- Department of Ultrastructural Research, National Institute of Neuroscience, National Center of Neurology and Psychiatry, Kodaira, Tokyo, Japan
Structures associated with the small-scale module called “minicolumn” can be observed frequently in the cerebral cortex. However, the description of functional characteristics remains obscure. A significant confounding factor is the marked variability both in the definition of a minicolumn and in the diagnostic markers for identifying a minicolumn (see for review, Jones, 2000; DeFelipe et al., 2002; Rockland and Ichinohe, 2004). Within a minicolumn, cell columns are easily visualized by conventional Nissl staining. Dendritic bundles were first discovered with Golgi methods, but are more easily seen with microtubule-associated protein 2 immunohistochemistry. Myelinated axon bundles can be seen by Tau immunohistochemistry or myelin staining. Axon bundles of double bouquet cell can be seen by calbindin immunohistochemistry. The spatial interrelationship among these morphological elements is more complex than expected and is neither clear nor unanimously agreed upon. In this review, I would like to focus first on the minicolumnar structure found in layers 1 and 2 of the rat granular retrosplenial cortex. This modular structure was first discovered as a combination of prominent apical dendritic bundles from layer 2 pyramidal neurons and spatially matched thalamocortical patchy inputs (Wyss et al., 1990). Further examination showed more intricate components of this modular structure, which will be reviewed in this paper. Second, the postnatal development of this structure and potential molecular players for its formation will be reviewed. Thirdly, I will discuss how this modular organization is transformed in mutant rodents with a disorganized layer structure in the cerebral cortex (i.e., reeler mouse and shaking rat Kawasaki). Lastly, the potential significance of this type of module will be discussed.
Introduction
Morphologic small-scale modules or “minicolumn” structures can be observed frequently in the cerebral cortex. Functional minicolumns have also been identified, but the relationship between morphologic and functional minicolumns is ambiguous. Although morphologic minicolumn structures can be observed frequently, there is a marked variability in the definition of minicolumns, as well as in the diagnostic markers used for identifying these minicolumns (for review see Jones, 2000; DeFelipe et al., 2002; Rockland and Ichinohe, 2004). For example, the cell column is visualized by conventional Nissl staining. Dendritic bundles were first discovered using Golgi methods, but these structures can today be more easily seen using microtubule-associated protein 2 (MAP2) immunohistochemistry. Myelinated axon bundles can be visualized using Tau immunohistochemistry or conventional myelin staining, and axon bundles of double bouquet cell can be seen using calbindin immunohistochemistry. The spatial interrelationship between these differently identified morphologic minicolumns is more complex than originally expected, and is neither clear nor unanimously defined.
In this review, I will focus on a well-examined small module exiting in layers 1 and 2 of the rat granular retrosplenial (GRS) cortex. This modular structure was first described as a module consisting of a combination of prominent apical dendritic bundles from layer 2 pyramidal neurons and thalamocortical patchy inputs, which are spatially matched with the dendritic bundles (Wyss et al., 1990). Further examination showed more intricate components of this modular structure, which will be reviewed first in this paper. Second, the postnatal development of this structure and potential molecular mechanisms underlying the formation of this module will be reviewed. Thirdly, I will describe the way in which this modular organization in wild-type rodents is transformed in mutant rodents, which have a disorganized layer structure in the cerebral cortex (i.e., the reeler mouse and shaking rat Kawasaki, SRK). Lastly, the potential significance of this type of module will be discussed.
Structural and Chemical Organization of Modular Structures in Layers 1 and 2 of the Adult Rat GRS
The modular GRS organization was first discovered by Wyss et al. (1990) by injecting the retrograde tracer Fluoro-Gold into the contralateral rat GRS. The well-filled dendrites of callosally projecting layer 2 pyramidal neurons were found to group together in discrete bundles, as they ascend toward the pial surface (Figure 1). Following this initial discovery, these prominent dendritic bundles have been demonstrated by several other methods. Most recently, molecular techniques have been used for visualizing fluorescent protein-filled layer 2 pyramidal neurons (Figure 2A; Miyashita et al., 2010; Zgraggen et al., 2011). As shown in Figure 1, the dendritic bundles in layer 1b/c are tighter than those in other layers, with a reported width of 30–100 μm and a distance between bundles of approximately 30–200 μm (Wyss et al., 1990; Ichinohe and Rockland, 2002). Near the layer 1b/1a interface, the dendritic tufts diverge and spread out in layer 1a to occupy the entire width of the layer (Figure 2A). The extension range of these tufts is about the same range as the distance between dendritic bundles (i.e., 30–200 μm; Wyss et al., 1990; Ichinohe and Rockland, 2002).
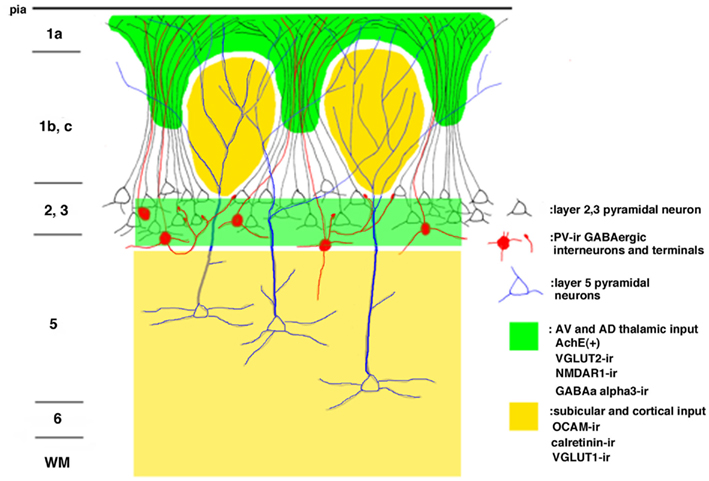
Figure 1. Schematic drawing of the modular organization of the layer 1 granular retrosplenial cortex (GRS). Arabic numbers represent layer numbers. ir, immunoreactive.
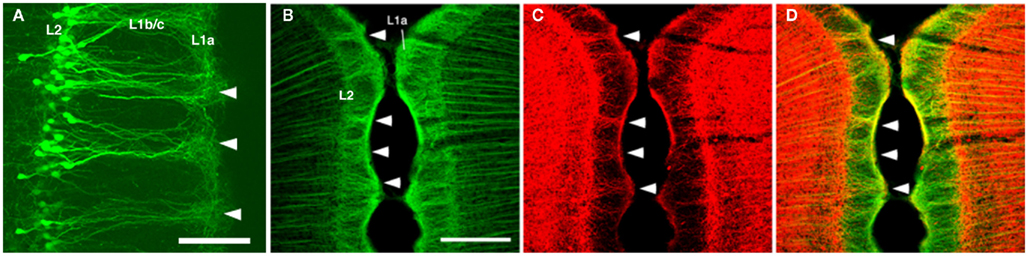
Figure 2. (A) Dendritic bundles in layer 1 [postnatal day (P) 10], visualized by electroporation of enhanced green fluorescence protein (EGFP) at embryonic day (E) 18. (B–D) Confocal micrographs of layer 1 of the rat GRS stained by double-immunofluorescence for microtubule-associated protein 2 (MAP2; green) and parvalbumin (PV; red). Coronal sections. Scale bar: (A), 50 μm; (B–D), 200 μm.
Immunohistofluorescence for MAP2 has been a convenient method for visualizing these dendritic modules (Figure 2B; Ichinohe and Rockland, 2002; Ichinohe et al., 2003b; Miró-Bernié et al., 2006). Using double/triple-immunofluorescence with a combination of appropriate antibodies, including antibodies against MAP2, the spatial relationship between dendritic modules and other structures labeled by the different antibodies can be easily examined (summarized in Figure 1). One interesting example is that double-immunofluorescence for MAP2 and parvalbumin (PV), a marker for a subpopulation of GABAergic neurons, reveals that PV-positive dendrites co-localize with apical dendritic bundles (Figures 1 and 2B–D). This intricate structure may be related to feedforward inhibition, as characterized by electrophysiologic studies in other thalamocortical systems (Swadlow and Gusev, 2000). That is, these PV-positive neurons, activated by thalamic inputs, may inhibit layer 2 pyramidal neurons receiving the same thalamic inputs. Another possibility is that, since some PV-positive neurons have laterally widespread axonal fields (Kisvárday et al., 2002), these PV-positive neurons can inhibit intricate combinations of pyramidal neurons within different modules, which receive thalamic inputs different from inputs to these PV-positive interneurons. This potential organization may work as a substrate for lateral inhibition between modules.
Immunohistochemistry using OCAM, a cell adhesion molecule, has revealed patchy structures in layer 1 of the GRS (Figures 1 and 3A,D). The patches of OCAM-positive and MAP2-positive areas can be seen to interdigitate (Figure 3). To identify OCAM-positive structures in layer 1, electron microscopy was used. This shows that OCAM-positive structures are composed mostly of dendrites (Ichinohe et al., 2003b). In situ hybridization for the OCAM gene shows that expression of the gene occurs in layer 5/6 pyramidal neurons (Ichinohe et al., 2008). Thus, the OCAM-positive patches in layer 1 appear to constitute an aggregation of apical dendritic tufts of layer 5 pyramidal neurons (Figure 1). OCAM is associated with the fasciculation of subsets of olfactory and vomeronasal axons (Yoshihara et al., 1997) and the accurate segregation of odorant receptor-specific axons (Alenius and Bohm, 2003). Moreover, recent studies in olfactory glomeruli have shown that OCAM is required for establishing or maintaining the compartmental organization and segregation of axodendritic and dendrodendritic synapses within glomeruli (Walz et al., 2006), and is important for the synchrony of mitral cell activity in olfactory glomeruli (Borisovska et al., 2011). In the dendritic module in GRS layer 1, OCAM may be also involved in the maintenance of compartmental organization (i.e., segregation of MAP2-positive dendritic bundles from layer 2 pyramidal neurons and the complementary aggregation of OCAM-positive apical dendrites from layer 5 pyramidal neurons).
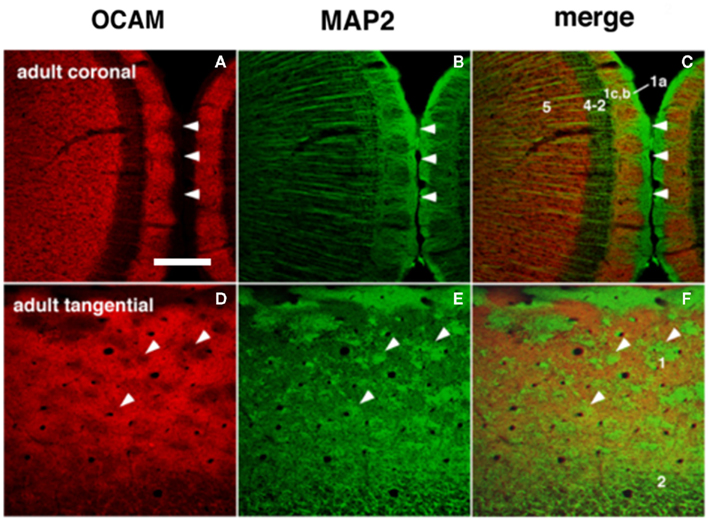
Figure 3. Dendritic modules in layer 1 are visualized by OCAM-immunoreactive (ir) and MAP2-ir in adults. The right column shows merged images where patches of OCAM-ir and MAP2-ir are seen to interdigitate. (A–C) Coronal sections. (D–F) Semi-tangential sections. Arrowheads point to corresponding locations in two sets consisting of three figures (A–F). Arabic numbers indicate layers. Scale bar: 200 μm.
Vesicular glutamate transporter 1 (VGLUT1) and VGLUT2 are convenient markers for corticocortical and thalamocortical terminals in the cerebral cortex, respectively (Fujiyama et al., 2001). Double-immunofluorescence for OCAM and VGLUT1 or VGLUT2 has shown that OCAM- and VGLUT1-positive modules are co-localized and that OCAM- and VGLUT2-positive modules are interdigitated in layer 1 (Figures 1 and 4). These results underscore the notion that OCAM-negative (i.e., MAP2-positive) apical dendritic bundles from layer 2 pyramidal neurons are targeted by thalamocortical terminals and that OCAM-positive aggregations of apical dendritic tufts of layer 5 pyramidal neurons are targeted by corticocortical inputs (Shibata, 1993; Van Groen and Wyss, 1995, 2003; Miró-Bernié et al., 2006; Ichinohe et al., 2008). These results indicate that not only different types of dendrites but also different kinds of axon terminals are aggregated and segregated in this module. That is, specific combinations of dendritic modules (“recipients”) and axon terminal modules (“inputs”) are co-localized. This type of co-localization of recipient and input modules has previously been described in other cortical areas and layers (e.g., the rodent barrel cortex and the honeycomb-like structure at the border of layers 1 and 2 of the cerebral cortex; Datwani et al., 2002; Ichinohe et al., 2003a; Ichinohe and Rockland, 2004).
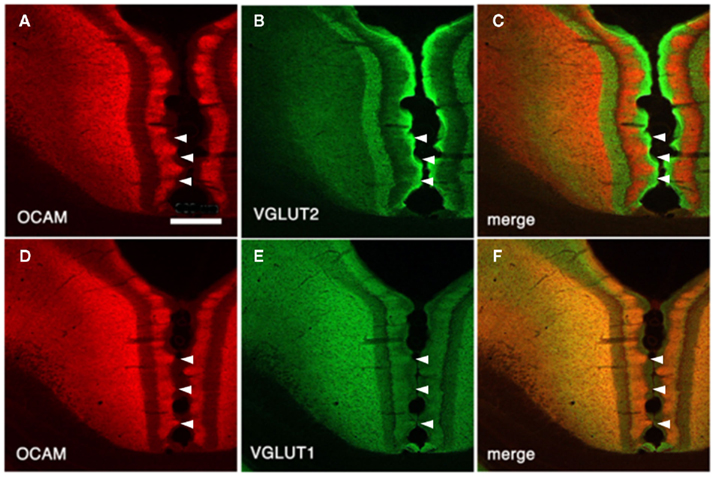
Figure 4. Architectonics of the rat GRS, visualized by immunohistochemistry for OCAM and vesicular glutamate transporter 1 (VGLUT2; presumptive thalamocortical terminations) or VGLUT1 (presumptive corticocortical terminations). (A–C) Coronal sections reacted for OCAM and VGLUT2. OCAM-ir in the wild-type rat (A) is bistratified, with one superficial band in layers 1b and c and another, deeper band in layers 5 and 6. Note the notch-like appearance of layer 1. Similarly, VGLUT2 concentrates are seen in two bands, but these correspond to layer 1a and layers 3 and 4 (B). Double-immunofluorescence for OCAM and VGLUT2 directly demonstrates this complementary relationship (C). Layer 2 has low levels of both OCAM and VGLUT2. (D–F) Coronal sections reacted for OCAM and VGLUT2. Scale bar: 300 μm.
Postnatal Development of the Modular Organization in the GRS
The developmental time-course of the dendritic bundles in the GRS has been investigated using immunohistochemistry for MAP2 and glutamate receptor subunits 2/3 (GluR2/3; Ichinohe et al., 2003b). Bundles in layer 1 are apparent as early as postnatal day (P) 5, first using GluR2/3 immunohistochemistry and then, from P14, using MAP2 immunohistochemistry. As a step toward understanding the mechanisms underlying dendritic aggregation, we further investigated the ontogeny of expression of the cell adhesion molecule OCAM. OCAM exhibits a patchy distribution in layer 1 from P3 to adulthood, and the regions of weak OCAM immunoreactivity selectively correspond to the dendritic bundles (using GluR2/3 and MAP2 immunohistochemistry). The periodic geometry of OCAM-positive regions, the time-course of their appearance, and their distinct localization, complementary to the bundles, support the possibility that OCAM significantly contributes to the establishment and maintenance of dendritic modules. More specifically, the interdigitating relationship between regions of high OCAM immunoreactivity and the dendritic bundles in layer 1 suggests that OCAM may have a repellent influence on the formation of these bundles (see also above section).
In order to identify molecules other than OCAM that are involved in the formation of the GRS layer 1 bundles from layer 2 pyramidal neurons, microarray techniques have been used (Miyashita et al., 2010). Several genes, including that for neurotrophin-3 (NT-3), have been found to be highly and specifically expressed in GRS layer 2 at P3 versus P12 (i.e., before and after bundle formation). Specificity was inferred by comparisons with GRS layer 5 at P3 and with barrel cortex layer 2 at P3. In barrel cortex (with low NT-3 expression), layer 2 pyramidal neurons do not form prominent apical dendritic bundles. To examine whether NT-3-mediated events are causally involved in bundle formation, we used in utero electroporation to overexpress NT-3 in other cortical areas. This overexpression succeeded in producing prominent bundles of dendrites originating from layer 2 neurons in the barrel field cortex, where layer 2 bundles are normally absent (Figure 5). The controlled ectopic induction of dendritic bundles identifies a new role for NT-3 and a new in vivo model for investigating dendritic bundles and their formation.
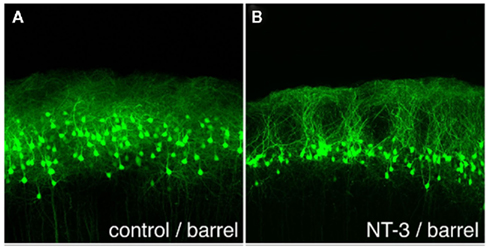
Figure 5. Ectopically expressed neurotrophin-3 (NT-3) induces dendritic bundles in neocortical layer 1. (A) EGFP-expressing layer 2 pyramidal neurons at P20, after electroporation of EGFP alone at E18. Note the uniform distribution of cells and dendrites, without bundles. (B) Electroporation of EGFP and NT-3 at E18 induces distinct dendritic bundles in the barrel cortex. Scale bar: 100 μm.
Transformation of the GRS Architecture from Wild-Type Rodents to Mutant Rodents
To further investigate the distinctive compartmental organization in the GRS, we examined reelin-deficient mutant rodents; namely, the reeler mouse and SRK. In these animals, the normal GRS lamination is conspicuously disrupted, and dendrites are malpositioned (Lambert de Rouvroit and Goffinet, 1998; Kikkawa et al., 2003). To understand these abnormalities in more detailed level, it is necessary to examine how the highly organized laminar and modular architecture of the GRS in the wild-type rodent is transformed in these mutant rodents (Ichinohe et al., 2008).
In both the SRK and reeler GRS, immunohistochemistry for OCAM revealed a patch and matrix-like mosaic, with large OCAM-negative patches, in the middle of the cortical thickness, embedded in an OCAM-positive matrix (Figure 6). Steindler et al. (1994) also reported a similar patch-matrix pattern in the reeler visual cortex using acetylcholinesterase staining; however, the visual cortex described in their study may actually have been the GRS, as discussed by Ichinohe et al. (2008). It is difficult to conclude that the patch-matrix organization in mutant rodents is comparable to the micromodularity of layer 1 in wild-type rodents. Nevertheless, it may be significant that the discontinuous appearance of both features is strikingly similar between mutant and wild-type rodents. Moreover, the thalamic and cortical afferents in mutant rodents maintain the same relationship in the patch-matrix configuration as in wild-type rodents (Figure 4); namely, OCAM-positive areas match VGLUT1 (corticocortical input)-rich areas and OCAM-negative areas match VGLUT2 (thalamocortical input)-rich areas. Further, by filling neurons with Lucifer Yellow in fixed slices of the reeler GRS, we ascertained that these neurons can be grouped into two populations, with dendrites showing preference to either OCAM-negative patches (Figure 7A) or the OCAM-positive matrix (Figures 7B–E; Ichinohe et al., 2008). From additional in situ hybridization and electron microscopy results, we conclude that the OCAM-positive matrix in mutant rodents is filled with aggregated dendrites of neurons, comparable to layer 5 neurons in wild-type, and OCMA-negative patch in mutant rodents with aggregated dendrites of layer 2 neurons. Thus, even in the disrupted laminar cortex of the reeler mouse and SRK, OCAM may exert dendritic population-dependent homophilic or repellent effects. Further, these results suggest that, even though layer formation (and subsequently the position of the cell bodies) is disorganized in mutant rodents, recipient dendrites, and specific inputs aggregate appropriately and probably maintain proper synaptic connectivity, sufficient for maintaining relatively normal cortical function in these mutant animals (Simmons and Pearlman, 1983).
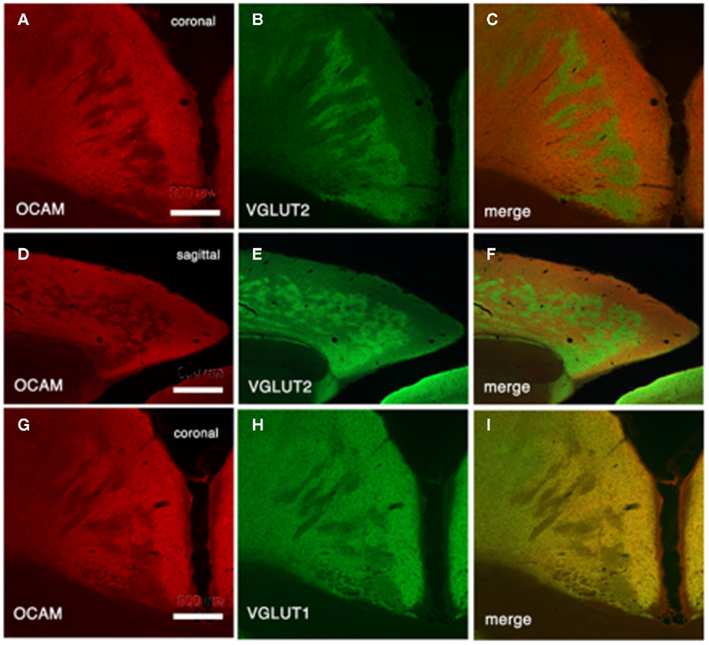
Figure 6. Architectonics of mutant GRS, visualized by immunohistochemistry for OCAM and VGLUT2 (presumptive thalamocortical terminations) or VGLUT1 (presumptive corticocortical terminations). (A–C) Coronal sections reacted for OCAM and VGLUT2 from shaking rat Kawasaki. (D–F) Tangential sections. (G–I) Coronal sections reacted for OCAM and VGLUT1. Scale bar: (A–C,G–I), 300 μm; (D–F), 600 μm.

Figure 7. Pyramidal cell dendrites preferentially arborize in either patch or matrix compartments in reeler GRS. (A) Coronal section reacted for VGLUT2 (red), with a Lucifer yellow (LY)-filled neuron (green). This LY-filled neuron has a soma located in a zone of low VGLUT2-ir (presumably equivalent to the OCAM-positive matrix) and many dendrite branches within a VGLUT2-dense patch (presumably equivalent to an OCAM-negative patch). These dendritic portions within VGLUT2-dense patches have spines [arrowheads in (A″)], but not the portions outside the VGLUT2-dense region (A′). Insets in (A) [i.e., (A′,A″)] show a higher magnification of the images in the white boxes [(A′) from left box and (A″) from right box]. (B–E) Serial sections of a LY-filled neuron (green), double reacted for OCAM (red). The cell body is located within the OCAM-positive matrix (C). An apical dendrite-like process gives off an oblique dendrite proximally, before entering an OCAM-negative patch. An apical tuft branches just at the border between patch and matrix compartments (C). The basal dendrites tend, like the cell body, to stay within the OCAM-dense matrix. Scale bar: (A–E), 50 μm; (A′,A″), 20 μm.
Potential Significance of GRS Small-Scale Modular Organization
The GRS in rats is implicated in a wide range of behaviors, including visual and vestibular integration, path integration, and spatial navigation, as well as certain aspects of learning and memory (Cooper et al., 2001; Garden et al., 2009; Vann et al., 2009; Aggleton, 2010). The GRS is part of a heavily interconnected limbic circuit, including the anterior thalamic nuclei and subiculum. The underlying substrates involved in GRS functioning and the true significance of upper layer modular organization are undoubtedly complex. More anatomical and physiological studies will obviously be necessary (see recent anatomical study by Odagiri et al., 2011).
Prominent characteristics of the modular organization of the rat GRS in layer 1 include (1) the aggregation or segregation of apical dendrites (“recipients”) of the same or different types of pyramidal neurons, respectively, and (2) the fact that certain types of terminals (“inputs”) either match or interdigitate with a particular type of dendritic aggregation. As mentioned above, this type of recipient and input matching also occurs in other small-scale modular organizations in the neocortex (i.e., honeycomb-like organization in the uppermost layers: Ichinohe et al., 2003a; Ichinohe and Rockland, 2004). Modular aggregations consisting of appropriate types of recipients and inputs, such as those seen in the rat GRS, may help to achieve efficient and quick synaptic wiring changes, such as occur in the context of learning and memory.
Conflict of Interest Statement
The author declares that the research was conducted in the absence of any commercial or financial relationships that could be construed as a potential conflict of interest.
Acknowledgments
This study was supported by a Grant-in-Aid for Scientific Research on Innovative Areas, “Face perception and recognition,” by the Ministry of Education, Science, Sports and Culture, Japan.
References
Aggleton, J. P. (2010). Understanding retrosplenial amnesia: insights from animal studies. Neuropsychologia 48, 2328–2338.
Alenius, M., and Bohm, S. (2003). Differential function of RNCAM isoforms in precise target selection of olfactory sensory neurons. Development 130, 917–927.
Borisovska, M., McGinley, M. J., Bensen, A., and Westbrook, G. L. (2011). Loss of olfactory cell adhesion molecule reduces the synchrony of mitral cell activity in olfactory glomeruli. J. Physiol. 589, 1927–1941.
Cooper, B. G., Manka, T. F., and Mizumori, S. J. (2001). Finding your way in the dark: the retrosplenial cortex contributes to spatial memory and navigation without visual cues. Behav. Neurosci. 115, 1012–1028.
Datwani, A., Iwasato, T., Itohara, S., and Erzurumlu, R. S. (2002). NMDA receptor-dependent pattern transfer from afferents to postsynaptic cells and dendritic differentiation in the barrel cortex. Mol. Cell. Neurosci. 21, 477–492.
DeFelipe, J., Alonso-Nanclares, L., and Arellano, J. I. (2002). Microstructure of the neocortex: comparative aspects. J. Neurocytol. 31, 299–316.
Fujiyama, F., Furuta, T., and Kaneko, T. (2001). Immunocytochemical localization of candidates for vesicular glutamate transporters in the rat cerebral cortex. J. Comp. Neurol. 435, 379–387.
Garden, D. L., Massey, P. V., Caruana, D. A., Johnson, B., Warburton, E. C., Aggleton, J. P., and Bashir, Z. I. (2009). Anterior thalamic lesions stop synaptic plasticity in retrosplenial cortex slices: expanding the pathology of diencephalic amnesia. Brain 132, 1847–1857.
Ichinohe, N., Fujiyama, F., Kaneko, T., and Rockland, K. S. (2003a). Honeycomb-like mosaic at the border of layers 1 and 2 in the cerebral cortex. J. Neurosci. 23, 1372–1382.
Ichinohe, N., Yoshihara, Y., Hashikawa, T., and Rockland, K. S. (2003b). Developmental study of dendritic bundles in layer 1 of the rat granular retrosplenial cortex with special reference to a cell adhesion molecule, OCAM. Eur. J. Neurosci. 18, 1764–1774.
Ichinohe, N., Knight, A., Ogawa, M., Ohshima, T., Mikoshiba, K., Yoshihara, Y., Terashima, T., and Rockland, K. S. (2008). Unusual patch-matrix organization in the retrosplenial cortex of the reeler mouse and shaking rat Kawasaki. Cereb. Cortex 18, 1125–1138.
Ichinohe, N., and Rockland, K. S. (2002). Parvalbumin positive dendrites co-localize with apical dendritic bundles in rat retrosplenial cortex. Neuroreport 13, 757–761.
Ichinohe, N., and Rockland, K. S. (2004). Region specific micromodularity in the uppermost layers in primate cerebral cortex. Cereb. Cortex 14, 1173–1184.
Jones, E. G. (2000). Microcolumns in the cerebral cortex. Proc. Natl. Acad. Sci. U.S.A. 97, 5019–5021.
Kikkawa, S., Yamamoto, T., Misaki, K., Ikeda, Y., Okado, H., Ogawa, M., Woodhams, P. L., and Terashima, T. (2003). Missplicing resulting from a short deletion in the reelin gene causes reeler-like neuronal disorders in the mutant shaking rat Kawasaki. J. Comp. Neurol. 463, 303–315.
Kisvárday, Z. F., Ferecskó, A. S., Kovács, K., Buzás, P., Budd, J. M., and Eysel, U. T. (2002). One axon-multiple functions: specificity of lateral inhibitory connections by large basket cells. J. Neurocytol. 31, 255–364.
Lambert de Rouvroit, C., and Goffinet, A. M. (1998). The reeler mouse as a model of brain development. Adv. Anat. Embryol. Cell Biol. 150, 1–106.
Miró-Bernié, N., Ichinohe, N., Pérez-Clausell, J., and Rockland, K. S. (2006). Zinc-rich transient vertical modules in the rat retrosplenial cortex during postnatal development. Neuroscience 138, 523–535.
Miyashita, T., Wintzer, M., Kurotani, T., Konishi, T., Ichinohe, N., and Rockland, K. S. (2010). Neurotrophin-3 is involved in the formation of apical dendritic bundles in cortical layer 2 of the rat. Cereb. Cortex 20, 229–240.
Odagiri, S., Meguro, R., Asano, Y., Tani, T., and Ichinohe, N. (2011). Single axon branching analysis in rat thalamocortical projection from the anteroventral thalamus to the granular retrosplenial cortex. Front. Neuroanat. 5, 63. doi: 10.3389/fnana.2011.00063
Rockland, K. S., and Ichinohe, N. (2004). Some thoughts on cortical minicolumns. Exp. Brain Res. 158, 265–277.
Shibata, H. (1993). Efferent projections from the anterior thalamic nuclei to the cingulate cortex in the rat. J. Comp. Neurol. 330, 533–542.
Simmons, P. A., and Pearlman, A. L. (1983). Receptive-field properties of transcallosal visual cortical neurons in the normal and reeler mouse. J. Neurophysiol. 50, 838–848.
Steindler, D. A., Faissner, A., and Harrington, K. L. (1994). A unique mosaic in the visual cortex of the reeler mutant mouse. Cereb. Cortex 4, 129–137.
Swadlow, H. A., and Gusev, A. G. (2000). The influence of single VB thalamocortical impulses on barrel columns of rabbit somatosensory cortex. J. Neurophysiol. 83, 2802–2813.
Van Groen, T., and Wyss, J. M. (1995). Projections from the anterodorsal and anteroventral nucleus of the thalamus to the limbic cortex in the rat. J. Comp. Neurol. 358, 584–604.
Van Groen, T., and Wyss, J. M. (2003). Connections of the retrosplenial granular b cortex in the rat. J. Comp. Neurol. 463, 249–263.
Vann, S. D., Aggleton, J. P., and Maguire, E. A. (2009). What does the retrosplenial cortex do? Nat. Rev. Neurosci. 10, 792–802.
Walz, A., Mombaerts, P., Greer, C. A., and Treloar, H. B. (2006). Disrupted compartmental organization of axons and dendrites within olfactory glomeruli of mice deficient in the olfactory cell adhesion molecule, OCAM. Mol. Cell. Neurosci. 32, 1–14.
Wyss, J. M., Van Groen, T., and Sripanidkulchai, K. (1990). Dendritic bundling in layer I of granular retrosplenial cortex: intracellular labeling and selectivity of innervation. J. Comp. Neurol. 295, 33–42.
Yoshihara, Y., Kawasaki, M., Tamada, A., Fujita, H., Hayashi, H., Kagamiyama, H., and Mori, K. (1997). OCAM: a new member of the neural cell adhesion molecule family related to zone-to-zone projection of olfactory and vomero-nasal axons. J. Neurosci. 17, 5830–5842.
Keywords: cortical modular organization, dendritic bundle, input and recipient matching, thalamocortical, corticocortical
Citation: Ichinohe N (2012) Small-scale module of the rat granular retrosplenial cortex: an example of the minicolumn-like structure of the cerebral cortex. Front. Neuroanat. 5:69. doi: 10.3389/fnana.2011.00069
Received: 13 September 2011;
Paper pending published: 02 November 2011;
Accepted: 28 December 2011;
Published online: 10 January 2012.
Edited by:
Zoltan F. Kisvarday, University of Debrecen, HungaryReviewed by:
Antonio Pereira, Federal University of Rio Grande do Norte, BrazilToshio Terashima, Kobe University School of Medicine, Japan
Copyright: © 2012 Ichinohe. This is an open-access article distributed under the terms of the Creative Commons Attribution Non Commercial License, which permits non-commercial use, distribution, and reproduction in other forums, provided the original authors and source are credited.
*Correspondence: Noritaka Ichinohe, Department of Ultrastructural Research, National Institute of Neuroscience, National Center of Neurology and Psychiatry, 4-1-1 Ogawa-Higashi, Kodaira, Tokyo 187-8502, Japan. e-mail: nichino@ncnp.go.jp