling mechanisms needed for the correct wiring of this tract.
The Robo-Slits integrate one of the main chemo-repulsive signal mechanisms involved in axon guidance (Blockus and Chédotal, 2016). It has been directly related to TCAs guidance and their repulsion from the hypothalamic territory (Braisted et al., 1999). Robo2 receptor expression was detected in the Th of both Gli2–/– and wild-type embryos (Figures 6A,B). Therefore, we decided to analyze the expression pattern of Slit1 and Slit2, principal ligands for the Robo receptors, in E12.5 Gli2–/– and wild-type embryos.
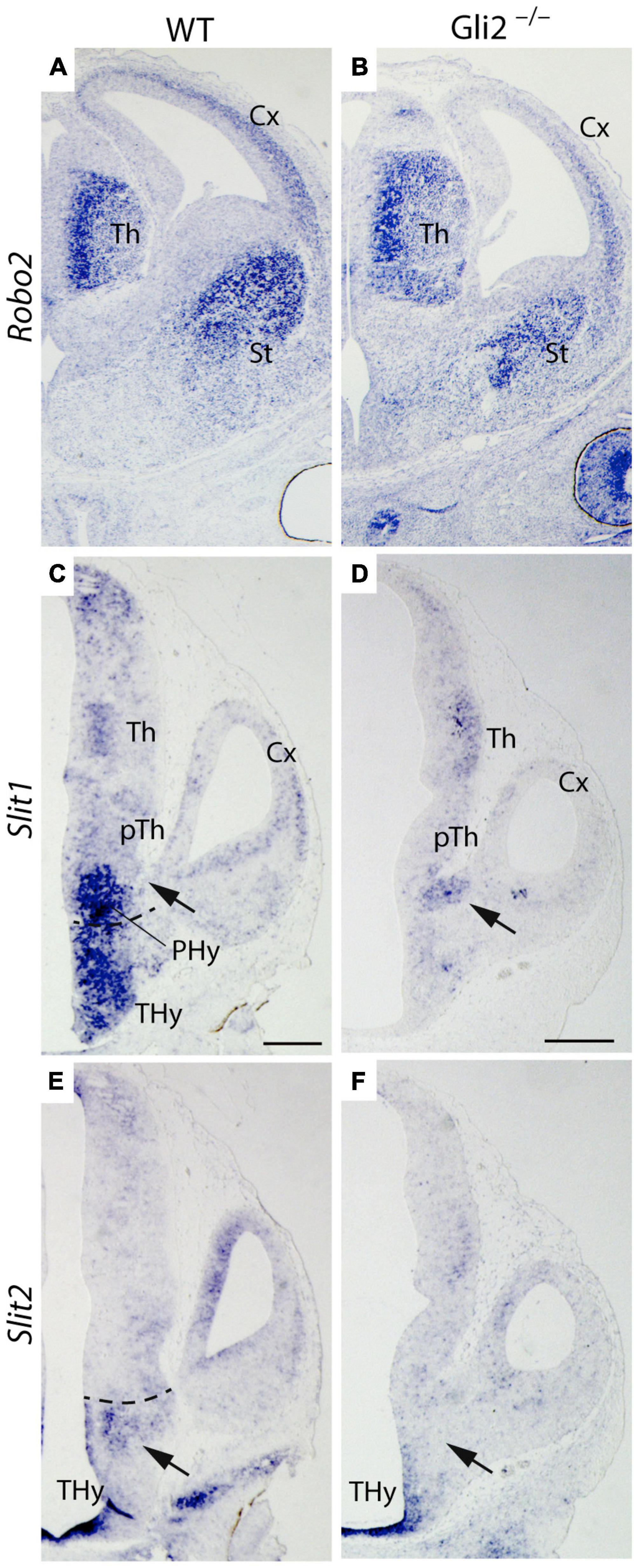
Figure 6. Robo/Slit mechanism in TCAs pathfinding in Gli2 mutant mice. Frontal sections of E14.5 wild-type and Gli2–/– brains with Robo2 in situ hybridization. Horizontal sections of E12.5 wild-type and Gli2–/– embryos with Slit1 (C,D) and Slit2 (E,F) in situ hybridization. In (A,B), Robo2 is detected in the thalamus, striatum, and cortex of wild type and Gli2–/–. In (C), Slit1 expression was founded in PHy and THy. A gap of expression is detected in the area where the TCAs cross (arrow). In (D), Slit1 expression was localized in the zone used by the TCAs to enter the telencephalic vesicle (arrow). In (E), Slit2 was detected in the midline of the THy and in a domain bordering the limit with the PHy (arrow). In (F), the Slit2 expression in the mutant appeared only in the THy midline, being absent in the rest of the Thy territory (arrow). The dotted line indicates the limit between Thy and Phy. Cx, Cortex; PHy, peduncular hypothalamus; pTh, prethalamus; St, striatum; Th, thalamus; THy, terminal hypothalamus. Scale bar: 300 μm.
We focus our attention in the PHy, the territory where the axons defasciculate and change their normal direction. The main area of interest is located under the interventricular foramen, the place where the TCAs cross the PHy-telencephalic boundary.
In the wild type, Slit1 expression was found in a large domain in the PHy and THy. A clear gap of expression was detected in the area where the TCAs cross in order to get into the telencephalon (arrow in Figure 6C). In the mutant, Slit1 expression was localized in a spot underneath the interventricular foramen (arrow in Figure 6D), a precise location where the TCAs enter the telencephalic vesicle. No expression was detected in any other area of the PHy and THy. Regarding Slit2 expression, in the wild type, Slit2 was detected in the THy bordering the PHy (arrow in Figure 6E). In the mutant, it was found in a thin territory at both sides of the THy midline. No expression was detected in other areas of both hypothalamic territories (arrow in Figure 6F). The data obtained allowed us to hypothesize that in the mutant, the expression of Slit1 underneath the interventricular foramen prevents the TCAs from crossing into the telencephalic vesicle. The lack of Slit2 expression in the THy allows the TCAs to invade the territory.
Discussion
Our working hypothesis is based on the role of the Shh signaling mechanism through Gli2 in the pathfinding machinery of the TCAs. The axons are guided by guidance molecules located along specific pathways (Tessier-Lavigne and Goodman, 1996; Dickson, 2002). They travel following a series of distinct steps in which specific guidance cues located at fixed decision points determine their direction. In axon pathfinding, therefore, not only guidance factors are crucial but their precise distribution in time and space constitutes an essential part of the process.
Aberrant Thalamocortical Axons in Gli2 Mutant Mice
The data obtained pointed out a clear defect in the TCAs trajectory in the loss of the function of Gli2. The TCAs are able to leave the Th, cross the pTh, and after entering the Phy, they defasciculate and derail from their normal course. Some axons turn caudalward, others get into the basal Thy, and the rest try to enter unsuccessfully into the telencephalic vesicle. This behavior of the tract implies a complete failure in the signaling mechanisms needed to drive the TCAs into the cortex. The different Th axons are not able to read their way and this lack of information drives them in different directions (almost randomly; Figure 7). Haddad-Tóvolli et al. (2012) described the Th alterations in Gli2 and Gli3 loss of function. They briefly described in the Gli2 mutant some thin and sparse axons in the cortical layers without describing any misrouting of the tract out of the telencephalic vesicle.
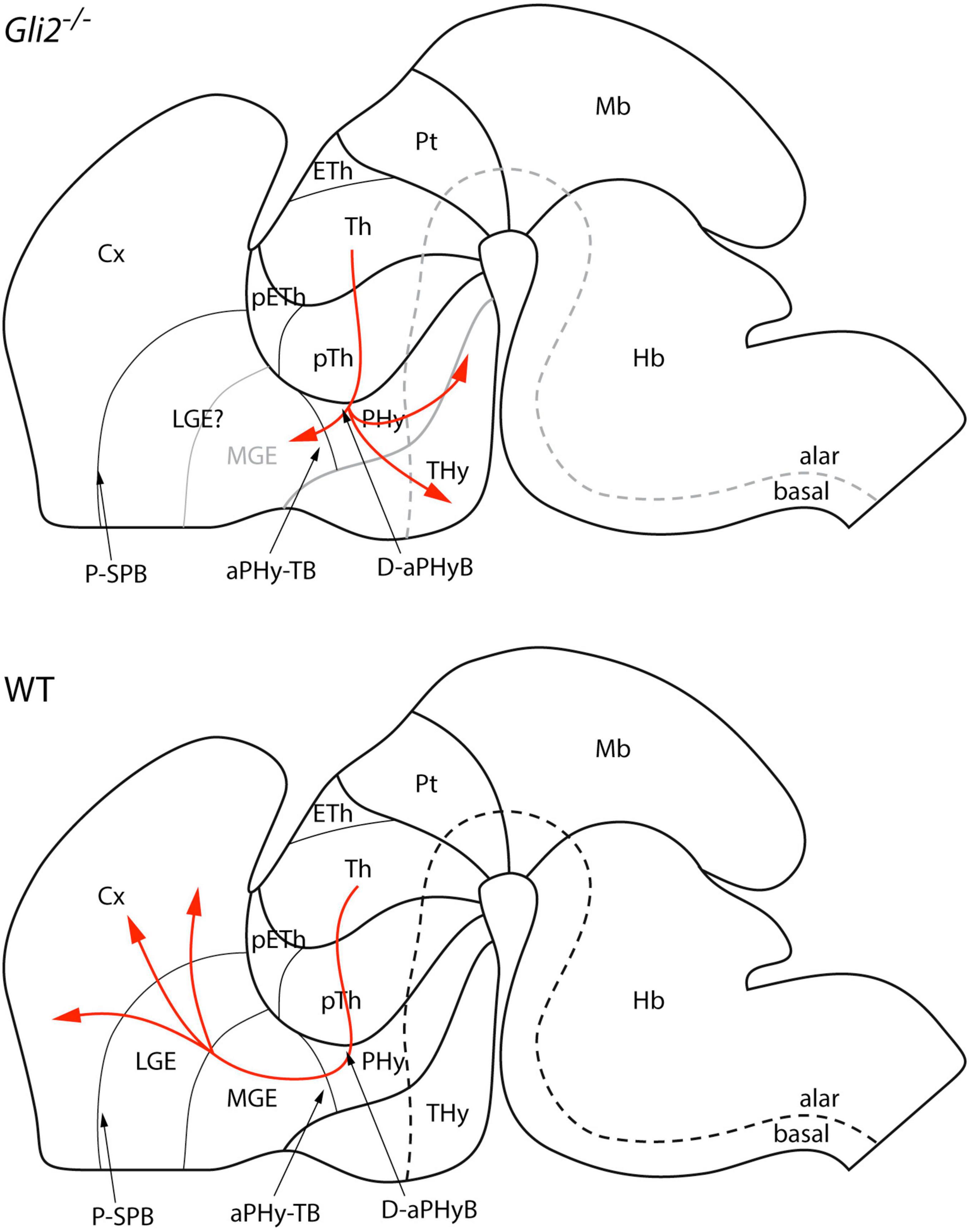
Figure 7. Schematic representation of thalamocortical projections in Gli2 mutant and wild type in the context of the prosomeric model. aPHy-TB, alar peduncular hypothalamus-telencephalic boundary; Cx, cortex; D-aPHyB, diencephalon alar peduncular hypothalamus boundary; Eth, epithalamus; Hb, hindbrain; LGE, lateral ganglionic eminence; Mb, midbrain; MGE, medial ganglionic eminence; pETh, prethalamic eminence; PHy, peduncular hypothalamus; THy, terminal hypothalamus; P-SPB, pallial-subpallial boundary; Pt, pretectum; pTh, prethalamus; Th, thalamus.
We have closely followed the prosomeric model to describe and interpret the pathway of the TCAs in the wild-type and in the Gli2 mutant. Hence, our interpretation differs slightly from the previous published descriptions (López-Bendito and Molnár, 2003; Garel and López-Bendito, 2014; López-Bendito, 2018; Nakagawa, 2019). First, the trajectory in the diencephalon involves the alar plate of Th and pTh. Second, to enter into the secondary prosencephalon, the TCAs must cross the diencephalic (prethalamic)-secondary prosencephalon (PHy; Figure 7). In this area, the TCAs bend dorsally and get into the telencephalic vesicle (PHy-telencephalic boundary; Figure 7). Finally, the TCAs cross the pallial-subpallial boundary inside the telencephalic vesicle (López-Bendito and Molnár, 2003; Garel and López-Bendito, 2014).
Specification of the Territories Involved in Thalamocortical Axons Trajectory: Thalamus, Prethalamus, and Secondary Prosencephalon
The specification of two important prosomeres of the diencephalon, Th and pTh, is slightly altered in Gli2 mutant mice. In fact, the thalamocortical efferents development is a intricated process that depends on the accurate fate specification of thalamic neurons by a cascade of key transcription factor genes (López-Bendito and Molnár, 2003). Furthermore, it is shown that the Shh signaling pathway plays a role in the thalamic development (Kiecker and Lumsden, 2004; Vieira et al., 2005; Scholpp and Lumsden, 2010). The GLI transcription factors act downstream of Shh and cooperate to integrate Shh and other essential morphogenetic signals (Zhou et al., 2004; Kataoka and Shimogori, 2008). While Gli2 and Gli3 have transcriptional activator and repressor activities, Gli1 is mainly a transcriptional activator [reviewed by Ruiz i Altaba et al. (2007), Jetten (2018), and Niewiadomski et al. (2019)].
In the Gli2 zfd/zfd mutants, the Th is reduced (mostly in caudal regions) and not completely absent (Haddad-Tóvolli et al., 2012). Apparently, this is due to the Gli3 activator compensation (Gli3A). In the Gli2 absence, the Gli3 activate form can be induced by high levels of Shh signaling, and in combination with Gli1A, it can rescue at least partially the Gli2 loss of function (Bai et al., 2004). However, Gli3A is a weak activator (Dai et al., 1999; Shin et al., 1999; Litingtung and Chiang, 2000; Motoyama et al., 2003; Bai et al., 2004) and therefore, it cannot fully restore the Th proliferation and differentiation.
On the other hand, the pTh is an intermediate target of TCAs (Deng and Elberger, 2003; Molnár et al., 2012). There are evidences that Fgf10 plays an important dose-dependent role as a guidance cue in the pTh to direct the TCAs (Liu et al., 2020). In the Olig2-KO mouse or Rfx3-KO (ciliopathy) mouse diencephalon, it was described as a prethalamic malformation, followed by disorganized extension of TCAs (Ono et al., 2014; Magnani et al., 2015; Andreu-Cervera et al., 2021). So, the proper formation of the pTh is crucial for the correct extension of TCAs.
The alteration in the specification of the alar diencephalic territories by the loss of function of Gli2, therefore in an altered Shh signaling cascade, was expected. Being the Th and the pTh alar structures, it is plausible to believe that they would not be strongly affected by the lack of Shh signaling from the basal and floor plates. However, these two structures lay at both sides of the zona limitans intrathamica (zli). That is the main secondary organizer of the diencephalon. It secretes SHH as morphogen, so it is reasonable to think that any alteration of this signaling mechanism should strongly affect the patterning of these two structures. The capability of the Gli members to compensate each other could explain the weak phenotype of the diencephalon in the loss of function of Gli2.
The TCAs leave the pTh to reach the Hy in the caudal secondary prosencephalon. Our results indicate that alar and basal plates of Hy are strongly affected in Gli2 mutant mice. This altered phenotype sustains the reason for the defasciculation and misdirection of the TCAs as soon as they enter the PHy. One of the possible reasons for this altered course of the TCAs is the incorrect specification of the PHy and THy.
It was demonstrated that the Hy is a repulsive territory for TCAs, avoiding their ventral navigation in their route toward the telencephalon (Braisted et al., 1999). The morphogene Fgf10 has been recently related to this repulsive effect (Liu et al., 2020). Indeed, in Nkx2.1 mutant, the absence of the basal Hy does not impair thalamocortical projections due to the normal specification of the alar Hy (Marín et al., 2002). In the Gli2 mutant, the basal Hy is severely altered but not absent, this could explain that in our case, the TCAs are able to find a permissive territory to invade. The alteration of Gli genes results in the malformation or absence of the floor plate marked by the Shh target gene Hnf3β (Matise et al., 1998). Therefore, the Gli2 absence generates an altered basal plate that probably lacks the correct code of navigating signals.
In fact, mutations in the zebrafish gene you-too (yot; homologous of mammalian Gli2) affected the ventral forebrain patterning (Karlstrom et al., 1999). The alterations described in yot mutant embryos unveiled the role of Gli genes and the Hh signaling pathway in the ventral forebrain cell fate specification. These defects include the alteration of the cellular cues needed to guide the ventral forebrain midline cross by the axons and disrupt optic chiasm formation and optic nerve crossing. The defects described and the Shh involvement in human holoprosencephaly (for review, refer Ming and Muenke, 1998) suggest that Gli2 mutations might be responsible for human diseases such as facial and forebrain midline structures congenital malformations.
At last, some TCAs are able to leave the PHy and enter into the evaginated portion of the telencephalic vesicle. They reach the MGEs, in the presumptive territory of the corridor where they stop their course. We cannot exclude from our experiments that some residual TCAs are able to cross the LGE and finally reach the cortex, agreeing with the conclusions of Haddad-Tóvolli et al. (2012).
The specification of the ganglionic eminences in the Gli2 mutant is strongly affected, mainly the MGE. This is translated into the incapability of the TCAs to go across this territory. Surprisingly, a medial transformation into LGE with an expansion of the striatum, by the loss of Nkx2.1 function (Sussel et al., 1999), described the normal generation of the thalamocortical projections (Marín et al., 2002). A reasonable explanation for these two opposite phenotypes is that in the Nkx2.1 mutant, the LGE is completely unaffected and therefore the needed corridor is correctly formed. In the Gli2 mutant, we cannot exclude an alteration in the specification of this territory and an aberrant generation of the corridor; in fact, this structure appeared abnormally wide in our mutant. It has been demonstrated that the corridor, migrated cells from the LGE into the MGE, is necessary and sufficient to allow the correct trajectory of the TCAs (Casarosa et al., 1999; López-Bendito et al., 2006).
Signaling Mechanism Alterations in Thalamocortical Axons Pathfinding in Gli2 Mutant Mice
The previous studies in Gli2 mutants have reported errors in longitudinal axonal tract navigation in forebrain, midbrain, and hindbrain (Farmer et al., 2008; Mastick et al., 2010). These included midline crossing, anteroposterior misdirection, and dorsoventral repositioning. Therefore, these diverse phenotypes suggest the interaction of Gli2 with multiple guidance mechanisms (Farmer et al., 2008; Mastick et al., 2010).
In our mutant, the TCAs follow a normal trajectory until the PHy where they disorganize, misdirect, and grow in all directions. This behavior of the tract indicates an almost complete absence of its signaling sources. This territory of the secondary prosencephalon has severe defaults in its specification and this could therefore indirectly affect its signaling molecules cocktail.
The Th is a heterogeneous group of neuronal populations. Each of them has a unique combination of receptors that will supply them the capability to display different behaviors in front of the same signaling molecules (Leyva-Díaz and López-Bendito, 2013). This fact explains the diversity found in the analysis of our mutants; some TCAs followed a caudal direction, others a ventral direction, and finally some of them were able to get into the MGE.
The Robo-Slit repulsive signaling mechanism has been analyzed in the thalamic projections. It plays a pivotal role in the development of these projections, acting at all choice points along its pathway (Braisted et al., 1999; Leyva-Díaz and López-Bendito, 2013; Blockus and Chédotal, 2014). We found alterations in the expression patterns of Slit1 and Slit2, which could explain the TCAs misdirection. A Slit1 positive patch of expression was detected in the putative area of entrance to the telencephalic vesicle. The TCAs sensible to this molecule were repelled and redirected into caudal and ventral directions. The TCAs non-sensitive to Slit1 were able to enter into the MGE. The lack of Slit2 expression in the THy would explain the capability of the TCAs repelled by Slit1 to invade the Hy. This conclusion is also supported by the analysis of the TCAs trajectory in Pax6-KO mice in which their axons are able to reach the hypothalamic territory due to a strong reduction in their levels of Robo2 in the thalamic neurons (Clegg et al., 2015). A similar result has been recently described by the loss of c-Jun N-terminal kinase (JNK) signaling in the Dlx5/6 territory, the TCAs axons enter in the hypothalamic due to a total failure of the guidance cues and guideposts (Cunningham et al., 2021).
Conclusion
The alteration of the Shh signaling driven by Gli2 affects the specification of different territories related with the TCAs course. The PHy, Thy, and MGE are strongly affected. The LGE is also altered but in a lower degree, its alteration affects mainly the generation of the corridor. This erroneous specification of the territories produces huge modifications in the pathfinding signaling mechanisms of the TCAs.
Data Availability Statement
The original contributions presented in the study are included in the article/supplementary material, further inquiries can be directed to the corresponding author.
Ethics Statement
All mouse experiments were performed according to protocols approved by the Universidad Miguel Hernández de Elche OIR Committee (ref. 2014/VSC/PEA/0055).
Author Contributions
AC-M, JM-B, and EP conceived and designed the experiments and analyzed the data. AC-M, JM-B, VC, MM, and FA-G performed the experiments. AC-M, JM-B, SM, and EP wrote the article. SM and EP obtained funding. All authors had full access to all the data in the study and took responsibility for the integrity of the data and the accuracy of the data analysis.
Funding
This work was supported by the MINECO/AEI/FEDER (BFU2013-48230) to EP, MINECO/AEI/FEDER (PID2020-118171RB-I00), GVA (PROMETEO/2018/041), ISCIII (“RD16/001/0010”), co-funded by ERDF/ESF, “Investing in Your Future,” and FTPGB (FTPGB18/SM) to SM, MECD (FPU16/03853) to VC. Spanish Ministry of Science, Innovation and Universities (RYC2018-023868-I) to JM-B. The Institute of Neurosciences is a “Centre of Excellence Severo Ochoa (SEV-2017-0723).”
Conflict of Interest
The authors declare that the research was conducted in the absence of any commercial or financial relationships that could be construed as a potential conflict of interest.
Publisher’s Note
All claims expressed in this article are solely those of the authors and do not necessarily represent those of their affiliated organizations, or those of the publisher, the editors and the reviewers. Any product that may be evaluated in this article, or claim that may be made by its manufacturer, is not guaranteed or endorsed by the publisher.
References
Andreu-Cervera, A., Catala, M., and Schneider-Maunoury, S. (2021). Cilia, ciliopathies and hedgehog-related forebrain developmental disorders. Neurobiol. Dis. 150:105236. doi: 10.1016/J.NBD.2020.105236
Angevine, J. B. (1970). Time of neuron origin in the diencephalon of the mouse. an autoradiographic study. J. Comp. Neurol. 139, 129–187. doi: 10.1002/cne.901390202
Aza-Blanc, P., Lin, H. Y., Ruiz i Altaba, A., and Kornberg, T. B. (2000). Expression of the vertebrate Gli proteins in Drosophila reveals a distribution of activator and repressor activities. Development 127, 4293–4301. doi: 10.1242/dev.127.19.4293
Bai, C. B., Auerbach, W., Lee, J. S., Stephen, D., and Joyner, A. L. (2002). Gli2, but not Gli1, is required for initial Shh signaling and ectopic activation of the Shh pathway. Development 129, 4753–4761. doi: 10.1242/dev.129.20.4753
Bai, C. B., Stephen, D., and Joyner, A. L. (2004). All mouse ventral spinal cord patterning by Hedgehog is Gli dependent and involves an activator function of Gli3. Dev. Cell 6, 103–115. doi: 10.1016/S1534-5807(03)00394-0
Blockus, H., and Chédotal, A. (2014). The multifaceted roles of slits and robos in cortical circuits: from proliferation to axon guidance and neurological diseases. Curr. Opin. Neurobiol. 27, 82–88. doi: 10.1016/j.conb.2014.03.003
Blockus, H., and Chédotal, A. (2016). Slit-robo signaling. Development 143, 3037–3044. doi: 10.1242/dev.132829
Braisted, J. E., Catalano, S. M., Stimac, R., Kennedy, T. E., Tessier-Lavigne, M., Shatz, C. J., et al. (2000). Netrin-1 promotes thalamic axon growth and is required for proper development of the thalamocortical projection. J. Neurosci. 20, 5792–5801. doi: 10.1523/jneurosci.20-15-05792.2000
Braisted, J. E., Tuttle, R., and O’Leary, D. D. M. (1999). Thalamocortical axons are influenced by chemorepellent and chemoattractant activities localized to decision points along their path. Dev. Biol. 208, 430–440. doi: 10.1006/dbio.1999.9216
Bulfone, A., Puelles, L., Porteus, M. H., Frohman, M. A., Martin, G. R., and Rubenstein, J. L. R. (1993). Spatially restricted expression of Dlx-1, Dlx-2 (Tes-1), Gbx-2, and Wnt-3 in the embryonic day 12.5 mouse forebrain defines potential transverse and longitudinal segmental boundaries. J. Neurosci. 13, 3155–3172. doi: 10.1523/jneurosci.13-07-03155.1993
Casarosa, S., Fode, C., and Guillemot, F. (1999). Mash1 regulates neurogenesis in the ventral telencephalon. Development 126, 525–534. doi: 10.1242/dev.126.3.525
Castillo-Paterna, M., Moreno-Juan, V., Filipchuk, A., Rodríguez-Malmierca, L., Susín, R., and López-Bendito, G. (2015). DCC functions as an accelerator of thalamocortical axonal growth downstream of spontaneous thalamic activity. EMBO Rep. 16, 851–862. doi: 10.15252/embr.201439882
Clegg, J. M., Li, Z., Molinek, M., Caballero, I. M., Manuel, M. N., and and Price, D. J. (2015). Pax6 is required intrinsically by thalamic progenitors for the normal molecular patterning of thalamic neurons but not the growth and guidance of their axons. Neural Dev. 10:26 doi: 10.1186/s13064-015-0053-7
Cruikshank, S. J., Urabe, H., Nurmikko, A. V., and Connors, B. W. (2010). Pathway-specific feedforward circuits between thalamus and neocortex revealed by selective optical stimulation of axons. Neuron 65, 230–245. doi: 10.1016/J.NEURON.2009.12.025
Cunningham, J. G., Scripter, J. D., Nti, S. A., and and Tucker, E. S. (2021). Early construction of the thalamocortical axon pathway requires c-Jun N-terminal kinase signaling within the ventral forebrain. Dev. Dyn. doi: 10.1002/dvdy.416 Online ahead of print,
Dai, P., Akimaru, H., Tanaka, Y., Maekawa, T., Nakafuku, M., and Ishii, S. (1999). Sonic hedgehog-induced activation of the Gli1 promoter is mediated by GLI3. J. Biol. Chem. 274, 8143–8152. doi: 10.1074/jbc.274.12.8143
Deng, J., and Elberger, A. J. (2003). Corticothalamic and thalamocortical pathfinding in the mouse: dependence on intermediate targets and guidance axis. Anat. Embryol. 207, 177–192. doi: 10.1007/s00429-003-0338-1
Dickson, B. J. (2002). Molecular mechanisms of axon guidance. Science 298, 1959–1964. doi: 10.1126/science.1072165
Ding, Q., Balasubramanian, R., Zheng, D., Liang, G., and Gan, L. (2017). Barhl2 determines the early patterning of the diencephalon by regulating Shh. Mol. Neurobiol. 54, 4414–4420. doi: 10.1007/s12035-016-0001-5
Epstein, D. J. (2012). Regulation of thalamic development by sonic hedgehog. Front. Neurosci. 6:57. doi: 10.3389/FNINS.2012.00057
Farmer, W. T., Altick, A. L., Nural, H. F., Dugan, J. P., Kidd, T., Charron, F., et al. (2008). Pioneer longitudinal axons navigate using floor plate and Slit/Robo signals. Development 135, 3643–3653. doi: 10.1242/dev.023325
Garel, S., and López-Bendito, G. (2014). Inputs from the thalamocortical system on axon pathfinding mechanisms. Curr. Opin. Neurobiol. 27, 143–150. doi: 10.1016/J.CONB.2014.03.013
Garel, S., and Rubenstein, J. L. R. (2004). Intermediate targets in formation of topographic projections: inputs from the thalamocortical system. Trends Neurosci. 27, 533–539. doi: 10.1016/j.tins.2004.06.014
Haddad-Tóvolli, R., Heide, M., Zhou, X., Blaess, S., and Alvarez-Bolado, G. (2012). Mouse thalamic differentiation: gli-dependent pattern and gli-independent prepattern. Front. Neurosci. 6:27. doi: 10.3389/fnins.2012.00027
Hui, C. C., Slusarski, D., Platt, K. A., Holmgren, R., and and Joyner, A. L. (1994). Expression of three mouse homologs of the Drosophila segment polarity gene cubitus interruptus, Gli, Gli-2, and Gli-3, in ectoderm- and mesoderm-derived tissues suggests multiple roles during postimplantation development. Dev. Biol. 162, 402–413. doi: 10.1006/DBIO.1994.1097
Ingham, P. W., and McMahon, A. P. (2001). Hedgehog signaling in animal development: paradigms and principles. Genes Dev. 15, 3059–3087. doi: 10.1101/gad.938601
Jacob, J., and Briscoe, J. (2003). Gli proteins and the control of spinal-cord patterning. EMBO Rep. 4, 761–765. doi: 10.1038/SJ.EMBOR.EMBOR896
Jeong, Y., Dolson, D. K., Waclaw, R. R., Matise, M. P., Sussel, L., Campbell, K., et al. (2011). Spatial and temporal requirements for sonic hedgehog in the regulation of thalamic interneuron identity. Development 138, 531–541. doi: 10.1242/DEV.058917/-/DC1
Jetten, A. M. (2018). GLIS1–3 transcription factors: critical roles in the regulation of multiple physiological processes and diseases. Cell. Mol. Life Sci. 75, 3473–3494. doi: 10.1007/S00018-018-2841-9
Jones, E. G. (2007). The Thalamus 2 Volume Set. 1708. Available online at: http://books.google.de/books?id=IR0fSgAACAAJ (accessed December 2, 2021).
Karlstrom, R. O., Talbot, W. S., and Schier, A. F. (1999). Comparative synteny cloning of zebrafish you-too: mutations in the hedgehog target gli2 affect ventral forebrain patterning. Genes Dev. 13, 388–393. doi: 10.1101/gad.13.4.388
Kataoka, A., and Shimogori, T. (2008). Fgf8 controls regional identity in the developing thalamus. Development 135, 2873–2881. doi: 10.1242/dev.021618
Kiecker, C., and Lumsden, A. (2004). Hedgehog signaling from the ZLI regulates diencephalic regional identity. Nat. Neurosci. 7, 1242–1249. doi: 10.1038/nn1338
Lee, J., Platt, K. A., Censullo, P., and Ruiz I Altaba, A. (1997). Gli1 is a target of Sonic hedgehog that induces ventral neural tube development. Development 124, 2537–2552. doi: 10.1242/dev.124.13.2537
Leyva-Díaz, E., and López-Bendito, G. (2013). In and out from the cortex: development of major forebrain connections. Neuroscience 254, 26–44. doi: 10.1016/j.neuroscience.2013.08.070
Litingtung, Y., and Chiang, C. (2000). Control of Shh activity and signaling in the neural tube. Dev. Dyn. 219, 143–154. doi: 10.1002/1097-017720009999:9999<::aid-dvdy1050<3.3.CO;2-h
Liu, K., Lv, Z., Huang, H., Li, M., Xiao, L., Li, X., et al. (2020). FGF10 regulates thalamocortical axon guidance in the developing thalamus. Neurosci. Lett. 716:134685. doi: 10.1016/j.neulet.2019.134685
Logan, C. Y., and Nusse, R. (2004). The Wnt signaling pathway in development and disease. Annu. Rev. Cell Dev. Biol. 20, 781–810. doi: 10.1146/ANNUREV.CELLBIO.20.010403.113126
López-Bendito, G. (2018). Development of the thalamocortical interactions: past, present and future. Neuroscience 385, 67–74. doi: 10.1016/j.neuroscience.2018.06.020
López-Bendito, G., Cautinat, A., Sánchez, J. A., Bielle, F., Flames, N., Garratt, A. N., et al. (2006). Tangential neuronal migration controls axon guidance: a role for neuregulin-1 in thalamocortical axon navigation. Cell 125, 127–142. doi: 10.1016/j.cell.2006.01.042
López-Bendito, G., and Molnár, Z. (2003). Thalamocortical development: how are we going to get there? Nat. Rev. Neurosci. 4, 276–289. doi: 10.1038/nrn1075
Magnani, D., Morlé, L., Hasenpusch-Theil, K., Paschaki, M., Jacoby, M., Schurmans, S., et al. (2015). The ciliogenic transcription factor Rfx3 is required for the formation of the thalamocortical tract by regulating the patterning of prethalamus and ventral telencephalon. Hum. Mol. Genet. 24, 2578–2593. doi: 10.1093/hmg/ddv021
Marín, O., Baker, J., Puelles, L., and Rubenstein, J. L. R. (2002). Patterning of the basal telencephalon and hypothalamus is essential for guidance of cortical projections. Development 129, 761–773. doi: 10.1242/dev.129.3.761
Martí, E., and Bovolenta, P. (2002). Sonic hedgehog in CNS development: one signal, multiple outputs. Trends Neurosci. 25, 89–96. doi: 10.1016/S0166-2236(02)02062-3
Martinez-Ferre, A., and Martinez, S. (2012). Molecular regionalization of the diencephalon. Front. Neurosci. 6:73. doi: 10.3389/fnins.2012.00073
Martinez-Lopez, J. E., Moreno-Bravo, J. A., Madrigal, M. P., Martinez, S., and Puelles, E. (2015). Red nucleus and rubrospinal tract disorganization in the absence of Pou4f1. Front. Neuroanat. 9:8. doi: 10.3389/fnana.2015.00008
Mastick, G. S., Farmer, W. T., Altick, A. L., Nural, H. F., Dugan, J. P., Kidd, T., et al. (2010). Longitudinal axons are guided by Slit/Robo signals from the floor plate. Cell Adh. Migr. 4, 337–341. doi: 10.4161/cam.4.3.11219
Matise, M. P., Epstein, D. J., Park, H. L., Platt, K. A., and Joyner, A. L. (1998). Gli2 is required for induction of floor plate and adjacent cells, but not most ventral neurons in the mouse central nervous system. Development 125, 2759–2770. doi: 10.1242/dev.125.15.2759
Ming, J. E., and Muenke, M. (1998). Holoprosencephaly: from homer to hedgehog. Clin. Genet. 53, 155–163. doi: 10.1111/j.1399-0004.1998.tb02666.x
Minlebaev, M., Colonnese, M., Tsintsadze, T., Sirota, A., and Khazipov, R. (2011). Early gamma oscillations synchronize developing thalamus and cortex. Science 334, 226–229. doi: 10.1126/science.1210574
Mitsogiannis, M. D., Little, G. E., and Mitchell, K. J. (2017). Semaphorin-Plexin signaling influences early ventral telencephalic development and thalamocortical axon guidance. Neural Dev. 12:6. doi: 10.1186/s13064-017-0083-4
Miyashita-Lin, E. M., Hevner, R., Wassarman, K. M., Martinez, S., and Rubenstein, J. L. R. (1999). Early neocortical regionalization in the absence of thalamic innervation. Science 285, 906–909. doi: 10.1126/science.285.5429.906
Mo, R., Freer, A. M., Zinyk, D. L., Crackower, M. A., Michaud, J., Heng, H. H. Q., et al. (1997). Specific and redundant functions of Gli2 and Gli3 zinc finger genes in skeletal patterning and development. Development 124, 113–123. doi: 10.1242/dev.124.1.113
Molnár, Z., Garel, S., López-Bendito, G., Maness, P., and Price, D. J. (2012). Mechanisms controlling the guidance of thalamocortical axons through the embryonic forebrain. Eur. J. Neurosci. 35, 1573–1585. doi: 10.1111/j.1460-9568.2012.08119.x
Molnár, Z., Higashi, S., and López-Bendito, G. (2003). Choreography of early thalamocortical development. Cereb. Cortex 13, 661–669. doi: 10.1093/cercor/13.6.661
Motoyama, J., Milenkovic, L., Iwama, M., Shikata, Y., Scott, M. P., and Hui, C. C. (2003). Differential requirement for Gli2 and Gli3 in ventral neural cell fate specification. Dev. Biol. 259, 150–161. doi: 10.1016/S0012-1606(03)00159-3
Nakagawa, Y. (2019). Development of the thalamus: from early patterning to regulation of cortical functions. Wiley Interdiscip. Rev. Dev. Biol. 8:e345. doi: 10.1002/wdev.345
Niewiadomski, P., Niedziółka, S. M., Markiewicz, Ł., Uśpieński, T., Baran, B., and Chojnowska, K. (2019). Gli proteins: regulation in development and cancer. Cells 8:147. doi: 10.3390/CELLS8020147
Ono, K., Clavairoly, A., Nomura, T., Gotoh, H., Uno, A., Armant, O., et al. (2014). Development of the prethalamus is crucial for thalamocortical projection formation and is regulated by Olig2. Development 141, 2075–2084. doi: 10.1242/dev.097790
Pan, Y., Bai, C. B., Joyner, A. L., and Wang, B. (2006). Sonic hedgehog signaling regulates Gli2 transcriptional activity by suppressing its processing and degradation. Mol. Cell. Biol. 26, 3365–3377. doi: 10.1128/mcb.26.9.3365-3377.2006
Poulet, J. F. A., Fernandez, L. M. J., Crochet, S., and Petersen, C. C. H. (2012). Thalamic control of cortical states. Nat. Neurosci. 15, 370–372. doi: 10.1038/nn.3035
Powell, A. W., Sassa, T., Wu, Y., Tessier-Lavigne, M., and Polleux, F. (2008). Topography of thalamic projections requires attractive and repulsive functions of netrin-1 in the ventral telencephalon. PLoS Biol. 6:e116. doi: 10.1371/journal.pbio.0060116
Price, D. J., Kennedy, H., Dehay, C., Zhou, L., Mercier, M., Jossin, Y., et al. (2006). The development of cortical connections. Eur. J. Neurosci. 23, 910–920. doi: 10.1111/j.1460-9568.2006.04620.x
Puelles, L., Amat, J. A., and Martinez-de-la-Torre, M. (1987). Segment-related, mosaic neurogenetic pattern in the forebrain and mesencephalon of early chick embryos: I. Topography of ache-positive neuroblasts up to stage HH18. J. Comp. Neurol. 266, 247–268. doi: 10.1002/cne.902660210
Puelles, L., and Rubenstein, J. L. R. (1993). Expression patterns of homeobox and other putative regulatory genes in the embryonic mouse forebrain suggest a neuromeric organization. Trends Neurosci. 16, 472–479. doi: 10.1016/0166-2236(93)90080-6
Puelles, L., and Rubenstein, J. L. R. (2003). Forebrain gene expression domains and the evolving prosomeric model. Trends Neurosci. 26, 469–476. doi: 10.1016/S0166-2236(03)00234-0
Quintana-Urzainqui, I., Hernández-Malmierca, P., Clegg, J. M., Li, Z., Kozić, Z., and Price, D. J. (2020). The role of the diencephalon in the guidance of thalamocortical axons in mice. Development 147:dev184523. doi: 10.1242/dev.184523
Ruiz i Altaba, A. (1998). Combinatorial Gli gene function in floor plate and neuronal inductions by sonic hedgehog. Development 125, 2203–2212. doi: 10.1242/dev.125.12.2203
Ruiz I Altaba, A. (1999). Gli proteins encode context-dependent positive and negative functions: implications for development and disease. Development 126, 3205–3216. doi: 10.1242/dev.126.14.3205
Ruiz i Altaba, A., Mas, C., and Stecca, B. (2007). The Gli code: an information nexus regulating cell fate, stemness and cancer. Trends Cell Biol. 17, 438–447. doi: 10.1016/j.tcb.2007.06.007
Scholpp, S., and Lumsden, A. (2010). Building a bridal chamber: development of the thalamus. Trends Neurosci. 33, 373–380. doi: 10.1016/j.tins.2010.05.003
Shimogori, T., and Grove, E. A. (2005). Fibroblast growth factor 8 regulates neocortical guidance of area-specific thalamic innervation. J. Neurosci. 25, 6550–6560. doi: 10.1523/JNEUROSCI.0453-05.2005
Shin, S. H., Kogerman, P., Lindström, E., Toftgárd, R., and Biesecker, L. G. (1999). GLI3 mutations in human disorders mimic Drosophila cubitus interruptus protein functions and localization. Proc. Natl. Acad. Sci. U.S.A. 96, 2880–2884. doi: 10.1073/PNAS.96.6.2880
Sussel, L., Marin, O., Kimura, S., and Rubenstein, J. L. R. (1999). Loss of Nkx2.1 homeobox gene function results in a ventral to dorsal molecular respecification within the basal telencephalon: evidence for a transformation of the pallidum into the striatum. Development 126, 3359–3370. doi: 10.1242/dev.126.15.3359
Tessier-Lavigne, M., and Goodman, C. S. (1996). The molecular biology of axon guidance. Science 274, 1123–1133. doi: 10.1126/science.274.5290.1123
Theyel, B. B., Llano, D. A., and Sherman, S. M. (2010). The corticothalamocortical circuit drives higher-order cortex in the mouse. Nat. Neurosci. 13, 84–88. doi: 10.1038/nn.2449
Vieira, C., Garda, A. L., Shimamura, K., and Martinez, S. (2005). Thalamic development induced by Shh in the chick embryo. Dev. Biol. 284, 351–363. doi: 10.1016/J.YDBIO.2005.05.031
Vue, T. Y., Bluske, K., Alishahi, A., Yang, L. L., Koyano-Nakagawa, N., Novitch, B., et al. (2009). Sonic hedgehog signaling controls thalamic progenitor identity and nuclei specification in mice. J. Neurosci. 29, 4484. doi: 10.1523/JNEUROSCI.0656-09.2009
Keywords: thalamocortical axons, guidance, Gli2, Slit1, Slit2
Citation: Callejas-Marin A, Moreno-Bravo JA, Company V, Madrigal MP, Almagro-García F, Martínez S and Puelles E (2022) Gli2-Mediated Shh Signaling Is Required for Thalamocortical Projection Guidance. Front. Neuroanat. 16:830758. doi: 10.3389/fnana.2022.830758
Received: 07 December 2021; Accepted: 13 January 2022;
Published: 10 February 2022.
Edited by:
Fernando de Castro, Cajal Institute, Spanish National Research Council (CSIC), SpainReviewed by:
Julien Ferent, Institut National de la Santé et de la Recherche Médicale (INSERM), FranceElisabeth Traiffort, Institut National de la Santé et de la Recherche Médicale (INSERM), France
Copyright © 2022 Callejas-Marin, Moreno-Bravo, Company, Madrigal, Almagro-García, Martínez and Puelles. This is an open-access article distributed under the terms of the Creative Commons Attribution License (CC BY). The use, distribution or reproduction in other forums is permitted, provided the original author(s) and the copyright owner(s) are credited and that the original publication in this journal is cited, in accordance with accepted academic practice. No use, distribution or reproduction is permitted which does not comply with these terms.
*Correspondence: Eduardo Puelles, ZXB1ZWxsZXNAdW1oLmVz
†These authors have contributed equally to this work