- 1Department of Biology, McGill University, Montreal, QC, Canada
- 2Integrated Program in Neuroscience, McGill University, Montreal, QC, Canada
- 3Laboratory of Behavioral Neuroendocrinology, GIGA Neurosciences, University of Liege, Liege, Belgium
- 4Centre for Research in Brain, Language and Music, McGill University, Montreal, QC, Canada
Parvalbumin (PV) neurons play an integral role in regulating neural dynamics and plasticity. Therefore, understanding the factors that regulate PV expression is important for revealing modulators of brain function. While the contribution of PV neurons to neural processes has been studied in mammals, relatively little is known about PV function in non-mammalian species, and discerning similarities in the regulation of PV across species can provide insight into evolutionary conservation in the role of PV neurons. Here we investigated factors that affect the abundance of PV in PV neurons in sensory and motor circuits of songbirds and rodents. In particular, we examined the degree to which perineuronal nets (PNNs), extracellular matrices that preferentially surround PV neurons, modulate PV abundance as well as how the relationship between PV and PNN expression differs across brain areas and species and changes over development. We generally found that cortical PV neurons that are surrounded by PNNs (PV+PNN neurons) are more enriched with PV than PV neurons without PNNs (PV-PNN neurons) across both rodents and songbirds. Interestingly, the relationship between PV and PNN expression in the vocal portion of the basal ganglia of songbirds (Area X) differed from that in other areas, with PV+PNN neurons having lower PV expression compared to PV-PNN neurons. These relationships remained consistent across development in vocal motor circuits of the songbird brain. Finally, we discovered a causal contribution of PNNs to PV expression in songbirds because degradation of PNNs led to a diminution of PV expression in PV neurons. These findings reveal a conserved relationship between PV and PNN expression in sensory and motor cortices and across songbirds and rodents and suggest that PV neurons could modulate plasticity and neural dynamics in similar ways across songbirds and rodents.
Introduction
Parvalbumin (PV) neurons play a central role in regulating neural plasticity and in shaping neural dynamics. For example, the emergence of PV neurons in the visual cortex of rodents coincides with the onset of the critical period for visual plasticity (del Río et al., 1994; Sugiyama et al., 2008; Takesian and Hensch, 2013; Cisneros-Franco et al., 2020), and manipulations of PV expression affect plasticity and the timing of critical periods for sensory and cognitive systems (Murray et al., 2015; Wöhr et al., 2015; Hou et al., 2017; Xia et al., 2017; Cisneros-Franco and de Villers-Sidani, 2019; Deng et al., 2019; Miranda et al., 2022). Furthermore, manipulations of PV neuron activity affects neural dynamics across various brain systems (e.g., Spiro et al., 1999; Cardin et al., 2009; Sohal et al., 2009; Pyka et al., 2011; Albéri et al., 2013; Liu et al., 2013; Hu et al., 2014; Xue et al., 2014; Wöhr et al., 2015; Xia et al., 2017; Carceller et al., 2020), and PV neuron dysfunction can lead to cognitive and behavioral dysfunctions (Cardin et al., 2009; Sohal et al., 2009; Hu et al., 2014; Wöhr et al., 2015; Enwright et al., 2016; Favuzzi et al., 2017; Xiang et al., 2022). Therefore, it is important to reveal the factors that influence PV expression.
Developmental experiences and the expression of various molecular and cellular markers are known to affect the abundance of PV within PV neurons (i.e., PV intensity). For example, as PV neurons in the visual cortex mature and accumulate more of the orthodenticle homeobox protein Otx2, PV neurons become more enriched with PV, and manipulations that revert PV neurons to an immature and plastic state (e.g., inhibition of Otx2 expression) decrease PV intensity (Sugiyama et al., 2008; Beurdeley et al., 2012; Spatazza et al., 2013). Further, PV neurons are preferentially surrounded by perineuronal nets (PNN), and PNN ensheathment affects not only the excitability and plasticity of PV neurons but also the intensity of PV expression within PV neurons (Hensch et al., 1998; Pizzorusso et al., 2002; Dityatev et al., 2007; Carulli et al., 2010; Beurdeley et al., 2012; Wang and Fawcett, 2012; Takesian and Hensch, 2013; Yamada et al., 2015; Hou et al., 2017; Rowlands et al., 2018; Gogola et al., 2019; Shi et al., 2019; Carceller et al., 2020; Carulli et al., 2020). Changes in PV intensity are informative because PV intensity can serve as a proxy for the excitability, activity, and state of PV neurons (e.g., Kinney et al., 2006; Favuzzi et al., 2017).
To date, most of our understanding of factors that affect PV expression stems from studies in mammals, in particular rodents. Given that important forms of plasticity shape the behavior and cognition of many non-mammalian species (e.g., critical periods for vocal learning and individual recognition in birds: Brainard and Doupe, 2002, 2013; Horn, 2004; Gobes et al., 2019; Sakata and Woolley, 2020), it is important to assess the role of PV neurons in shaping these forms of plasticity (e.g., Hara et al., 2012; Sakata and Woolley, 2022). Previous research indicates that brain areas important for vocal learning and performance in songbirds are replete with PV neurons (Braun et al., 1985, 1991; Wild et al., 2001, 2005; Balmer et al., 2009; Cornez et al., 2015, 2017, 2018, 2020a,b; Olson et al., 2015), and it has been proposed that PV interneurons provide strategic inhibition to coordinate the motor outputs necessary for singing (Spiro et al., 1999). Brain areas active during the performance of non-vocal communicative displays (e.g., drumming in woodpeckers) also express a high number of PV neurons (Schuppe et al., 2022). Collectively, these data motivate research into the factors that affect PV expression in brain circuits in non-mammalian vertebrates.
Here we analyzed the effects of PNN ensheathment on the intensity of PV expression within PV neurons in auditory and motor areas of songbirds and rodents, as well as how the effect of PNN ensheathment on PV intensity could vary across development in songbirds. We focused our attention in songbirds on the song system - a collection of interconnected forebrain areas that regulate vocal performance and learning and that consist of the forebrain areas HVC (acronym used as a proper noun), the robust nucleus of the arcopallium (RA), the lateral magnocellular nucleus of the anterior nidopallium (LMAN), and Area X (the vocal portion of the avian basal ganglia) – as well as the primary auditory cortex (Field L). We measured PV expression in the motor and auditory cortices, dorsolateral striatum (DLS), and external nucleus of the globus pallidus (GPe) in rodents because many brain areas within the song and auditory systems of songbirds are analogous to these areas (Leblois and Perkel, 2020; Sakata and Yazaki-Sugiyama, 2020; Woolley and Woolley, 2020; Colquitt et al., 2021). Finally, we assessed the causal contribution of PNNs to PV intensity in songbirds by analyzing how degradation of PNNs in HVC affects the intensity of PV within PV neurons.
Materials and methods
Animals
Thirty-one normally reared (i.e., with mother and father) zebra finches [n = 7 juvenile males: 50–70 days post-hatch (dph), n = 24 adult males: 0.3–3.5 years old] were raised in our colony. Six adults were used for an initial investigation of the relationship between PNN ensheathment and PV intensity; 12 individuals were used in an analysis of age on PV intensity (n = 7 juveniles and n = 5 adults); and 13 adults were used to analyze the effects of PNN degradation on PV intensity. All birds were housed on a 14:10 light–dark cycle with food and water provided ad libitum. In addition, we analyzed the effect of PNN ensheathment on PV intensity in n = 7 adult male mice (22–27 months) that were hemizygous or wildtype from strain B6.Cg-Tg (Pcp2-cre)3555Jdhu/J; stock number: 010536 (Jackson Laboratory). Hemizygous mice express Cre under the L7 promoter, which should not alter brain development or function. All procedures were approved by the McGill University Animal Care and Use Committee in accordance with the guidelines of the Canadian Council on Animal Care.
Surgical procedures
To experimentally assess how PNNs contribute to PV abundance in sensorimotor structures, we examined how degradation of PNNs in the sensorimotor structure HVC affected the intensity of PV in PV neurons. We selected HVC for this experiment because of the consistent relationship between PV intensity and PNN ensheathment (see Results) and because HVC is necessary for song learning and production (reviewed in Murphy et al., 2020; Sakata and Yazaki-Sugiyama, 2020). For surgery, birds were anesthetized with intramuscular injections of ketamine (0.03 mg/g) and midazolam (0.0015 mg/g) followed by vaporized isoflurane (0.2–3.0% in oxygen) to maintain a deep state of anesthesia throughout the surgical procedure. Birds were placed in a stereotaxic device, with their beaks stabilized at a 45° angle. Following a craniotomy, chondroitinase ABC (ChABC; Sigma C3667; 100 U/mL, in 0.1% BSA in PBS) or penicillinase (PEN; Sigma P038; 100 U/mL) was bilaterally injected into HVC (0.8 mm rostral from the caudal edge of the bifurcation of the midsagittal sinus, 1.8 mm lateral from the midline, and 0.5 mm in depth) using a Nanoject III Programmable Nanoliter Injector (Drummond Scientific, Broomall, PA) assembled with a glass pipette. ChABC is an enzyme that degrades chondrointin sulfate proteoglycans, a major structural component of PNNs, whereas PEN is often used as a control enzyme for experiments involving ChABC because it does not degrade PNNs (e.g., Pizzorusso et al., 2002; Yamada et al., 2015; Carceller et al., 2020). Drugs were infused at 10 nL/s with 50 or 100 nL per cycle and 1–3 cycles for each hemisphere, and the glass pipette was left in place for ~2 min before retraction. Brains of ChABC- or PEN-treated birds were collected 6–7 days after surgery.
Tissue collection
Zebra finches were deeply anesthetized with isoflurane vapor and transcardially perfused with heparinized saline (100 IU/100 mL) followed by 150 mL of 4% paraformaldehyde (PFA; pH 7.4). Brains were left to postfix overnight at 4°C then moved to 30% sucrose PBS solution for cryoprotection. Brains were cut on a freezing microtome (Leica Biosystems, Wetzlar, Germany) in 40 μm sagittal sections and collected in Tris-buffered saline (TBS) with sodium azide.
Mouse brains were collected in 4% PFA. Briefly, mice were deeply anaesthetized using 2,2,2-tribromoethanol (Avertin) injected intraperitoneally. PBS (0.1 M, pH 7.4) with heparin salt (5.6 μg/mL) was perfused transcardially, followed by 30 mL ice-cold 4% PFA in Phosphate Buffer (PB, pH 7.4). Brains were extracted and allowed to postfix in 4% PFA at 4°C for a further 24 h before being transferred for long-term storage at 4°C in PBS with 0.5% sodium azide. Brains were moved to a 30% sucrose PBS solution for cryoprotection before cutting. Coronal sections (40 μm) were cut on a freezing microtome and collected in PBS with sodium azide.
Immunohistochemistry (IHC)
One set of brain sections from one hemisphere of each bird was processed for both PNN and PV expression. Free-floating sections were washed 3X for 5 min in 1X TBS and then blocked for 30 min in TBS + 5% donkey serum +0.1% Triton-X. Then the tissue was incubated overnight at 4°C in a mouse monoclonal anti-chondroitin sulfate (ACS; C8035; Sigma-Aldrich; 1:500) and a rabbit polyclonal anti-PV (ab11427; Abcam; 1:2000). Thereafter, sections were washed 3X for 5 min in TBS followed by a 2-h incubation at room temperature with secondary antibodies [donkey anti-mouse Alexa Fluor 488: 1:100 (ThermoFisher); donkey anti-rabbit Alexa Fluor 594: 1:200 (ThermoFisher)] in TBS + 0.1% Triton-X. The tissue was then washed 3X for 5 min in TBS and transferred to TBS before mounting. Sections were coverslipped with Prolong Gold Antifade (Life Technologies, P36930).
Rat and mouse brains were processed in a similar manner as finch brains, albeit with different primary compounds (Cisneros-Franco et al., 2018). Briefly, rat brains were immunolabeled with an antibody against PV (#P-3088; Sigma-Aldrich; 1:10,000), and PNN were stained with fluorescein Wisteria floribunda lectin (WFL; #FL-1351; Vector Laboratories; 1:200). For mouse sections, PV neurons were stained using same PV antibody used in finches (ab11427; 1:500), while PNNs were stained using Wisteria floribunda lectin (Sigma L1516; 1:1000). Secondary antibodies for this staining included donkey anti-rabbit Alexa Fluor 594 (1:200) and Streptavidin conjugated to Alexa Fluor 488 (1:500: S11223; Life Technologies).
Different methods were used to visualize PNNs in zebra finches and rodents because Wisteria floribunda lectin (WFL; aka WFA), the most widely used tool to visualize PNNs in mammals, does not work in zebra finches (A.S. Wang, Y. Yazaki-Sugiyajma, & J.T. Sakata, unpublished data). However, both WFL and ACS bind to the glycosaminoglycan portion of chondroitin sulfate (CS) proteoglycans of PNNs, so we anticipate that the antibodies target similar types of PNNs. Similarly, just as ChABC injections reduce WFL staining in rodents, ChABC injections reduce ACS labeling in songbirds (Balmer et al., 2009; Cornez et al., 2021; this paper).
Imaging and analysis
We analyzed images of PV neurons and PNNs acquired in our lab as well as images obtained from previously published studies (Cisneros-Franco et al., 2018; Cornez et al., 2018). Images of HVC, RA, LMAN, Area X, and Field L in juvenile and adult male zebra finches from our lab were taken with a Zeiss Axio Imager.A2 microscope at 40X using the ZEN Imaging software (Carl Zeiss). These areas in the songbird brain are readily identifiable following staining for PV and PNNs (e.g., Balmer et al., 2009; Cornez et al., 2015; see also zebrafinchatlas.org).
Brain areas in the mouse brain were identified based on previous studies as well as the Allen Brain atlas. In the mouse brain, images of the motor cortex were taken around bregma −1.1 to −1.6 mm; for the external nucleus of the globus pallidus (GPe) around bregma −1.0 to −1.5 mm; for the dorsolateral striatum (DLS) around 0.8 to 1.3 mm; and for the primary auditory cortex around −2.0 to −3.3 mm. 40X images were taken for mouse motor and auditory cortices, and 20X images were taken for the DLS and GPe in mouse brains. 20X images were taken of the DLS because of its low density of PV neurons (a lower magnification enabled us to count more PV neurons), and we took images at the same magnification and exposure times for the GPe to enable comparisons of PV intensities between these parts of the basal ganglia (see Discussion). We imaged cortical layers with the highest density of PV neurons and PNNs (Alpár et al., 2006; Fader et al., 2016; Lupori et al., 2023); for the auditory cortex, PV neurons and PNNs in layer IV were imaged and quantified, and for the motor cortex, PV neurons and PNNs in layers II/III and V were separately imaged and quantified (Supplementary Figure 1). No layers are found in Field L or in brain areas surrounding the song circuitry in songbirds, and PV neurons and PNN expression were analyzed in parts of the brain areas that were representative of the brain area.
Exposure times were kept constant for all images within a given brain area and immunocytochemical batch. Exposure times were optimized for each brain area and were generally different for different brain areas; this allowed for maximal sensitivity to detect effects of age and PNNs on PV intensity. An exception was the mouse basal ganglia, wherein exposure times were the same for GPe and DLS neurons, allowing for quantification of regional variation in PV intensity. This was particularly useful to draw parallels to Area X, which consists of pallidal and striatal neurons (Carrillo and Doupe, 2004; Reiner et al., 2004; reviewed in Leblois and Perkel, 2020).
In addition to images acquired in our lab, images of PV neurons and PNNs within the auditory cortex of adult male Long-Evans rats (n=9; age: 2–25 months) were acquired from Cisneros-Franco et al. (2018) (bregma: −3.7 to −6.2 mm), and images of PV neurons and PNNs across various developmental ages in male zebra finches were acquired from Cornez et al. (2018). For the latter, we obtained and analyzed images of PV neurons and PNNs in HVC, RA, LMAN, and Area X of male zebra finches that were 40, 60, 90, and 120 dph (see Cornez et al., 2018 for details of methods).
Quantification of PV neurons and PNNs was conducted in Fiji, with each fluorophore imaged independently. The images were converted to grayscale (16-bit), and cells were manually counted by a single experimenter across 3–8 images per brain area per individual. The experimenter was blind to the experimental condition (e.g., age) of the animals. After identifying the PV neurons, PV intensity was measured; for this, the mean gray value of each individual cell identified was measured in a 30×30 pixel square placed in the center of the cell body. Background measurements were done in a similar fashion, measuring the mean gray value of a 30×30 pixel square in three randomly chosen locations across the image that did not contain cells. The average background intensity was computed per section and this value was subtracted from the raw PV intensities. From here on, “PV intensity” reflects this background-subtracted value and is reported in arbitrary units (a.u.).
Identification of neurons surrounded by PNNs was conducted independent of PV quantification. Images were converted to grayscale (16-bit), and neurons surrounded by PNNs were manually identified by a single experimenter across 3–8 images per brain area per animal. Neurons were identified as being surrounded by a PNN if the PNN surrounded >75% of the circumference of the cell body. After identifying neurons surrounded by PNNs, the experimenter compared the PV and PNN images and determined whether the PV neuron was or was not surrounded by PNNs (PV+PNN or PV-PNN, respectively). This allowed us to classify each PV intensity into a PV+PNN or PV-PNN category. Again, the experimenter was blind to experimental condition.
Statistical analyses
We used mixed-effects models to analyze how various parameters affected PV intensity. The specific independent variables included in the model depended on the experimental analysis but PNN ensheathment (PV+PNN vs. PV-PNN) was always an independent variable. When birds of different ages were analyzed, a full-factorial model with PNN ensheathment and age (juvenile vs. adult, days post-hatching) was used to analyze variation in PV intensity. For all models, animalID and sectionID nested within animalID were included as random effects because multiple PV neurons were measured per brain section and multiple sections were measured per animal. In situations in which multiple batches of IHCs were conducted (e.g., analysis of mouse sections as well as data from Cornez et al., 2018), batch was included as a random effect. In cases where significant interactions between PNN ensheathment and other variables were found, planned post-hoc contrasts were performed (see Results). JMP v16 (SAS, Cary, NC) were used for all analyses, with ɑ = 0.05 throughout.
Results
Modulation of PV intensity by PNNs in the primary auditory cortex of adult songbirds and rodents
In both rodents and songbirds, PV neurons are abundant in the primary auditory cortex, and many of these PV neurons are surrounded by PNNs. Field L is homologous to the primary auditory cortex of mammals (Woolley and Woolley, 2020), and PV neurons in Field L demonstrated variation in PV intensity depending on whether they were surrounded by PNNs or not. For example, in Figure 1A, there are two PV neurons surrounded by a PNN (PV+PNN; white arrows) and three PV neurons not surrounded by a PNN (PV-PNN; orange arrows), and PV appears more abundant in the two PV+PNN neurons compared to the three PV-PNN neurons. This variation was consistently observed, and across all PV neurons in Field L (n = 6 birds), PV intensity was significantly higher for PV+PNN neurons (F1,169.7 = 9.6, p = 0.0023; Figure 1B).
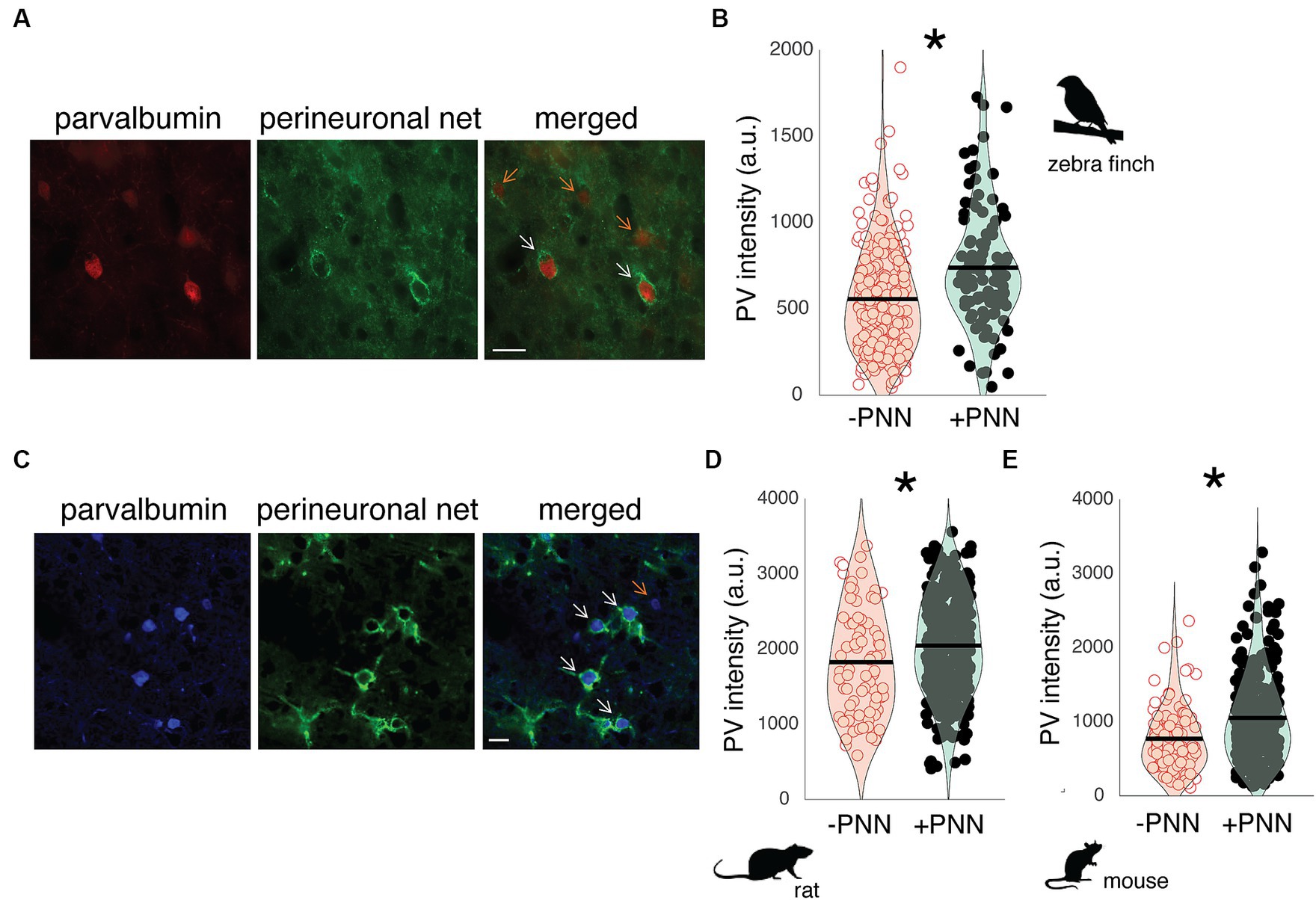
Figure 1. PV intensity of PV neurons and PNNs in the primary auditory cortices of zebra finches, rats, and mice. (A) Representative image of PV neurons (red) and PNNs (green) in Field L of adult zebra finches. White arrows point to PV neurons with PNNs (PV+PNN) while orange arrows point to PV neurons without PNNs (PV-PNN). Scale bar = 20 um; (B) PV intensity [in arbitrary units (a.u.)] is significantly higher for PV+PNN (filled circles) neurons than for PV-PNN neurons (empty circles) in Field L of adult zebra finches (n = 175 neurons in 6 finches; 13–45 months). (C) Representative image of PV neurons (blue) and PNNs (green) in the primary auditory cortex of adult rats. White arrows point to PV neurons with PNNs (PV+PNN) while the orange arrow points to a PV neuron without PNNs (PV-PNN). Scale bar = 20 um. PV intensity is significantly higher for PV+PNN (filled circles) neurons than for PV-PNN neurons (empty circles) in layer IV of the primary auditory cortices of (D) adult rats (n = 401 neurons in 9 rats; 5–25 months) and (E) mice (n = 341 neurons in 7 mice; 22–26 months). Each symbol in (B), (D), and (E) represents the PV intensity of a neuron. A violin plot summarizing the distribution is plotted on top of the raw data, with the black line representing the mean of the distribution. “*” denotes p < 0.05 (mixed-effects model).
PNNs are abundant within layer IV of the auditory cortex (Fader et al., 2016; Lupori et al., 2023), and just as in male zebra finches, the abundance of PV within PV neurons in the auditory cortex of male rats and mice seems to vary depending on PNN ensheathment. In Figure 1C, PV+PNN neurons (white arrows) in a male rat are more intense with PV than the PV-PNN neuron (orange arrow). Overall, PV intensity was significantly higher for PV+PNN neurons than for PV-PNN neurons in rats (n = 9 rats; F1,388.2 = 10.5, p = 0.0013; Figure 1D) and mice (n = 7 mice; F1,315 = 13.8, p = 0.0002; Figure 1E).
Modulation of PV intensity by PNNs in motor circuitry of adult songbirds and rodents
The song circuit consists of four forebrain areas – HVC, RA, LMAN, and Area X – that are critical for song learning and performance and are replete with PV neurons and PNNs (Figures 2A,B; Supplementary Figure S2). HVC and RA have been proposed to be analogous, respectively, to premotor and motor cortices, whereas Area X is a basal ganglia structure with both striatal and pallidal components (reviewed in Doupe et al., 2005; Leblois and Perkel, 2020). The intensity of PV was significantly higher in PV+PNN neurons than in PV-PNN neurons in HVC (n = 6 adults; F1,140.8 = 6.5, p = 0.0118) and LMAN (F1,172.9 = 15.5, p = 0.0001; Figure 2B). On the other hand, there were no significant differences in PV intensity between PV+PNN and PV-PNN neurons in RA or Area X (p > 0.15 for each).
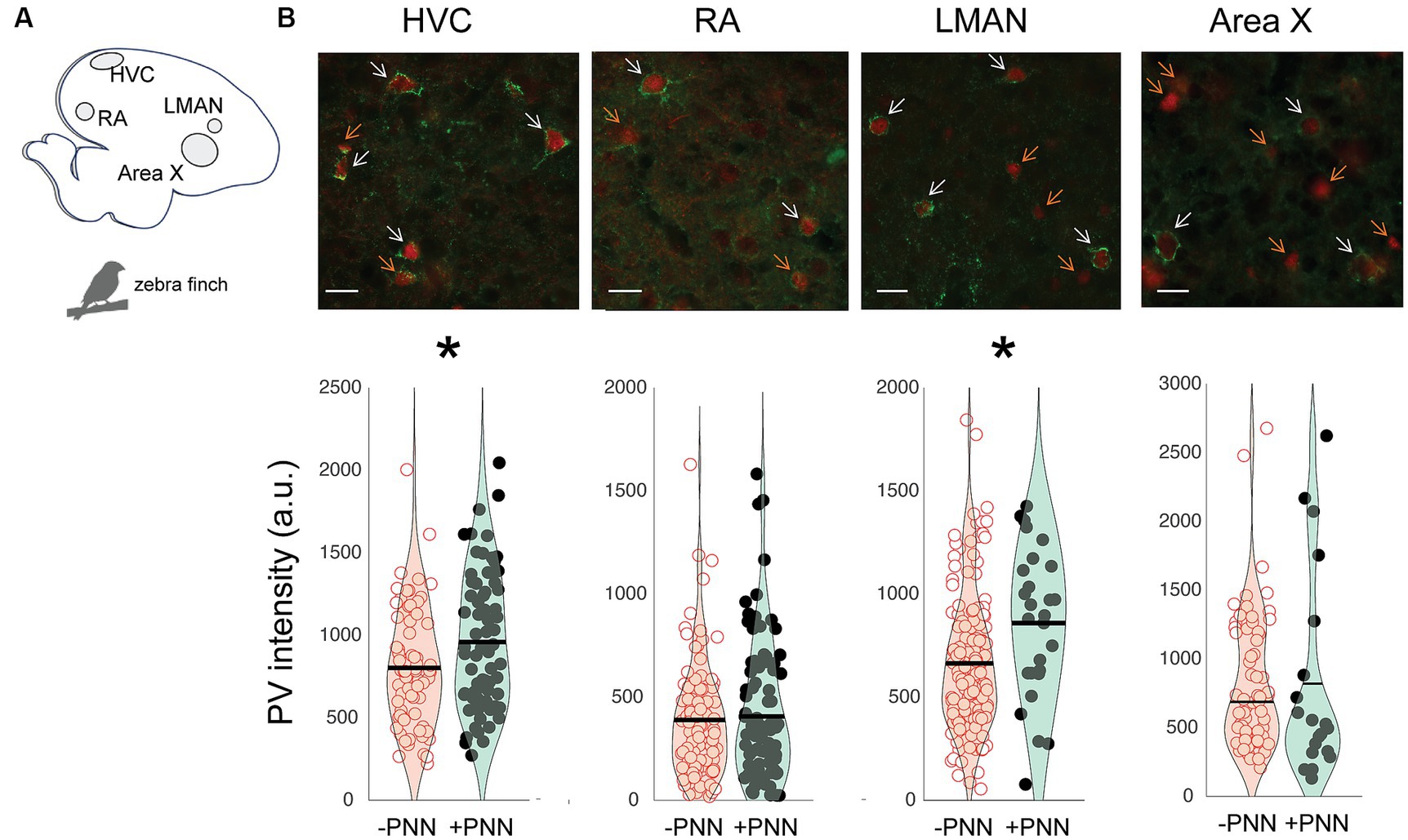
Figure 2. Regional variation in the effect of PNN ensheathment on PV intensity in the song system. (A) Sagittal representation of the zebra finch brain (left = posterior, right = anterior). (B) Representative images of PV neurons (red) and PNNs (green) in HVC, RA, LMAN, and Area X, with white arrows pointing to PV+PNN neurons and orange arrows pointing to PV-PNN neurons. Below the images are PV intensities [in arbitrary units (a.u.)] of PV+PNN (filled circles) and PV-PNN neurons (empty circles) in HVC (n = 145 neurons), RA (n = 188 neurons), LMAN (n = 182 neurons), and Area X (n = 84 neurons) of adult zebra finches (n = 6 birds). Different exposure times were used for different brain nuclei; therefore, differences in PV intensity across brain regions should not be taken to reflect differences in PV intensity. Each symbol represents the PV intensity of a neuron. A violin plot summarizing the distribution is plotted on top of the raw data, with the black line representing the mean of the distribution. “*” denotes p < 0.05 (mixed-effects model).
Similar relationships between PV intensity and PNN ensheathment were observed in the motor cortex and basal ganglia of mice (n = 7 male mice; Figure 3). In both layer II/III and V of the motor cortex, there was a significant difference in PV intensity between PV+PNN and PV-PNN neurons, with PV+PNN neurons being more enriched with PV than PV-PNN neurons (layer II/III: F1,208.9 = 21.0, p < 0.0001; layer V: F1,203.4 = 14.9, p = 0.0002; Figures 3A,B). PV intensities were also significantly higher in PV+PNN neurons than in PV-PNN neurons in the external nucleus of the globus pallidus (GPe; F1,646 = 6.4, p = 0.0114; Figure 3C). PV intensities were not significantly different between PV+PNN and PV-PNN neurons in the dorsolateral striatum (DLS; F1,209.8 = 2.6, p = 0.1076; Figure 3D). This lack of statistical difference seems to be caused by a single high value for PV-PNN neurons, and removal of this point leads to the observation of higher PV intensity for PV+PNN neurons in the DLS (F1,209.5 = 5.2, p = 0.0240).
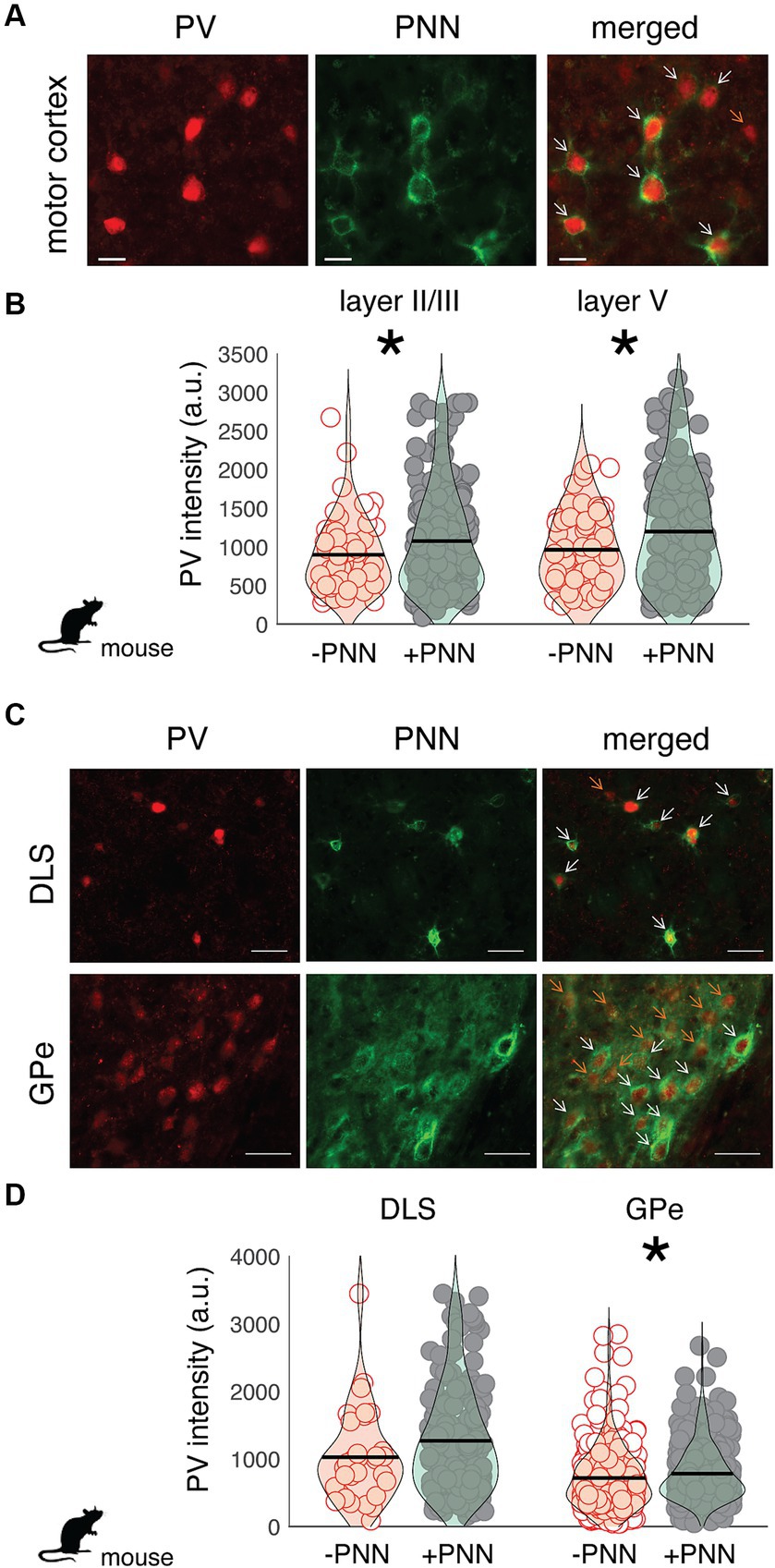
Figure 3. Regional variation in the effect of PNN ensheathment on the intensity of PV expression across the mouse motor cortex, dorsolateral striatum (DLS), and external nucleus of the globus pallidus (GPe). (A) Image of PV neurons (red) and PNNs (green) in the mouse motor cortex (scale bar = 20 um). On the merged (right) image, orange arrows point to PV neurons without PNNs (PV-PNN) and white arrows point to PV neurons with PNNs (PV+PNN). (B) The intensity of PV [in arbitrary units (a.u.)] within PV+PNN neurons (filled circles) was significantly higher than that within PV-PNN neurons (empty circles) in layers II/III (n = 236 neurons) and V (n = 229 neurons) of the motor cortex. (C) Images of PV neurons (red) and PNNs (green) in the dorsolateral striatum (DLS) and external nucleus of the globus pallidus (GPe; scale bar = 50 um). On the merged (right) image, orange arrows point to PV-PNN neurons and white arrows point to PV+PNN neurons. (D) PV intensity within PV+PNN neurons (filled circles) was significantly higher than that within PV-PNN neurons (empty circles) in the GPe (n = 665 neurons) but not in the DLS (n = 230 neurons). For (B) and (D), each symbol represents the PV intensity of a neuron, and a violin plot summarizing the distribution is plotted on top of the raw data, with the black line representing the mean of the distribution. “*” denotes p < 0.05 (mixed-effects model).
Variation in PV intensity in motor circuitry across development in songbirds
Neurons in song control circuitry change in various ways over development, and we investigated the extent to which PV intensity and the relationship between PV intensity and PNN expression changed across development. We first analyzed differences in PV expression between juvenile (~2 months old; n = 7) and adult zebra finches (10–15 months old; n = 5; Figure 4). Overall, PV intensities were significantly higher in PV+PNN neurons than in PV-PNN neurons in HVC (F1,201.3 = 37.4, p < 0.0001), RA (F1,260.1 = 49.1, p < 0.0001), and LMAN (F1,338.5 = 34.0, p < 0.0001; Figures 4A–C). However, we found the opposite pattern in Area X, with PV intensities being significantly lower for PV+PNN neurons than for PV-PNN neurons (F1,286.1 = 5.7, p = 0.0172; Figure 4D). While there were no significant differences between juveniles and adults, there was a trend for PV intensities to be lower in adults than in juveniles in HVC (F1,10.0 = 4.4, p = 0.0619). Interestingly, there was a significant interaction between age and PNN expression in LMAN (F1,338.5 = 20.2, p < 0.0001) and a trend for an interaction in RA (F1,260 = 3.2, p = 0.0759). In LMAN, PV intensities were significantly higher for PV+PNN neurons compared to PV-PNN neurons in juveniles but not in adults, and PV intensities decreased with age for PV+PNN neurons but not for PV-PNN neurons (planned contrasts, p < 0.01). Similar patterns were observed for RA.
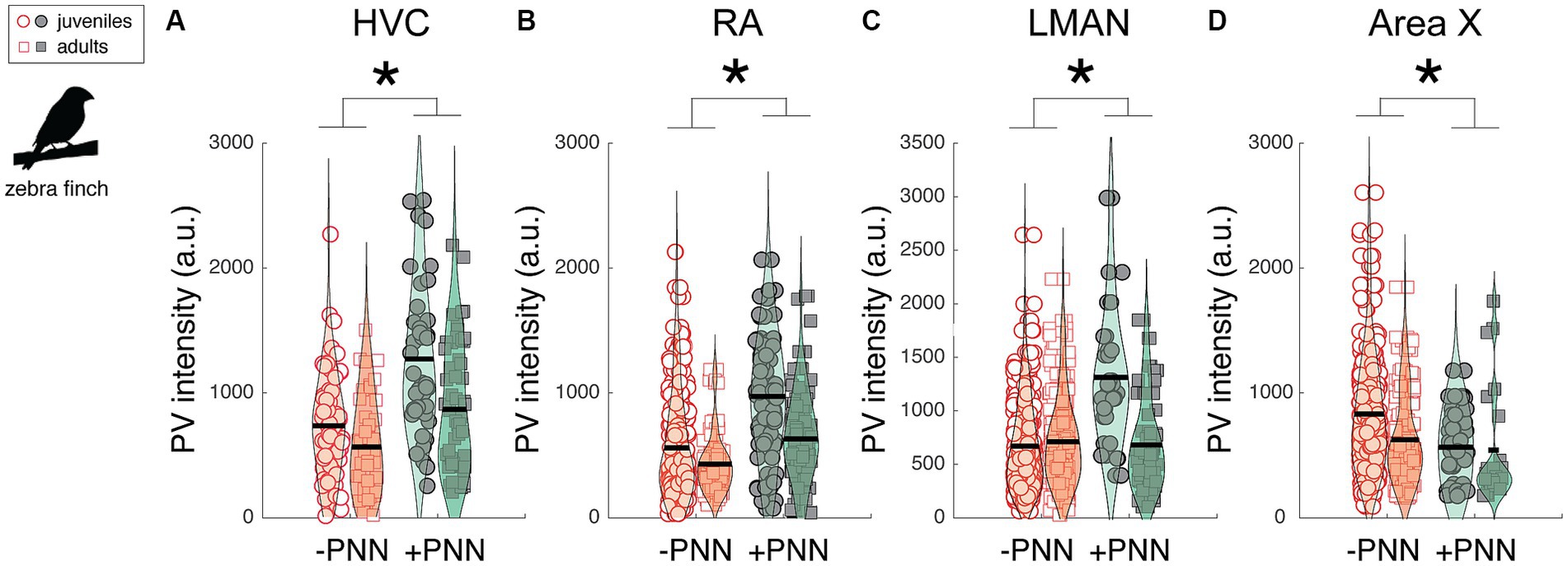
Figure 4. Effects of PNN ensheathment and age (juvenile vs. adult) on PV intensity within (A) HVC, (B) RA, (C) LMAN, and (D) Area X. Similar to the previous analysis (Figure 2), PV+PNN neurons (filled symbols) were significantly more enriched with PV than PV-PNN neurons (empty symbols) in HVC (n = 215 neurons) and LMAN (n = 351 neurons). A similar difference was additionally observed in RA (n = 282 neurons). However, the opposite pattern was observed in Area X (n = 296 neurons), with PV intensity being higher in PV-PNN neurons than in PV+PNN neurons. While there were no significant differences in PV intensity between juveniles and adults, there was a trend for PV intensities to be lower in adults than in juveniles in HVC. Each symbol represents the PV intensity of a neuron, and a violin plot summarizing the distribution is plotted on top of the raw data, with the black line representing the mean of the distribution. “*” denotes p < 0.05 for the effect of PNN ensheathment on PV intensity (mixed-effects model).
The previous analysis highlights differences in PV intensity between juvenile and adult zebra finches that were, on average, nine months apart. Many changes to neural circuitry and behavior occur during the first few months of development; consequently, we analyzed images at different developmental time points (i.e., 40, 60, 90, and 120 dph) from a previously published study (Cornez et al., 2018; Figures 5A–D). There was no significant change in overall PV intensity across development for HVC, LMAN, or Area X but there was a trend for PV intensity to decrease over development up to 90 dph for RA (F3,19.1 = 2.8, p = 0.0657; Figure 5B). Over development, PV+PNN neurons were significantly more enriched with PV than PV-PNN neurons in LMAN (F1,592.1 = 16.1, p < 0.0001; Figure 5C), with a similar trend for HVC (F1,785.9 = 3.6, p = 0.0586; Figure 5A). Additionally, PV+PNN neurons tended to be less enriched with PV than PV-PNN neurons in Area X (F1,368.3 = 2.9, p = 0.0869; Figure 5D).
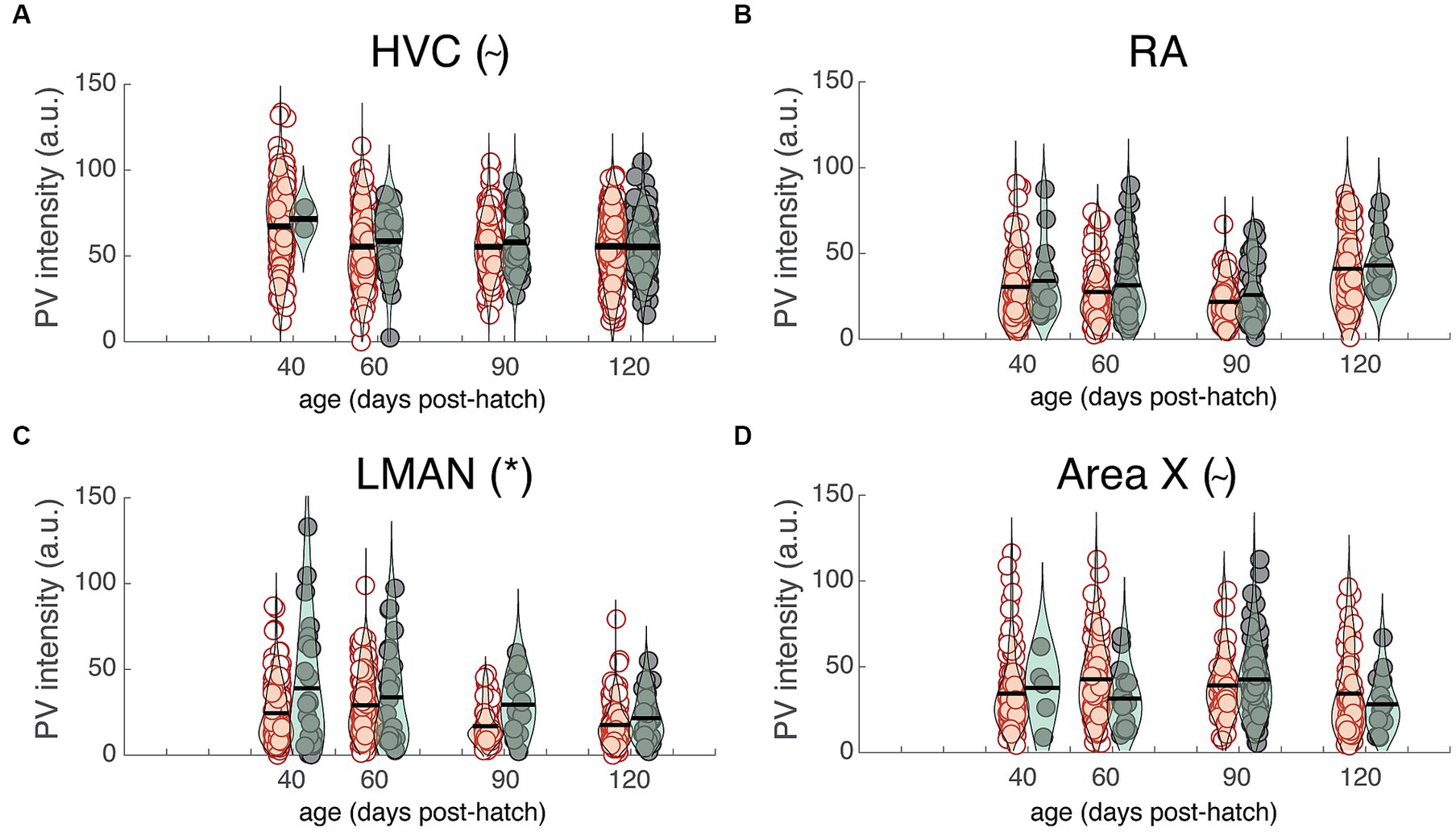
Figure 5. PV intensities of PV+PNN (filled circles) and PV-PNN neurons (empty circles) across development in (A) HVC, (B) RA, (C) LMAN, and (D) Area X. There was no significant overall change in PV intensity from 40 to 120 dph (mixed-effects models), but similar trends or significant differences between PV+PNN and PV-PNN neurons were observed in these birds in HVC (n = 879 neurons; p = 0.0586), RA (n = 528 neurons), LMAN (n = 622 neurons; p < 0.0001), and Area X (n = 389 neurons; p = 0.0869; see also Figure 4). Each symbol represents the PV intensity of a neuron, and a violin plot summarizing the distribution is plotted on top of the raw data, with the black line representing the mean of the distribution. “*” and “~” denote p < 0.05 and p < 0.10, respectively, for the effect of PNN ensheathment on PV intensity (mixed-effects model).
Causal contribution of PNNs to PV intensity in adult songbirds
The preceding analyses suggest that PNNs could augment PV expression in the PV neurons they surround or that PNNs could differentially surround PV neurons that express more PV. To test the former hypothesis, we infused the HVCs of individual birds with either chondroitinase ABC (ChABC; n = 7 birds) or a control enzyme penicillinase (PEN; n = 6 birds) and investigated how degrading PNNs (via ChABC) affected PV expression within PV neurons in HVC. We hypothesized that PV neurons in parts of HVC with degraded PNNs will express less PV than PV+PNN neurons but will display the same degree of PV as PV-PNN neurons.
Similar to mammals, PNNs in HVC were degraded following ChABC infusions but remained intact following PEN infusions (Figures 6A–C). In many instances, ChABC infusions failed to remove PNNs throughout all of HVC (Figures 6A,D); we took advantage of this and analyzed PV intensities within PV+PNN and PV-PNN neurons in the non-affected part of HVC as well as PV intensities of PV neurons in the affected portion (PV+ChABC neurons).
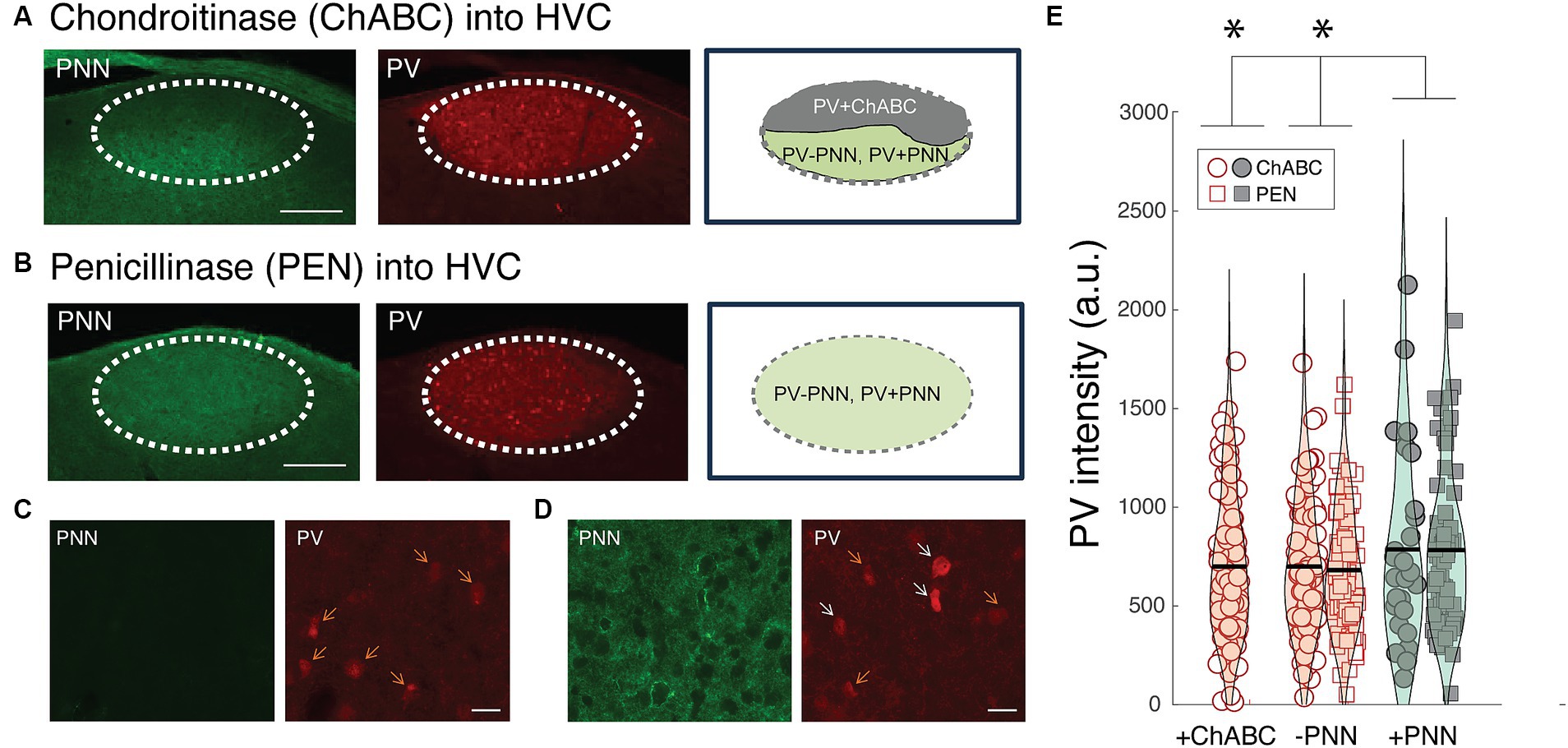
Figure 6. Effects of PNN degradation in HVC on PV intensities. (A) Infusions of ChABC degraded PNNs in HVC. In many instances, the infusions did not cover all of HVC, leaving some portion of HVC with intact PNNs. In this example, PNNs (green) are degraded in the dorsal portion of HVC whereas PNNs remain intact in the ventral portion. The intensity of PV (red) within PV neurons in the affected region (PV+ChABC neurons) and within PV+PNN and PV-PNN neurons in the unaffected region of HVC were analyzed. Scale bar = 200 um. (B) Image of intact PNNs and PV neurons in the HVC of a bird infused with PNN (i.e., infusions of PEN did not degrade PNNs). Scale bar = 200 um. (C) High magnification image of PNN degradation (left) and PV neurons (right) following a ChABC infusion into HVC. (D) High magnification image of intact PNNs (left) and PV neurons from a portion of the HVC without PNN degradation from the same bird as in (C). For both (C) and (D), white arrows point to PV+PNN neurons and orange arrows point to PV-PNN neurons, and scale bar = 20 um. (E) PV+ChABC neurons were comparable in PV intensity to PV-PNN neurons and less intensely stained with PV than PV+PNN neurons in ChABC-treated birds (n = 212 neurons) and PEN-treated birds (n = 154 neurons). Each symbol represents the PV intensity of a neuron, and a violin plot summarizing the distribution is plotted on top of the raw data, with the black line representing the mean of the distribution. “*” denotes p < 0.05 (mixed-effects model).
Consistent with the preceding analyses, PV+PNN neurons in the non-affected portion of HVC in ChABC-treated birds were more enriched with PV than PV-PNN neurons in the same region (F1,97.6 = 3.8, p = 0.0543). This same trend was observed between PV+PNN and PV-PNN neurons in the HVC of PEN-treated birds (F1,151.7 = 3.6, p = 0.0584). Not surprisingly, when the PV intensities of PV+PNN and PV-PNN neurons of birds treated with ChABC or PEN were analyzed together, there was a significant effect of PNN ensheathment (PV+PNN > PV-PNN; F1,248.6 = 6.6, p = 0.0109). Importantly, for this analysis of parts of HVC without PNN degradation, there was no significant effect of group (ChABC vs. PEN) or significant interaction between group and PNN ensheathment (p > 0.8 for each), indicating that the effect of PNN ensheathment was consistent across groups.
We next compared PV intensities of PV+ChABC neurons to PV+PNN and PV-PNN neurons in ChABC- and PEN-treated birds. In ChABC-treated birds, there was no statistically significant difference in PV intensity among PV+PNN, PV-PNN, and PV+ChABC neurons (p > 0.15), but PV+PNN neurons displayed on average the most intense PV staining. When comparing PV intensities of PV+ChABC neurons in ChABC-treated birds and PV+PNN and PV-PNN neurons in PEN-treated birds, no significant difference was observed (p > 0.10), though PV+PNN neurons were the most enriched with PV among the three. Because these two analyses of the effects of ChABC could be underpowered (due to small sample sizes), we analyzed the PV intensities of PV+ChABC neurons, PV+PNN, and PV-PNN neurons in ChABC- and PEN-treated birds simultaneously. When analyzed together, there was a significant difference between PV+ChABC, PV+PNN, and PV-PNN neurons (F2,352.8 = 3.8, p = 0.0241; Figure 6E), with PV+PNN neurons being more enriched with PV than PV+ChABC and PV-PNN neurons (planned contrasts; p < 0.02 for each) and with PV+ChABC neurons expressing indistinguishable levels of PV as PV-PNN neurons (p > 0.60).
Discussion
Despite the prevalence of parvalbumin (PV) neurons across vertebrate taxa, little is known about the regulation of PV in non-mammalian species. Additionally, little is known about the regulation of PV in motor circuitry in either mammals or non-mammalian species. We investigated the degree to which PV expression was modulated by perineuronal nets (PNNs), extracellular matrices that affect plasticity and functional properties of neurons they surround, in the auditory cortex, motor cortex, and basal ganglia of songbirds and rodents. We specifically analyzed differences in PV intensity between PV neurons surrounded or not surrounded by PNNs within each species, and then discerned common patterns of differences across species. We also investigated the causal contribution of PNNs to PV intensity in the songbird brain.
We discovered similarities and differences in the relationship between PV and PNN expression between songbirds and rodents and between motor systems and other circuitry. PV intensity was higher for PV+PNN neurons in the auditory cortices of mice, rats, and songbirds, in the motor cortex of mice, and in the cortical-like areas HVC, RA, and LMAN of songbirds. Despite different methods to visualize PNNs in zebra finches compared to rodents (see Methods), this pattern was consistent across auditory and motor cortices in songbirds and rodents. Our data additionally highlight similarities in the relationship between PV and PNN expression in auditory and motor circuitry and other brain areas including the visual, prefrontal, and dorsolateral cortices and hippocampus in rodents (Yamada and Jinno, 2013; Yamada et al., 2015; Enwright et al., 2016; Favuzzi et al., 2017; Hou et al., 2017; Rowlands et al., 2018; Slaker et al., 2018; Carceller et al., 2020; Lupori et al., 2023). We observed some species variation in the relationship between PNN expression and PV intensity: whereas differences between PV+PNN and PV-PNN neurons in the DLS and GPe of mice were similar to those observed in motor and auditory cortices (PV+PNN > PV-PNN), PV expression was lower for PV+PNN neurons than for PV-PNN neurons in the basal ganglia nucleus Area X of songbirds. Although Area X consists of striatal- and pallidal-like neurons (Leblois and Perkel, 2020), Area X itself is not considered directly homologous to DLS and GPe; therefore, differences between Area X, DLS, and GPe might not be unexpected.
This regional variation in the relationship between PNN and PV expression in the songbird brain supports findings from a recent, comprehensive study of PNN and PV expression in the rodent brain (Lupori et al., 2023) and suggests that PNNs differentially affect neural activity across cortical and basal ganglia circuitry in songbirds. Regional variation in the effects of PNNs on neural activity have been previously documented; for example, decreases in PNN expression in the mouse somatosensory cortex, visual cortex, and deep cerebellar nucleus are associated with decreased neural activity (Lensjø et al., 2017; Tewari et al., 2018; Carulli et al., 2020). In contrast, enzymatic removal of PNNs in mouse hippocampal cultures decreases the amount of depolarizing current required to generate action potentials and reduces after-hyperpolarizations, thereby increasing firing rates (Dityatev et al., 2007). Given the regional variation in the relationship between PV and PNN expression in the song system, our data suggest that PNNs could differentially modulate neural activity and dynamics in HVC, RA, and LMAN compared to Area X. Differences in the relationship between PV ensheathment and PV intensity across brain areas in the song system could also be linked to regional variation in neuronal types (e.g., Pfenning et al., 2014; Colquitt et al., 2021).
Differential PV expression depending on PNN ensheathment suggests that PV+PNN and PV-PNN neurons could represent different neuronal subtypes and could differentially regulate neural dynamics. In the rodent hippocampus, PV neurons with lower PV expression are less likely to be surrounded by PNNs and express somatostatin and neuropeptide Y, whereas PV neurons that express high levels of PV are more likely to be surrounded by PNNs and do not express somatostatin and neuropeptide Y (Yamada et al., 2015). Previous studies in songbirds document that distinct cell types within song circuitry of songbirds differ in PV intensity (Braun et al., 1985; Wild et al., 2001, 2005; Zengin-Toktas and Woolley, 2017). For example, interneurons in RA are enriched with PV whereas RA projection neurons only moderately express PV (Wild et al., 2001). Given that PV neurons in RA that are surrounded by PNNs are more enriched with PV than PV neurons not surrounded by PNNs, this suggests that PNNs might differentially surround RA interneurons. Similarly, a previous study identified at least two types of PV neurons in Area X (Braun et al., 1985): a population of large cells (~16 um in diameter) that were relatively weakly stained with PV and a population of small cells (~10–13 um in diameter) that were more intensely stained with PV. Because a number of our analyses suggest that PV+PNN neurons in Area X are less enriched with PV than PV-PNN neurons in Area X, it is possible that large, weakly stained PV neurons in Area X are differentially surrounded by PNNs compared with small, intensely stained PV neurons. Further, larger PV neurons in Area X are putatively analogous to mammalian GPe neurons whereas smaller PV neurons are more analogous to mammalian striatal fast-spiking interneurons (Carrillo and Doupe, 2004; Reiner et al., 2004; Woolley, 2016; Zengin-Toktas and Woolley, 2017; Kumar et al., 2020; reviewed in Leblois and Perkel, 2020). Our data support this contention because PV neurons in the GPe are less intensely stained with PV than PV neurons in the DLS (Figure 3).
The effect of PNN degradation on PV intensity in the songbird nucleus HVC resembles the effect of PNN degradation in various parts of the mammalian brain. For example, just as experimental reductions of PNNs decrease in PV intensity in PV neurons in the hippocampus and visual cortex (Beurdeley et al., 2012; Yamada et al., 2015; Hou et al., 2017; Rowlands et al., 2018), degradation of PNNs decreased the intensity of PV expression within PV neurons in HVC. Given that PNN degradation also modulates neural activity and oscillations of various types of neurons in mammals, including PV neurons (Dityatev et al., 2007; Balmer, 2016; Carceller et al., 2020), we propose that degradation of PNNs in the song circuitry of songbirds could also modulate neural activity and dynamics and, consequently, vocal performance (e.g., canary: Cornez et al., 2021).
How PNNs modulate PV intensity in songbirds remain unknown. In mammals, PV expression is influenced by Otx2 or brevican. Specifically, PNNs allow for the transfer of Otx2 from the extracellular environment into PV neurons, which subsequently increases PV intensity (Sugiyama et al., 2008; Spatazza et al., 2013). Brevican, a protein commonly expressed in PNNs, affects the localization of potassium channels and AMPA receptors in PV neurons in rodents (Favuzzi et al., 2017), and PV neurons surrounded by brevican are innervated by more excitatory inputs than PV neurons not surrounded by brevican (Favuzzi et al., 2017). Therefore, depending on the degree to which the composition of PNNs vary between mammals and birds, Otx2 and brevican could contribute to elevated PV expression within PV neurons in areas like HVC, RA, LMAN, and Field L.
Taken together, these findings expand the similarities in neural processes and function across songbirds and mammals. These data further support the utility of songbirds as model organisms for the study of sensory and sensorimotor circuitry, especially as they relate to vocal learning and performance. This research also motivates subsequent studies into differences between PV+PNN and PV-PNN neurons in sensory and motor circuitry in mammals and songbirds as well as the contribution of PNNs in motor circuitry to motor performance and plasticity.
Data availability statement
The raw data supporting the conclusions of this article will be made available by the authors, without undue reservation.
Ethics statement
The animal study was approved by the McGill University Animal Care and Use Committee. The study was conducted in accordance with the local legislation and institutional requirements.
Author contributions
AW: Data curation, Investigation, Methodology, Visualization, Writing – original draft, Writing – review & editing. XW: Data curation, Investigation, Visualization, Writing – review & editing. D-SS: Data curation, Investigation, Visualization, Writing – review & editing. VL: Data curation, Investigation, Visualization, Writing – review & editing. GC: Data curation, Writing – review & editing, Investigation, Visualization. JB: Data curation, Writing – review & editing, Funding acquisition. JC-F: Data curation, Investigation, Writing – review & editing. EV-S: Conceptualization, Data curation, Funding acquisition, Investigation, Writing – review & editing. JTS: Conceptualization, Data curation, Formal analysis, Funding acquisition, Investigation, Methodology, Project administration, Resources, Supervision, Visualization, Writing – original draft, Writing – review & editing.
Funding
The author(s) declare financial support was received for the research, authorship, and/or publication of this article. Research was supported by the Natural Sciences and Engineering Research Council (NSERC; #05016 to JTS and #04761 to EV-S), by the Fonds de recherche du Quebec - Nature et technologies (FRQNT; #284884 to JTS), the Canadian Institutes for Health Research (CIHR #438114 to EV-S) and the National Institute for Neurological Disorders and Stroke (NINDS RO1 NS104008 to JB), and funds from the Centre for Research for Brain, Language, and Music (CRBLM) to JTS.
Acknowledgments
Thank you to Y. X. Chen, A. Meilayi, S. Miner, A. Vochin, and Y. Chen for their assistance with histology and data collection, and to E. Fields, and A. J. Watt for supplying mouse tissue for analysis. We also thank S. C. Woolley for input on data analysis and presentation.
Conflict of interest
The authors declare that the research was conducted in the absence of any commercial or financial relationships that could be construed as a potential conflict of interest.
The author(s) declared that they were an editorial board member of Frontiers, at the time of submission. This had no impact on the peer review process and the final decision.
Publisher’s note
All claims expressed in this article are solely those of the authors and do not necessarily represent those of their affiliated organizations, or those of the publisher, the editors and the reviewers. Any product that may be evaluated in this article, or claim that may be made by its manufacturer, is not guaranteed or endorsed by the publisher.
Supplementary material
The Supplementary material for this article can be found online at: https://www.frontiersin.org/articles/10.3389/fncir.2023.1297643/full#supplementary-material
References
Albéri, L., Lintas, A., Kretz, R., Schwaller, B., and Villa, A. E. P. (2013). The calcium-binding protein parvalbumin modulates the firing 1 properties of the reticular thalamic nucleus bursting neurons. J. Neurophysiol. 109, 2827–2841. doi: 10.1152/jn.00375.2012
Alpár, A., Gärtner, U., Härtig, W., and Brückner, G. (2006). Distribution of pyramidal cells associated with perineuronal nets in the neocortex of rat. Brain Res. 1120, 13–22. doi: 10.1016/j.brainres.2006.08.069
Balmer, T. S. (2016). Perineuronal nets enhance the excitability of fast-spiking neurons. eNeuro 3:ENEURO.0112-16.2016. doi: 10.1523/ENEURO.0112-16.2016
Balmer, T. S., Carels, V. M., Frisch, J. L., and Nick, T. A. (2009). Modulation of perineuronal nets and parvalbumin with developmental song learning. J. Neurosci. 29, 12878–12885. do i: 10.1523/JNEUROSCI.2974-09.2009. doi: 10.1523/JNEUROSCI.2974-09.2009
Beurdeley, M., Spatazza, J., Lee, H. H. C., Sugiyama, S., Bernard, C., Di Nardo, A. A., et al. (2012). Otx2 binding to perineuronal nets persistently regulates plasticity in the mature visual cortex. J. Neurosci. 32, 9429–9437. doi: 10.1523/JNEUROSCI.0394-12.2012
Brainard, M. S., and Doupe, A. J. (2002). What songbirds teach us about learning. Nature 417, 351–358. doi: 10.1038/417351a
Brainard, M. S., and Doupe, A. J. (2013). Translating birdsong: songbirds as a model for basic and applied medical research. Annu. Rev. Neurosci. 36, 489–517. doi: 10.1146/annurev-neuro-060909-152826
Braun, K., Scheich, H., Heizmann, C. W., and Hunziker, W. (1991). Parvalbumin and calbindin-D28K immunoreactivity as developmental markers of auditory and vocal motor nuclei of the zebra finch. Neuroscience 40, 853–869. doi: 10.1016/0306-4522(91)90017-i
Braun, K., Scheich, H., Schachner, M., and Heizmann, C. W. (1985). Distribution of parvalbumin, cytochrome oxidase activity and 14C-2-deoxyglucose uptake in the brain of the zebra finch. Cell Tissue Res. 240, 101–115. doi: 10.1007/BF00217563
Carceller, H., Guirado, R., Ripolles-Campos, E., Teruel-Marti, V., and Nacher, J. (2020). Perineuronal nets regulate the inhibitory perisomatic input onto parvalbumin interneurons and γ activity in the prefrontal cortex. J. Neurosci. 40, 5008–5018. doi: 10.1523/JNEUROSCI.0291-20.2020
Cardin, J. A., Carlén, M., Meletis, K., Knoblich, U., Zhang, F., Deisseroth, K., et al. (2009). Driving fast-spiking cells induces gamma rhythm and controls sensory responses. Nature 459, 663–667. doi: 10.1038/nature08002
Carrillo, G. D., and Doupe, A. J. (2004). Is the songbird area X striatal, pallidal, or both? An anatomical study. J. Comp. Neurol. 473, 415–437. doi: 10.1002/cne.20099
Carulli, D., Broersen, R., De Winter, F., Muir, E. M., Mešković, M., De Waal, M., et al. (2020). Cerebellar plasticity and associative memories are controlled by perineuronal nets. Proc. Natl. Acad. Sci. U. S. A. 117, 6855–6865. doi: 10.1073/pnas.1916163117
Carulli, D., Pizzorusso, T., Kwok, J. C. F., Putignano, E., Poli, A., Forostyak, S., et al. (2010). Animals lacking link protein have attenuated perineuronal nets and persistent plasticity. Brain 133, 2331–2347. doi: 10.1093/brain/awq145
Cisneros-Franco, J. M., and de Villers-Sidani, É. (2019). Reactivation of critical period plasticity in adult auditory cortex through chemogenetic silencing of parvalbumin-positive interneurons. Proc. Natl. Acad. Sci. U. S. A. 116, 26329–26331. doi: 10.1073/pnas.1913227117
Cisneros-Franco, J. M., Ouellet, L., Kamal, B., and de Villers-Sidani, É. (2018). A brain without brakes: reduced inhibition is associated with enhanced but dysregulated plasticity in the aged rat auditory cortex. eNeuro :5. doi: 10.1523/ENEURO.0051-18.2018
Cisneros-Franco, J. M., Voss, P., Thomas, M. E., and de Villers-Sidani, É. (2020). “Chapter 8 – critical periods of brain development” in Handbook of clinical neurology neurocognitive development: normative development. eds. A. Gallagher, C. Bulteau, D. Cohen, and J. L. Michaud (Netherlands: Elsevier), 75–88.
Colquitt, B. M., Merullo, D. P., Konopka, G., Roberts, T. F., and Brainard, M. S. (2021). Cellular transcriptomics reveals evolutionary identities of songbird vocal circuits. Science 371:eabd9704. doi: 10.1126/science.abd9704
Cornez, G., Collignon, C., Müller, W., Cornil, C. A., Ball, G. F., and Balthazart, J. (2020a). Development of perineuronal nets during ontogeny correlates with sensorimotor vocal learning in canaries. eNeuro 7:ENEURO.0361-19.2020. doi: 10.1523/ENEURO.0361-19.2020
Cornez, G., Jonckers, E., Ter Haar, S. M., Van Der Linden, A., Cornil, C. A., and Balthazart, J. (2018). Timing of perineuronal net development in the zebra finch song control system correlates with developmental song learning. Proc. R. Soc. B 285:20180849. doi: 10.1098/rspb.2018.0849
Cornez, G., Langro, J., Cornil, C. A., Balthazart, J., and Lynch, K. S. (2020b). Comparing perineuronal nets and parvalbumin development between blackbird species with differences in early developmental song exposure. J. Exp. Biol. 223:jeb. 212910. doi: 10.1242/jeb.212910
Cornez, G., Madison, F. N., Van Der Linden, A., Cornil, C., Yoder, K. M., Ball, G. F., et al. (2017). Perineuronal nets and vocal plasticity in songbirds: a proposed mechanism to explain the difference between closed-ended and open-ended learning: perineuronal nets and singing plasticity in songbirds. Devel Neurobio 77, 975–994. doi: 10.1002/dneu.22485
Cornez, G., Ter Haar, S. M., Cornil, C. A., and Balthazart, J. (2015). Anatomically discrete sex differences in neuroplasticity in Zebra finches as reflected by Perineuronal nets. PLoS One 10:e0123199. do i: 10.1371/journal.pone.0123199. doi: 10.1371/journal.pone.0123199
Cornez, G., Valle, S., and Santos, E. B. dos, Chiver, I., Müller, W., Ball, G. F., et al. (2021). Perineuronal nets in HVC and plasticity in male canary song. PLoS One 16:e0252560. doi: 10.1371/journal.pone.0252560
del Río, J. A., de Lecea, L., Ferrer, I., and Soriano, E. (1994). The development of parvalbumin-immunoreactivity in the neocortex of the mouse. Dev. Brain Res. 81, 247–259. doi: 10.1016/0165-3806(94)90311-5
Deng, X., Gu, L., Sui, N., Guo, J., and Liang, J. (2019). Parvalbumin interneuron in the ventral hippocampus functions as a discriminator in social memory. Proc. Natl. Acad. Sci. U. S. A. 116, 16583–16592. doi: 10.1073/pnas.1819133116
Dityatev, A., Brückner, G., Dityateva, G., Grosche, J., Kleene, R., and Schachner, M. (2007). Activity-dependent formation and functions of chondroitin sulfate-rich extracellular matrix of perineuronal nets. Devel Neurobio 67, 570–588. doi: 10.1002/dneu.20361
Doupe, A. J., Perkel, D. J., Reiner, A., and Stern, E. A. (2005). Birdbrains could teach basal ganglia research a new song. Trends Neurosci. 28, 353–363. doi: 10.1016/j.tins.2005.05.005
Enwright, J. F., Sanapala, S., Foglio, A., Berry, R., Fish, K. N., and Lewis, D. A. (2016). Reduced labeling of parvalbumin neurons and perineuronal nets in the dorsolateral prefrontal cortex of subjects with schizophrenia. Neuropsychopharmacology 41, 2206–2214. doi: 10.1038/npp.2016.24
Fader, S. M., Imaizumi, K., Yanagawa, Y., and Lee, C. C. (2016). Wisteria floribunda agglutinin-labeled perineuronal nets in the mouse inferior colliculus, thalamic reticular nucleus and auditory cortex. Brain Sci. 6:13. doi: 10.3390/brainsci6020013
Favuzzi, E., Marques-Smith, A., Deogracias, R., Winterflood, C. M., Sánchez-Aguilera, A., Mantoan, L., et al. (2017). Activity-dependent gating of parvalbumin interneuron function by the perineuronal net protein Brevican. Neuron 95, 639–655.e10. doi: 10.1016/j.neuron.2017.06.028
Gobes, S. M. H., Jennings, R. B., and Maeda, R. K. (2019). The sensitive period for auditory-vocal learning in the zebra finch: consequences of limited-model availability and multiple-tutor paradigms on song imitation. Behav. Process. 163, 5–12. doi: 10.1016/j.beproc.2017.07.007
Gogola, J. V., Gores, E. O., and London, S. E. (2019). Inhibitory cell populations depend on age, sex, and prior experience across a neural network for critical period learning. Sci. Rep. 9:19867. doi: 10.1038/s41598-019-56293-2
Hara, E., Rivas, M. V., Ward, J. M., Okanoya, K., and Jarvis, E. D. (2012). Convergent differential regulation of parvalbumin in the brains of vocal learners. PLoS One 7:e29457. doi: 10.1371/journal.pone.0029457
Hensch, T. K., Fagiolini, M., Mataga, N., Stryker, M. P., Baekkeskov, S., and Kash, S. F. (1998). Local GABA circuit control of experience-dependent plasticity in developing visual cortex. Science 282, 1504–1508. doi: 10.1126/science.282.5393.1504
Horn, G. (2004). Pathways of the past: the imprint of memory. Nat. Rev. Neurosci. 5, 108–120. doi: 10.1038/nrn1324
Hou, X., Yoshioka, N., Tsukano, H., Sakai, A., Miyata, S., Watanabe, Y., et al. (2017). Chondroitin sulfate is required for onset and offset of critical period plasticity in visual cortex. Sci. Rep. 7:12646. doi: 10.1038/s41598-017-04007-x
Hu, H., Gan, J., and Jonas, P. (2014). Fast-spiking, parvalbumin+ GABAergic interneurons: from cellular design to microcircuit function. Science 345:1255263. doi: 10.1126/science.1255263
Kinney, J. W., Davis, C. N., Tabarean, I., Conti, B., Bartfai, T., and Behrens, M. M. (2006). A specific role for NR2A-containing NMDA receptors in the maintenance of parvalbumin and GAD67 immunoreactivity in cultured interneurons. J. Neurosci. 26, 1604–1615. do i: 10.1523/JNEUROSCI.4722-05.2006. doi: 10.1523/JNEUROSCI.4722-05.2006
Kumar, S., Mohapatra, A. N., Pundir, A. S., Kumari, M., Din, U., Sharma, S., et al. (2020). Blocking opioid receptors in a songbird cortical region modulates the acoustic features and levels of female-directed singing. Front. Neurosci. 14:554094. doi: 10.3389/fnins.2020.554094
Leblois, A., and Perkel, D. J. (2020). “The Song circuit as a model of basal ganglia function” in The neuroethology of birdsong springer handbook of auditory research. eds. J. T. Sakata, S. C. Woolley, R. R. Fay, and A. N. Popper (Cham: Springer International Publishing), 93–125.
Lensjø, K. K., Lepperød, M. E., Dick, G., Hafting, T., and Fyhn, M. (2017). Removal of perineuronal nets unlocks juvenile plasticity through network mechanisms of decreased inhibition and increased gamma activity. J. Neurosci. 37, 1269–1283. do i: 10.1523/JNEUROSCI.2504-16.2016. doi: 10.1523/JNEUROSCI.2504-16.2016
Liu, H., Gao, P.-F., Xu, H.-W., Liu, M.-M., Yu, T., Yao, J.-P., et al. (2013). Perineuronal nets increase inhibitory GABAergic currents during the critical period in rats. Int. J. Ophthalmol. 6, 120–125. doi: 10.3980/j.issn.2222-3959.2013.02.02
Lupori, L., Totaro, V., Cornuti, S., Ciampi, L., Carrara, F., Grilli, E., et al. (2023). A comprehensive atlas of perineuronal net distribution and colocalization with parvalbumin in the adult mouse brain. Cell Rep. 42:112788. doi: 10.1016/j.celrep.2023.112788
Miranda, J. M., Cruz, E., Bessières, B., and Alberini, C. M. (2022). Hippocampal parvalbumin interneurons play a critical role in memory development. Cell Rep. 41:111643. doi: 10.1016/j.celrep.2022.111643
Murphy, K., Lawley, K. S., Smith, P., and Prather, J. F. (2020). “New insights into the avian song system and neuronal control of learned vocalizations” in The neuroethology of birdsong, springer handbook of auditory research. eds. J. T. Sakata, S. C. Woolley, R. R. Fay, and A. N. Popper, vol. 71 (Cham: Springer International Publishing), 65–92.
Murray, A. J., Woloszynowska-Fraser, M. U., Ansel-Bollepalli, L., Cole, K. L. H., Foggetti, A., Crouch, B., et al. (2015). Parvalbumin-positive interneurons of the prefrontal cortex support working memory and cognitive flexibility. Sci. Rep. 5:16778. doi: 10.1038/srep16778
Olson, C. R., Hodges, L. K., and Mello, C. V. (2015). Dynamic gene expression in the song system of zebra finches during the song learning period: ontogeny of Song system gene expression. Devel Neurobio 75, 1315–1338. doi: 10.1002/dneu.22286
Pfenning, A. R., Hara, E., Whitney, O., Rivas, M. V., Wang, R., Roulhac, P. L., et al. (2014). Convergent transcriptional specializations in the brains of humans and song-learning birds. Science 346:1256846. doi: 10.1126/science.125684
Pizzorusso, T., Medini, P., Berardi, N., Chierzi, S., Fawcett, J. W., and Maffei, L. (2002). Reactivation of ocular dominance plasticity in the adult visual cortex. Science 298, 1248–1251. doi: 10.1126/science.1072699
Pyka, M., Wetzel, C., Aguado, A., Geissler, M., Hatt, H., and Faissner, A. (2011). Chondroitin sulfate proteoglycans regulate astrocyte-dependent synaptogenesis and modulate synaptic activity in primary embryonic hippocampal neurons. Eur. J. Neurosci. 33, 2187–2202. doi: 10.1111/j.1460-9568.2011.07690.x
Reiner, A., Laverghetta, A. V., Meade, C. A., Cuthbertson, S. L., and Bottjer, S. W. (2004). An immunohistochemical and pathway tracing study of the striatopallidal organization of area X in the male zebra finch. J. Comp. Neurol. 469, 239–261. doi: 10.1002/cne.11012
Rowlands, D., Lensjø, K. K., Dinh, T., Yang, S., Andrews, M. R., Hafting, T., et al. (2018). Aggrecan directs extracellular matrix-mediated neuronal plasticity. J. Neurosci. 38, 10102–10113. doi: 10.1523/JNEUROSCI.1122-18.2018
Sakata, J. T., and Woolley, S. C. (2020). “Scaling the levels of birdsong analysis” in The neuroethology of birdsong springer handbook of auditory research. eds. J. T. Sakata, S. C. Woolley, R. R. Fay, and A. N. Popper (Cham: Springer International Publishing), 1–27.
Sakata, J. T., and Woolley, S. C. (2022). The role of parvalbumin neurons in the evolution of skilled behaviours. PLoS Biol. 20:e3001795. doi: 10.1371/journal.pbio.3001795
Sakata, J. T., and Yazaki-Sugiyama, Y. (2020). “Neural circuits underlying vocal learning in songbirds” in The neuroethology of birdsong springer handbook of auditory research. eds. J. T. Sakata, S. C. Woolley, R. R. Fay, and A. N. Popper, vol. 71 (Cham: Springer International Publishing), 29–63.
Schuppe, E. R., Cantin, L., Chakraborty, M., Biegler, M. T., Jarvis, E. R., Chen, C.-C., et al. (2022). Forebrain nuclei linked to woodpecker territorial drum displays mirror those that enable vocal learning in songbirds. PLoS Biol. 20:e3001751. doi: 10.1371/journal.pbio.3001751
Shi, W., Wei, X., Wang, X., Du, S., Liu, W., Song, J., et al. (2019). Perineuronal nets protect long-term memory by limiting activity-dependent inhibition from parvalbumin interneurons. Proc. Natl. Acad. Sci. U. S. A. 116, 27063–27073. doi: 10.1073/pnas.1902680116
Slaker, M. L., Jorgensen, E. T., Hegarty, D. M., Liu, X., Kong, Y., Zhang, F., et al. (2018). Cocaine exposure modulates perineuronal nets and synaptic excitability of fast-spiking interneurons in the medial prefrontal cortex. eNeuro 5:ENEURO.0221-18.2018. doi: 10.1523/ENEURO.0221-18.2018
Sohal, V. S., Zhang, F., Yizhar, O., and Deisseroth, K. (2009). Parvalbumin neurons and gamma rhythms enhance cortical circuit performance. Nature 459, 698–702. doi: 10.1038/nature07991
Spatazza, J., Lee, H. H. C., Di Nardo, A. A., Tibaldi, L., Joliot, A., Hensch, T. K., et al. (2013). Choroid-plexus-derived Otx2 homeoprotein constrains adult cortical plasticity. Cell Rep. 3, 1815–1823. doi: 10.1016/j.celrep.2013.05.014
Spiro, J. E., Dalva, M. B., and Mooney, R. (1999). Long-range inhibition within the zebra finch song nucleus RA can coordinate the firing of multiple projection neurons. J. Neurophysiol. 81, 3007–3020. doi: 10.1152/jn.1999.81.6.3007
Sugiyama, S., Di Nardo, A. A., Aizawa, S., Matsuo, I., Volovitch, M., Prochiantz, A., et al. (2008). Experience-dependent transfer of Otx2 homeoprotein into the visual cortex activates postnatal plasticity. Cells 134, 508–520. doi: 10.1016/j.cell.2008.05.054
Takesian, A. E., and Hensch, T. K. (2013). Balancing plasticity/stability across brain development. Prog Brain Res 207, 3–34. doi: 10.1016/B978-0-444-63327-9.00001-1
Tewari, B. P., Chaunsali, L., Campbell, S. L., Patel, D. C., Goode, A. E., and Sontheimer, H. (2018). Perineuronal nets decrease membrane capacitance of peritumoral fast spiking interneurons in a model of epilepsy. Nat. Commun. 9:4724. doi: 10.1038/s41467-018-07113-0
Wang, D., and Fawcett, J. (2012). The perineuronal net and the control of CNS plasticity. Cell Tissue Res. 349, 147–160. doi: 10.1007/s00441-012-1375-y
Wild, J. M., Williams, M. N., Howie, G. J., and Mooney, R. (2005). Calcium-binding proteins define interneurons in HVC of the zebra finch (Taeniopygia guttata). J. Comp. Neurol. 483, 76–90. doi: 10.1002/cne.20403
Wild, J. M., Williams, M. N., and Suthers, R. A. (2001). Parvalbumin-positive projection neurons characterise the vocal premotor pathway in male, but not female, zebra finches. Brain Res. 917, 235–252. doi: 10.1016/S0006-8993(01)02938-9
Wöhr, M., Orduz, D., Gregory, P., Moreno, H., Khan, U., Vörckel, K. J., et al. (2015). Lack of parvalbumin in mice leads to behavioral deficits relevant to all human autism core symptoms and related neural morphofunctional abnormalities. Transl. Psychiatry 5:e525. doi: 10.1038/tp.2015.19
Woolley, S. C. (2016). Social context differentially modulates activity of two interneuron populations in an avian basal ganglia nucleus. J. Neurophysiol. 116, 2831–2840. doi: 10.1152/jn.00622.2016
Woolley, S. C., and Woolley, S. M. N. (2020). “Integrating form and function in the songbird auditory forebrain” in The neuroethology of birdsong springer handbook of auditory research. eds. J. T. Sakata, S. C. Woolley, R. R. Fay, and A. N. Popper (Cham: Springer International Publishing), 127–155.
Xia, F., Richards, B. A., Tran, M. M., Josselyn, S. A., Takehara-Nishiuchi, K., and Frankland, P. W. (2017). Parvalbumin-positive interneurons mediate neocortical-hippocampal interactions that are necessary for memory consolidation. elife 6:e27868. doi: 10.7554/eLife.27868
Xiang, L., Wu, Q., Sun, H., Miao, X., Lv, Z., Liu, H., et al. (2022). SARM1 deletion in parvalbumin neurons is associated with autism-like behaviors in mice. Cell Death Dis. 13, 638–611. doi: 10.1038/s41419-022-05083-2
Xue, M., Atallah, B. V., and Scanziani, M. (2014). Equalizing excitation–inhibition ratios across visual cortical neurons. Nature 511, 596–600. doi: 10.1038/nature13321
Yamada, J., and Jinno, S. (2013). Spatio-temporal differences in perineuronal net expression in the mouse hippocampus, with reference to parvalbumin. Neuroscience 253, 368–379. doi: 10.1016/j.neuroscience.2013.08.061
Yamada, J., Ohgomori, T., and Jinno, S. (2015). Perineuronal nets affect parvalbumin expression in GABAergic neurons of the mouse hippocampus. Eur. J. Neurosci. 41, 368–378. doi: 10.1111/ejn.12792
Keywords: songbird, zebra finch, auditory cortex, motor cortex, basal ganglia, globus pallidus, striatum, development
Citation: Wang AS, Wan X, Storch D-S, Li VY, Cornez G, Balthazart J, Cisneros-Franco JM, de Villers-Sidani E and Sakata JT (2023) Cross-species conservation in the regulation of parvalbumin by perineuronal nets. Front. Neural Circuits. 17:1297643. doi: 10.3389/fncir.2023.1297643
Edited by:
Paul B. Manis, University of North Carolina at Chapel Hill, United StatesReviewed by:
Jeffrey Garrett Mellott, Northeast Ohio Medical University, United StatesJulie E. Miller, University of Arizona, United States
Copyright © 2023 Wang, Wan, Storch, Li, Cornez, Balthazart, Cisneros-Franco, de Villers-Sidani and Sakata. This is an open-access article distributed under the terms of the Creative Commons Attribution License (CC BY). The use, distribution or reproduction in other forums is permitted, provided the original author(s) and the copyright owner(s) are credited and that the original publication in this journal is cited, in accordance with accepted academic practice. No use, distribution or reproduction is permitted which does not comply with these terms.
*Correspondence: Jon T. Sakata, am9uLnNha2F0YUBtY2dpbGwuY2E=