- Department of Integrative Neurophysiology, Graduate School of Medical Sciences, Kanazawa University, Kanazawa, Japan
Animals need sleep, and the suprachiasmatic nucleus, the center of the circadian rhythm, plays an important role in determining the timing of sleep. The main input to the suprachiasmatic nucleus is the retinohypothalamic tract, with additional inputs from the intergeniculate leaflet pathway, the serotonergic afferent from the raphe, and other hypothalamic regions. Within the suprachiasmatic nucleus, two of the major subtypes are vasoactive intestinal polypeptide (VIP)-positive neurons and arginine-vasopressin (AVP)-positive neurons. VIP neurons are important for light entrainment and synchronization of suprachiasmatic nucleus neurons, whereas AVP neurons are important for circadian period determination. Output targets of the suprachiasmatic nucleus include the hypothalamus (subparaventricular zone, paraventricular hypothalamic nucleus, preoptic area, and medial hypothalamus), the thalamus (paraventricular thalamic nuclei), and lateral septum. The suprachiasmatic nucleus also sends information through several brain regions to the pineal gland. The olfactory bulb is thought to be able to generate a circadian rhythm without the suprachiasmatic nucleus. Some reports indicate that circadian rhythms of the olfactory bulb and olfactory cortex exist in the absence of the suprachiasmatic nucleus, but another report claims the influence of the suprachiasmatic nucleus. The regulation of circadian rhythms by sensory inputs other than light stimuli, including olfaction, has not been well studied and further progress is expected.
Introduction
Sleep is essential for survival, and animals maintain their physical and mental health by maintaining a certain rhythm of waking and sleeping at the right time and in the right state. In humans, waking and sleeping roughly correspond to the day and night in the outside world. Diurnal organisms, such as humans, are active during the day, while nocturnal animals, such as mice, are more active at night and sleep more at the opposite time. The suprachiasmatic nucleus (SCN) is the center that controls all circadian rhythms, including wake–sleep, appetite, autonomic system, and neuroendocrine rhythms (Hastings et al., 2018). This control of circadian rhythms allows animals to maintain a roughly 24-h cycle, even in the absence of light from the outside world. The circadian rhythm period of the body clock is approximately 24 h, not exactly 24 h, so each morning’s exposure to light resets the body clock and aligns it with the external day-night cycle. In this review, we will discuss the inputs to the SCN, the networks within the SCN, the outputs from the SCN, and the effects of stimuli other than light on circadian rhythms, particularly the olfactory system.
Inputs to the SCN
There are three major inputs to the SCN (Abrahamson and Moore, 2001; Reghunandanan and Reghunandanan, 2006; Morin, 2013) (Figure 1). The most important input is from the retina through the retinohypothalamic tract (RHT) to the ventral core region of the SCN which is thought to provide input primarily to vasoactive intestinal polypeptide (VIP)-positive and gastrin-releasing peptide (GRP)-positive neurons (Lokshin et al., 2015). Loss of melanopsin-containing intrinsically photosensitive retinal ganglion cells (ipRGCs, Opn4+) that form the RHT, abolishes circadian photoentrainment (Güler et al., 2008), suggesting that ipRGCs are essential for photoentrainment. The major neurotransmitters are thought to be glutamate and PACAP (Hannibal, 2002, 2021), and the glutamatergic input is mediated by AMPA and NMDA receptors (Kim and Dudek, 1991).
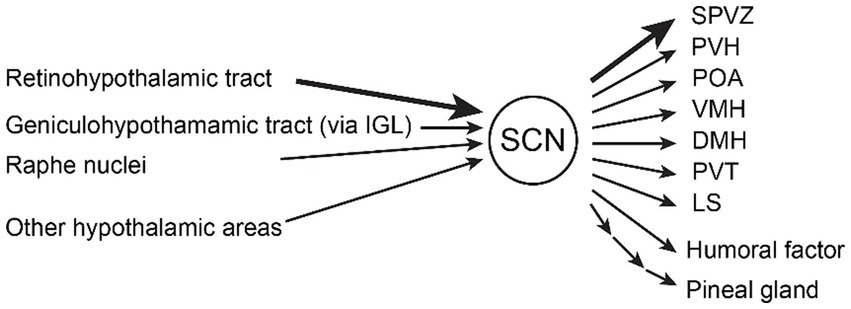
Figure 1. Inputs and outputs of the suprachiasmatic nucleus (SCN). The major inputs to the SCN are (1) the retinohypothalamic tract from melanopsin-containing photosensitive ganglion cells in the retina, (2) the geniculohypothalamic tract via the intergeniculate leaflet (IGL), and (3) the median raphe nuclei containing serotonergic neurons. Other hypothalamic areas (arcuate nucleus, medial preoptic area) and thalamic areas (paraventricular thalamic nuclei) also project to the SCN. Humoral factors such as hormones also modulate the SCN. The major outputs of the SCN are (1) subparaventricular zone (SPVZ), (2) paraventricular hypothalamic nucleus (PVH), (3) preoptic area (POA), (4) ventromedial hypothalamic nucleus (VMH), (5) dorsomedial hypothalamic nucleus (DMH), (6) paraventricular thalamic nuclei (PVT), and (7) lateral septum (LS). Other outputs include humoral factor and pineal gland polysynaptically (Abrahamson and Moore, 2001; Saper et al., 2005; Starnes and Jones, 2023).
The second is the geniculohypothalamic input via the thalamic intergeniculate leaflet, which also originates from the retina. The third is serotonergic input from the raphe nuclei, which enters the ventral region of the SCN (Abrahamson and Moore, 2001). Serotonin receptors (5-HT1A, 2A, 2B, 2C, 5A, 7) are expressed in the SCN (Moyer and Kennaway, 1999; Takeuchi et al., 2014). Other inputs come from other hypothalamic areas that can modulate the activity of the SCN. The circadian rhythm of the SCN is thought to be altered by sleep, wakefulness, animal state, and external conditions but either the pathway and mechanism are unknown (Deboer et al., 2003, 2007).
Inside the SCN
The two of the major neuronal subtypes in the SCN are VIP-positive neurons in the ventrolateral region, also known as the core region, and AVP-positive neurons in the dorsomedial, or shell, region (Abrahamson and Moore, 2001). VIP-positive neurons receive direct projection from the retina (Todd et al., 2020) and are thought to shift the phases of the circadian rhythm. Spontaneous Ca2+ activity of VIP neurons is higher during daytime and subjective daytime (Jones et al., 2018). VIP neuron intracellular Ca2+ is increased by light stimulation of the retina (Jones et al., 2018; Todd et al., 2020). Suppression of VIP neurons abolished the phase shift induced by light pulses (Jones et al., 2018). Diphtheria toxin ablation of VIP neurons disrupted the light-entrained rhythm of locomotor activity (Todd et al., 2020). All of these results suggest that SCN VIP neurons play an important role in light entrainment. In addition, because VIP or VIP receptor, VPAC2, knock-out mice show either arrhythmicity, multiple circadian periods, or a shorter single period in locomotor activity (home-cage activity) or wheel-running activity (Harmar et al., 2002; Colwell et al., 2003; Hughes et al., 2004; Aton et al., 2005; Peng et al., 2022), the VIP peptide itself is important for synchronizing circadian rhythmicity within the SCN and animal behavior.
AVP-positive neurons are important in determining the period of the circadian rhythm. Knockout of the clock gene Bmal1 specifically in AVP neurons impaired the behavioral circadian rhythm (Mieda et al., 2015). The clock proteins CLOCK (circadian locomotor output cycles kaput) and BMAL1 (basic helix–loop–helix ARNT-like protein 1 or ARNTL, aryl hydrocarbon receptor nuclear translocator-like protein 1) are key transcription factors that form a heteromer and bind regulatory elements E-boxes to express the clock proteins PER1, PER2, CRY1, CRY2, which in turn inhibit CLOCK-BMAL1, generating the approximately 24-h rhythm (Takahashi, 2017). This machinery is called the transcriptional translational feedback loop (TTFL), which is the core machinery of the molecular clock. Therefore, knocking out Bmal1 in AVP neurons disrupts the cellular clock of AVP neurons.
Casein kinase 1 delta (CK1δ) regulates the nuclear translocation and degradation of the clock proteins PER and CRY. Therefore, the loss of CK1δ elongates the cellular circadian period (Etchegaray et al., 2009, 2010). AVP neuron-specific knockout of CK1δ prolonged the period of the behavioral circadian rhythm (Mieda et al., 2016). In addition, knockout of CK1δ specifically in AVP neurons prolonged the period of the Ca2+ activity circadian rhythm in both AVP neurons and VIP neurons (Tsuno et al., 2023). This suggests that AVP neurons regulate the period of the circadian rhythm of the entire SCN. However, since the period of the circadian rhythm was not altered by loss of GABA release (Maejima et al., 2021) or AVP release (Tsuno et al., 2023) specifically in AVP neurons, it is likely that neurotransmitters other than GABA and AVP are responsible for the period regulation of the SCN ensemble circadian rhythm and locomotion by AVP neurons, and further studies are needed to identify the mechanism.
In addition to AVP neurons and VIP neurons, the SCN contains neurons that express a variety of neuropeptides. For example, CCK-positive neurons in mice have a peak in Ca2+ activity 5 h before the onset of locomotor activity under different light/dark conditions, suggesting that they may determine the onset of locomotion (Xie et al., 2023). Prok2-positive neurons have a peak in Ca2+ activity in the middle of the day (Onodera et al., 2023), which may inhibit locomotor output (Cheng et al., 2002). Abrahamson and Moore showed that ventral core neurons contain GABA, VIP, gastrin-releasing peptide (GRP), neurotensin (NT), calretinin (CALR), and calbindin (CALB), whereas the dorsal shell neurons contain GABA, AVP, met-enkephalin (mENK), angiotensin II (AII), and CALB (Abrahamson and Moore, 2001). Recent single-cell transcriptomic analyses have shown that the SCN neurons can be classified into 5 (Park et al., 2016; Wen et al., 2020), 11 (Morris et al., 2021) or 12 subtypes (Xu et al., 2021). Park et al. (2016) classified 5 groups of neurons, VIP+, AVP+/VIPR2+, PACAP+/Prok2+, PACAPR+, and others. Wen et al. (2020) classified 5 groups, Vip+/Nms+, Avp+/Nms+, Cck+/C1ql3+, Cck+/Bdnf+, and Grp+/Vip+. Morris et al. (2021) classified 11 neuronal subpopulations, 8 groups in day and 8 groups in night including 5 overlapping groups. Xu et al. (2021) classified 12 groups, 4 AVP+, 3 VIP+, 2 CCK+, and 3 unknown groups. One of 4 AVP+ groups by Xu et al. strongly correlated with the Cck+/C1ql3+ group by Wen et al., while another 3 of 4 AVP+ groups significantly correlated with the Avp+/Nms + group. In addition, 1 of 3 VIP+ groups by Xu et al. correlated with the Vip+/Nms + group by Wen et al., while another 1 VIP+ group correlated with the Grp+/Vip + group. Finally, 1 of 2 CCK+ groups correlated with the Cck+/Bdnf+ group (Wen et al., 2020; Xu et al., 2021). Further studies are needed to elucidate the function of each cell type and the interactions between them.
Output from the SCN
Output from the SCN is primarily to other hypothalamic nuclei and the thalamus (Abrahamson and Moore, 2001; Saper et al., 2005; Reghunandanan and Reghunandanan, 2006; Morin, 2013; Starnes and Jones, 2023). Many projections from the SCN terminate in the subparaventricular zone (SPVZ, projecting DMH), adjacent to the dorsal SCN. Other major projections include the paraventricular hypothalamic nucleus (PVH, including the pituitary–adrenal axis), the preoptic area [POA, a critical area of thermoregulation (Nakamura et al., 2022) and one of non-REM sleep centers (Scammell et al., 2017)], and other hypothalamic nuclei that control instinctive behavior and homeostasis such as feeding, energy metabolism, sleep/wakefulness, and motivated behavior, including the ventromedial hypothalamic nucleus (VMH, projecting SPVZ), dorsomedial hypothalamic nucleus (DMH), paraventricular thalamic nuclei (PVT), and lateral septum (LS). The SCN inhibits the pineal gland, which produces melatonin with sleep-inducing properties, via the PVH, the intermediolateral nucleus of the medulla (IML), and the cervical sympathetic ganglion (Tonon et al., 2021).
Olfaction and circadian rhythms
There are reports suggesting a relationship between the olfactory system and circadian rhythms. Almost every cell in the body can generate circadian rhythms, with the SCN acting as a master pacemaker to synchronize and entrain the peripheral clock (Reppert and Weaver, 2002; Takahashi, 2017). The olfactory bulb (OB), the first center of olfactory information processing, is thought to be capable of generating circadian rhythms. In slices, the OB maintains a circadian rhythm of Per1 expression, recorded by the Per1-Luc reporter, and neuronal firing activity in rats (Granados-Fuentes et al., 2004) or Per2 expression by the Per2::Luc reporter in mice (Ono et al., 2015), suggesting that the OB can generate its own circadian rhythm. However, the phase of the rhythm is easily altered by external perturbations, as the peak of Per2::Luc varies with the cutting time (Ono et al., 2015). Furthermore, the SCN lesions alter (Abraham et al., 2005) or eliminate (Ono et al., 2015) the peak phase of clock gene expression in the OB. These results suggest that the OB expresses clock genes and can generate circadian rhythms. However, without the SCN, the peaks become desynchronized with the whole body, indicating a hierarchical structure with the SCN as the central clock.
Some reports suggest that the daily rhythm of the olfactory function is influenced by both the SCN and the OB. Granados-Fuentes et al. reported in 2006 that the cedar oil-induced daily rhythm of c-Fos expression remained in the OB and piriform cortex after SCN removal, whereas it disappeared in the piriform cortex after OB ablation, suggesting that the OB generates the rhythm. Granados-Fuentes et al. (2011) reported that the SCN lesion altered the peak phases of the daily rhythm of olfactory discrimination performance (Granados-Fuentes et al., 2011). In addition, Takeuchi et al. reported in 2023 that knockout of Bmal1 in Vgat-positive neurons, including SCN neurons, altered the peak phase of the circadian rhythm of odor-induced c-fos positive cell number in the piriform cortex (Takeuchi et al., 2023). These results indicate that the SCN alters the peak phase of olfactory function, suggesting that it contributes to the synchronization of the circadian rhythms of olfactory function and the central clock.
Clock gene expression itself is important for odor detection. Granados-Fuentes et al. (2011) reported that there is a daily rhythm in the performance and sensitivity of the odor detection task that disappears when the clock genes Bmal1 or both Per1 and Per2 genes are knocked out (Granados-Fuentes et al., 2011). Takeuchi et al. report that Bmal1 knockout in the piriform cortex abolishes the circadian rhythm of the odor-evoked activity (Takeuchi et al., 2023). Using quantitative PCR, they report that the circadian rhythm of gene expression observed in the piriform cortex is dependent on Bmal1 expression in Emx-positive cells, including pyramidal cells in the piriform cortex (Takeuchi et al., 2023). These results suggest that the circadian rhythm of odor detection and odor-evoked neural activity requires clock genes.
The mechanism by which the SCN modulates the circadian rhythm of the OB is not yet clear. It is known that the SCN projects to various hypothalamic regions and releases neurotransmitters, including neuropeptides, and hormones, while the OB receives centrifugal inputs from the olfactory cortex and neuromodulatory centers and is also influenced by neuropeptides and hormones (Brunert and Rothermel, 2021). Therefore, it is possible that the SCN can alter the circadian rhythm of the OB through centrifugal inputs from the olfactory cortex or neuromodulatory centers via the hypothalamus, neuromodulatory inputs, and neuropeptides, or hormones in the bloodstream.
Conversely, there is some evidence that the olfactory system can modify the activity of the SCN via the OB. Amir et al. (1999) reported that light-induced c-Fos expression in the SCN is enhanced by olfactory stimuli. Removal of the OB also altered the time of re-entrainment and altered the phase of the odor-evoked c-Fos expression rhythm in the SCN (Granados-Fuentes et al., 2006). Because odor stimulation increased c-Fos expression in the paraventricular thalamic nucleus (PVT) (Amir et al., 1999), a review has argued that the PVT may be related to the pathway from the OB to the SCN (Jeffs et al., 2023).
Discussion
Although the basic mechanisms of circadian rhythm control are becoming clearer, much remains unknown about how the individual subpopulations within the SCN interact to generate a synchronized circadian rhythm as an ensemble. Furthermore, although circadian rhythms are closely linked to the sleep–wake cycle (Franken and Dijk, 2024), the interaction between the SCN and the sleep–wake center remains elusive. Although the modulation of circadian rhythms by light stimulation has been well studied, the modulation of circadian rhythms by environmental changes other than light has not been fully investigated, and further research is needed.
The SCN, the center of the circadian rhythm, modulates the rhythm in response to external conditions. In addition to light, possible conditions include food availability, the presence of predators, and environmental temperature, which in turn affect the wake–sleep states and the brain’s information processing mode (Pickel and Sung, 2020; Franken and Dijk, 2024). From this perspective, the olfactory system, which is important for feeding, finding mates, and escaping predators, is thought to be closely related to circadian rhythms and wake–sleep states (Mori and Sakano, 2022). Because the OB has the unique property of generating a circadian rhythm without the SCN, investigating the relationship between diurnal variation in olfactory information processing and circadian rhythms of the SCN is an interesting target for future research. In addition, a reduced sense of smell and instability of the wake–sleep rhythm are two prominent features of Alzheimer’s disease (AD), so both may be easily impaired and may be an indicator of preclinical AD (Jeffs et al., 2023).
Author contributions
YT: Conceptualization, Funding acquisition, Writing – original draft, Writing – review & editing. MM: Conceptualization, Funding acquisition, Writing – original draft, Writing – review & editing.
Funding
The author(s) declare financial support was received for the research, authorship, and/or publication of this article. This work was supported in part by JSPS KAKENHI Grant Numbers JP23K06345 (YT); JP22H02802 (MM).
Conflict of interest
The authors declare that the research was conducted in the absence of any commercial or financial relationships that could be construed as a potential conflict of interest.
The author(s) declared that they were an editorial board member of Frontiers, at the time of submission. This had no impact on the peer review process and the final decision.
Publisher’s note
All claims expressed in this article are solely those of the authors and do not necessarily represent those of their affiliated organizations, or those of the publisher, the editors and the reviewers. Any product that may be evaluated in this article, or claim that may be made by its manufacturer, is not guaranteed or endorsed by the publisher.
References
Abraham, U., Prior, J. L., Granados-Fuentes, D., Piwnica-Worms, D. R., and Herzog, E. D. (2005). Independent circadian oscillations of Period1 in specific brain areas in vivo and in vitro. J. Neurosci. 25, 8620–8626. doi: 10.1523/JNEUROSCI.2225-05.2005
Abrahamson, E. E., and Moore, R. Y. (2001). Suprachiasmatic nucleus in the mouse: retinal innervation, intrinsic organization and efferent projections. Brain Res. 916, 172–191. doi: 10.1016/s0006-8993(01)02890-6
Amir, S., Cain, S., Sullivan, J., Robinson, B., and Stewart, J. (1999). In rats, odor-induced Fos in the olfactory pathways depends on the phase of the circadian clock. Neurosci. Lett. 272, 175–178. doi: 10.1016/S0304-3940(99)00609-6
Aton, S. J., Colwell, C. S., Harmar, A. J., Waschek, J., and Herzog, E. D. (2005). Vasoactive intestinal polypeptide mediates circadian rhythmicity and synchrony in mammalian clock neurons. Nat. Neurosci. 8, 476–483. doi: 10.1038/nn1419
Brunert, D., and Rothermel, M. (2021). Extrinsic neuromodulation in the rodent olfactory bulb. Cell Tissue Res. 383, 507–524. doi: 10.1007/s00441-020-03365-9
Cheng, M. Y., Bullock, C. M., Li, C., Lee, A. G., Bermak, J. C., Belluzzi, J., et al. (2002). Prokineticin 2 transmits the behavioural circadian rhythm of the suprachiasmatic nucleus. Nature 417, 405–410. doi: 10.1038/417405a
Colwell, C. S., Michel, S., Itri, J., Rodriguez, W., Tam, J., Lelievre, V., et al. (2003). Disrupted circadian rhythms in VIP- and PHI-deficient mice. Am. J. Physiol. Regul. Integr. Comp. Physiol. 285, R939–R949. doi: 10.1152/ajpregu.00200.2003
Deboer, T., Détári, L., and Meijer, J. H. (2007). Long term effects of sleep deprivation on the mammalian circadian pacemaker. Sleep 30, 257–262. doi: 10.1093/sleep/30.3.257
Deboer, T., Vansteensel, M. J., Détári, L., and Meijer, J. H. (2003). Sleep states alter activity of suprachiasmatic nucleus neurons. Nat. Neurosci. 6, 1086–1090. doi: 10.1038/nn1122
Etchegaray, J.-P., Machida, K. K., Noton, E., Constance, C. M., Dallmann, R., Di Napoli, M. N., et al. (2009). Casein kinase 1 Delta regulates the pace of the mammalian circadian clock. Mol. Cell. Biol. 29, 3853–3866. doi: 10.1128/mcb.00338-09
Etchegaray, J. P., Yu, E. A., Indic, P., Dallmann, R., and Weaver, D. R. (2010). Casein kinase 1 delta (CK1) regulates period length of the mouse suprachiasmatic circadian clock in vitro. PLoS One 5:e10303. doi: 10.1371/journal.pone.0010303
Franken, P., and Dijk, D. J. (2024). Sleep and circadian rhythmicity as entangled processes serving homeostasis. Nat. Rev. Neurosci. 25, 43–59. doi: 10.1038/s41583-023-00764-z
Granados-Fuentes, D., Ben-Josef, G., Perry, G., Wilson, D. A., Sullivan-Wilson, A., and Herzog, E. D. (2011). Daily rhythms in olfactory discrimination depend on clock genes but not the suprachiasmatic nucleus. J. Biol. Rhythm. 26, 552–560. doi: 10.1177/0748730411420247
Granados-Fuentes, D., Saxena, M. T., Prolo, L. M., Aton, S. J., and Herzog, E. D. (2004). Olfactory bulb neurons express functional, entrainable circadian rhythms. Eur. J. Neurosci. 19, 898–906. doi: 10.1111/j.1460-9568.2004.03117.x
Granados-Fuentes, D., Tseng, A., and Herzog, E. D. (2006). A circadian clock in the olfactory bulb controls olfactory responsivity. J. Neurosci. 26, 12219–12225. doi: 10.1523/JNEUROSCI.3445-06.2006
Güler, A. D., Ecker, J. L., Lall, G. S., Haq, S., Altimus, C. M., Liao, H.-W., et al. (2008). Melanopsin cells are the principal conduits for rod–cone input to non-image-forming vision. Nature 453, 102–105. doi: 10.1038/nature06829
Hannibal, J. (2002). Neurotransmitters of the retino-hypothalamic tract. Cell Tissue Res. 309, 73–88. doi: 10.1007/s00441-002-0574-3
Hannibal, J. (2021). Comparative neurology of circadian photoreception: the retinohypothalamic tract (RHT) in sighted and naturally blind mammals. Front. Neurosci. 15:640113. doi: 10.3389/fnins.2021.640113
Harmar, A. J., Marston, H. M., Shen, S., Spratt, C., West, K. M., Sheward, W. J., et al. (2002). The VPAC(2) receptor is essential for circadian function in the mouse suprachiasmatic nuclei. Cell 109, 497–508. doi: 10.1016/s0092-8674(02)00736-5
Hastings, M. H., Maywood, E. S., and Brancaccio, M. (2018). Generation of circadian rhythms in the suprachiasmatic nucleus. Nat. Rev. Neurosci. 19, 453–469. doi: 10.1038/s41583-018-0026-z
Hughes, A. T., Fahey, B., Cutler, D. J., Coogan, A. N., and Piggins, H. D. (2004). Aberrant gating of photic input to the suprachiasmatic circadian pacemaker of mice lacking the VPAC 2 receptor. J. Neurosci. 24, 3522–3526. doi: 10.1523/JNEUROSCI.5345-03.2004
Jeffs, Q. L., Prather, J. F., and Todd, W. D. (2023). Potential neural substrates underlying circadian and olfactory disruptions in preclinical Alzheimer’s disease. Front. Neurosci. 17:1295998. doi: 10.3389/fnins.2023.1295998
Jones, J. R., Simon, T., Lones, L., and Herzog, E. D. (2018). SCN VIP neurons are essential for normal light-mediated resetting of the circadian system. J. Neurosci. 38, 7986–7995. doi: 10.1523/JNEUROSCI.1322-18.2018
Kim, Y. I., and Dudek, F. E. (1991). Intracellular electrophysiological study of suprachiasmatic nucleus neurons in rodents: excitatory synaptic mechanisms. J. Physiol. 444, 269–287. doi: 10.1113/jphysiol.1991.sp018877
Lokshin, M., LeSauter, J., and Silver, R. (2015). Selective distribution of retinal input to mouse SCN revealed in analysis of sagittal sections. J. Biol. Rhythm. 30, 251–257. doi: 10.1177/0748730415584058
Maejima, T., Tsuno, Y., Miyazaki, S., Tsuneoka, Y., Hasegawa, E., Islam, M. T., et al. (2021). GABA from vasopressin neurons regulates the time at which suprachiasmatic nucleus molecular clocks enable circadian behavior. Proc. Natl. Acad. Sci. 118:e2010168118. doi: 10.1073/pnas.2010168118
Mieda, M., Okamoto, H., and Sakurai, T. (2016). Manipulating the cellular circadian period of arginine vasopressin neurons alters the behavioral circadian period. Curr. Biol. 26, 2535–2542. doi: 10.1016/j.cub.2016.07.022
Mieda, M., Ono, D., Hasegawa, E., Okamoto, H., Honma, K., Ichi Honma, S., et al. (2015). Cellular clocks in AVP neurons of the SCN are critical for interneuronal coupling regulating circadian behavior rhythm. Neuron 85, 1103–1116. doi: 10.1016/j.neuron.2015.02.005
Mori, K., and Sakano, H. (2022). Neural circuitry for stress information of environmental and internal odor worlds. Front. Behav. Neurosci. 16:943647. doi: 10.3389/fnbeh.2022.943647
Morin, L. P. (2013). Neuroanatomy of the extended circadian rhythm system. Exp. Neurol. 243, 4–20. doi: 10.1016/j.expneurol.2012.06.026
Morris, E. L., Patton, A. P., Chesham, J. E., Crisp, A., Adamson, A., and Hastings, M. H. (2021). Single-cell transcriptomics of suprachiasmatic nuclei reveal a Prokineticin-driven circadian network. EMBO J. 40, e108614–e108623. doi: 10.15252/embj.2021108614
Moyer, R. W., and Kennaway, D. J. (1999). Immunohistochemical localization of serotonin receptors in the rat suprachiasmatic nucleus. Neurosci. Lett. 271, 147–150. doi: 10.1016/S0304-3940(99)00536-4
Nakamura, K., Nakamura, Y., and Kataoka, N. (2022). A hypothalamomedullary network for physiological responses to environmental stresses. Nat. Rev. Neurosci. 23, 35–52. doi: 10.1038/s41583-021-00532-x
Ono, D., Honma, S., and Honma, K. ichi (2015). Circadian PER2: LUC rhythms in the olfactory bulb of freely moving mice depend on the suprachiasmatic nucleus but not on behaviour rhythms. Eur. J. Neurosci. 42, 3128–3137. doi: 10.1111/ejn.13111
Onodera, K., Tsuno, Y., Hiraoka, Y., Tanaka, K., Maejima, T., and Mieda, M. (2023). In vivo recording of the circadian calcium rhythm in Prokineticin 2 neurons of the suprachiasmatic nucleus. Sci. Rep. 13:16974. doi: 10.1038/s41598-023-44282-5
Park, J., Zhu, H., O’Sullivan, S., Ogunnaike, B. A., Weaver, D. R., Schwaber, J. S., et al. (2016). Single-cell transcriptional analysis reveals novel neuronal phenotypes and interaction networks involved in the central circadian clock. Front. Neurosci. 10:481. doi: 10.3389/fnins.2016.00481
Peng, Y., Tsuno, Y., Matsui, A., Hiraoka, Y., Tanaka, K., Horike, S., et al. (2022). Cell type-specific genetic manipulation and impaired circadian rhythms in Vip tTA Knock-in mice. Front. Physiol. 13:895633. doi: 10.3389/fphys.2022.895633
Pickel, L., and Sung, H.-K. (2020). Feeding rhythms and the circadian regulation of metabolism. Front. Nutr. 7:39. doi: 10.3389/fnut.2020.00039
Reghunandanan, V., and Reghunandanan, R. (2006). Neurotransmitters of the suprachiasmatic nuclei. J. Circadian Rhythms 4:2. doi: 10.1186/1740-3391-4-2
Reppert, S. M., and Weaver, D. R. (2002). Coordination of circadian timing in mammals. Nature 418, 935–941. doi: 10.1038/nature00965
Saper, C. B., Scammell, T. E., and Lu, J. (2005). Hypothalamic regulation of sleep and circadian rhythms. Nature 437, 1257–1263. doi: 10.1038/nature04284
Scammell, T. E., Arrigoni, E., and Lipton, J. O. (2017). Neural circuitry of wakefulness and sleep. Neuron 93, 747–765. doi: 10.1016/j.neuron.2017.01.014
Starnes, A. N., and Jones, J. R. (2023). Inputs and outputs of the mammalian circadian clock. Biology (Basel) 12:508. doi: 10.3390/biology12040508
Takahashi, J. S. (2017). Transcriptional architecture of the mammalian circadian clock. Nat. Rev. Genet. 18, 164–179. doi: 10.1038/nrg.2016.150
Takeuchi, K., Mohammad, S., Ozaki, T., Morioka, E., Kawaguchi, K., Kim, J., et al. (2014). Serotonin-2C receptor involved serotonin-induced ca 2+ mobilisations in neuronal progenitors and neurons in rat suprachiasmatic nucleus. Sci. Rep. 4:4106. doi: 10.1038/srep04106
Takeuchi, S., Shimizu, K., Fukada, Y., and Emoto, K. (2023). The circadian clock in the piriform cortex intrinsically tunes daily changes of odor-evoked neural activity. Commun. Biol. 6:332. doi: 10.1038/s42003-023-04691-8
Todd, W. D., Venner, A., Anaclet, C., Broadhurst, R. Y., De Luca, R., Bandaru, S. S., et al. (2020). Suprachiasmatic VIP neurons are required for normal circadian rhythmicity and comprised of molecularly distinct subpopulations. Nat. Commun. 11:4410. doi: 10.1038/s41467-020-17197-2
Tonon, A. C., Pilz, L. K., Markus, R. P., Hidalgo, M. P., and Elisabetsky, E. (2021). Melatonin and depression: a translational perspective from animal models to clinical studies. Front. Psych. 12:638981. doi: 10.3389/fpsyt.2021.638981
Tsuno, Y., Peng, Y., Horike, S., Wang, M., Matsui, A., Yamagata, K., et al. (2023). In vivo recording of suprachiasmatic nucleus dynamics reveals a dominant role of arginine vasopressin neurons in circadian pacesetting. PLoS Biol. 21:e3002281. doi: 10.1371/journal.pbio.3002281
Wen, S., Ma, D., Zhao, M., Xie, L., Wu, Q., Gou, L., et al. (2020). Spatiotemporal single-cell analysis of gene expression in the mouse suprachiasmatic nucleus. Nat. Neurosci. 23, 456–467. doi: 10.1038/s41593-020-0586-x
Xie, L., Xiong, Y., Ma, D., Shi, K., Chen, J., Yang, Q., et al. (2023). Cholecystokinin neurons in mouse suprachiasmatic nucleus regulate the robustness of circadian clock. Neuron 111, 2201–2217.e4. doi: 10.1016/j.neuron.2023.04.016
Keywords: circadian rhythm, suprachiasmatic nucleus, in vivo, olfaction, arginine vasopressin (AVP)
Citation: Tsuno Y and Mieda M (2024) Circadian rhythm mechanism in the suprachiasmatic nucleus and its relation to the olfactory system. Front. Neural Circuits. 18:1385908. doi: 10.3389/fncir.2024.1385908
Edited by:
Kensaku Mori, RIKEN, JapanReviewed by:
Hideki Kashiwadani, Kagoshima University, JapanYoshimasa Koyama, Fukushima University, Japan
Copyright © 2024 Tsuno and Mieda. This is an open-access article distributed under the terms of the Creative Commons Attribution License (CC BY). The use, distribution or reproduction in other forums is permitted, provided the original author(s) and the copyright owner(s) are credited and that the original publication in this journal is cited, in accordance with accepted academic practice. No use, distribution or reproduction is permitted which does not comply with these terms.
*Correspondence: Yusuke Tsuno, dHN1bm9AbWVkLmthbmF6YXdhLXUuYWMuanA=