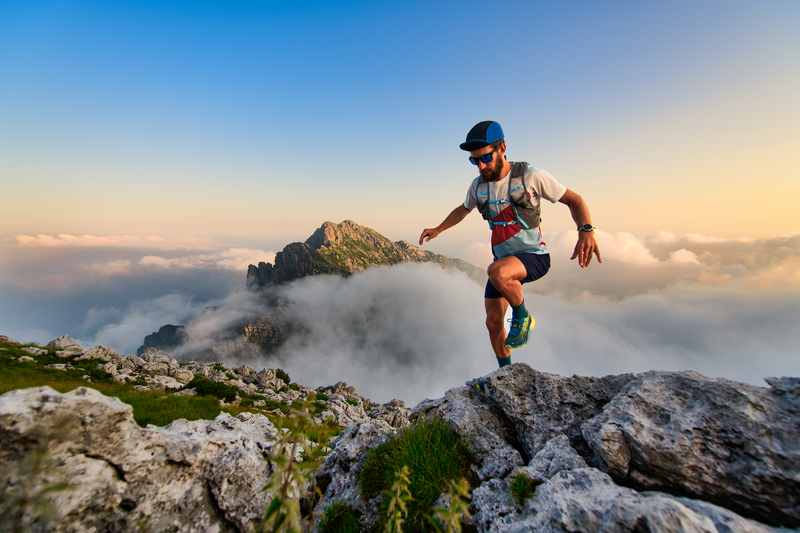
95% of researchers rate our articles as excellent or good
Learn more about the work of our research integrity team to safeguard the quality of each article we publish.
Find out more
REVIEW article
Front. Neuroanat. , 02 April 2015
Volume 9 - 2015 | https://doi.org/10.3389/fnana.2015.00032
This article is part of the Research Topic Parkinson's Disease: Cell Vulnerability and Disease Progression View all 19 articles
Chronic inflammation is a major characteristic feature of Parkinson’s disease (PD). Studies in PD patients show evidence of augmented levels of potent pro-inflammatory molecules e.g., TNF-α, iNOS, IL-1β whereas in experimental Parkinsonism it has been consistently demonstrated that dopaminergic neurons are particularly vulnerable to activated glia releasing these toxic factors. Recent genetic studies point to the role of immune system in the etiology of PD, thus in combination with environmental factors, both peripheral and CNS-mediated immune responses could play important roles in onset and progression of PD. Whereas microglia, astrocytes and infiltrating T cells are known to mediate chronic inflammation, the roles of other immune-competent cells are less well understood. Inflammation is a tightly controlled process. One major effector system of regulation is HPA axis. Glucocorticoids (GCs) released from adrenal glands upon stimulation of HPA axis, in response to either cell injury or presence of pathogen, activate their receptor, GR. GR regulates inflammation both through direct transcriptional action on target genes and by indirectly inhibiting transcriptional activities of transcriptional factors such as NF-κB, AP-1 or interferon regulatory factors. In PD patients, the HPA axis is unbalanced and the cortisol levels are significantly increased, implying a deregulation of GR function in immune cells. In experimental Parkinsonism, the activation of microglial GR has a crucial effect in diminishing microglial cell activation and reducing dopaminergic degeneration. Moreover, GCs are also known to regulate human brain vasculature as well as blood brain barrier (BBB) permeability, any dysfunction in their actions may influence infiltration of cytotoxic molecules resulting in increased vulnerability of dopamine neurons in PD. Overall, deregulation of glucocorticoid receptor actions is likely important in dopamine neuron degeneration through establishment of chronic inflammation.
Parkinson’s disease (PD) is a common age-related neurodegenerative disorder characterized by cardinal motor symptoms that include bradykinesia with resting tremor, rigidity and gait disturbance. These motor symptoms become evident when already 70–80% of nigrostriatal terminals have degenerated. At present, therapeutic treatments, for example, levodopa, mostly address motor symptoms. However, a wide spectrum of non-motor clinical features such as REM (Rapid eye movement) sleep disturbances, autonomic dysfunction, depression, anxiety, cognitive impairment or falling are associated with PD, and are moreover debilitating and unresponsive to dopamine-related treatments. Thus, PD is a complex systemic disorder with non-motor symptoms often preceding motor symptoms and worsening with disease progression (Berg et al., 2014; Goldman and Postuma, 2014). PD has an age-adjusted incidence of 13.5–13.9 per 100.000 person-years, and a prevalence of 315 per 100,000 individuals (the second most common worldwide). As PD affects predominantly older people, its prevalence increases with age from 428 at 60–69 years to 1,903 per 100.000 in 80 years old people (Abdullah et al., 2014; Pringsheim et al., 2014).
The major neuropathological hallmarks of PD are progressive degeneration of dopaminergic neurons in the Substantia Nigra pars compacta (SNpc); presence of proteinaceous inclusions called Lewy bodies (LBs) and chronic inflammation. However, the initial causes and underlying mechanisms pertaining to these neuropathological features in the majority of patients, classified as sporadic PD, remain unknown. Recent studies indicate that combined genetic, environmental factors and aging confer risk for developing sporadic PD rather than genetic or environmental factor individually. Approximately 5–10% of PD patients present the familial form of the disease with either autosomal dominant or recessive mode of inheritance. Epidemiological analysis confirm that up to 40% of PD patients with age at onset of less than 30 years and 17% of those with age at onset of less than 50 years will probably present the familial form of the disease. At least 18 loci as well as 12 genes with Mendelian inheritance and highly penetrant mutations causing rare monogenic forms have been identified. The genetic discovery of point mutations, duplication or triplication of SNCA (synuclein) gene coding for α-synuclein protein (reviewed in Goedert et al., 2013) with demonstration by Spillantini et al. (1997) that α-synuclein is a major component of LBs, led Braak et al. (2003) to staging PD according to appearance of α-synuclein containing LBs and Lewy neuritis with disease severity. Accordingly to Braak’s hypothesis PD progresses in neuronally-connected ascending manner to dorsal motor nucleus of the glossopharyngeal and vagus nerves likely from gut and/or olfactory mucosa (stage 1 and 2), then from lower brain stem to midbrain including nigral regions (stage 3 and 4) and lastly to the neocortical regions (stage 5 and 6). However, it has been suggested that LB pathology alone is not sufficient and that associated neuronal loss leads to Parkinsonism (Buchman et al., 2012).
In last few years, large genetic and genome-wide association (GWA) studies together with meta-analyses have led to significant and rapid advances in the genetic basis of sporadic PD, with realization both for its wide implication and complexity (Lill et al., 2012; Clarimón and Kulisevsky, 2013). There is great expectation for further insights with advent of new DNA sequencing technologies (exome and whole-genome sequencing) and the NeuroX genotyping platform (Nalls et al., 2015). These studies have identified at least 20 susceptibility loci as risk for sporadic PD (Nalls et al., 2014). As yet, the true significance of many of these loci is still unknown. Of interest, susceptibility related to SNCA and LRRK2 (Leucine rich repeat kinase 2) loci consistently observed are also genes that have been identified in the monogenic, autosomal dominant familial PD patients. Occurrence of somatic mosaicism has been also hypothesized in the etiology of some cases of PD (Kim and Jeon, 2014). Cases of somatic mosaicism in many central nervous system (CNS) disorders have been reported, for example, somatic mutation in the presenilin-1 gene associated with Alzheimer’s disease (Beck et al., 2004), in SPG4/SPAST (spastic paraplegia4/spastin) causing spastic paraplegia (Depienne et al., 2007) or MECP2 (methyl CpG binding protein) resulting in Rett syndrome (Topçu et al., 2002). Thus far, no cases of PD with somatic mosaicism are known, as well, in this regard results of study on SNCA somatic mutations by Proukakis et al. (2014) were negative.
Summing up: (a) although at present there is a rapid progress and evolution in technology to unravel genetic basis of PD, most PD risk is not understood; (b) the pathogenicity arising from several of identified gene mutations remains to be determined; (c) highly penetrant gene mutations (as DJ-1, LRRK2, Parkin, PINK1 (PTEN-induced putative kinase 1), and SNCA) cause rare monogenic forms of the disease; (d) somatic mosaicism could shed light on the heterogeneity of PD; and (e) additive mechanisms is suggested in risk for PD, increasing with the number of risk alleles carried by a single subject e.g., in HLA (human leucocyte antigen) region (Hill-Burns et al., 2011).
There is strong epidemiological evidence to show that aging is a single most important risk factor for PD, with increase in incidence between fifth to eight decades. Modifications that occur in specific brain regions during aging, such as increased oxidative and nitrative stress, changes in glial functions, dysfunction of proteasomes and lysosomes and altered α-synuclein protein are also manifestations of PD (Collier et al., 2011; Kieburtz and Wunderle, 2013). Environmental toxins identified as risk for PD are herbicides (e.g., paraquat or rotenone), heavy metals such as manganese and lead, nanoparticles as air pollutants, head trauma or well water. Thus, for example, it was shown that people exposed to pesticides and harboring Cytochrome P450 2D6 (CYP2D6) genotype with poor metabolic capacity for xenobiotics are at increased risk for developing PD (Elbaz et al., 2004). Epidemiologic link also exists between rotenone and PD. Rotenone is a powerful inhibitor of mitochondrial complex 1 and interestingly complex I deficiency is found in PD. The role of viral infections as risk factor has been evoked ever since the famous and controversial von Economo’s encephalitis lethargica pandemic suspected to be caused by H1N1 (Hemagglutinin1 neuraminidase1) influenza virus where patients exhibited Parkinsonism symptoms (Ravenholt and Foege, 1982). Recently, animals infected with highly pathogenic H5N1 virus showed clear motor deficits as well human cases with encephalitis have been reported (Jang et al., 2009). Epigenetic modifiers could be potential mediators of environmental factors (Portela and Esteller, 2010). Aberrant epigenetic modifications include changes in gene functions or gene expression but without changing the DNA sequence: non-coding RNA-mediated changes of gene expression, DNA methylation or post-transcriptional modifications and acetylation of histones (Nalls et al., 2014). For instance, the methylation of the Tumor Necrosis Factor alpha (TNF-α) promoter is significantly decreased in the SNpc of PD patients compared with controls or with the methylation in the cortex (Pieper et al., 2008) suggesting increased susceptibility of dopamine neurons to TNF-α mediated inflammation (Barcia et al., 2005, 2011).
Thus, aging together with genetic susceptibility and cumulative environmental factors such as air pollutants, pesticides, infections or exposure to heavy metals likely have a role in the development of idiopathic PD.
All of the above environmental factors together with multiple cellular changes occurring during aging can impact immune functions. There is now a growing realization, particularly from genetic studies, that immune system is most likely involved in the etiology as well as early phases of PD, thus the inflammatory component of the disease may simply not be a consequence of neuronal dysfunction and neurodegeneration. In GWA studies, a number of susceptibility loci that have been identified as strong risk factors, are related to both innate and adaptive immune functions, for example, HLA-DQB1, LRRK2, GPNMB (glycoprotein NMB), or BST-1 (bone marrow stromal cell antigen) (Liu et al., 2011; Pihlstrøm et al., 2013). In this regard, LRRK2, Parkin, PLA2G6 (phospholipase A2, group VI), DJ-1 and SNCA genes mutated in both familial and idiopathic PD are also known to function in microglia and astrocytes (Russo et al., 2014). Interestingly, several studies have identified risk of PD with polymorphisms present in the promoter regions of IL-1β and TNF-α genes that augment the expression of these genes and whose protein products have potent pro-inflammatory activity (Wahner et al., 2007). Moreover, polymorphisms reported in other pro-inflammatory genes e.g., CD14, HLA-DBQ1, HLA-DRA, HLA-DRB1, HLA-DRB5 can also increase the risk for PD (Ahmed et al., 2012). In the analysis of potential markers of motor and cognitive progression, SNPrs 6482992 of clarin3 (CLRN3) was described as the best predictor of cognitive deterioration (Chung et al., 2012) whereas SNPrs 10958605 as involved in neuroinflammatory pathways (Cappellano et al., 2013). The implication of early involvement of immune system is also reinforced by epidemiological studies showing a prolonged use of NSAIDs (Nonsteroidal anti-inflammatory) particularly ibruprofen subsequently lowers the risk of PD (Rees et al., 2011).
As a progressive neurodegenerative disorder, PD is a multifactorial complex disease most likely evolving because of the genetic and environmental risk factors, as well as cellular alterations and aging. Inflammatory component in PD not only encompasses deregulation of inflammatory pathways resulting from genetic vulnerability but also immune alterations associated with aging and with primary activation of glia in the face of neuronal injury. Aging affects the functions of immune system, resulting in so-called “immune senescence”. Specifically, advancing age has been associated with chronic mild inflammation in the SNpc, thereby rendering dopaminergic neurons vulnerable to degeneration (Kanaan et al., 2010). Increasing evidence points to the role of active peripheral inflammation in PD that can contribute to the initiation and/or the progression of the disease by, for example, exacerbating and synergizing with the discordant central inflammatory response to drive dopaminergic neurodegeneration. Combination of aging, heritable risk factors and exposure to environmental agents has been suggested as potential host-pathogen specific pathophysiologic elements that can cause deregulation of both innate and adaptive immune system responses (Kanaan et al., 2008; Chao et al., 2014). Thus, in both sporadic and familial PD, immune activation occurring at multiple levels would play an important role in PD pathology.
Evidence of an on-going neuroinflammation in affected brain regions in PD stems from analyses of pro-inflammatory cytokines (Interferon gamma, IFN-γ; TNF-α; Interleukin-6, IL-6; or Interleukin-1β, IL-1β) showing their accumulation in both cerebrospinal fluid and post-mortem brain (Mogi et al., 1994; Dobbs et al., 1999; Reale et al., 2009a,b). Recently, it has been demonstrated that the serum levels of IL-6 and the chemokine ligand 5 (CCL5) also known as Regulated on Activation, Normal T cell Expressed and Secreted (RANTES) were significantly increased in PD patients, and importantly, RANTES levels correlated with the severity and duration of the disease (Tang et al., 2014). Furthermore, the augmentation of iNOS (Inducible nitric oxide synthase) observed in SN and striatum of PD (Hunot et al., 1996) suggests that the toxicity originating both from cytokines/chemokines and inflammation-derived oxidative stress could contribute to dopaminergic neuronal degeneration and progression of the disease (Orr et al., 2005; Wilms et al., 2007). Numerous studies in experimental PD models indicate that dopamine neurons are particularly vulnerable to both oxidative stress and inflammatory attack (McGeer and McGeer, 2008; Pott Godoy et al., 2008). Interestingly, in this regard, Lipopolysaccharide (LPS)-activated microglia in the vicinity of dopamine neurons in SN induce degeneration of these neurons whilst sparing GABAergic and serotonergic neurons, suggesting a selective dopamine neuron vulnerability to inflammation (Liu and Bing, 2011).
Inflammation and immune-related responses may be viewed not only as determinant factors in disease progression but also as pathogenic processes in the onset of both familial and sporadic PD (Halliday and Stevens, 2011; Chao et al., 2014; Dzamko et al., 2014). On this point, presence of activated microglia, visualized by PET (positron emission tomography) analysis using radioligand 11C-PK-11195, was recently reported in the SN and putamen of PD patients diagnosed within a year from clinical onset (Iannaccone et al., 2013). This, together with study of Ouchi et al. (Ouchi et al., 2005) suggests a microglial-mediated inflammatory process in early stage of PD. Several lines of evidence also point to relevant actions of different PD-linked gene mutations e.g., SNCA or LRRK2 in stimulating inflammatory responses through activation of microglia and astrocytes thereby participating directly in chronic PD progression (Gillardon et al., 2012; Moehle et al., 2012; Harms et al., 2013). Both central and peripheral inflammation occurs in the prodromal stage of PD, which thus sustains disease progression (Dzamko et al., 2014; Su and Federoff, 2014). Overall, accumulation of pathological α-synuclein in PD brain leads to neurodegeneration with T-cell infiltration, microglial activation and increased production of inflammatory cytokines and chemokines (Harms et al., 2013). The detection of T lymphocytes and activated microglia in the SN of Parkinsonian patients is striking because systemic immune cells have to penetrate several barriers in order to reach the brain parenchyma.
The CNS was considered as an immunologically privileged site because of the lack of lymphatic vessels, the absence of classical major histocompatibility complex (MHC) positive antigen presenting cells, and the presence of barriers as the tanycytic barrier around the circumventricular organs or the neurovascular unit of the BBB. The latter is composed of endothelial cells, pericytes and astrocytes and associated strong, tight junctions prevent the entry of immune cells into the brain parenchyma. BBB is a metabolic and physical barrier that separates the CNS from the peripheral circulation, actively allowing the transports of nutrients to the brain but limiting passive diffusion of blood-borne solutes. However, in aging and in PD, a BBB disruption has been described with loss of the barrier permeability leading to secondary leukocyte migration within the brain parenchyma, reactive gliosis and damage to neurons (Stolp and Dziegielewska, 2009; Cabezas et al., 2014). BBB dysfunction in PD favors an invasion of immune cells (and/or peripheral mediators and factors as toxins or elements of adaptive immunity) into the brain parenchyma that provokes a progressive and self-perpetuating degenerative process (Monahan et al., 2008). Additionally, it has been demonstrated that PD patients have increased permeability of the intestinal epithelial barrier (Forsyth et al., 2011) as well as chronic enteric/colonic inflammation (Devos et al., 2013). As proposed by Braak et al. (2003), an environmental pathogen can cross the monolayer of polarized epithelial cells (the intestinal epithelial barrier) (Sharkey and Savidge, 2014) and enter into the terminal axons of the submucosal plexus spreading to the medulla oblongata via the vagal preganglionic innervation of the gut (Hawkes et al., 2009). Moreover, brain injuries or systemic infections can induce systemic inflammatory responses that easily communicate with brain. Both Alzheimer’s disease and PD have been associated with both the HLA region (Ahmed et al., 2012; Wissemann et al., 2013), and with the production of autoantibodies (Maetzler et al., 2014) suggesting putative genetic susceptibility to inflammation that could initiate the neuronal dysfunction.
Microglia are the resident innate immune cells in the brain. Being only 5–15% of the total cells of the brain, microglia functions include tissue repair and cellular homeostasis after neuronal injury. Activated microglia produce neurotoxic molecules, for example, pro-inflammatory cytokines, chemokines, complement proteins or nitric oxide. Additionally, activated microglia acquire phagocytic properties and develop neuro-immune interactions involving the expression of surface molecules as CD200/CD200R, CD47/CD172a, CX3C chemokine ligand 1 and its receptor (CX3CL1/CX3CR) and the complement regulatory proteins, complement components C1q and C3 in order to eliminate cellular debris and damaged neurons by gliapses (Barcia et al., 2012). However, microglial responses can have neuroprotective as well as harmful consequences mainly if there is a continuous exposure to a pro-inflammatory environment with a persistent release of inflammatory mediators (Bardou et al., 2014) as activated microglia can still persist even years after the toxic insults (Barcia et al., 2004; Jackson-Lewis and Smeyne, 2005; Block et al., 2007). In fact, if as a defense mechanism of the organism, an inflammatory response starts and continues without control, a chronic persistent inflammation environment in the brain can result in tissue destruction and progressive neurodegeneration.
Inflammation is normally a tightly regulated process that acts to prevent pathogen invasion as well as cellular injury, whilst at the same time enabling tissue repair. Several endogenous mechanisms act to regulate the immune cell functions, which are involved in triggering an inflammatory process. Among them, the steroid hormone, glucocorticoid, is a known major regulator of immune system and inflammation. Glucocorticoids (GCs) are one of the most potent and effective anti-inflammatory agents in clinical use ever since the isolation of cortisone and its clinical application in the early 1950s by the Nobel Prize winners Hench, Kendall and Reichstein (Hench et al., 1950; Reichstein, 1951).
GCs (cortisol in humans and corticosterone in rodents) are endogenous steroid hormones synthesized in adrenal glands and secreted into systemic circulation. The GC secretion occurs in ultradian pulsatile manner (Hellman et al., 1970; Veldhuis et al., 1989) and over-riding this pattern is acute GC rise in response to a stressor (psychogenic or physical e.g., tissue injury or pathogen invasion) whereby increased levels of GCs exert important adaptive actions in multiple tissues to restore homeostasis (Young et al., 2004; McEwen, 2007). Both ultradian/circadian and stress-evoked GC secretion is tightly controlled by various negative feedback mechanisms affecting each component of HPA axis, notably synthesis and release of corticotropin-releasing hormone (CRH) from the paraventricular nucleus (PVN) of the hypothalamus and adrenocorticotropic hormone (ACTH) from anterior pituitary. Any change in negative feedback loops will affect HPA axis, resulting in altered ultradian/circadian rhythm of GC release often with abnormally high basal GC levels, which in turn could lead to GC resistance.
Measurement of plasma cortisol in idiopathic PD patients has consistently shown significantly elevated levels compared to age-matched control subjects, and as well correlating with impulsive behaviors (Bellomo et al., 1991; Stypula et al., 1996; Hartmann et al., 1997; Charlett et al., 1998; Djamshidian et al., 2011; Ros-Bernal et al., 2011). The high cortisol levels seem unrelated to L-DOPA treatment or disease duration (Müller et al., 2007). Elevated cortisol levels are observed in many other neurodegenerative diseases including Alzheimer disease (Huang et al., 2009). In PD, however, the normally quiescent nocturnal cortisol secretory pattern is particularly affected (Hartmann et al., 1997) raising the question as to whether the circadian control of HPA axis by suprachiasmatic nucleus is altered. The underlying causes of HPA deregulation and whether or how it impacts PD pathology is presently not well understood. However, presence of LBs in both adrenal glands and hypothalamus in PD has been reported (Wakabayashi and Takahashi, 1997; Braak et al., 2006), which may imply a role of α-synuclein pathology in HPA axis deregulation. In addition to neuronal networks regulating HPA axis through feed back loops, cytokines liberated by peripheral immune cells can also stimulate HPA axis in several ways. Potent inflammatory cytokines (TNF-α, Il-1β and Il-6) can induce release of GCs by directly stimulating CRH synthesizing neurons of PVN or indirectly by stimulating production of prostaglandin E2 synthesis in perivascular cells (Ericsson et al., 1994; Kang et al., 2006; Serrats et al., 2010). In addition, IL-6 was shown to directly act on anterior pituitary cells as well as in adrenal glands, via its receptor, to stimulate the synthesis of ACTH and GC respectively (Zarković et al., 2008). Thus, deregulated immune responses with elevated levels of pro-inflammatory cytokins may lead to chronic activation of HPA axis.
Once secreted, GCs act on diverse physiological processes ranging from metabolism, immune responses to cognition and behavior. Their therapeutic potential, however, has limitations as chronic use with sustained high levels of GCs can result in serious side effects such as diabetes, obesity, dyslipidemia, hypertension, osteoporosis or behavioral anomalies. GCs clearly exert anti-inflammatory actions especially in an inflammatory setting, however, a number of recent studies indicate that they also exert pro-inflammatory responses, which are cell-type dependent. Thus, in response to acute stress resulting in increased GC levels, high levels of pro-inflammatory mediators such as IL-1β were found (Dhabhar, 2002; O’Connor et al., 2003; Sorrells et al., 2007). In a microarray study by Galon et al. (2002) on human mononuclear cells, dexamethasone treatment was found to induce the expression of several innate-immune related genes in addition to down-regulation of pro-inflammatory genes. It is believed that this opposing action of GCs “prepares” the immune system to respond rapidly to harmful stimulus and subsequently GCs act to down-regulate the immune response to restore homeostasis.
In brain, GC signaling is mediated by almost ubiquitously expressed GRs (GRs) as well as mineralocorticoid receptors (MR) that have restricted expression in neurons. However, it should be noted that MR is also expressed in glia (Sierra et al., 2008). GR, a prototype member of nuclear receptor superfamily (designated as NR3C1 in nomenclature of nuclear receptor family) is a ligand-activated transcription factor, it can also exert non-genomic actions (Groeneweg et al., 2011). GR is a modular protein with an N-terminal transactivation domain, a C-terminal ligand binding domain (LBD) and a central Zinc fingers-containing DNA-binding domain (DBD) that recognizes a specific DNA sequence. The LBD is the high affinity binding site for cortisol and other ligands. In humans, two major isoforms of GR, hGRα and hGRβ arising from alternative splicing have been described (Zhou and Cidlowski, 2005) and they differ in their C-terminal ligand-binding domain such that hGRβ cannot bind to endogenous or synthetic GCs. Experimental evidence indicates that hGRβ is expressed at low levels and it antagonizes the transcriptional activity of hGRα thus acting as dominant negative inhibitor of hGRα. However, recent genome-wide microarray studies indicate that hGRβ also regulates gene transcription (Kino et al., 2009). Interestingly, reduction in hGRα:hGRβ ratio has been associated with behavioral and mood disorders such as depression and schizophrenia (Perlman et al., 2004; Matsubara et al., 2006). In addition, alternative translational initiation sites generating 8 different GR proteins both in mouse and humans have been described (Oakley and Cidlowski, 2013).
Recent evidence shows that pulsatile pattern of GC secretion is crucial to proper GR transcriptional activity, thus loss of GC oscillatory pattern can result in continuous transcription with abnormal protein accumulation or GR targeting inappropriate genes leading to undesirable outcomes. GR is normally inert in the cytoplasm, in association with complex of proteins including heat-shock chaperones (HSP90, HSP70, HSP40, HSP23) and immunophilins such as FKBP51 (FK506 binding protein51), FKBP52, CP44 and PP5 (Grad and Picard, 2007). GC binding to this complex results in conformational change in GR exposing a nuclear localization signal resulting in importin-mediated translocation through the nuclear pore to the nucleoplasm.
In the nucleus, GR regulates transcription of its target genes in multiple, complex ways as well in highly cell- and context-specific manner. The transcriptional activity of GR has been especially studied with respect to its actions on metabolism and regulation of immune responses in the periphery. Multitude of studies indicates that GCs through GR influence each stage of inflammatory response i.e., from initiation, effector to resolution phases of an inflammatory reaction. Inflammatory response is triggered by specific receptors in immune cells and in this regard toll-like receptors (TLRs) activation and intracellular signaling cascade is thus far best characterized (Kawai and Akira, 2010) resulting in activation of transcriptional factors such as Nuclear factor kappa B (NF-κB), Activator Protein 1 (AP-1) or interferon response factors (IRFs). Each TLR family member (from 1–13 in mouse; all expressed in microglia) recognizes specific molecular signature present in either pathogens (PAMPs-Pathogen Associated Molecular Patterns) or molecules released by injured cells called DAMPs (Damage-associated Molecular Patterns). GR is reported to regulate key components of TLR signaling e.g., transforming growth factor beta-activated kinase 1 (TAK1; Bhattacharyya et al., 2010).
The GR regulation of inflammation is a result of both its transcriptional stimulatory and repressive activity. Classically, GR stimulates transcription of genes that act to inhibit inflammation and conversely it inhibits transcription of pro-inflammatory genes. GR stimulates transcription as homodimer binding to specific cognate DNA sequence, GAGAACAnnnTGTTCT, called Glucocorticoid Responsive Elements (GREs) present in the promoter regions of its target genes. This transcriptional activity of GR requires the presence of chromatin modifiers (e.g., Nuclear receptor coactivator NCoA1), basal transcriptional machinery and co-factors (CREB binding protein CBP, p300) (Rosenfeld and Glass, 2001). This mode of transcriptional activity has been notably described for genes coding for proteins of metabolic pathways such as glucose-6-phosphotase, fatty acid synthase or tyrosine aminotransferase as well as anti-inflammatory genes e.g., (Nuclear factor of kappa light polypeptide gene enhancer in B-cells inhibitor (IκB-α), MAPK phosphatase (MPK-1), IL-4, IL-10 or annexin-1 (De Bosscher et al., 2003). GR can also bind to negative GREs (nGREs) to repress transcription, thus among the genes identified containing nGREs are CRH as well as ACTH receptor in adrenal glands (Dostert and Heinzel, 2004; Surjit et al., 2011). Importantly, with regards to inflammation, GR can also inhibit transcription by tethering (i.e., through protein-protein interactions) or modulating the activity of other transcriptional factors, for example NF-κB, AP1 or IRF (Chinenov et al., 2013). This action mediated by GR monomers has been particularly studied in peripheral immune cells involving inhibition of expression of powerful pro-inflammatory genes as well as resolution of inflammation. The cross talk between AP1, NF-κB and GR is well documented (De Bosscher et al., 2003). As it has pertinence in the effects of GR observed in microglia it will be briefly reiterated here.
AP1 is comprised of heterodimers of c-Fos (C-Fos, FosB, Fra1 and 2), Jun (c-N, B-Jun, D-Jun) as well as ATF (Activating transcription factor) families of transcription factors, which controls expression of many cytokines. AP1 activity is stimulated by MAPK cascade resulting in activation of c-Jun-N terminal kinase (JNK), which phosphorylates c-Jun. GR regulates AP1 activity by associating with Jun-Fos complex at AP1 DNA elements in promoter regions of genes, inducing a conformational change in the complex that is not functional (Diamond et al., 1990). Additionally, GR also stimulates transcription of MAPK-phosphatase 1 (MKP-1) by binding to GRE elements present in its promoter region (as mentioned above) resulting in MKP-1 termination of JNK phosphorylation activity on c-Jun.
NF-κB signaling in positive immune regulation has been thoroughly characterized especially in the periphery (Karin and Greten, 2005). NF-κB comprises of RelA (p65), RelB, c-Rel, NF-κB1 (p50/p105 subunits) and NF-κB2 (p50/p100) proteins and the transcriptionally active dimers identified are: p65/p50 (classic NF-κB), p65/p65, p65/c-Rel, RelB/p50, RelB/p52 and, of note, Rel domains of these proteins bind to DNA. The p65/p50 NF-κB protein is normally sequestered in the cytoplasm by IκB family of proteins. NF-κB translocates to nucleus following phosphorylation of IκB by IKK kinases followed by rapid degradation of IκB. Importantly, phosphorylation of the p65 subunit is important for NF-κB activation. This involves phosphorylation of Serine 27Kuro6 of p65 by protein kinase A (PKA) catalytic subunit in complex with NF-κB and IκB as well as by nuclear localized MAPK-activated mitogen- and stress-activated protein kinase 1 (MSK1) in the nucleus. Interestingly GR was shown to decrease the nuclear pool of MSK1 thus down regulating NF-κB activity (Beck et al., 2008). With regards to its interaction with NF-κB, it was shown that upon GR activation by GC, GR is acetylated. In the nucleus, GR is deacetylated by histone deacetylase (HDAC2) (Ito et al., 2006) before it can physically bind to p65 subunit of NF-κB, functioning as transcriptional antagonist. Another manner by which GR can terminate NF-κB activation is by directly stimulating transcription of IκB-α as mentioned above.
In the CNS the role of endogenous GCs in regulating expression of pro-inflammatory cytokines such as IL-1β, TNF-α or IL-6 was shown originally following peripheral administration of LPS in adrenalectomized mice (Goujon et al., 1996). The finding that HPA axis is reactive to CNS inflammation triggered by an intrastriatal LPS injection was revealed through prior challenge with systemic LPS that resulted in rise in systemic corticosterone levels with concomitant and significant reductions in proinflammatory TNF-α, Monocyte chemoattractant protein MCP-1, IκBα transcripts in lesioned striatal/cortical region (Nadeau and Rivest, 2002). Interestingly in this paradigm, LPS does not trigger neuronal degeneration. However, neuronal death was observed by prior treatment with GR antagonist RU486 suggesting that GCs acting through GR prevent neuronal degeneration (Nadeau and Rivest, 2003). Recently, the role played by microglial GR in regulating neuronal survival in this intrastriatal model of LPS was shown conclusively in mice with selective inactivation of GR in microglia/macrophages, GRLysMCre mice (Carrillo-de Sauvage et al., 2013). Inflammation triggered by low dose of LPS (1–2 µg) injection has negligible effect on striatal or cortical neurons (Carrillo-de Sauvage et al., 2013), however the same dose of LPS injection in substantia nigra causes specific loss of dopamine neurons (Castaño et al., 2002) indicating a selective vulnerability of dopamine neurons to microglial inflammatory response mediated by LPS-activated TLR4. However, the fact that endogenous GCs activating GRs in microglia are neuroprotective during LPS-induced inflammation in cortex/striatum but not in midbrain substantia nigra implies that their actions in microglia during TLR4 activation may be region-specific. In this regard, recently the concept of microglial heterogeneity with respect to their functional capabilities, for example LPS/TLR4 signaling, has been evoked (Noh et al., 2014).
Nigral dopamine neurodegeneration triggered by MPTP is significantly reduced by pharmacological treatments with GC agonists e.g., corticosterone that artificially increase GCs above endogenous levels, conversely adrenalectomy augments dopamine neuronal loss (Kurkowska-Jastrzebska et al., 2004; Sugama et al., 2009; Ros-Bernal et al., 2011) indicating that high levels of GCs present during MPTP intoxication protect dopamine neurons. Immuno-labeling of GR revealed its localization mainly in the nucleus of microglia and its quantification was carried out in substantia nigra and striatum in saline and MPTP injected mice. The results showed that number of microglia with nuclear GR augmented from 35% in resting state to 70–80% 3 days after MPTP injections, which then declined to almost normal levels after 3 weeks. Measurement of endogenous corticosterone levels showed a three-fold rise 1 day after MPTP (Ros-Bernal et al., 2011). Importantly, these results indicate that GR activation during endogenous rise in corticosterone levels is progressive concurring loss of dopamine neurons (Figure 1). However increasing GC levels by corticosterone treatment results in significant neuroprotection likely because GR activation in microglia is rapid enough to counteract the inflammatory response mounted by activated glia.
Figure 1. Transcriptional regulation of inflammation by GR in microglia. (A) In resting, healthy state without any cellular injury or pathogen invasion, GR is inactive in most microglia. Toxins e.g., MPTP or powerful inflammogen LPS would rapidly trigger innate immune response in microglia. In the case of LPS, TLR4 present in microglia results in activation of major transcription factors NF-κB, IRF and AP-1, known to orchestrate an inflammatory response. Cellular injury or pro-inflammatory cytokines would activate HPA axis and increased GC secretion. High circulating GC levels results in GR activation in microglia, which act to repress the transcriptional activity of NF-κB, IRF and AP-1 and also stimulating the expression of genes such as IκB-α and or MKP-1 known to inhibit NF-κB and AP-1 respectively. Neuronal injury and death are prevented. (B) In the absence of GR activity in microglia, microglia remain in activated state causing neuronal death.
The precise actions of GR in microglia during dopamine neurodegeneration were studied using GRLysMCre mice (Ros-Bernal et al., 2011). Functionally, absence of GR in microglia/macrophages resulted in significant dopamine neuronal loss in two paradigms of MPTP intoxication: (a) acute toxicity (4 injections/day) which is accompanied by intense microglial and astroglial activation of short duration; and (b) sub-chronic treatment (1 injection for 5 days) the loss of dopamine neurons is less, and morphologically, microglial activation is less apparent. In microglial GR mutant mice, both MPTP paradigms augmented microglial activation i.e., number hypertrophied microglia, compared to controls. Additionally, in sub-chronic paradigm, GR was found to prolong the duration of activation. Several molecules released by degenerating dopamine neurons can potentially trigger morphological and functional changes in microglia i.e., its activation status or its mobility (e.g., Matrix metalloprotease MMP-9, α-synuclein, Annese et al., 2015), however how the primary signals emitted from degenerating dopamine neurons trigger microglial activation is not well elucidated. With regards to regulation of inflammation, microglial GR was found to modulate 3 classes of inflammatory genes: (a) increasing expression of pro-inflammatory molecules in particular, TNF-α, iNOS, Intercellular adhesion molecule (ICAM); (b) anti-inflammatory genes e.g., MKP-1 (as described above for inhibiting AP1 transcriptional activity) and IL-1R2 which is a decoy receptor for IL-1 receptor1; (c) inflammatory caspases, i.e., caspases 1 and 4 as well as TLR3, TLR4, TLR9 and MyD88. The inflammatory caspases and TLRs are core components of innate immunity important for stimulating the transcriptional activity of AP1, NF-κB and IRF and thereby expression of plethora of inflammatory mediators. These findings indicate that GR not only inhibits the molecules like TNF-α known to execute the inflammatory reaction but also prevent excessive expression of upstream activators that initiate an inflammatory reaction.
Nuclear expression of p65 subunit of NF-κB, indicative of transcriptional activity NF-κB, was observed in microglia of SNpc in PD patients as well as in mice treated with MPTP. Moreover inhibiting NF-κB in mice significantly protected dopamine neurons against MPTP toxicity (Ghosh et al., 2007). Thus sustained transcriptional activity of NF-κB is likely involved in chronic activation of microglia in PD. Interestingly, GR was found to associate with p65 subunit of NF-κB in microglial cultures, as well in luciferase reporter assays GR inhibited its transcriptional activity (Ros-Bernal et al., 2011; Carrillo-de Sauvage et al., 2013). In vivo, Serine 276 phosphorylation of P65 subunit of NF-κB, indicative of its activation, was sustained in MPTP-lesioned SNpc and striatum of mutant GR microglial mice (Ros-Bernal et al., 2011).
The halting of inflammation is central to immune response. A failure to limit the amplitude and duration of this process as well as initiate a resolution phase can lead to chronic inflammatory state. In addition to GR, other members of nuclear receptor family e.g., Peroxisome proliferator activated receptor gamma (PPAR-γ), Liver X receptor (LXR), Estrogen receptor ER-β, Nuclear receptor NR4A family (Nurr 77, Nurr1) are expressed in microglia, thus they can also control microglial activation. In this regard, Nurr1 inhibition was found to increase NF-κB activity in glia resulting in exaggerated expression of pro-inflammatory mediators and increased loss of dopamine neurons following LPS injection in substantia nigra (Saijo et al., 2009).
Regarding GCs, it is possible that in PD, GR functions in immune cells are compromised because of chronically elevated levels of cortisol. The putative scenario of dysfunction of GR signaling in PD is illustrated in Figure 2. Different stressors such as aging, infections, environmental and genetic susceptibility factors would activate HPA axis resulting in augmentation of circulating GC levels and activation of GR. In parallel, activation of peripheral immune system would result in increased circulating levels of pro-inflammatory molecules e.g., Il-1β known to activate HPA axis and also to induce microglial priming such that any subsequent insult exacerbates microglial inflammatory phenotype. Persistent activation of HPA axis with chronically high cortisol levels would compromise GR functions (Dejager et al., 2014). Further studies are needed to understand how GR activity is affected in microglia during chronically active HPA axis, as is the case for PD patients and whether GR inflammatory function is affected in PD. In addition, it would be important to understand the redundant and non-redundant functions of GR with closely related nuclear receptor members such as Nurr1 for envisaging therapeutic potentials in PD.
Figure 2. Putative roles of glucocorticoids (GC) and glucocorticoid receptor (GR) in progression to chronic inflammation and dopamine neurodegeneration. In healthy state, microglia and astroglia surrounding are quiescent. Aging as well as other stressors such as infections or PD-related genetic and environmental factors would put immune system on alert and possibly also stimulating HPA axis. Activation of HPA axis results in increase in GC levels and activation of GR. In pre-clinical stage, secretion of DAMPS, such as pathological form of α-synuclein would activate immune system as well as HPA axis. Persistent activation of HPA axis results in loss of its regulation and chronically high GC levels. Chronic GCs are known to result in GR dysfunction in immune cells. Microglia and astroglia remain activated creating a pro-inflammatory environment and augmenting oxidative stress. Disruption in blood brain barrier resulting in T cell infiltration further promotes glial activation. Dopamine degeneration is progressively increased leading to clinical manifestation of PD.
The authors declare that the research was conducted in the absence of any commercial or financial relationships that could be construed as a potential conflict of interest.
MTH was supported by grants from the Spanish Ministry of Science and Innovation (FIS PI13 01293), Fundación Séneca (FS/14902/PI/10), University Jaume I (13I004.01/1), IMIB (Institute for Bio-Health Research of Murcia) and CIBERNED (Centro de Investigación Biomédica en Red sobre Enfermedades Neurodegenerativas). SV acknowledges the financial support from Association France Parkinson, Fondation de France, ANR-13-BSV1-0013-02, CNRS, INSERM and UPMC. SV is a member of LabEX Biological Psychiatry.
ACTH, Adrenocorticotropic hormone; AP1, Activator protein 1; ATF, Activating transcription factor; BBB, Blood brain barrier; BST-1, Bone marrow stromal cell antigen; C1Q, Complement component 1q; C3, Complement component 3; CBP, CREB Binding protein; CCL5, Chemokine ligand 5; CD14, cluster of differentiation 14; CD200, Cluster of Differentiation 200; CD47/CD172A, Cluster of Differentiation 47; CLRN3, Clarin3; CNS, Central nervous system; CRH, Corticotropin-releasing hormone; CX3CL1, Chemokine (C-X3-C motif) ligand 1; CX3CR1, CX3C chemokine receptor 1; CYP2D6, Cytochrome P450 2D6; DAMP, Damage-associated molecular pattern; DBD, DNA-binding domain; ER-β, Estrogen Receptor β; FKBP51, FK506 binding protein; GC, Glucocorticoids; GPNMB, Glycoprotein NMB gene; GR, Glucocorticoid receptor; GRE, Glucocorticoid response element; H1N1, Hemagglutinin neuraminidase; HDAC2, Histone deacetylase 2; HLA, Human leucocyte antigen; HPA, Hypothalamic-pituitary-adrenal; HSP90, Heat shock protein 90; ICAM, Intercellular adhesion molecule; IFN-γ, Interferon γ; IκBa, Nuclear factor of κ light polypeptide gene enhancer in B-cells inhibitor; IKK, IκB kinase; IL-1β, Interleukin-1β; IL-6, Interleukin-6; iNOS, Inducible nitric oxide synthase; IRF, Interferon response factor; JNK, c-Jun N-terminal kinase; LB, Lewy bodies; LBD, Ligand-binding domain; LPS, Lipopolysaccharide; LRRK2, Leucine rich repeat kinase 2; LXR, Liver X receptor; MAPK, Mitogen-activated protein kinase; MCP1, Monocyte chemoattractant protein-1; MECP2, Methyl CpG binding protein 2; MHC, Major histocompatibility complex; MKP1, MAPK phosphatase 1; MMP9, Matrix metalloprotease 9; MPTP, 1-methyl, 4-phenyl, 1, 2, 3, 6-tetrahydropyridine; MR, Mineralocorticoid receptor; MSK1, Mitogen- and stress-activated protein kinase-1; MYD88, Myeloid differentiation primary response gene 88; NCOA1, Nuclear receptor coactivator 1; NF-κβ, Nuclear factor κ-light-chain-enhancer of activated β cells; NR3C1, Nuclear Receptor Subfamily 3, Group C, Member 1; NR4A, Nuclear receptor 4A; NSAID, Nonsteroidal anti-inflammatory drugs; P300, E1A binding protein p300; PAMP, Pathogen-associated molecular patterns; PD, Parkinson’s disease; PET, Positron emission tomography; PINK1, PTEN-induced putative kinase 1; PKA, Protein kinase A; PLA2G6, Phospholipase A2, group VI; PPAR-γ, Peroxisome proliferator-activated receptor γ; PVN, Paraventricular nucleus of hypothalamus; RANTES, Regulated on activation, normal T cell expressed and secreted- CCL5; REM, Rapid eye movement (REM) sleep; SN, Substantia nigra; SNCA, Synuclein, α; SNP, Single Nucleotide Polymorphism; SPG4/SPAST, Spastic paraplegia-4/spastin; TAK1, Transforming growth factor activated kinase-1; TLR, Toll-like receptor; TNF-α, Tumor necrosis factor-α.
Abdullah, R., Basak, I., Patil, K. S., Alves, G., Larsen, J. P., and Møll, S. G. (2014). Parkinson’s disease and age: the obvious but largely unexplored link. Exp. Gerontol. doi: 10.1016/j.exger.2014.09.014. [Epub ahead of print].
PubMed Abstract | Full Text | CrossRef Full Text | Google Scholar
Ahmed, I., Tamouza, R., Delord, M., Krishnamoorthy, R., Tzourio, C., Mulot, C., et al. (2012). Association between Parkinson’s disease and the HLA-DRB1 locus. Mov. Disord. 27, 1104–1110. doi: 10.1002/mds.25035
PubMed Abstract | Full Text | CrossRef Full Text | Google Scholar
Annese, V., Herrero, M. T., Di Pentima, M., Gomez, A., Lombardi, L., Ros, C. M., et al. (2015). Metalloproteinase-9 contributes to inflammatory glia activation and nigro-striatal pathway degeneration in both mouse and monkey models of 1-methyl-4-phenyl-1,2,3,6-tetrahydropyridine (MPTP)-induced Parkinsonism. Brain Struct Funct. 220, 703–727. doi: 10.1007/s00429-014-0718-8
PubMed Abstract | Full Text | CrossRef Full Text | Google Scholar
Barcia, C., de Pablos, V., Bautista-Hernández, V., Sánchez-Bahillo, A., Bernal, I., Fernández-Villalba, E., et al. (2005). Increased plasma levels of TNF-alpha but not of IL1-beta in MPTP-treated monkeys one year after the MPTP administration. Parkinsonism Relat. Disord. 11, 435–439. doi: 10.1016/j.parkreldis.2005.05.006
PubMed Abstract | Full Text | CrossRef Full Text | Google Scholar
Barcia, C., Ros, C. M., Annese, V., Carrillo-de Sauvage, M. A., Ros-Bernal, F., Gómez, A., et al. (2012). ROCK/Cdc42-mediated microglial motility and gliapse formation lead to phagocytosis of degenerating dopaminergic neurons in vivo. Sci. Rep. 2:809. doi: 10.1038/srep00809
PubMed Abstract | Full Text | CrossRef Full Text | Google Scholar
Barcia, C., Ros, C. M., Annese, V., Gómez, A., Ros-Bernal, F., Aguado-Yera, D., et al. (2011). IFN-γ signaling, with the synergistic contribution of TNF-α, mediates cell specific microglial and astroglial activation in experimental models of Parkinson’s disease. Cell Death Dis. 2:e142. doi: 10.1038/cddis.2011.17
PubMed Abstract | Full Text | CrossRef Full Text | Google Scholar
Barcia, C., Sánchez Bahillo, A., Fernández-Villalba, E., Bautista, V., Poza Y Poza, M., Fernández-Barreiro, A., et al. (2004). Evidence of active microglia in substantia nigra pars compacta of parkinsonian monkeys 1 year after MPTP exposure. Glia 46, 402–409. doi: 10.1002/glia.20015
PubMed Abstract | Full Text | CrossRef Full Text | Google Scholar
Bardou, I., Kaercher, R. M., Brothers, H. M., Hopp, S. C., Royer, S., and Wenk, G. L. (2014). Age and duration of inflammatory environment differentially affect the neuroimmune response and catecholaminergic neurons in the midbrain and brainstem. Neurobiol. Aging 35, 1065–1073. doi: 10.1016/j.neurobiolaging.2013.11.006
PubMed Abstract | Full Text | CrossRef Full Text | Google Scholar
Beck, J. A., Poulter, M., Campbell, T. A., Uphill, J. B., Adamson, G., Geddes, J. F., et al. (2004). Somatic and germline mosaicism in sporadic early-onset alzheimer’s disease. Hum. Mol. Genet. 13, 1219–1224. doi: 10.1093/hmg/ddh134
PubMed Abstract | Full Text | CrossRef Full Text | Google Scholar
Beck, I. M., Vanden Berghe, W., Vermeulen, L., Bougarne, N., Vander Cruyssen, B., Haegeman, G., et al. (2008). Altered subcellular distribution of MSK1 induced by glucocorticoids contributes to NF-kappaB inhibition. EMBO J. 27, 1682–1693. doi: 10.1038/emboj.2008.95
PubMed Abstract | Full Text | CrossRef Full Text | Google Scholar
Bellomo, G., Santambrogio, L., Fiacconi, M., Scarponi, A. M., and Ciuffetti, G. (1991). Plasma profiles of adrenocorticotropic hormone, cortisol, growth hormone and prolactin in patients with untreated Parkinson’s disease. J. Neurol. 238, 19–22. doi: 10.1007/bf00319704
PubMed Abstract | Full Text | CrossRef Full Text | Google Scholar
Berg, D., Postuma, R. B., Bloem, B., Chan, P., Dubois, B., Gasser, T., et al. (2014). Time to redefine PD? Introductory statement of the MDS task force on the definition of Parkinson’s disease. Mov. Disord. 29, 454–462. doi: 10.1002/mds.25844
PubMed Abstract | Full Text | CrossRef Full Text | Google Scholar
Bhattacharyya, S., Ratajczak, C. K., Vogt, S. K., Kelley, C., Colonna, M., Schreiber, R. D., et al. (2010). TAK1 targeting by glucocorticoids determines JNK and IkappaB regulation in toll-like receptor-stimulated macrophages. Blood 115, 1921–1931. doi: 10.1182/blood-2009-06-224782
PubMed Abstract | Full Text | CrossRef Full Text | Google Scholar
Block, M. L., Zecca, L., and Hong, J. S. (2007). Microglia-mediated neurotoxicity: uncovering the molecular mechanisms. Nat. Rev. Neurosci. 8, 57–69. doi: 10.1038/nrn2038
PubMed Abstract | Full Text | CrossRef Full Text | Google Scholar
Braak, H., Müller, C. M., Rüb, U., Ackermann, H., Bratzke, H., de Vos, R. A., et al. (2006). Pathology associated with sporadic Parkinson’s disease–where does it end? J. Neural Transm. Suppl. 70, 89–97. doi: 10.1007/978-3-211-45295-0_15
PubMed Abstract | Full Text | CrossRef Full Text | Google Scholar
Braak, H., Rüb, U., Gai, W. P., and Del Tredici, K. (2003). Idiopathic Parkinson’s disease: possible routes by which vulnerable neuronal types may be subject to neuroinvasion by an unknown pathogen. J. Neural Transm. 110, 517–536. doi: 10.1007/s00702-002-0808-2
PubMed Abstract | Full Text | CrossRef Full Text | Google Scholar
Buchman, A. S., Shulman, J. M., Nag, S., Leurgans, S. E., Arnold, S. E., Morris, M. C., et al. (2012). Nigral pathology and parkinsonian signs in elders without Parkinson disease. Ann. Neurol. 71, 258–266. doi: 10.1002/ana.22588
PubMed Abstract | Full Text | CrossRef Full Text | Google Scholar
Cabezas, R., Avila, M., Gonzalez, J., El-Bachá, R. S., Báez, E., García-Segura, L. M., et al. (2014). Astrocytic modulation of blood brain barrier: perspectives on Parkinson’s disease. Front. Cell. Neurosci. 4:211. doi: 10.3389/fncel.2014.00211
PubMed Abstract | Full Text | CrossRef Full Text | Google Scholar
Cappellano, G., Carecchio, M., Fleetwood, T., Magistrelli, L., Cantello, R., Dianzani, U., et al. (2013). Immunity and inflammation in neurodegenerative diseases. Am. J. Neurodegener. Dis. 2, 89–107.
Carrillo-de Sauvage, M. A., Maatouk, L., Arnoux, I., Pasco, M., Sanz Diez, A., Delahaye, M., et al. (2013). Potent and multiple regulatory actions of microglial glucocorticoid receptors during CNS inflammation. Cell Death Differ. 20, 1546–1557. doi: 10.1038/cdd.2013.108
PubMed Abstract | Full Text | CrossRef Full Text | Google Scholar
Castaño, A., Herrera, A. J., Cano, J., and Machado, A. (2002). The degenerative effect of a single intranigral injection of LPS on the dopaminergic system is prevented by dexamethasone and not mimicked by rh-TNF-alpha, IL-1beta and IFN-gamma. J. Neurochem. 81, 150–157. doi: 10.1046/j.1471-4159.2002.00799.x
PubMed Abstract | Full Text | CrossRef Full Text | Google Scholar
Chao, Y., Wong, S. C., and Tan, E. K. (2014). Evidence of inflammatory system involvement in Parkinson’s disease. Biomed. Res. Int. 2014:308654. doi: 10.1155/2014/308654
PubMed Abstract | Full Text | CrossRef Full Text | Google Scholar
Charlett, A., Dobbs, R. J., Purkiss, A. G., Wright, D. J., Peterson, D. W., Weller, C., et al. (1998). Cortisol is higher in parkinsonism and associated with gait deficit. Acta Neurol. Scand. 97, 77–85. doi: 10.1111/j.1600-0404.1998.tb00614.x
PubMed Abstract | Full Text | CrossRef Full Text | Google Scholar
Chinenov, Y., Gupte, R., and Rogatsky, I. (2013). Nuclear receptors in inflammation control: repression by GR and beyond. Mol. Cell. Endocrinol. 380, 55–64. doi: 10.1016/j.mce.2013.04.006
PubMed Abstract | Full Text | CrossRef Full Text | Google Scholar
Chung, S. J., Armasu, S. M., Biernacka, J. M., Anderson, K. J., Lesnick, T. G., Rider, D. N., et al. (2012). Genomic determinants of motor and cognitive outcomes in Parkinson’s disease. Parkinsonism Relat. Disord. 18, 881–886. doi: 10.1016/j.parkreldis.2012.04.025
PubMed Abstract | Full Text | CrossRef Full Text | Google Scholar
Clarimón, J., and Kulisevsky, J. (2013). Parkinson’s disease: from genetics to clinical practice. Curr. Genomics 14, 560–567. doi: 10.2174/1389202914666131210212305
PubMed Abstract | Full Text | CrossRef Full Text | Google Scholar
Collier, T. J., Kanaan, N. M., and Kordower, J. H. (2011). Ageing as a primary risk factor for Parkinson’s disease: evidence from studies of non-human primates. Nat. Rev. Neurosci. 12, 359–366. doi: 10.1038/nrn3039
PubMed Abstract | Full Text | CrossRef Full Text | Google Scholar
De Bosscher, K., Vanden Berghe, W., and Haegeman, G. (2003). The interplay between the glucocorticoid receptor and nuclear factor-kappaB or activator protein-1: molecular mechanisms for gene repression. Endocr. Rev. 24, 488–522. doi: 10.1210/er.2002-0006
PubMed Abstract | Full Text | CrossRef Full Text | Google Scholar
Dejager, L., Vandevyver, S., Petta, I., and Libert, C. (2014). Dominance of the strongest: inflammatory cytokines versus glucocorticoids. Cytokine Growth Factor Rev. 25, 21–33. doi: 10.1016/j.cytogfr.2013.12.006
PubMed Abstract | Full Text | CrossRef Full Text | Google Scholar
Depienne, C., Fedirko, E., Faucheux, J. M., Forlani, S., Bricka, B., Goizet, C., et al. (2007). A de novo SPAST mutation leading to somatic mosaicism is associated with a later age at onset in HSP. Neurogenetics 8, 231–233. doi: 10.1007/s10048-007-0090-4
PubMed Abstract | Full Text | CrossRef Full Text | Google Scholar
Devos, D., Lebouvier, T., Lardeux, B., Biraud, M., Rouaud, T., Pouclet, H., et al. (2013). Colonic inflammation in Parkinson’s disease. Neurobiol. Dis. 50, 42–48. doi: 10.1016/j.nbd.2012.09.007
PubMed Abstract | Full Text | CrossRef Full Text | Google Scholar
Dhabhar, F. S. (2002). Stress-induced augmentation of immune function-the role of stress hormones, leukocyte trafficking and cytokines. Brain Behav. Immun. 16, 785–798. doi: 10.1016/s0889-1591(02)00036-3
PubMed Abstract | Full Text | CrossRef Full Text | Google Scholar
Diamond, M. I., Miner, J. N., Yoshinaga, S. K., and Yamamoto, K. R. (1990). Transcription factor interactions: selectors of positive or negative regulation from a single DNA element. Science 249, 1266–1272. doi: 10.1126/science.2119054
PubMed Abstract | Full Text | CrossRef Full Text | Google Scholar
Djamshidian, A., O’Sullivan, S. S., Papadopoulos, A., Bassett, P., Shaw, K., Averbeck, B. B., et al. (2011). Salivary cortisol levels in Parkinson’s disease and its correlation to risk behaviour. J. Neurol. Neurosurg Psychiatry 82, 1107–1111. doi: 10.1136/jnnp.2011.245746
PubMed Abstract | Full Text | CrossRef Full Text | Google Scholar
Dobbs, R. J., Charlett, A., Purkiss, A. G., Dobbs, S. M., Weller, C., and Peterson, D. W. (1999). Association of circulating TNF-alpha and IL-6 with ageing and parkinsonism. Acta Neurol. Scand. 100, 34–41. doi: 10.1111/j.1600-0404.1999.tb00721.x
PubMed Abstract | Full Text | CrossRef Full Text | Google Scholar
Dostert, A., and Heinzel, T. (2004). Negative glucocorticoid receptor response elements and their role in glucocorticoid action. Curr. Pharm. Des. 10, 2807–2816. doi: 10.2174/1381612043383601
PubMed Abstract | Full Text | CrossRef Full Text | Google Scholar
Dzamko, N., Geczy, C. L., and Halliday, G. M. (2014). Inflammation is genetically implicated in Parkinson’s disease. Neuroscience doi: 10.1016/j.neuroscience.2014.10.028. [Epub ahead of print].
PubMed Abstract | Full Text | CrossRef Full Text | Google Scholar
Elbaz, A., Levecque, C., Clavel, J., Vidal, J. S., Richard, F., Amouyel, P., et al. (2004). CYP2D6 polymorphism, pesticide exposure and Parkinson’s disease. Ann. Neurol. 55, 430–434. doi: 10.1002/ana.20051
PubMed Abstract | Full Text | CrossRef Full Text | Google Scholar
Ericsson, A., Kovács, K. J., and Sawchenko, P. E. (1994). A functional anatomical analysis of central pathways subserving the effects of interleukin-1 on stress-related neuroendocrine neurons. J. Neurosci. 14, 897–913.
Forsyth, C. B., Shannon, K. M., Kordower, J. H., Voigt, R. M., Shaikh, M., Jaglin, J. A., et al. (2011). Increased intestinal permeability correlateswith sigmoidmucosa alpha-synuclein staining and endotoxin exposuremarkers in early Parkinson’s disease. PLoS One 6:e28032. doi: 10.1371/journal.pone.0028032
PubMed Abstract | Full Text | CrossRef Full Text | Google Scholar
Galon, J., Franchimont, D., Hiroi, N., Frey, G., Boettner, A., Ehrhart-Bornstein, M., et al. (2002). Gene profiling reveals unknown enhancing and suppressive actions of glucocorticoids on immune cells. FASEB J. 16, 61–71. doi: 10.1096/fj.01-0245com
PubMed Abstract | Full Text | CrossRef Full Text | Google Scholar
Ghosh, A., Roy, A., Liu, X., Kordower, J. H., Mufson, E. J., Hartley, D. M., et al. (2007). Selective inhibition of NF-kappaB activation prevents dopaminergic neuronal loss in a mouse model of Parkinson’s disease. Proc. Natl. Acad. Sci. U S A 104, 18754–18759. doi: 10.1073/pnas.0704908104
PubMed Abstract | Full Text | CrossRef Full Text | Google Scholar
Gillardon, F., Schmid, R., and Draheim, H. (2012). Parkinson’s disease-linked leucine-rich repeat kinase 2(R1441G) mutation increases proinflammatory cytokine release from activated primary microglial cells and resultant neurotoxicity. Neuroscience 208, 41–48. doi: 10.1016/j.neuroscience.2012.02.001
PubMed Abstract | Full Text | CrossRef Full Text | Google Scholar
Goedert, M., Spillantini, M. G., Del Tredici, K., and Braak, H. (2013). 100 years of Lewy pathology. Nat. Rev. Neurol. 9, 13–24. doi: 10.1038/nrneurol.2012.242
PubMed Abstract | Full Text | CrossRef Full Text | Google Scholar
Goldman, J. G., and Postuma, R. (2014). Premotor and nonmotor features of Parkinson’s disease. Curr. Opin. Neurol. 27, 434–441. doi: 10.1097/WCO.0000000000000112
PubMed Abstract | Full Text | CrossRef Full Text | Google Scholar
Goujon, E., Parnet, P., Layé, S., Combe, C., and Dantzer, R. (1996). Adrenalectomy enhances pro-inflammatory cytokines gene expression, in the spleen, pituitary and brain of mice in response to lipopolysaccharide. Brain Res. Mol. Brain Res. 36, 53–62. doi: 10.1016/0169-328x(95)00242-k
PubMed Abstract | Full Text | CrossRef Full Text | Google Scholar
Grad, I., and Picard, D. (2007). The glucocorticoid responses are shaped by molecular chaperones. Mol. Cell. Endocrinol. 275, 2–12. doi: 10.1016/j.mce.2007.05.018
PubMed Abstract | Full Text | CrossRef Full Text | Google Scholar
Groeneweg, F. L., Karst, H., de Kloet, E. R., and Joëls, M. (2011). Rapid non-genomic effects of corticosteroids and their role in the central stress response. J. Endocrinol. 209, 153–167. doi: 10.1530/JOE-10-0472
PubMed Abstract | Full Text | CrossRef Full Text | Google Scholar
Halliday, G. M., and Stevens, C. H. (2011). Glia: initiators and progressors of pathology in Parkinson’s disease. Mov. Disord. 26, 6–17. doi: 10.1002/mds.23455
PubMed Abstract | Full Text | CrossRef Full Text | Google Scholar
Harms, A. S., Cao, S., Rowse, A. L., Thome, A. D., Li, X., Mangieri, L. R., et al. (2013). MHCII is required for α-synuclein-induced activation of microglia, CD4 T cell proliferation and dopaminergic neurodegeneration. J. Neurosci. 33, 9592–9600. doi: 10.1523/JNEUROSCI.5610-12.2013
PubMed Abstract | Full Text | CrossRef Full Text | Google Scholar
Hartmann, A., Veldhuis, J. D., Deuschle, M., Standhardt, H., and Heuser, I. (1997). Twenty-four hour cortisol release profiles in patients with Alzheimer’s and Parkinson’s disease compared to normal controls: ultradian secretory pulsatility and diurnal variation. Neurobiol. Aging 18, 285–289. doi: 10.1016/s0197-4580(97)80309-0
PubMed Abstract | Full Text | CrossRef Full Text | Google Scholar
Hawkes, C. H., Del Tredici, K., and Braak, H. (2009). Parkinson’s disease: the dual hit theory revisited. Ann. N Y Acad. Sci. 1170, 615–622. doi: 10.1111/j.1749-6632.2009.04365.x
PubMed Abstract | Full Text | CrossRef Full Text | Google Scholar
Hellman, L., Nakada, F., Curti, J., Weitzman, E. D., Kream, J., Roffwarg, H., et al. (1970). Cortisol is secreted episodically by normal man. J. Clin. Endocrinol. Metab. 30, 411–422. doi: 10.1210/jcem-30-4-411
PubMed Abstract | Full Text | CrossRef Full Text | Google Scholar
Hench, P. S., Kendall, E. C., Slocumb, C. H., and Polley, H. F. (1950). Effects of cortisone acetate and pituitary ACTH on rheumatoid arthritis, rheumatic fever and certain other conditions. Arch. Intern. Med. (Chic). 85, 545–666. doi: 10.1001/archinte.1950.00230100002001
PubMed Abstract | Full Text | CrossRef Full Text | Google Scholar
Hill-Burns, E. M., Factor, S. A., Zabetian, C. P., Thomson, G., and Payami, H. (2011). Evidence for more than one Parkinson’s disease-associated variant within the HLA region. PLoS One 6:e27109. doi: 10.1371/journal.pone.0027109
PubMed Abstract | Full Text | CrossRef Full Text | Google Scholar
Huang, C. W., Lui, C. C., Chang, W. N., Lu, C. H., Wang, Y. L., and Chang, C. C. (2009). Elevated basal cortisol level predicts lower hippocampal volume and cognitive decline in Alzheimer’s disease. J. Clin. Neurosci. 16, 1283–1286. doi: 10.1016/j.jocn.2008.12.026
PubMed Abstract | Full Text | CrossRef Full Text | Google Scholar
Hunot, S., Boissière, F., Faucheux, B., Brugg, B., Mouatt-Prigent, A., Agid, Y., et al. (1996). Nitric oxide synthase and neuronal vulnerability in Parkinson’s disease. Neuroscience 72, 355–363. doi: 10.1016/0306-4522(95)00578-1
PubMed Abstract | Full Text | CrossRef Full Text | Google Scholar
Iannaccone, S., Cerami, C., Alessio, M., Garibotto, V., Panzacchi, A., Olivieri, S., et al. (2013). In vivo microglia activation in very early dementia with Lewy bodies, comparison with Parkinson’s disease. Parkinsonism Relat. Disord. 19, 47–52. doi: 10.1016/j.parkreldis.2012.07.002
PubMed Abstract | Full Text | CrossRef Full Text | Google Scholar
Ito, K., Yamamura, S., Essilfie-Quaye, S., Cosio, B., Ito, M., Barnes, P. J., et al. (2006). Histone deacetylase 2-mediated deacetylation of the glucocorticoid receptor enables NF-kappaB suppression. J. Exp. Med. 203, 7–13. doi: 10.1084/jem.20050466
PubMed Abstract | Full Text | CrossRef Full Text | Google Scholar
Jackson-Lewis, V., and Smeyne, R. J. (2005). MPTP and SNpc DA neuronal vulnerability: role of dopamine, superoxide and nitric oxide in neurotoxicity. Minireview. Neurotox. Res. 7, 193–201. doi: 10.1007/bf03036449
PubMed Abstract | Full Text | CrossRef Full Text | Google Scholar
Jang, H., Boltz, D., Sturm-Ramirez, K., Shepherd, K. R., Jiao, Y., Webster, R., et al. (2009). Highly pathogenic H5N1 influenza virus can enter the central nervous system and induce neuroinflammation and neurodegeneration. Proc. Natl. Acad. Sci. U S A 106, 14063–14068. doi: 10.1073/pnas.0900096106
PubMed Abstract | Full Text | CrossRef Full Text | Google Scholar
Kanaan, N. M., Kordower, J. H., and Collier, T. J. (2008). Age and region-specific responses of microglia, but not astrocytes, suggest a role in selective vulnerability of dopamine neurons after 1-methyl-4-phenyl-1,2,3,6-tetrahydropyridine exposure in monkeys. Glia 56, 1199–1214. doi: 10.1002/glia.20690
PubMed Abstract | Full Text | CrossRef Full Text | Google Scholar
Kanaan, N. M., Kordower, J. H., and Collier, T. J. (2010). Age-related changes in glial cells of dopamine midbrain subregions in rhesus monkeys. Neurobiol. Aging 31, 937–952. doi: 10.1016/j.neurobiolaging.2008.07.006
PubMed Abstract | Full Text | CrossRef Full Text | Google Scholar
Kang, Y. M., Zhang, Z. H., Johnson, R. F., Yu, Y., Beltz, T., Johnson, A. K., et al. (2006). Novel effect of mineralocorticoid receptor antagonism to reduce proinflammatory cytokines and hypothalamic activation in rats with ischemia-induced heart failure. Circ. Res. 99, 758–766. doi: 10.1161/01.res.0000244092.95152.86
PubMed Abstract | Full Text | CrossRef Full Text | Google Scholar
Karin, M., and Greten, F. R. (2005). NF-kappaB: linking inflammation and immunity to cancer development and progression. Nat. Rev. Immunol. 5, 749–759. doi: 10.1038/nri1703
PubMed Abstract | Full Text | CrossRef Full Text | Google Scholar
Kawai, T., and Akira, S. (2010). The role of pattern-recognition receptors in innate immunity: update on toll-like receptors. Nat. Immunol. 11, 373–384. doi: 10.1038/ni.1863
PubMed Abstract | Full Text | CrossRef Full Text | Google Scholar
Kieburtz, K., and Wunderle, K. B. (2013). Parkinson’s disease: evidence for environmental risk factors. Mov. Disord. 28, 8–13. doi: 10.1002/mds.25150
PubMed Abstract | Full Text | CrossRef Full Text | Google Scholar
Kim, H. J., and Jeon, B. S. (2014). Hypothesis: somatic mosaicism and parkinson disease. Exp. Neurobiol. 23, 271–276. doi: 10.5607/en.2014.23.4.271
PubMed Abstract | Full Text | CrossRef Full Text | Google Scholar
Kino, T., Manoli, I., Kelkar, S., Wang, Y., Su, Y. A., and Chrousos, G. P. (2009). Glucocorticoid receptor (GR) beta has intrinsic, GRalpha-independent transcriptional activity. Biochem. Biophys. Res. Commun. 381, 671–675. doi: 10.1016/j.bbrc.2009.02.110
PubMed Abstract | Full Text | CrossRef Full Text | Google Scholar
Kurkowska-Jastrzebska, I., Litwin, T., Joniec, I., Ciesielska, A., Przybyłkowski, A., Członkowski, A., et al. (2004). Dexamethasone protects against dopaminergic neurons damage in a mouse model of Parkinson’s disease. Int. Immunopharmacol. 4, 1307–1318. doi: 10.1016/j.intimp.2004.05.006
PubMed Abstract | Full Text | CrossRef Full Text | Google Scholar
Lill, C. M., Roehr, J. T., McQueen, M. B., Kavvoura, F. K., Bagade, S., Schjeide, B. M., et al. (2012). Comprehensive research synopsis and systematic metaanalyses in Parkinson’s disease genetics: the PDGene database. PLoS Genet. 8:e1002548. doi: 10.1371/journal.pgen.1002548
PubMed Abstract | Full Text | CrossRef Full Text | Google Scholar
Liu, M., and Bing, G. (2011). Lipopolysaccaride animal models for Parkinson’s disease. Parkinson Dis. 2011:327089. doi: 10.4061/2011/327089
PubMed Abstract | Full Text | CrossRef Full Text | Google Scholar
Liu, X., Cheng, R., Verbitsky, M., Kisselev, S., Browne, A., Mejia-Sanatana, H., et al. (2011). Genome-wide association study identifies candidate genes for Parkinson’s disease in an Ashkenazi Jewish population. BMC Med. Genet. 12:104. doi: 10.1186/1471-2350-12-104
PubMed Abstract | Full Text | CrossRef Full Text | Google Scholar
Maetzler, W., Apel, A., Langkamp, M., Deuschle, C., Dilger, S. S., Stirnkorb, J. G., et al. (2014). Comparable autoantibody serum levels against amyloid- and inflammation-associated proteins in Parkinson’s disease patients and controls. PLoS One 9:e88604. doi: 10.1371/journal.pone.0088604
PubMed Abstract | Full Text | CrossRef Full Text | Google Scholar
Matsubara, T., Funato, H., Kobayashi, A., Nobumoto, M., and Watanabe, Y. (2006). Reduced Glucocorticoid receptor alpha expression in mood disorder patients and first-degree relatives. Biol. Psychiatry 59, 689–695. doi: 10.1016/j.biopsych.2005.09.026
PubMed Abstract | Full Text | CrossRef Full Text | Google Scholar
McEwen, B. S. (2007). Physiology and neurobiology of stress and adaptation: central role of the brain. Physiol. Rev. 87, 873–904. doi: 10.1152/physrev.00041.2006
PubMed Abstract | Full Text | CrossRef Full Text | Google Scholar
McGeer, P. L., and McGeer, E. G. (2008). The alpha-synuclein burden hypothesis of Parkinson disease and its relationship to Alzheimer disease. Exp. Neurol. 212, 235–238. doi: 10.1016/j.expneurol.2008.04.008
PubMed Abstract | Full Text | CrossRef Full Text | Google Scholar
Moehle, M. S., Webber, P. J., Tse, T., Sukar, N., Standaert, D. G., DeSilva, T. M., et al. (2012). LRRK2 inhibition attenuates microglial inflammatory responses. J. Neurosci. 32, 1602–1611. doi: 10.1523/JNEUROSCI.5601-11.2012
PubMed Abstract | Full Text | CrossRef Full Text | Google Scholar
Mogi, M., Harada, M., Kondo, T., Riederer, P., Inagaki, H., Minami, M., et al. (1994). Interleukin-1 beta, interleukin-6, epidermal growth factor and transforming growth factor-alpha are elevated in the brain from parkinsonian patients. Neurosci. Lett. 180, 147–150. doi: 10.1016/0304-3940(94)90508-8
PubMed Abstract | Full Text | CrossRef Full Text | Google Scholar
Monahan, A. J., Warren, M., and Carvey, P. M. (2008). Neuroinflammation and peripheral immune infiltration in Parkinson’s disease: an autoimmune hypothesis. Cell Transplant. 17, 363–372. doi: 10.3727/096368908784423328
PubMed Abstract | Full Text | CrossRef Full Text | Google Scholar
Müller, T., Welnic, J., and Muhlack, S. (2007). Acute levodopa administration reduces cortisol release in patients with Parkinson’s disease. J. Neural Transm. 114, 347–350. doi: 10.1007/s00702-006-0552-0
PubMed Abstract | Full Text | CrossRef Full Text | Google Scholar
Nadeau, S., and Rivest, S. (2002). Endotoxemia prevents the cerebral inflammatory wave induced by intraparenchymal lipopolysaccharide injection: role of glucocorticoids and CD14. J. Immunol. 169, 3370–3381. doi: 10.4049/jimmunol.169.6.3370
PubMed Abstract | Full Text | CrossRef Full Text | Google Scholar
Nadeau, S., and Rivest, S. (2003). Glucocorticoids play a fundamental role in protecting the brain during innate immune response. J. Neurosci. 23, 5536–5544.
Nalls, M. A., Bras, J., Hernandez, D. G., Keller, M. F., Majounie, E., Renton, A. E., et al. (2015). NeuroX, a fast and efficient genotyping platform for investigation of neurodegenerative diseases. Neurobiol. Aging 36, 1605.e7–1605.e12. doi: 10.1016/j.neurobiolaging.2014.07.028
PubMed Abstract | Full Text | CrossRef Full Text | Google Scholar
Nalls, M. A., Pankratz, N., Lill, C. M., Do, C. B., Hernandez, D. G., Saad, M., et al. (2014). Large-scale meta-analysis of genome-wide association data identifies six new risk loci for Parkinson’s disease. Nat. Genet. 46, 989–993. doi: 10.1038/ng.3043
PubMed Abstract | Full Text | CrossRef Full Text | Google Scholar
Noh, H., Jeon, J., and Seo, H. (2014). Systemic injection of LPS induces region-specific neuroinflammation and mitochondrial dysfunction in normal mouse brain. Neurochem. Int. 69, 35–40. doi: 10.1016/j.neuint.2014.02.008
PubMed Abstract | Full Text | CrossRef Full Text | Google Scholar
Oakley, R. H., and Cidlowski, J. A. (2013). The biology of the glucocorticoid receptor: new signaling mechanisms in health and disease. J. Allergy Clin. Immunol. 132, 1033–1044. doi: 10.1016/j.jaci.2013.09.007
PubMed Abstract | Full Text | CrossRef Full Text | Google Scholar
O’Connor, K. A., Johnson, J. D., Hansen, M. K., Wieseler Frank, J. L., Maksimova, E., Watkins, L. R., et al. (2003). Peripheral and central proinflammatory cytokine response to a severe acute stressor. Brain Res. 991, 123–132. doi: 10.1016/j.brainres.2003.08.006
PubMed Abstract | Full Text | CrossRef Full Text | Google Scholar
Orr, C. F., Rowe, D. B., Mizuno, Y., Mori, H., and Halliday, G. M. (2005). A possible role for humoral immunity in the pathogenesis of Parkinson’s disease. Brain 128(Pt. 11), 2665–2674. doi: 10.1093/brain/awh625
PubMed Abstract | Full Text | CrossRef Full Text | Google Scholar
Ouchi, Y., Yoshikawa, E., Sekine, Y., Futatsubashi, M., Kanno, T., Ogusu, T., et al. (2005). Microglial activation and dopamine terminal loss in early Parkinson’s disease. Ann. Neurol. 57, 168–175. doi: 10.1002/ana.20338
PubMed Abstract | Full Text | CrossRef Full Text | Google Scholar
Perlman, W. R., Webster, M. J., Kleinman, J. E., and Weickert, C. S. (2004). Reduced glucocorticoid and estrogen receptor alpha messenger ribonucleic acid levels in the amygdala of patients with major mental illness. Biol. Psychiatry 56, 844–852. doi: 10.1016/j.biopsych.2004.09.006
PubMed Abstract | Full Text | CrossRef Full Text | Google Scholar
Pieper, H. C., Evert, B. O., Kaut, O., Riederer, P. F., Waha, A., and Wüllner, U. (2008). Different methylation of the TNF-alpha promoter in cortex and substantia nigra: implications for selective neuronal vulnerability. Neurobiol. Dis. 32, 521–527. doi: 10.1016/j.nbd.2008.09.010
PubMed Abstract | Full Text | CrossRef Full Text | Google Scholar
Pihlstrøm, L., Axelsson, G., Bjørnarå, K. A., Dizdar, N., Fardell, C., Forsgren, L., et al. (2013). Supportive evidence for 11 loci from genome-wide association studies in Parkinson’s disease. Neurobiol. Aging 34, 1708.e7–1708.e13. doi: 10.1016/j.neurobiolaging.2012.10.019
PubMed Abstract | Full Text | CrossRef Full Text | Google Scholar
Portela, A., and Esteller, M. (2010). Epigenetic modifications and human disease. Nat. Biotechnol. 28, 1057–1068. doi: 10.1038/nbt.1685
PubMed Abstract | Full Text | CrossRef Full Text | Google Scholar
Pott Godoy, M. C., Tarelli, R., Ferrari, C. C., Sarchi, M. I., and Pitossi, F. J. (2008). Central and systemic IL-1 exacerbates neurodegeneration and motor symptoms in a model of Parkinson’s disease. Brain 131(Pt. 7), 1880–1894. doi: 10.1093/brain/awn101
PubMed Abstract | Full Text | CrossRef Full Text | Google Scholar
Pringsheim, T., Jette, N., Frolkis, A., and Steeves, T. D. (2014). The prevalence of Parkinson’s disease: a systematic review and meta-analysis. Mov. Disord. 29, 1583–1590. doi: 10.1002/mds.25945
PubMed Abstract | Full Text | CrossRef Full Text | Google Scholar
Proukakis, C., Shoaee, M., Morris, J., Brier, T., Kara, E., Sheerin, U. M., et al. (2014). Analysis of Parkinson’s disease brain-derived DNA for alpha-synuclein coding somatic mutations. Mov. Disord. 29, 1060–1064. doi: 10.1002/mds.25883
PubMed Abstract | Full Text | CrossRef Full Text | Google Scholar
Ravenholt, R. T., and Foege, W. H. (1982). 1918 influenza, encephalitis lethargica, parkinsonism. Lancet 2, 860–864. doi: 10.1016/s0140-6736(82)90820-0
PubMed Abstract | Full Text | CrossRef Full Text | Google Scholar
Reale, M., Greig, N. H., and Kamal, M. A. (2009a). Peripheral chemo-cytokine profiles in Alzheimer’s and Parkinson’s diseases. Mini. Rev. Med. Chem. 9, 1229–1241. doi: 10.2174/138955709789055199
PubMed Abstract | Full Text | CrossRef Full Text | Google Scholar
Reale, M., Iarlori, C., Thomas, A., Gambi, D., Perfetti, B., Di Nicola, M., et al. (2009b). Peripheral cytokines profile in Parkinson’s disease. Brain Behav. Immun. 23, 55–63. doi: 10.1016/j.bbi.2008.07.003
PubMed Abstract | Full Text | CrossRef Full Text | Google Scholar
Rees, K., Stowe, R., Patel, S., Ives, N., Breen, K., Clarke, C. E., et al. (2011). Non-steroidal anti-inflammatory drugs as disease-modifying agents for Parkinson’s disease: evidence from observational studies. Cochrane Database Syst. Rev. 9:CD008454. doi: 10.1002/14651858.CD008454
PubMed Abstract | Full Text | CrossRef Full Text | Google Scholar
Reichstein, T. (1951). Chemistry of adrenal cortex hormones. Bull. Schweiz. Akad. Med. Wiss. 7, 359–370.
Ros-Bernal, F., Hunot, S., Herrero, M. T., Parnadeau, S., Corvol, J. C., Lu, L., et al. (2011). Microglial glucocorticoid receptors play a pivotal role in regulating dopaminergic neurodegeneration in parkinsonism. Proc. Natl. Acad. Sci. U S A 108, 6632–6637. doi: 10.1073/pnas.1017820108
PubMed Abstract | Full Text | CrossRef Full Text | Google Scholar
Rosenfeld, M. G., and Glass, C. K. (2001). Coregulator codes of transcriptional regulation by nuclear receptors. J. Biol. Chem. 276, 36865–36868. doi: 10.1074/jbc.r100041200
PubMed Abstract | Full Text | CrossRef Full Text | Google Scholar
Russo, I., Bubacco, L., and Greggio, E. (2014). LRRK2 and neuroinflammation: partners in crime in Parkinson’s disease?. J. Neuroinflammation 11:52. doi: 10.1186/1742-2094-11-52
PubMed Abstract | Full Text | CrossRef Full Text | Google Scholar
Saijo, K., Winner, B., Carson, C. T., Collier, J. G., Boyer, L., Rosenfeld, M. G., et al. (2009). A Nurr1/CoREST pathway in microglia and astrocytes protects dopaminergic neurons from inflammation-induced death. Cell 137, 47–59. doi: 10.1016/j.cell.2009.01.038
PubMed Abstract | Full Text | CrossRef Full Text | Google Scholar
Serrats, J., Schiltz, J. C., García-Bueno, B., van Rooijen, N., Reyes, T. M., and Sawchenko, P. E. (2010). Dual roles for perivascular macrophages in immune-to-brain signaling. Neuron 65, 94–106. doi: 10.1016/j.neuron.2009.11.032
PubMed Abstract | Full Text | CrossRef Full Text | Google Scholar
Sharkey, K. A., and Savidge, T. C. (2014). Role of enteric neurotransmission in host defense and protection of the gastrointestinal tract. Auton. Neurosci. 181, 94–106. doi: 10.1016/j.autneu.2013.12.006
PubMed Abstract | Full Text | CrossRef Full Text | Google Scholar
Sierra, A., Gottfried-Blackmore, A., Milner, T. A., McEwen, B. S., and Bulloch, K. (2008). Steroid hormone receptor expression and function in microglia. Glia 56, 659–674. doi: 10.1002/glia.20644
PubMed Abstract | Full Text | CrossRef Full Text | Google Scholar
Sorrells, A. D., Eicher, S. D., Harris, M. J., Pajor, E. A., and Richert, B. T. (2007). Periparturient cortisol, acute phase cytokine and acute phase protein profiles of gilts housed in groups or stalls during gestation. J. Anim. Sci. 85, 1750–1757. doi: 10.2527/jas.2007-0025
PubMed Abstract | Full Text | CrossRef Full Text | Google Scholar
Spillantini, M., Schmidt, M., Lee, V., Trojanowski, J. Q., Jakes, R., and Goedert, M. (1997). Alpha-synuclein in Lewy bodies. Nature 388, 839–840. doi: 10.1038/42166
PubMed Abstract | Full Text | CrossRef Full Text | Google Scholar
Stolp, H. B., and Dziegielewska, K. M. (2009). Review: role of developmental inflammation and blood-brain barrier dysfunction in neurodevelopmental and neurodegenerative diseases. Neuropathol. Appl. Neurobiol. 35, 132–146. doi: 10.1111/j.1365-2990.2008.01005.x
PubMed Abstract | Full Text | CrossRef Full Text | Google Scholar
Stypula, G., Kunert-Radek, J., Stepień, H., Zylińska, K., and Pawlikowski, M. (1996). Evaluation of interleukins, ACTH, cortisol and prolactin concentrations in the blood of patients with parkinson’s disease. Neuroimmunomodulation 3, 131–134. doi: 10.1159/000097237
PubMed Abstract | Full Text | CrossRef Full Text | Google Scholar
Su, X., and Federoff, J. H. (2014). Immune responses in Parkinson’s disease: interplay between central and peripheral immune systems. Biomed. Res. Int. 2014:275178. doi: 10.1155/2014/275178
PubMed Abstract | Full Text | CrossRef Full Text | Google Scholar
Sugama, S., Takenouchi, T., Kitani, H., Fujita, M., and Hashimoto, M. (2009). Microglial activation is inhibited by corticosterone in dopaminergic neurodegeneration. J. Neuroimmunol. 208, 104–114. doi: 10.1016/j.jneuroim.2009.01.016
PubMed Abstract | Full Text | CrossRef Full Text | Google Scholar
Surjit, M., Ganti, K. P., Mukherji, A., Ye, T., Hua, G., Metzger, D., et al. (2011). Widespread negative response elements mediate direct repression by agonist-liganded glucocorticoid receptor. Cell 145, 224–241. doi: 10.1016/j.cell.2011.03.027
PubMed Abstract | Full Text | CrossRef Full Text | Google Scholar
Tang, P., Chong, L., Li, X., Liu, Y., Liu, P., Hou, C., et al. (2014). Correlation between serum RANTES levels and the severity of Parkinson’s disease. Oxid. Med. Cell. Longev. 2014:208408. doi: 10.1155/2014/208408
PubMed Abstract | Full Text | CrossRef Full Text | Google Scholar
Topçu, M., Akyerli, C., Sayi, A., Törüner, G. A., Koçoğlu, S. R., Climbis, M., et al. (2002). Somatic mosaicism for a MECP2 mutation associated with classic Rett syndrome in a boy. Eur. J. Hum. Genet. 10, 77–81. doi: 10.1038/sj.ejhg.5200745
PubMed Abstract | Full Text | CrossRef Full Text | Google Scholar
Veldhuis, J. D., Iranmanesh, A., Lizarralde, G., and Johnson, M. L. (1989). Amplitude modulation of a burstlike mode of cortisol secretion subserves the circadian glucocorticoid rhythm. Am. J. Physiol. 257, E6–E14.
Wahner, A. D., Sinsheimer, J. S., Bronstein, J. M., and Ritz, B. (2007). Inflammatory cytokine gene polymorphisms and increased risk of Parkinson disease. Neurology 64, 836–840. doi: 10.1001/archneur.64.6.836
PubMed Abstract | Full Text | CrossRef Full Text | Google Scholar
Wakabayashi, K., and Takahashi, H. (1997). Neuropathology of autonomic nervous system in Parkinson’s disease. Eur. Neurol. 38(Suppl. 2), 2–7. doi: 10.1159/000113469
PubMed Abstract | Full Text | CrossRef Full Text | Google Scholar
Wilms, H., Zecca, L., Rosenstiel, P., Sievers, J., Deuschl, G., and Lucius, R. (2007). Inflammation in Parkinson’s diseases and other neurodegenerative diseases: cause and therapeutic implications. Curr. Pharm. Des. 13, 1925–1928. doi: 10.2174/138161207780858429
PubMed Abstract | Full Text | CrossRef Full Text | Google Scholar
Wissemann, W. T., Hill-Burns, E. M., Zabetian, C. P., Factor, S. A., Patsopoulos, N., Hoglund, B., et al. (2013). Association of Parkinson disease with structural and regulatory variants in the HLA region. Am. J. Hum. Genet. 93, 984–993. doi: 10.1016/j.ajhg.2013.10.009
PubMed Abstract | Full Text | CrossRef Full Text | Google Scholar
Young, E. A., Abelson, J., and Lightman, S. L. (2004). Cortisol pulsatility and its role in stress regulation and health. Front. Neuroendocrinol. 25, 69–76. doi: 10.1016/j.yfrne.2004.07.001
PubMed Abstract | Full Text | CrossRef Full Text | Google Scholar
Zarković, M., Ignjatović, S., Dajak, M., Cirić, J., Beleslin, B., Savić, S., et al. (2008). Cortisol response to ACTH stimulation correlates with blood interleukin 6 concentration in healthy humans. Eur. J. Endocrinol. 159, 649–652. doi: 10.1530/EJE-08-0544
PubMed Abstract | Full Text | CrossRef Full Text | Google Scholar
Zhou, J., and Cidlowski, J. A. (2005). The human glucocorticoid receptor: one gene, multiple proteins and diverse responses. Steroids 70, 407–417. doi: 10.1016/j.steroids.2005.02.006
PubMed Abstract | Full Text | CrossRef Full Text | Google Scholar
Keywords: glucocorticoid receptor, Parkinson’s disease (PD), neuroinflammation, neurodegeneration, microglia
Citation: Herrero M-T, Estrada C, Maatouk L and Vyas S (2015) Inflammation in Parkinson’s disease: role of glucocorticoids. Front. Neuroanat. 9:32. doi: 10.3389/fnana.2015.00032
Received: 31 January 2015; Accepted: 28 February 2015;
Published online: 02 April 2015.
Edited by:
Javier Blesa, Columbia University, USAReviewed by:
Jose L. Labandeira-Garcia, University of Santiago de Compostela, SpainCopyright © 2015 Herrero, Estrada, Maatouk and Vyas. This is an open-access article distributed under the terms of the Creative Commons Attribution License (CC BY). The use, distribution and reproduction in other forums is permitted, provided the original author(s) or licensor are credited and that the original publication in this journal is cited, in accordance with accepted academic practice. No use, distribution or reproduction is permitted which does not comply with these terms.
*Correspondence: María-Trinidad Herrero, Clinical and Experimental Neuroscience (NiCE-IMIB), Institute for Bio-Health Research of Murcia, School of Medicine, Campus Mare Nostrum, University of Murcia, 30100 Murcia, SpainbXRoZXJyZXJAdW0uZXM=;
Sheela Vyas, Laboratory of Gene Regulation and Adaptive Behaviors, Department of Neuroscience Paris Seine, INSERM U 1130, CNRS UMR 8246, UPMC UM 119, Université Pierre et Marie Curie, Bâtiment B, 9 Quai Saint Bernard, 75252 Paris CEDEX 05, Francec2hlZWxhLnZ5YXNAdXBtYy5mcg==
Disclaimer: All claims expressed in this article are solely those of the authors and do not necessarily represent those of their affiliated organizations, or those of the publisher, the editors and the reviewers. Any product that may be evaluated in this article or claim that may be made by its manufacturer is not guaranteed or endorsed by the publisher.
Research integrity at Frontiers
Learn more about the work of our research integrity team to safeguard the quality of each article we publish.