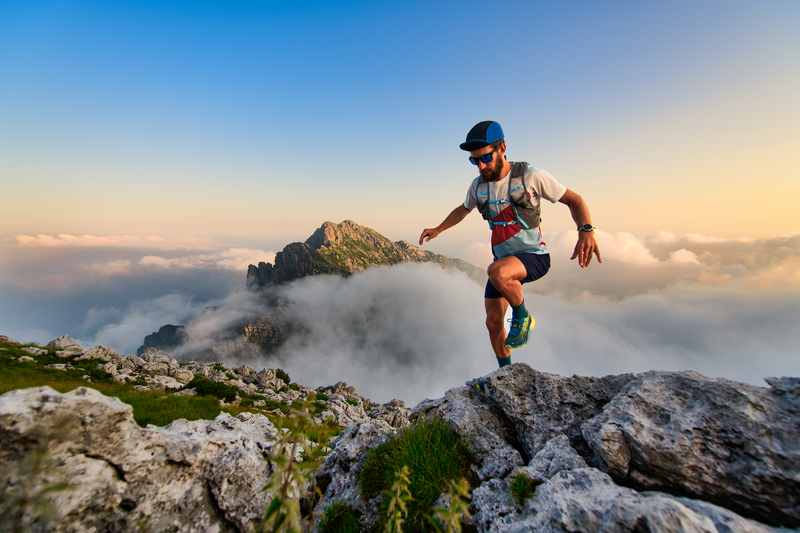
94% of researchers rate our articles as excellent or good
Learn more about the work of our research integrity team to safeguard the quality of each article we publish.
Find out more
MINI REVIEW article
Front. Neural Circuits , 29 September 2023
Volume 17 - 2023 | https://doi.org/10.3389/fncir.2023.1223891
This article is part of the Research Topic GABAergic Circuits in Health and Disease View all 11 articles
GABAergic inhibitory neurons are the principal source of inhibition in the brain. Traditionally, their role in maintaining the balance of excitation-inhibition has been emphasized. Beyond homeostatic functions, recent circuit mapping and functional manipulation studies have revealed a wide range of specific roles that GABAergic circuits play in dynamically tilting excitation-inhibition coupling across spatio-temporal scales. These span from gating of compartment- and input-specific signaling, gain modulation, shaping input–output functions and synaptic plasticity, to generating signal-to-noise contrast, defining temporal windows for integration and rate codes, as well as organizing neural assemblies, and coordinating inter-regional synchrony. GABAergic circuits are thus instrumental in controlling single-neuron computations and behaviorally-linked network activity. The activity dependent modulation of sensory and mnemonic information processing by GABAergic circuits is pivotal for the formation and maintenance of episodic memories in the hippocampus. Here, we present an overview of the local and long-range GABAergic circuits that modulate the dynamics of excitation-inhibition and disinhibition in the main output area of the hippocampus CA1, which is crucial for episodic memory. Specifically, we link recent findings pertaining to GABAergic neuron molecular markers, electrophysiological properties, and synaptic wiring with their function at the circuit level. Lastly, given that area CA1 is particularly impaired during early stages of Alzheimer’s disease, we emphasize how these GABAergic circuits may contribute to and be involved in the pathophysiology.
Gamma-aminobutyric acid (GABA) is the primary inhibitory neurotransmitter in the mammalian central nervous system. It is released by GABAergic inhibitory neurons (INs), which serve as one of the main sources of inhibition (Caputi et al., 2013; Le Magueresse and Monyer, 2013). GABAergic INs modulate the activity of other neurons to maintain a homeostatic excitation-inhibition balance. This is a striking feature of the cortex where E/I ratios are tightly maintained within various layers (Pfeffer et al., 2013; Xue et al., 2014; Adesnik, 2018; Yen et al., 2022). Do GABAergic inputs just act to balance excitation, or do they have specific roles in organizing information flow? The balance of excitation and inhibition is critical for normal brain function. Disruptions to excitation-inhibition balance can result in hyperexcitability, runaway excitation, and disturbance of oscillatory synchrony, which can be seen in epilepsy, neuropsychiatric disorders such as post-traumatic stress disorder (PTSD), schizophrenia, anxiety, and depression (Marín, 2012), and neurodegenerative diseases such as Alzheimer’s disease (AD; Han et al., 2012; Busche et al., 2015; Busche and Konnerth, 2016; O’Donnell et al., 2017; Bridi et al., 2020). However, during learning, shifts in the weight of excitation and inhibition are important to discriminate and store only the relevant information based on contexts. A precise model of how excitatory and inhibitory neurons cooperate to tune information flow is crucial to our understanding of the brain. In this context, hippocampal area CA1 is particularly interesting to study- it provides the main output of the hippocampus and thus critical for memory-guided behavior. Interestingly, CA1 is also the part of hippocampus affected earliest and the most in AD (Braak and Braak, 1991).
A single hippocampal CA1 pyramidal neuron receives several different inputs (glutamatergic, GABAergic) from various sources (hippocampus, entorhinal cortex, prefrontal cortex). Glutamatergic inputs drive excitation in a circuit; GABAergic inputs typically inhibit the propagation of excitation. How do local and long-range GABAergic circuits interact to change the dynamics of excitation and inhibition for acquiring important information? The hippocampal CA1 region alone has >21 different types of local GABAergic neurons with distinct molecular make-up and physiological properties. However, we know little about how these GABAergic neurons contribute to hippocampal functions such as plasticity or learning behavior.
Several lines of evidence suggest that local and long-range circuit interactions between pyramidal neurons (PNs) and GABAergic INs are poised to play a prominent role in higher-order cognitive functions. Within the hippocampus, dynamically controlling the excitation-inhibition (E/I) balance – tilting it in favor of excitation or inhibition in a context-specific manner at the single cell and network levels – can influence memory processing, multisensory coding, and fine-tuning of behaviorally-relevant neuronal activity (Klausberger and Somogyi, 2008; Joshi et al., 2017; Szabo et al., 2022). For example, functional interactions between the prefrontal cortex, entorhinal cortex, and hippocampus that support formation of episodic memories of context and events in the hippocampus rely on the activity of long-range GABAergic projection neurons from the cortex and local GABAergic microcircuits in the hippocampus (Basu et al., 2013, 2016; Malik et al., 2022).
At the single-neuron level, the distribution of specific types of inhibitory synapses varies along the somato-dendritic axis of pyramidal neurons found in hippocampal area CA1, even within a dendritic branch (Megías et al., 2001; Bloss et al., 2016; Cembrowski et al., 2016). Surprisingly, the electrophysiological, neurochemical, and functional characteristics of the inhibitory synapses correlate with their axo-dendritic distribution. At the network level, the interaction between local and long-range excitation and inhibition in the cortex and hippocampus could be important for supporting context-dependent stability and flexibility of memory representations encoding familiar and novel experiences and generating adaptive learned behaviors. Furthermore, GABAergic circuits may substantially coordinate oscillations (Somogyi et al., 2014), such as gamma oscillations during learning (Wulff et al., 2009), theta oscillations during locomotion (Csicsvari et al., 1999; Buzsáki, 2002; Bezaire et al., 2016; Melzer and Monyer, 2020), and sharp wave ripples (SWR; Cutsuridis and Taxidis, 2013; Schlingloff et al., 2014; Stark et al., 2014; Evangelista et al., 2020; Noguchi et al., 2022) in quiet wakefulness, and sleep (Eichenbaum, 2000; Squire, 2004; Basu and Siegelbaum, 2015; Francavilla et al., 2018; Eyre and Bartos, 2019; Udakis et al., 2020; Malik et al., 2022; Yen et al., 2022).
In the present review, we describe the local and long-range GABAergic circuits in hippocampal area CA1. However, rather than describing inhibitory neuron types based on their expressed molecular markers, we focus on a functional classification approach. We present our perspective on how specific inhibitory microcircuits modulate compartment-specific activity, as well as how the dynamic interaction between excitation, inhibition, and disinhibition shapes dendritic integration, plasticity, and behavior. We particularly showcase the recently described GABAergic disinhibitory circuit motifs to emphasize the role of ‘inhibitory’ neurons in boosting excitatory signaling rather than curbing it, and coordinating long-range inter-regional interactions beyond local ‘interneuron’ domains. Finally, we highlight how these circuits may contribute to the neurodegeneration seen during Alzheimer’s disease.
Within the hippocampus, local inhibition is mediated by GABAergic microcircuits comprising of INs that target PNs directly to suppress their activity. Within these GABAergic microcircuits that drive inhibition there are two organizational motifs.
Within hippocampal area CA1, feed-forward inhibition (FFI) is locally mediated by INs that are directly excited by the glutamatergic inputs arriving from the EC or by intra-hippocampal glutamatergic inputs from CA3 or CA2 (Chevaleyre and Siegelbaum, 2010; Basu and Siegelbaum, 2015; Ferrante and Ascoli, 2015; Zemla and Basu, 2017; Bilash et al., 2023). These INs then target PNs to limit their activity and the propagation of information (Buzsaki, 1984; Pouille and Scanziani, 2001; Price et al., 2008; Kullmann, 2011; Basu et al., 2013, 2016; Figure 1).
Figure 1. General GABAergic Circuit Motifs in area CA1. Schematic representations of (A) feed-forward inhibition (FFI) and (B) feed-back inhibition (FBI) in CA1 highlighting the pyramidal neuron (PN, in blue) with the Schaffer Collateral inputs (SC, arrows in black) and the inhibition of local interneurons (IN, in red) in somatic (S) and dendritic (D) compartments. (C) Long-range disinhibition (D) Local disinhibition in hippocampal area CA1.
Feedback inhibition (FBI) occurs when CA1 PNs recurrently target INs through direct monosynaptic connections and the INs in turn target the PNs (Tremblay et al., 2016). Thus, FBI is driven by recurrent excitation and the overall effect is auto-inhibition of PN activity. The initial drive for the PN spiking to trigger FBI arises from strong activity of the proximal CA3 or CA2 excitatory inputs and on occasion from distal EC (Buzsaki, 1984; Kullmann, 2011; Tyan et al., 2014; Figure 1).
Disinhibition is a circuit motif that results from an IN directly targeting another IN, which releases the inhibition from a downstream PN (Basu et al., 2013, 2016; Francavilla et al., 2015; Guet-McCreight et al., 2020; Bilash et al., 2023). So rather than inhibiting a PN, the GABAergic IN-specific-IN (ISI) mediates inhibition of inhibition and thereby disinhibition of coincident excitatory transmission. In the hippocampus, disinhibitory gating can be local (LEC driven VIP INs) or long-range (direct GABAergic projections from EC). Curiously, most long-range GABAergic projection neurons synapse onto INs in their target region and are therefore primarily disinhibitory in nature (Fuchs et al., 2007; Melzer et al., 2012; Caputi et al., 2013; Tyan et al., 2014; Basu et al., 2016; Melzer and Monyer, 2020; Yen et al., 2022; Figure 1).
The hippocampus is anatomically divided into different sub-regions, namely dentate gyrus (DG), CA3, CA2, CA1 and subiculum, which are each believed to perform distinct roles in memory operations. Each of these subregions have a laminar organization through the dorso-ventral axis (Amaral and Witter, 1989; Zemla and Basu, 2017). Hippocampal area CA1 consists of four layers. The pyramidal cell layer, or stratum pyramidale, is where all the PN somata are located. These PNs are innervated exclusively by GABAergic synapses from perisomatic basket interneurons. The stratum oriens (SO) is where the basal dendrites and axonal arborizations of CA1 PNs are located. SO hosts oriens lacunosom moleculare (OLM) interneurons and basket cell soma and axo-axonic interneurons (Maccaferri, 2005), and receives input from area CA2 (Chevaleyre and Siegelbaum, 2010), and medial septum (Lovett-Barron et al., 2014). The stratum radiatum (SR), where PN apical dendrites are located, receives excitatory input from CA3 PNs via the Schaffer Collateral (SC) synapses and is embedded with some bistratified dendrite-targeting GABAergic neurons. Lastly, the stratum lacunosum moleculare (SLM), where most distal tuft dendrites are located, receives input from the entorhinal cortex (EC) and has cell bodies of several dendrite-targeting and some soma-targeting INs (Amaral and Witter, 1989; Witter et al., 2000; Basu and Siegelbaum, 2015). The SR/SLM border region is particularly rich in GABAergic neuron cell bodies that control the flow of direct cortical inputs arriving distally upon CA1 PNs and their integration with proximal inputs carrying content processed through the indirect intra-hippocampal trisynaptic circuit (DG → CA3 → CA1). Both the direct EC and indirect trisynaptic pathways have been implicated in learning and memory storage (Barnes et al., 1977; Van Strien et al., 2009; Basu et al., 2013, 2016; Jonas and Lisman, 2014; Eyre and Bartos, 2019; Figure 2).
Figure 2. Hippocampal Formation and area CA1 Circuitry. (A) Schematic representation of coronal section of a rodent brain highlighting the hippocampal region with its sub-areas dentate gyrus (DG), CA3, CA2, and CA1. (B) Stratification of hippocampal area CA1 namely stratum oriens (SO), stratum pyramidale (SP), stratum radiatum (SR), and stratum lacunosum moleculare (SLM). The CA1 pyramidal neuron soma and dendrites organization are shown in blue, and INs in red. Created with BioRender.
GABAergic INs constitute ~10-20% of all neurons in cortex (Bartos et al., 2007; Klausberger and Somogyi, 2008; Caputi et al., 2013) and ~10–15% of all neurons in the hippocampus (Bezaire and Soltesz, 2013; Pelkey et al., 2017). Within the hippocampus, area CA1 contains more than 21 types of GABAergic INs (Klausberger and Somogyi, 2008). Each of these GABAergic IN types can be classified based on single cell transcriptome analyses and according to their morphology, molecular markers, intrinsic physiological properties, postsynaptic target cells, developmental origin, and function in the adult brain (Ascoli et al., 2008; Klausberger and Somogyi, 2008; Le Magueresse and Monyer, 2013; Pelkey et al., 2017; Harris et al., 2018). However, the integrated classification of interneuron types remains a daunting challenge in cellular and circuit neuroscience (Ascoli et al., 2008; Kepecs and Fishell, 2014; Pelkey et al., 2017; Zeng, 2022). Circuit mapping studies have greatly benefitted from classifying INs based on the expression of specific molecular markers, and the development of transgenic animals based on these molecular markers has provided a relatively precise and consistent strategy to label and manipulate major interneuron populations selectively (Taniguchi et al., 2011; Dimidschstein et al., 2016).
In terms of their expression of molecular markers, GABAergic INs have been traditionally categorized as parvalbumin (PV), vasoactive intestinal peptide (VIP), cholecystokinin (CCK), somatostatin (SST), neuropeptide Y (NPY), and neuron-derived neurotrophic factor (NDNF) and/or nitric oxide synthase (nNOS), among others. These interneuron types generally possess characteristic intrinsic properties and short-term synaptic plasticity dynamics due to their unique molecular make up. Moreover, they can perform specific functions in modulating compartment-specific and behaviorally-relevant activity based on their layer specificity location, connectivity onto downstream PNs and responses to neuromodulators (Figure 3). Each of these interneuron populations can be further subdivided based on co-expressed molecular markers, intrinsic properties, morphology, location within a particular CA1 layer, or input–output connectivity [thoroughly reviewed in (Pelkey et al., 2017)]. Here we provide a brief overview of these sub-classes.
Figure 3. Local Inhibitory microcircuits in area CA1. Schematic representation of CA1 microcircuit connectivity, the CA1 pyramidal neuron (blue) is surrounded by local GABAergic microcircuitry: PV+ IN (pink), SST+ IN (yellow), soma-targeting CCK+ basket cell and SR/SLM border CCK IN (light blue), CR+ VIP IN (dark purple), CCK+ VIP+ IN (light purple), and NPY+ IN (gray). Excitatory and inhibitory inputs from EC into SLM of area CA1 shown in green and red, respectively. Inhibitory inputs from the Medial septum (MS) into SLM of area CA1 shown in red.
Parvalbumin-expressing (PV) INs are classically considered to provide perisomatic inhibition onto a neighboring CA1 PN by surrounding its soma with a basket-like axon morphology (Sik et al., 1995; Cope et al., 2002; Pawelzik et al., 2002; Klausberger et al., 2005; Takács et al., 2015; Dudok et al., 2021a). Moreover, PV INs can also have bistratified morphological subtypes that specifically target the proximal apical dendrites to mediate dendritic feed-forward inhibition (FFI; Basu et al., 2013, 2016; Udakis et al., 2020; Bilash et al., 2023; Chamberland et al., 2023). PV INs also participate in FBI circuit motifs. Few recurrent collaterals of CA1 PNs make functional synaptic contacts onto PV basket cells that target basal dendrites in stratum oriens (Ribak et al., 1993; Hu et al., 2014). Furthermore, the apical dendrite targeting OLM neurons, which are a classical FBI also express low levels of parvalbumin (Klausberger, 2009). Recent studies in CA1 show that PV INs particularly target PNs in the deep layer, while being driven by PNs the superficial layer (Lee A. T. et al., 2014). Lastly, parvalbumin is also expressed in axo-axonic interneurons that directly inhibit the axon initial segment of a pyramidal neuron (Pawelzik et al., 2002; Takács et al., 2015). Functionally, fast-spiking PV INs in CA1 are involved in networking oscillations modulating the PN synchrony particularly in the gamma frequency regime (English et al., 2017) and spatial working memory (Murray et al., 2011; Hu et al., 2014; Harris et al., 2018). PV IN synapses undergo inhibitory long-term depression (i-LTD) during theta burst stimulation (TBS) as well as a spike-timing-dependent plasticity (STDP) paradigm, which is mediated via the activation of GABAA receptors and T-type voltage-gated calcium channels (VGCCs; Udakis et al., 2020). In area CA1, the activity of PV INs is modulated by opioids, specifically via the μ-opioid receptors (Glickfeld et al., 2008). Furthermore, neuromodulation of PV INs is mediated by oxytocin, which increases PV IN firing rate through GABAA receptors (Owen et al., 2013) and by D4-dopamine receptors that enhances the CA3 SC input-driven from CA1 to mediate PV FFI upon PNs to suppress SC pathway output (Rosen et al., 2015).
Cholecystokinin-expressing (CCK) INs were classically considered to mediate FBI (Glickfeld and Scanziani, 2006) at the CA1 pyramidal neuron soma because of their slower responses compared to PV basket cells. Furthermore, these INs are known for their asynchronous GABA release (Hefft and Jonas, 2005), where they fail to keep up with fidelity during high-frequency trains of activation. However, recent studies using optogenetic activation and pharmacogenetic silencing (Basu et al., 2013) show that CCK INs are recruited in a FFI manner by the Schaffer Collateral inputs from CA3, as well as by the perforant path inputs from the entorhinal cortex. In fact, CCK INs can mediated robust and fast FFI both at the soma and dendrites to considerably suppress the amplitude of coincident EPSPs. In vivo juxtacellular recordings of single CCK INs in area CA1 reveals their preferred theta phase firing precedes that of CA1 PNs, putting them in a strategic position to modulate place cell firing at the peak of the theta cycle (Klausberger and Somogyi, 2008). Recent in vivo two-photon imaging studies show that CCK INs (Geiller et al., 2020; Dudok et al., 2022; Dudok and Soltesz, 2022) are particularly active during rest or when animals are stationary, creating a potential functional link to quiet, awake behavioral states associated with sharp wave ripples (Buzsáki, 2015). A well-characterized feature of the CCK INs, is their sensitivity to cannabinoid modulation by virtue of the cannabinoid 1 receptors (CB1Rs) they express (Chevaleyre and Castillo, 2003; Glickfeld and Scanziani, 2006; Freund and Katona, 2007; Castillo et al., 2012). Release of retrograde endocannabinoid messengers upon activation and depolarization of CA1 PNs allows for local and rapid suppression of GABA release from CCK basket cells, which are particularly enriched in CB1Rs (Freund and Katona, 2007). This can occur across shorter time scales, e.g., depolarization induced suppression of inhibition (DSI; Wilson et al., 2001; Yoshida et al., 2002) as well as longer time scales, e.g., inhibitory long-term depression (iLTD) time scales (Chevaleyre et al., 2007; Heifets and Castillo, 2009; Castillo et al., 2012; Basu et al., 2013). Notably, the genetic targeting of CCK INs has been challenging considering CCK pre- pro- hormone is widely expressed in glutamatergic pyramidal neurons as well. Thus, targeting CCK INs exclusively requires intersectional strategies (Taniguchi et al., 2011; Basu et al., 2013, 2016). Finally, recent studies suggest that immediate early gene NPAS4 preferentially modulates CCK IN activity and synaptic output during exposure to enriched environments (Hartzell et al., 2018) and contextual fear learning (Sun et al., 2020).
Somatostatin-expressing (SST) INs classically mediate dendritic inhibition onto pyramidal neurons either in a FFI or FBI manner (Oliva et al., 2000; Leão et al., 2012; Lovett-Barron et al., 2012, 2014; Müller and Remy, 2014; Udakis et al., 2020). SST INs in area CA1 consist of two main subpopulations: OLM INs, which mediate FBI (Leão et al., 2012), and bistratified SST INs, which modulate CA3-driven FFI (Lovett-Barron et al., 2012). OLM INs are recruited by spiking CA1 PNs and inhibit the distal dendrites of CA1 PNs. OLM neurons modulate SC input plasticity (Leão et al., 2012) and gate EC inputs that are relevant for contextual fear learning (Cutsuridis et al., 2010; Lovett-Barron et al., 2014). The OLM neurons have been more extensively studied, and can be characterized by the specific expression of nicotinic acetylcholine receptor α2 subunit (CHRNA2; Leão et al., 2012; Lovett-Barron et al., 2014). Their cell bodies are located in the oriens layer with the axons projecting to the stratum lacunosum moleculare, and known to be modulated by cholinergic input (Leão et al., 2012; Lovett-Barron et al., 2014), and the effect of this modulation is amplified by gap junctions interconnecting the OLM cells. SST IN synapses upon CA1 PN have also been shown to undergo LTP during theta burst stimulation (Udakis et al., 2020) through voltage-gated T- and L-type calcium channel modulation. Imaging studies comparing SST IN and PV IN activity in animals navigating between familiar and novel environments, have shown that the activity of SST INs is transiently suppressed when animals are exposed to novel environments to disinhibit dendrites, while somatic inhibition is dialed up by boosting PV IN activity (Sheffield et al., 2017).
Vasoactive intestinal peptide-expressing (VIP) INs generally target other inhibitory neurons, thereby disinhibiting downstream pyramidal neurons (Acsády et al., 1996; Chamberland and Topolnik, 2012). They have an important role in the modulation of distal dendritic inhibition of PNs with specific target of bistratified and oriens INs controlling the firing rate and FFI (Tyan et al., 2014). They are strongly stimulated by cholinergic modulation (Bell et al., 2015; Askew et al., 2019; Ren et al., 2022) through α4β2 nicotinic acetylcholine (Ach) receptors (Bell et al., 2015). VIP INs can be divided into sub-populations based on their additional expression of calretinin (CR), CCK, or muscarinic acetylcholine receptor 2 (Tyan et al., 2014; Francavilla et al., 2018; Guet-McCreight et al., 2020). Genetically targeting these VIP IN subtypes requires intersectional tools, so dissociating their specific functions has been challenging. Nevertheless, a couple of recent studies have used intersectional tools and modeling to dissociate the function of VIP/CR+ and VIP/CCK INs. The VIP/CR+ INs are primarily disinhibitory and target a host of different interneurons types in area CA1, particularly the dendrite-targeting OLM INs (Tyan et al., 2014; Bilash et al., 2023). On the other hand, the CCK-expressing VIP INs can target PNs and mediate perisomatic FFI (Turi et al., 2019; Guet-McCreight et al., 2020; Kullander and Topolnik, 2021) through asynchronous GABA release (Tyan et al., 2014). For detailed information regarding their disinhibitory function please see Disinhibitory GABAergic Circuits section.
Neurogliaform cells (NGF) express neuron-derived neurotrophic factor (NDNF) but can also be defined by NPY and/or nNOS expression (Price et al., 2005; Tricoire et al., 2010; Armstrong et al., 2012; Milstein et al., 2015; Tasic et al., 2016; Guo et al., 2021). NGF INs soma are predominantly restricted to the distal dendritic SLM layer in CA1, with a smaller distinct subset residing at the border of SLM/SR (Capogna, 2011). Given their position, NGF INs are likely predominantly driven by glutamatergic inputs from EC, as well as thalamus. However, NPY+ NGF neurons have been shown to modulate integration of SC and EC inputs (Milstein et al., 2015). A unique feature of NGF INs is their volume transmission of GABA to provide slow-acting inhibition, via activation of both GABAA and GABAB receptors onto neurons within a particular radius (Price et al., 2005, 2008; Tasic et al., 2016; Abs et al., 2018; Mercier et al., 2022).
In conclusion, molecular markers can be a helpful tool to classify the different GABAergic INs and determine their modulation of excitation-inhibition balance. Nevertheless, they can co-express similar molecular markers and have specific locations throughout the various layers of area CA1. For instance, the expression of molecular markers PV, SST, or NPY can overlap in morphologically-defined oriens-lacunosum moleculare (OLM) interneurons or bistratified neurons (Klausberger and Somogyi, 2008; Klausberger, 2009; Katona et al., 2014; Müller and Remy, 2014). This calls for IN classification and nomenclature systems to use a combination of the molecular markers and their localization in laminar stratification of hippocampal area CA1 (Figure 3).
Synaptic inhibition can powerfully influence the dendritic and somatic activity of a pyramidal neuron (PN; Miles et al., 1996; Gidon and Segev, 2012; Müller et al., 2012; Pouille et al., 2013; Marlin and Carter, 2014; Müllner et al., 2015). Arranged in specialized microcircuit motifs, interneurons can provide axonal (Kiss et al., 1996; Dudok et al., 2021b), perisomatic (Pawelzik et al., 2002; Klausberger et al., 2005; Dudok et al., 2021a), or dendritic (Lacaille and Schwartzkroin, 1988; Klausberger, 2009; Lovett-Barron et al., 2012; Müller and Remy, 2014) inhibition onto specific compartments of CA1 pyramidal neurons. Other interneurons specifically target GABAergic interneurons, thereby serving a disinhibitory function within the larger neural circuit in area CA1 (Acsády et al., 1996; Chamberland and Topolnik, 2012). GABAergic synapses that densely surround the perisomatic region of a CA1 PNs strongly modulate somatic output (Pouille and Scanziani, 2001), while those found along the dendritic tree can serve to precisely modulate EC- or CA3-driven dendritic computations (Klausberger, 2009; Lovett-Barron et al., 2012; Basu et al., 2013, 2016; Bloss et al., 2016; Schulz et al., 2018; Bilash et al., 2023; Chamberland et al., 2023). Ultimately, by affecting the entire span of a CA1 pyramidal neuron (Buhl et al., 1994; Glickfeld et al., 2009; Bloss et al., 2016), GABAergic INs can serve as powerful circuit switches to rapidly, precisely, and flexibly shape single-cell and network computations (Lovett-Barron et al., 2012; Royer et al., 2012; Milstein et al., 2015; Basu et al., 2016; Grienberger et al., 2017).
PV INs mediate FFI in area CA1, modulating somatic and axonal activity in an apparent temporarily silent state (Sik et al., 1995; Pawelzik et al., 2002; Takács et al., 2015; Pelkey et al., 2017; Dudok et al., 2021a; Chamberland et al., 2023). On the other hand, CCK IN subpopulations provide FFI onto the somata and dendrites of downstream pyramidal neurons, curbing compartment-specific activity. Although soma-targeting CCK INs basket cells have been more widely investigated (Mátyás et al., 2004; Somogyi et al., 2004; Glickfeld and Scanziani, 2006; Bartos and Elgueta, 2012; Del Pino et al., 2017; Whissell et al., 2019; Dudok et al., 2021a), non-basket cell CCK-expressing INs have been shown to target the dendrites of CA1 PNs (Cope et al., 2002; Pawelzik et al., 2002; Klausberger, 2009) and likely mediate dendritic inhibition (Basu et al., 2016; Bilash et al., 2023). Neurogliaform INs release GABA through volume transmission (Oláh et al., 2009; Armstrong et al., 2012), a non-specific form of neurotransmitter release that affects many downstream neurons within a particular radius, producing a slow, GABAB receptor (GABABR)-mediated inhibitory response (Price et al., 2005, 2008). Additionally, they can form gap junctions with a variety of interneuron types in area CA1 (Zsiros and Maccaferri, 2005; Armstrong et al., 2012). Studies have demonstrated that NDNF INs in area CA1 modulate learning and recall (Guo et al., 2021) and that SST INs in area CA1 modulate input-specific plasticity (Leão et al., 2012), as well as object- and fear-related memory encoding (Siwani et al., 2018). In area CA1, NPY+ neurons receive converging inputs from EC and CA3 (Milstein et al., 2015) and bistratified SST INs mediate CA3-driven FFI (Lovett-Barron et al., 2012), to shape dendritic activity and somatic firing in CA1 PNs. Therefore, NDNF INs and SST INs could feasibly modulate the integration of extrahippocampal and intrahippocampal (CA3) inputs within CA1 PNs dendrites. This may influence input-timing-dependent plasticity mechanisms (Basu et al., 2013). Neurogliaform cells may additionally modulate the soma-dendrite coupling or dendritic excitability in large populations of CA1 PNs, given that they release GABA through volume transmission and given that GABABRs are enriched in the distal dendrites of CA1 PNs (Degro et al., 2015).
As described above, GABAergic INs allow for the precise modulation of pyramidal neuron activity (Basu et al., 2013; Francavilla et al., 2015; Guet-McCreight et al., 2020; Bilash et al., 2023). Within the cortico-hippocampal circuit, long-range excitatory projections from the entorhinal cortex recruit specific inhibitory neurons within the hippocampus to influence local computations. For example, Milstein et al. (2015) found that EC inputs recruit NPY-expressing neurogliaform neurons in area CA1 (Milstein et al., 2015). Using optogenetics to selectively activate projections from each EC subdivision, Li et al. (2017) found that medial entorhinal cortex (MEC) and lateral entorhinal cortex (LEC) inputs target local GABAergic INs in area CA1, including morphologically-defined axo-axonic cells, basket cells, and bistratified cells. This circuit organization likely enables EC subdivisions to drive inhibition onto the axon initial segment, soma, and dendrites of CA1 PNs, respectively (Li et al., 2017). Combining optogenetics and pharmacology, Bilash et al. (2023) found that glutamatergic LEC inputs drive strong amounts of FFI onto both the somatic and dendritic compartments of CA1 PNs by recruiting CCK-expressing INs, while also recruiting disinhibition by exciting local CR co-expressing VIP INs in area CA1 (Bilash et al., 2023).
Beyond FFI and FBI, some particular INs can modulate disinhibition by directly inhibiting other INs and thereby activating the downstream PNs (Basu et al., 2013, 2016; Francavilla et al., 2015, 2018; Guet-McCreight et al., 2020; Bilash et al., 2023). In hippocampal CA1 area, this phenomenon can occur at the local microcircuit level and through long-range inhibitory projections (LRIPs). Locally, VIP INs are classically a disinhibitory IN subtype. However, disinhibitory roles of SST, CCK, and PV INs have also been described (Karson et al., 2009; Chamberland et al., 2023). Across the brain, most LRIPs described to date act in a disinhibitory manner by targeting local INs (Karson et al., 2009; Leão et al., 2012; Melzer et al., 2012; Basu et al., 2016; Pelkey et al., 2017; Artinian and Lacaille, 2018). Here, we provide an updated view of local and long-range disinhibitory GABAergic circuits.
VIP INs are famous for their role in mediating disinhibition in the hippocampus (Acsády et al., 1996; Tyan et al., 2014; Guet-McCreight et al., 2020) and cortex (Lee et al., 2013; Pfeffer et al., 2013; Pi et al., 2013). They inhibit other interneurons, thereby relieving downstream principal cells from inhibitory forces (Chamberland and Topolnik, 2012; Kepecs and Fishell, 2014). Tyan et al. (2014), a pioneering study in hippocampal area CA1 that defined the functional connectivity of the interneuron-specific calretinin co-expressing VIP INs (CR+/VIP+ INs) using paired recordings and optogenetics. This study showed that when synchronously activated these VIP neurons target OLM INs to control their firing rate and timing (Tyan et al., 2014). In a more recent study, Bilash et al. (2023) used intersectional genetics to confirm that CR co-expressing VIP INs (CR+/VIP+ INs) are disinhibitory. These INs are mainly located in SLM and primarily target other INs including, CCK INs and SST INs in area CA1 (Bilash et al., 2023). Whereas the CCK+/VIP+ INs target CA1 PNs and modulate somatic inhibition. Curiously, this study found that both the disinhibitory CR+/VIP+ INs and the inhibitory CCK+/VIP+ INs are recruited by glutamatergic LEC inputs, raising the question of whether these opposing IN subtypes are differentially or simultaneously recruited during behavior to modulate cortico-hippocampal excitation-inhibition dynamics. In vivo, VIP INs are recruited during quiescent states and are weakly active during theta oscillations in locomotion (Magnin et al., 2019; Luo et al., 2020; Kullander and Topolnik, 2021). General optogenetic silencing of VIP INs highlight their role in goal-directed spatial learning (Turi et al., 2019).
SST INs can also play a disinhibitory role within the hippocampal area CA1 to regulate synaptic plasticity and activity of PNs. They make direct contact with PV, VIP, CCK, and CR INs in lacunosum moleculare (LM) as well as with NDNF INs in SR and in LM strata (Katona et al., 1999; Elfant et al., 2008; Leão et al., 2012; Artinian and Lacaille, 2018). Leão et al. (2012), observed that optogenetic stimulation of SST INs in OLM disinhibits SC inputs upon the PNs to increase the magnitude of LTP (Leão et al., 2012). A recent study showed using brief inhibition mediated by optogenetic activation of SST INs persistently interrupts firing of PV INs, so as to disinhibit spike generation in CA1 PNs (Chamberland et al., 2023).
Beyond their classical role in mediating FFI and FBI, CCK and PV INs show bidirectional synaptic coupling. Karson et al. (2009) demonstrated that PV INs anatomically project onto CCK INs and that CCK INs make functional synaptic connections onto PV INs. Such coupling exists at the level of axo-somatic, axo-dendritic, and axo-axonic synapses, between the perisomatic-targeting CCK and PV INs (Karson et al., 2009). This is of great relevance, considering that local disinhibitory circuit motifs can operate with sub-layer specific precision. This is because CCK INs show stronger inhibition upon superficial layer CA1 PNs (Valero et al., 2015), whereas PV INs preferentially target and inhibit the deep layer CA1 PNs (Lee A. T. et al., 2014). The immediate early gene, NPAS4 strengthens CB1R-expressing CCK synapses upon superficial PNs in CA1 in an experience-dependent manner (Hartzell et al., 2018). Hence CCK and PV neurons may orchestrate a fine-tuned modulation of sub-layer specific inhibition and disinhibition between the deep and superficial layer PNs in a behavioral or experience state-dependent manner. More recently, Dudok et al. (2021a) demonstrated that in area CA1, CCK and PV INs are capable of scaling their activities with respect to ensemble neuronal activity in opposite paths but both within brain states and their transitions, leading to a mechanism that PV inhibits the CCK IN activity (Dudok et al., 2021a).
In general, novel tools and transgenic rodents have allowed for the functional and circuit interrogation of the different subtypes of GABAergic neurons that play an important but non-canonical role in disinhibiting the activity of PNs. The favorable impact of rapid and context-selective disinhibitory gating of sensory and mnemonic information flow warrants further investigation into how these disinhibitory microcircuits in hippocampal area CA1 contribute to memory function and adaptive learnt behaviors relying on one trial learning and efficient recall.
While GABAergic inhibitory neurons are typically attributed to maintaining local excitation-inhibition balance and dynamically modulate activity at the microcircuit level, there is surmounting evidence that GABAergic neurons also send long-range inhibitory projections (LRIPs) between different brain areas (Jinno et al., 2007; Melzer et al., 2012; Caputi et al., 2013; Lee A. T. et al., 2014; Basu et al., 2016; Joshi et al., 2017; Melzer and Monyer, 2020; Sans-Dublanc et al., 2020; Szabo et al., 2022; Yen et al., 2022; Schroeder et al., 2023). It has been postulated that LRIPs exist to coordinate activity across longer distances within the brain and are important for generating inter-regional synchrony. An emerging principle for connectivity of LRIPs is that they usually target local GABAergic INs, thereby acting as a disinhibitory system of the principal neurons, found in the downstream target brain region (Melzer et al., 2012; Caputi et al., 2013; Basu et al., 2016; Joshi et al., 2017; Melzer and Monyer, 2020; Malik et al., 2022). Within the cortico-hippocampal circuit, LRIPs from medial and lateral entorhinal cortex (MEC and LEC), medial septum (MS), prefrontal cortex (PFC) and retrosplenial cortex (RS) have been found. In fact, LRIPs mediate bidirectionally connectivity between MEC (Ino et al., 1990; Melzer et al., 2012), MS, PFC and RS (Gulyás et al., 2003; Fanselow and Dong, 2010; Joshi et al., 2017; Yuan et al., 2017) and the hippocampus. Although LRIPs have been found to project from LEC into CA1 (Basu et al., 2016), it has yet to be determined whether similar to glutamatergic cortico-hippocampal loop there exists a GABAergic reciprocal loop counterpart from CA1 to LEC.
Hippocampal area CA1 also sends LRIPs to other sub-cortical regions as amygdala, nucleus accumbens, subiculum and band of Broca (Cohen and Squire, 1980; Jinno et al., 2007; Eichenbaum et al., 2012; Melzer et al., 2012; Caputi et al., 2013; Lee S. H. et al., 2014; Lübkemann et al., 2015; Basu et al., 2016; Witter et al., 2017; Wick et al., 2019). Given the extensive regions that interact with CA1 by sending and receiving GABAergic projections, here we discuss recent findings about the heterogeneity and functionality in circuit computations and episodic memory functions of the different LRIPs.
Within the cortico-hippocampal circuit, most LRIP axons from EC terminate in SR and SLM of hippocampal area CA1, where information is integrated in the distal dendrites of CA1 PNs (Melzer et al., 2012; Basu et al., 2016; Xu et al., 2020). Melzer et al. (2012) reported that MEC sends LRIPs to the dorsal and intermediate hippocampal areas into SLM and deep SR. These MEC LRIPs consist of axons from PV INs but likely include other GABAergic subtypes as well (Melzer et al., 2012). Although retrograde labelling experiments previously uncovered the existence of GABAergic LRIPs from EC into the hippocampus (Germroth et al., 1989), Melzer et al. (2012) was the first to address the molecular composition and functional connectivity of MEC LRIPs, as well as their potential function in modulating local GABAergic neuron theta synchrony in the hippocampus.
Basu et al. (2016) discovered that LEC also sends long-range inhibitory inputs into hippocampal area CA1. Interestingly, in vivo two-photon imaging of a pan-GABAergic neuron Cre line (Gad2-Cre) shows that these LEC LRIPs are strongly activated by behaviorally-salient cues such as water rewards and air-puffs. Neutral sensory cue-driven presynaptic activity in these LEC LRIP boutons is boosted supra-linearly when combined with appetitive or aversive stimuli. Accordingly, LEC LRIPs are important for novelty and contextual salience discrimination: silencing the LEC LRIPs leads to impaired novel object recognition and to the over-generalization of context-dependent fear memory. At the circuit level, LEC LRIPs prominently target Schaffer collateral-associated, dendrite-targeting CCK INs in CA1 to effectively relieve dendritic FFI. This long-range disinhibitory circuit motif facilitates the integration of EC and CA3 inputs within CA1 PNs to boost dendritic spike probability and induce input-timing-dependent plasticity. Interestingly, LRIPs from MEC and LEC are differentially distributed within hippocampal area CA1. MEC LRIPs target mostly proximal area CA1, similar to excitatory MEC projections. Meanwhile, LEC LRIPs distribute uniformly along the proximal-distal axis of CA1, in contrast to the excitatory LEC long range excitatory projections (LREP) that target mostly distal area CA1 (Melzer et al., 2012; Basu et al., 2016). It is therefore possible that long-range GABAergic projections from LEC could serve to modulate the integration of MEC, LEC, and CA3 inputs within CA1 PNs, thereby influencing the output of area CA1. This circuit organization could provide LEC LRIPs with the means to influence contextual and spatial information processing. For example, perhaps the long-range disinhibition of dendritic spikes by this salience detection circuit may contribute to context-dependent spatial tuning or remapping of place cells (Bittner et al., 2015, 2017; Figure 4).
Figure 4. Long-range Inhibitory projections circuits in the hippocampus. Long-range Inhibitory projections (LRIPs) into hippocampal area CA1 and LRIPs outputs from hippocampal area. Inhibition or disinhibition of hippocampal CA1 pyramidal neuron (in blue), with GABAergic inhibitory interneurons (in yellow) and disinhibitory interneurons (in purple). Inputs into hippocampal area CA1 from dentate gyrus granule cells and CA3 pyramidal neurons (in black).
Besides the LRIPs from the entorhinal cortex into the hippocampus, LRIPs have also been found from other brain regions that modulate hippocampal information processing (Joshi et al., 2017; Malik et al., 2022). Joshi et al. (2017) reported a new set of LRIPs neurons, named “Teevra cells,” that originate in the medial septum with general IN targets in CA3 with a few inputs into CA1. Teevra cells target axo-axonic GABAergic neurons (likely PV INs) and CCK INs in hippocampal area CA3. Teevra cells were themselves positive for parvalbumin, as well as for the transcription factor SATB1. These cells increase their rhythmicity during run and rest periods, coincident with heightened excitation in area CA1 (Joshi et al., 2017). Medial septum LRIPs are recruited for recall of contextual fear memory. Photostimulation of these projections, selectively inhibited local PV INs in area CA1, whereas chemogenetic silencing blocked memory retrieval (Sans-Dublanc et al., 2020). Furthermore, Malik et al. (2022) described a new form of LRIPs from PFC to PNs from dorsal CA1. These projections directly synapse onto VIP INs, so activating the PFC LRIPs increases FFI, enhancing the signal-to-noise ratio for hippocampus to encode object locations with increasing spatial information. Furthermore, silencing of these projections suppresses object exploration (Malik et al., 2022; Figure 4).
Once information is integrated in area CA1, hippocampal output is sent back to EC, as well as to various other cortical and sub-cortical areas (Cohen and Squire, 1980; Eichenbaum et al., 2012; Melzer et al., 2012; Basu et al., 2016; Witter et al., 2017). LRIPs from the hippocampus to retrosplenial cortex (Jinno et al., 2007), amygdala (Lübkemann et al., 2015), frontal cortex (Wick et al., 2019) and nucleus accumbens (Lee S. H. et al., 2014) have also been reported. GABAergic LRIPs from CA1 have diverse molecular identities and firing properties (Melzer and Monyer, 2020). GABAergic INs originating in SO of area CA1 project to subiculum and medial septum, and increase the firing during sharp wave ripples (SWR; Jinno et al., 2007; Caputi et al., 2013; Melzer and Monyer, 2020). GABAergic INs originating in SP of area CA1 project to band of Broca and the frontal cortex, express the nNOS and NPY markers and connect to PNs and other local IN subtypes (Wick et al., 2019). GABAergic LRIPs from CA1 also project to retrosplenial cortex (RS), and to MEC, which are part of the brain’s navigation system. Both of these projections include a sub-population expressing somatostatin (Jinno et al., 2007; Miyashita and Rockland, 2007; Melzer et al., 2012). Within the CA1-subiculum circuit, LRIP from VIP INs mostly restricted in stratum oriens (SO), stratum pyramidale (SP) and radiatum (SR) of CA1 target INs in subiculum through gap junctions. These VIP IN LRIP have sparse spiking in vitro but are highly active during quiet wakefulness (Francavilla et al., 2018; Figure 4).
In summary, the cortico-hippocampal network for memory and navigation has bidirectional functional interactions mediated by glutamatergic and GABAergic circuits that can drive excitation, inhibition, and disinhibition in hippocampal area, locally and across long-distances. The diversity of activity-dependent and neuromodulatory tuning of specific subsets of INs contributes to a wide dynamic range for spatio-temporal modulation of balance of excitation-inhibition-disinhibition. This will allow for gating of cortico-hippocampal information flow and CA1 ensemble output by GABAergic circuits in vivo in a behavioral state- or task-selective manner.
Clinical AD is staged across normal, preclinical, mild cognitive impairment (MCI), and dementia stages that are defined by worsening biomarkers, cognition, and function (Jack et al., 2018). These clinical stages are grossly associated with the spatiotemporal progression of AD pathology through the brain. In particular, memory impairment becomes first evident at the pre-dementia MCI stage when AD pathology progresses from EC to CA1, the region of hippocampus first and most affected during the course of AD (Braak and Braak, 1991; Bennett et al., 2005; Lace et al., 2009). Histological analysis has demonstrated that this correlates with synaptic loss and neurodegeneration initiated by this AD pathology in area CA1 (Price et al., 2001; Scheff et al., 2007; Kerchner et al., 2010). Molecular pathways associated with these changes relate to spine integrity, glutamate receptor loss, cellular stress response, inflammation and calcium dyshomeostasis (imbalance of the homeostasis system; Colangelo et al., 2002; Ginsberg et al., 2010, 2012; Counts et al., 2014; Hondius et al., 2016). The advent of transgenic and, more recently, knock-in rodent models of AD has further established a link between various forms of memory deficits and aspects of AD pathology within the hippocampus (Webster et al., 2014). Studies using these models have elucidated neuronal mechanisms that relate to synaptic dysfunction (Pozueta et al., 2013), aberrant synaptic plasticity (Cuestas Torres and Cardenas, 2020), and altered information coding properties (Zhao et al., 2014; Mably et al., 2017; Jun et al., 2020). Human and rodent models studies have also suggested disruption of network activity and oscillations (Zott et al., 2018; Giustiniani et al., 2022).
Excitatory pyramidal neurons have primarily drawn the focus of such cellular-level AD research, as they carry the main output from brain areas and are the principal cell type that develop neurofibrillary tangles (NFTs; Braak and Braak, 1991). Moreover, surmounting evidence of pyramidal neuron heterogeneity has suggested further studies to elucidate how these subgroups are differentially impacted by AD (Masurkar, 2018). However, as delineated above, inhibitory GABAergic neurons play a critical role in shaping and transforming information processing by pyramidal neurons through local and long-range interactions. Pyramidal neuron functional heterogeneity can in part be mediated by differential associations with specific GABAergic neuron subtypes (Lee S. H. et al., 2014; Valero et al., 2015). Therefore, the loss or degeneration of GABAergic neurons due to AD pathology can play a significant role in driving symptomatology. Moreover, reduction of GABAergic tone can increase net excitability of a network. This may impact the development and progression of AD pathology itself, as tau and amyloid can be released by activity and/or exert their effects across synapses (Kamenetz et al., 2003; Cirrito et al., 2005; De Calignon et al., 2012; Liu et al., 2012; Khan et al., 2014; Wu et al., 2016).
Broad interrogation of the GABAergic system in AD has indeed revealed relevant changes that leads to alterations. The temporal lobe in human AD features a reduction of GABAA mRNA and protein, and reduced physiologic function of GABAA receptors (Limon et al., 2012). Rodent AD models also show alteration of GABA function in the hippocampus (Palop et al., 2007) that can associate with disruptions in hippocampal theta oscillations and sharp wave ripples (Mahar et al., 2016; Caccavano et al., 2020), as well as disruption of hippocampal excitation-inhibition balance that impairs spatial learning (Yang et al., 2018). Moreover, recent studies in the APP-KI (amyloid-beta precursor protein-knock in) mice show that GABAergic neurons in area CA1 contribute to 30% of amyloid plaque load, highlighting the bidirectional relationship between GABAergic interneurons (INs) and AD pathology (Rice et al., 2020). While global measures of GABAAR dysfunction and altered excitatory/inhibitory balance were not as prominent in an analysis of human AD hippocampal regions (Scaduto et al., 2023), this does not rule out alterations in specific hippocampal IN populations and/or compensatory changes resulting from such IN-specific changes. Given the heterogeneity of IN function delineated above, here we discuss the cell type specific IN vulnerability in the hippocampus in human patients of AD, as well as in AD mouse models. A summary of these findings are described in Table 1.
PV INs are the most extensively studied of the hippocampal INs in the setting of AD. While overall there is no difference in PV INs counts in AD versus control human cases (Waller et al., 2020), there is region-specific loss of PV INs and/or PV immunoreactivity limited to DG, CA1, and CA2 (Brady and Mufson, 1997; Takahashi et al., 2010). In rodent AD models, PV INs can develop tau pathology (Soler et al., 2017; Siddhartha et al., 2018), and are found to degenerate, with loss in CA1 observed most consistently (Takahashi et al., 2010; Loreth et al., 2012; Silva Albequerque et al., 2015; Verdaguer et al., 2015; Huh et al., 2016; Mahar et al., 2016; Cattaud et al., 2018; Zallo et al., 2018; Giesers and Wirths, 2020; Seo et al., 2021; Li et al., 2022). In contrast, a few studies using different amyloid and tau models show resilience (Verdaguer et al., 2015; Ahnaou et al., 2017; Sos et al., 2020; Morrone et al., 2022). Comparing the CA1 layers, PV INs in stratum pyramidale are most vulnerable to degeneration, followed by those in stratum oriens (Villette et al., 2012; Silva Albequerque et al., 2015; Mahar et al., 2016).
The degenerating or remaining PV INs appear to also have a critical role in modulating excitation-inhibition balance, plasticity, and network oscillations during the course of disease. Loss of excitatory drive to PV INs, in particular from EC Layer 2, leads to excitation-inhibition balance disruption, which can be rescued with optogenetically-driven plasticity (Xiao et al., 2017; Yang et al., 2018). Intrinsically, PV INs show an NaV1.1 channelopathy in the J20 amyloid model, which reduces their function and leads to epileptiform activity in the brain (Verret et al., 2012). With regard to plasticity, PV INs also mediate amyloid-induced suppression of CA3 → CA1 long term potentiation (Huh et al., 2016; Zhang et al., 2017). Oscillatory behavior of the hippocampus is altered in AD models, and amyloid impact on PV interneurons appears to play a critical role (Hollnagel et al., 2019; Chung et al., 2020; Park et al., 2020).
PV INs also appear to have relationship to AD pathology that are intriguing areas for future work. Through their impact on network excitability, PV INs not only promote hyperexcitability, a process that may give rise more pathology, but also can induce vulnerability to further amyloid-induced damage (Hijazi et al., 2019, 2020). Moreover, in APP/PS1 mice (AD model with human amyloid-beta precursor protein and presenilin-1 mutations), PV INs show close interactions with microglia (Gervais et al., 2022). However, another study has called in to question the relationship between PV INs and AD pathogenesis (Mackenzie-Gray Scott et al., 2022). Of note, PV INs also express amyloid-degrading enzymes which would indeed be counterintuitive (Pacheco-Quinto et al., 2016).
While PV INs have been extensively studied in the context of AD, many further questions have arisen. In particular, reconciling how they promote hyperexcitability while also suppressing plasticity is needed to better understand how chronic PV IN dysfunction alters the course of disease. Furthermore, PV INs are themselves heterogeneous, and it is not clear if subtypes are differentially affected. Notably, in 5 × FAD mice, an AD model harboring 5 familial AD mutations, the soma-targeting PV basket cells are affected, reducing their activity during sharp wave ripples, whereas the bistratified and axo-axonic subpopulations were unchanged (Caccavano et al., 2020). Lastly, there has been limited study on the physiologic impact of tauopathy on PV INs.
CCK hormone, highly expressed in the hippocampus, has been suggested to enhance healthy mnemonic functions and serves as a biomarker of cognitive and neuronal integrity in neurodegenerative disease (Plagman et al., 2019; Zhang et al., 2022). There have been few studies specifically examining CCK INs, relative to their extensive study in non-pathologic settings, and these are primarily limited to rodent models featuring or exposed to amyloid pathology. Those examining CCK expression or total number show varied results. Whereas intraventricular injection of amyloid does not alter CCK mRNA levels in the hippocampus (Aguado-Llera et al., 2018), and the number of CCK INs in CA1 (in SO) remained unchanged with intrahippocampal injection of amyloid (Villette et al., 2012), APP/PS1 mice feature a reduction of CB1R-expressing CCK interneurons in DG and CA1 (He et al., 2021). While these differences may arise from exogenous versus endogenous exposure to amyloid, it is also possible that CCK IN subpopulations show a differential vulnerability. In addition, CCK INs appear to undergo physiologic changes to their synaptic drive and overall excitability. In the APP KI model, these interneurons feature signs of reduced inhibitory input (Petrache et al., 2020; Shi et al., 2020) and show early hyperactivity that precedes amyloid accumulation, which is then followed by reduction in GABA production (Shi et al., 2020). The above studies suggest that AD-induced changes in CCK INs function may play a critical role in cognitive deficits and promoting disease progression. Of note, cannabinoids (CB) that target CB1R-expressing CCK INs show promising effects in reducing amyloid plaque deposition and hyperphosphorylation of tau (Aso and Ferrer, 2014; Aguirre-Rueda et al., 2015; Abate et al., 2021; Xiong and Lim, 2021; Khavandi et al., 2023). As such, further studies examining subtypes of CCK INs and their impact on function and pathology are needed. Moreover, the relationship between tau pathology and these interneurons have not been studied, despite suggestion of a relationship in human AD (Lenders et al., 1989). Further study of CCK INs in human AD is also needed.
As a critical modulator of pyramidal neuron dendritic excitability, SST INs have been the focus of several studies. Importantly, there is no difference in SST INs counts in AD and control human cases (Waller et al., 2020), suggesting that they may be largely resilient to neurodegeneration. This finding is recapitulated in the TauPS2APP (Loreth et al., 2012) and hAPP (Palop et al., 2007) rodent models. In contrast, loss of SST mRNA is found with intraventricular injection of amyloid in rat (Aguado-Llera et al., 2018), and SST INs loss appears in the mouse 5xFAD and PS1xAPP and rat TgF344-AD models (Ramos et al., 2006; Li et al., 2022; Morrone et al., 2022). A reason for these discrepancies, beyond choice of animal model, may because SST INs vulnerability is location- and subtype-specific. Indeed, hippocampal amyloid injection causes loss of subset of SST INs in CA1 stratum oriens that do no co-express NPY or PV (Villette et al., 2012). In the TgCRND8 amyloid model, there is a subregion- and lamina-specific decrease of SST-expressing interneurons primarily in stratum oriens of CA1, CA2 and stratum radiatum of CA3 (Silva Albequerque et al., 2015).
SST INs may also play a more active role in AD as other studies have evaluated SST INs physiology and relationship to AD pathogenesis. For example, CA1 SST INs show early hyperactivity in the APP KI model preceding amyloid accumulation, which is then followed by reduction in GABA production (Shi et al., 2020). Relatedly, the impact of A-beta oligomers on hippocampal oscillations is suggested to be mediated by effects on SST INs (Chung et al., 2020; Park et al., 2020). In APP/PS1 mice, SST INs show increased interactions with microglia (Gervais et al., 2022). Interestingly, SST INs may directly modulate AD pathology. SST INs express amyloid-degrading enzymes (Pacheco-Quinto et al., 2016). Examination of human AD pathology suggests a relationship of SST INs to the development of tau pathology (Lenders et al., 1989).
In summary, SST INs present an intriguing target for modulating AD pathophysiology due to their targeting of pyramidal neuron dendrites, partial resilience to neurodegeneration, and potential influence over amyloidosis and tauopathy. Open questions remain over their exact influence on cognitive and behavioral deficits in the context of hippocampal circuits and the efficacy of leveraging this knowledge to improve hippocampal function and counteract AD pathogenesis.
There are several studies examining CR-expressing INs, which often co-express VIP and mediate disinhibition, as discussed above. In human AD hippocampus, loss of CR immunoreactivity is found in DG (Takahashi et al., 2010). In rodent models of AD featuring amyloid or both amyloid and tau, evidence is inconsistent that CR INs degenerate in various hippocampal subregions (Baglietto-Vargas et al., 2010; Takahashi et al., 2010; Loreth et al., 2012; Aguado-Llera et al., 2018; Zallo et al., 2018; Giesers and Wirths, 2020; Shi et al., 2020; Li et al., 2022). Physiologic characterization has demonstrated that in CA1 they have unchanged intrinsic excitability but show hyper-inhibition through upregulated purinoreceptors in an APP KI model (Shi et al., 2020). Given their targeting of interneuron, this associates with pyramidal neuron hyperexcitability. Together, these results imply that CR-expressing INs may be spared during AD in some subregions, and could thus be a potential target for pharmacological and therapeutic intervention. Furthermore, there is promising evidence that VIP peptide secreted by VIP INs alongside GABA contributes to neuroprotection and neurotrophic processes, and inhibits Aβ induced neurodegeneration by suppressing microglial secreted neurotoxic factors (Song et al., 2012; Korkmaz et al., 2019). Further studies are needed to more directly elucidate the impact of altered disinhibition by CR, VIP, and CR+/VIP+ INs populations on hippocampal function and related behaviors.
As mentioned above, NPY expression defines a heterogeneous population of INs that co-express other molecular markers and have unique functions in non-pathologic settings. Most work on NPY interneurons has been centered on quantifying expression levels or neurodegeneration. In human AD there is a reduction in NPY that is most prominent in the DG hilus, CA1, and parasubiculum (Chan-Palay et al., 1986). Intraventricular injection of amyloid in rat reduces NPY mRNA levels in the hippocampus (Aguado-Llera et al., 2018). At a subregion level, the human pattern is largely mimicked in rodent AD models with amyloidosis and dual pathology, with some models also demonstrating CA3 and CA2 vulnerability (Palop et al., 2007; Loreth et al., 2012; Silva Albequerque et al., 2015; Mahar et al., 2016). Additional complexity to alteration of the NPY INs network is suggested by laminar-specific vulnerability, with stratum pyramidale most consistently vulnerable (Villette et al., 2012; Mahar et al., 2016), alterations of NPY-subtype expression (Palop et al., 2007), and findings that NPY-expression identifies SST INs most vulnerable to degeneration (Ramos et al., 2006). Open questions remain on the impact of this region-specific vulnerability on hippocampal function and related behaviors. Furthermore, as NPY can subcategorize other IN types, it is unclear if AD-related physiologic alterations also occur based on NPY expression. Additional work is also needed to investigate possible changes in their excitability and impact of this on plasticity and network activity.
Given their diversity, GABAergic circuits play a wide range of critical roles – from modulation of single-neuron and network activity in a compartment- and layer-specific manner, to acting as gain control switches or knobs to modulate the dynamics between excitation-inhibition-disinhibition, to synchronizing distant brain areas and creating spatio-temporal windows for integration, coincidence-detection and signal-to-noise modulation. As highlighted above, there is surmounting evidence that specific GABAergic circuits in the hippocampus may be involved in the pathology and progression of AD. In this context, several open questions remain regarding their differential engagement with pyramidal neuron subtypes (Masurkar, 2018), their role in dysregulated information processing, plasticity, and network activity, and their impact on behavioral deficits and disease progression. Notably, while their specific role in AD is yet to be explored, the more recently discovered long-range GABAergic projections support inter-regional coordination of activity and context discrimination. Given their disinhibitory role in area CA1, the dysfunction of these LRIPs in AD might disrupt spatial and non-spatial memory processing. These modifications, may elicit hyperactivity and increased plasticity to exacerbate the spread of AD pathology and neurodegeneration. This warrants future studies using mouse models of AD as well as proteomics and transcriptomics-based studies in human patients to examine the pathological vulnerability of specific types of long-range inhibitory projections (regional: MEC vs. LEC; cell-type: SST- vs. PV- vs. VIP-expressing) and their downstream interactions. Such information will be important for correlating their dysfunction with disease pathology and cognitive and physiological phenotypes and identifying potential targets for therapeutic intervention (Figure 5).
Figure 5. Summary of CA1 INs in health and Alzheimer’s disease. (A) Schematic representation of the hippocampal area and a normal pyramidal neuron of CA1 highlighting the INs subtypes and the inhibition and disinhibition processes in healthy circuit. (B) Schematic representation of the hippocampal area and a degenerated pyramidal neuron of CA with amyloid plaques depositions and Tau tangles. In lower panel highlighting the INs subtypes and the dysregulation of hyperexcitability or hypoexcitability processes found in AD. Top panels of (A,B), created with BioRender.
MH-F, AM, and JB: planning and conceptualization. MH-F, OB, AM, and JB: manuscript preparation. MH-F prepared the figures with input from OB and JB. AM prepared the table. All authors contributed to the article and approved the submitted version.
This work was supported by NIH NINDS 1R01NS109362-01, BRAIN INITIATIVE NIH NINDS 1R01NS109994, Mathers Charitable Foundation Investigator Award, McKnight Scholar Award in Neuroscience, Klingenstein-Simons Fellowship Award in Neuroscience, Alfred P. Sloan Research Fellowship, Whitehall Research Grant, American Epilepsy Society Junior Investigator Award, Blas Frangione Young Investigator Research Grant, New York University Whitehead Fellowship for Junior Faculty in Biomedical and Biological Sciences, and Leon Levy Foundation Award to JB. JB was supported by Alzheimer’s Association grant AARGD-NTF-23-1151101, NIH NCATS NYU Clinical and Translational Science Institute (CTSI) Pilot Project: UL1TR001445 and Parekh Center for Interdisciplinary Neurology (PCIN) Pilot Research Program 2023. AM and JB were jointly supported by an NIH RF1AG072507. AM was supported by NIH grants RF1AG072507, R21AG070880, and P30AG066512, Alzheimer’s Association grant AACF-17-524288, BrightFocus Foundation grant A2019602S, and support from the Blas Frangione and Leon Levy Foundations. MH-F was supported by REC Scholar Program of NYU Alzheimer’s Disease Center (ADRC) P30 AG066512 and the Alzheimer’s Association grant 23AARFD-1026841. OB was supported by the NIH 5T32NS86750 and REC Scholar Program of NYU Alzheimer’s Disease Center (ADRC) P30 AG066512.
The authors declare that the research was conducted in the absence of any commercial or financial relationships that could be construed as a potential conflict of interest.
All claims expressed in this article are solely those of the authors and do not necessarily represent those of their affiliated organizations, or those of the publisher, the editors and the reviewers. Any product that may be evaluated in this article, or claim that may be made by its manufacturer, is not guaranteed or endorsed by the publisher.
Abate, G., Uberti, D., and Tambaro, S. (2021). Potential and limits of cannabinoids in Alzheimer’s disease therapy. Biology 10. doi: 10.3390/biology10060542
Abs, E., Poorthuis, R. B., Apelblat, D., Muhammad, K., Pardi, M. B., Enke, L., et al. (2018). Learning-related plasticity in dendrite-targeting layer 1 interneurons. Neuron 100, 684–699.e6. doi: 10.1016/j.neuron.2018.09.001
Acsády, L., Görcs, T. J., and Freund, T. F. (1996). Different populations of vasoactive intestinal polypeptide-immunoreactive interneurons are specialized to control pyramidal cells or interneurons in the hippocampus. Neuroscience 73, 317–334. doi: 10.1016/0306-4522(95)00609-5
Adesnik, H. (2018). Layer-specific excitation/inhibition balances during neuronal synchronization in the visual cortex. J. Physiol. 596, 1639–1657. doi: 10.1113/JP274986
Aguado-Llera, D., Canelles, S., Frago, L. M., Chowen, J. A., Argente, J., Arilla, E., et al. (2018). The protective effects of IGF-I against β-amyloid-related downregulation of hippocampal somatostatinergic system involve activation of Akt and protein kinase a. Neuroscience 374, 104–118. doi: 10.1016/j.neuroscience.2018.01.041
Aguirre-Rueda, D., Guerra-Ojeda, S., Aldasoro, M., Iradi, A., Obrador, E., Mauricio, M. D., et al. (2015). WIN 55, 212-2, agonist of cannabinoid receptors, prevents amyloid β1-42 effects on astrocytes in primary culture. PLoS One 10, 1–42. doi: 10.1371/JOURNAL.PONE.0122843
Ahnaou, A., Moechars, D., Raeymaekers, L., Biermans, R., Manyakov, N. V., Bottelbergs, A., et al. (2017). Emergence of early alterations in network oscillations and functional connectivity in a tau seeding mouse model of Alzheimer’s disease pathology. Sci. Rep. 7, 1–14. doi: 10.1038/s41598-017-13839-6
Amaral, D. G., and Witter, M. P. (1989). The three-dimensional organization of the hippocampal formation: a review of anatomical data. Neuroscience 31, 571–591. doi: 10.1016/0306-4522(89)90424-7
Armstrong, C., Krook-Magnuson, E., and Soltesz, I. (2012). Neurogliaform and ivy cells: a major family of nNOS expressing GABAergic neurons. Front. Neural Circ. 6, 1–10. doi: 10.3389/FNCIR.2012.00023
Artinian, J., and Lacaille, J. C. (2018). Disinhibition in learning and memory circuits: new vistas for somatostatin interneurons and long-term synaptic plasticity. Brain Res. Bull. 141, 20–26. doi: 10.1016/j.brainresbull.2017.11.012
Ascoli, G. A., Alonso-Nanclares, L., Anderson, S. A., Barrionuevo, G., Benavides-Piccione, R., Burkhalter, A., et al. (2008). Petilla terminology: nomenclature of features of GABAergic interneurons of the cerebral cortex. Neuroscience 9, 557–568. doi: 10.1038/nrn2402
Askew, C. E., Lopez, A. J., Wood, M. A., and Metherate, R. (2019). Nicotine excites VIP interneurons to disinhibit pyramidal neurons in auditory cortex. Synapse 73. doi: 10.1002/syn.22116
Aso, E., and Ferrer, I. (2014). Cannabinoids for treatment of Alzheimer’s disease: moving toward the clinic. Front. Pharmacol. 5:37. doi: 10.3389/fphar.2014.00037
Baglietto-Vargas, D., Moreno-Gonzalez, I., Sanchez-Varo, R., Jimenez, S., Trujillo-Estrada, L., Sanchez-Mejias, E., et al. (2010). Calretinin interneurons are early targets of extracellular amyloid-beta pathology in PS1/AbetaPP Alzheimer mice hippocampus. J. Alzheimer’s Dis. 21, 119–132. doi: 10.3233/JAD-2010-100066
Barnes, C. A., Mcnaughton, B. L., Goddard, G. V., Douglas, R. M., and Adamec, R. (1977). Circadian rhythm of synaptic excitability in rat and monkey central nervous system. Science 197, 91–92. doi: 10.1126/science.194313
Bartos, M., and Elgueta, C. (2012). Functional characteristics of parvalbumin- and cholecystokinin-expressing basket cells. J. Physiol. 590, 669–681. doi: 10.1113/jphysiol.2011.226175
Bartos, M., Vida, I., and Jonas, P. (2007). Synaptic mechanisms of synchronized gamma oscillations in inhibitory interneuron networks. Nat. Rev. Neurosci. 8, 45–56. doi: 10.1038/nrn2044
Basu, J., and Siegelbaum, S. A. (2015). The Corticohippocampal circuit, synaptic plasticity, and memory. Cold Spring Harb. Perspect. Biol. 7, 1–27. doi: 10.1101/cshperspect.a021733
Basu, J., Srinivas, K. V., Cheung, S. K., Taniguchi, H., Huang, Z. J., and Siegelbaum, S. A. (2013). A Cortico-hippocampal learning rule shapes inhibitory microcircuit activity to enhance hippocampal information flow. Neuron 79, 1208–1221. doi: 10.1016/j.neuron.2013.07.001
Basu, J., Zaremba, J. D., Cheung, S. K., Hitti, F. L., Zemelman, B. V., Losonczy, A., et al. (2016). Gating of hippocampal activity, plasticity, and memory by entorhinal cortex long-range inhibition. Science 351. doi: 10.1126/science.aaa5694
Bell, L. A., Bell, K. A., and McQuiston, A. R. (2015). Acetylcholine release in mouse hippocampal CA1 preferentially activates inhibitory-selective interneurons via α4β2* nicotinic receptor activation. Front. Cell. Neurosci. 9:134226. doi: 10.3389/FNCEL.2015.00115/BIBTEX
Bennett, D. A., Schneider, J. A., Bienias, J. L., Evans, D. A., and Wilson, R. S. (2005). Mild cognitive impairment is related to Alzheimer disease pathology and cerebral infarctions. Neurology 64, 834–841. doi: 10.1212/01.WNL.0000152982.47274.9E
Bezaire, M. J., Raikov, I., Burk, K., Vyas, D., and Soltesz, I. (2016). Interneuronal mechanisms of hippocampal theta oscillations in a full-scale model of the rodent CA1 circuit. elife 5. doi: 10.7554/ELIFE.18566
Bezaire, M. J., and Soltesz, I. (2013). Quantitative assessment of CA1 local circuits: knowledge base for interneuron-pyramidal cell connectivity. Hippocampus 23, 751–785. doi: 10.1002/hipo.22141
Bilash, O. M., Chavlis, S., Johnson, C. D., Poirazi, P., and Basu, J. (2023). Lateral entorhinal cortex inputs modulate hippocampal dendritic excitability by recruiting a local disinhibitory microcircuit. Cell Rep. 42. doi: 10.1016/j.celrep.2022.111962
Bittner, K. C., Grienberger, C., Vaidya, S. P., Milstein, A. D., Macklin, J. J., Suh, J., et al. (2015). Conjunctive input processing drives feature selectivity in hippocampal CA1 neurons. Nat. Neurosci. 18, 1133–1142. doi: 10.1038/nn.4062
Bittner, K. C., Milstein, A. D., Grienberger, C., Romani, S., and Magee, J. C. (2017). Behavioral time scale synaptic plasticity underlies CA1 place fields. Science 357, 1033–1036. doi: 10.1126/science.aan3846
Bloss, E. B., Cembrowski, M. S., Karsh, B., Colonell, J., Fetter, R. D., and Spruston, N. (2016). Structured dendritic inhibition supports branch-selective integration in CA1 pyramidal cells. Neuron 89, 1016–1030. doi: 10.1016/j.neuron.2016.01.029
Braak, H., and Braak, E. (1991). Neuropathological stageing of Alzheimer-related changes. Acta Neuropathol. 82, 239–259. doi: 10.1007/BF00308809
Brady, D. R., and Mufson, E. J. (1997). Parvalbumin-immunoreactive neurons in the hippocampal formation of Alzheimer’s diseased brain. Neuroscience 80, 1113–1125. doi: 10.1016/S0306-4522(97)00068-7
Bridi, M. C. D., Zong, F. J., Min, X., Luo, N., Tran, T., Qiu, J., et al. (2020). Daily oscillation of the excitation-inhibition balance in visual cortical circuits. Neuron 105, 621–629.e4. doi: 10.1016/j.neuron.2019.11.011
Buhl, E. H., Halasy, K., and Somogyi, P. (1994). Diverse sources of hippocampal unitary inhibitory postsynaptic potentials and the number of synaptic release sites. Nature 368, 823–828. doi: 10.1038/368823a0
Busche, M. A., Kekuš, M., Adelsberger, H., Noda, T., Förstl, H., Nelken, I., et al. (2015). Rescue of long-range circuit dysfunction in Alzheimer’s disease models. Nat. Neurosci. 18, 1623–1630. doi: 10.1038/nn.4137
Busche, M. A., and Konnerth, A. (2016). Impairments of neural circuit function in Alzheimer’s disease. Philos. Trans. R. Soc. Lond., B, Biol. Sci. 371. doi: 10.1098/rstb.2015.0429
Buzsaki, G. (1984). Feed-forward inhibition in the hippocampal formation. Prog. Neurobiol. 22, 131–153. doi: 10.1016/0301-0082(84)90023-6
Buzsáki, G. (2002). Theta oscillations in the hippocampus. Neuron 33, 325–340. doi: 10.1016/S0896-6273(02)00586-X
Buzsáki, G. (2015). Hippocampal sharp wave-ripple: a cognitive biomarker for episodic memory and planning. Hippocampus 25, 1073–1188. doi: 10.1002/hipo.22488
Caccavano, A., Lorenzo Bozzelli, P., Forcelli, P. A., Pak, D. T. S., Wu, J. Y., Conant, K., et al. (2020). Inhibitory Parvalbumin basket cell activity is selectively reduced during hippocampal sharp wave ripples in a mouse model of familial Alzheimer’s disease. J. Neurosci. 40, 5116–5136. doi: 10.1523/JNEUROSCI.0425-20.2020
Capogna, M. (2011). Neurogliaform cells and other interneurons of stratum lacunosum-moleculare gate entorhinal-hippocampal dialogue. J. Physiol. 589, 1875–1883. doi: 10.1113/jphysiol.2010.201004
Caputi, A., Melzer, S., Michael, M., and Monyer, H. (2013). The long and short of GABAergic neurons. Curr. Opin. Neurobiol. 23, 179–186. doi: 10.1016/j.conb.2013.01.021
Castillo, P. E., Younts, T. J., Chávez, A. E., and Hashimotodani, Y. (2012). Endocannabinoid signaling and synaptic function. Neuron 76, 70–81. doi: 10.1016/j.neuron.2012.09.020
Cattaud, V., Bezzina, C., Rey, C. C., Lejards, C., Dahan, L., and Verret, L. (2018). Early disruption of parvalbumin expression and perineuronal nets in the hippocampus of the Tg2576 mouse model of Alzheimer’s disease can be rescued by enriched environment. Neurobiol. Aging 72, 147–158. doi: 10.1016/j.neurobiolaging.2018.08.024
Cembrowski, M. S., Wang, L., Sugino, K., Shields, B. C., and Spruston, N. (2016). Hipposeq: a comprehensive RNA-seq database of gene expression in hippocampal principal neurons. elife 5. doi: 10.7554/ELIFE.14997
Chamberland, S., Nebet, E. R., Valero, M., Hanani, M., Egger, R., Larsen, S. B., et al. (2023). Brief synaptic inhibition persistently interrupts firing of fast-spiking interneurons. Neuron. 111, 1264–1281. doi: 10.1016/j.neuron.2023.01.017
Chamberland, S., and Topolnik, L. (2012). Inhibitory control of hippocampal inhibitory neurons. Front. Neurosci. 6:165. doi: 10.3389/FNINS.2012.00165/BIBTEX
Chan-Palay, V., Lang, W., Haesler, U., Köhler, C., and Yasargil, G. (1986). Distribution of altered hippocampal neurons and axons immunoreactive with antisera against neuropeptide Y in Alzheimer’s-type dementia. J. Comp. Neurol. 248, 376–394. doi: 10.1002/cne.902480307
Chevaleyre, V., and Castillo, P. E. (2003). Heterosynaptic LTD of hippocampal GABAergic synapses: a novel role of endocannabinoids in regulating excitability. Neuron 38, 461–472. doi: 10.1016/S0896-6273(03)00235-6
Chevaleyre, V., Heifets, B. D., Kaeser, P. S., Südhof, T. C., Purpura, D. P., and Castillo, P. E. (2007). Endocannabinoid-mediated long-term plasticity requires cAMP/PKA signaling and RIM1alpha. Neuron 54, 801–812. doi: 10.1016/j.neuron.2007.05.020
Chevaleyre, V., and Siegelbaum, S. A. (2010). Strong CA2 pyramidal neuron synapses define a powerful disynaptic cortico-hippocampal loop. Neuron 66, 560–572. doi: 10.1016/j.neuron.2010.04.013
Chung, H., Park, K., Jang, H. J., Kohl, M. M., and Kwag, J. (2020). Dissociation of somatostatin and parvalbumin interneurons circuit dysfunctions underlying hippocampal theta and gamma oscillations impaired by amyloid β oligomers in vivo. Brain Struct. Funct. 225, 935–954. doi: 10.1007/s00429-020-02044-3
Cirrito, J. R., Yamada, K. A., Finn, M. B., Sloviter, R. S., Bales, K. R., May, P. C., et al. (2005). Synaptic activity regulates interstitial fluid amyloid-beta levels in vivo. Neuron 48, 913–922. doi: 10.1016/j.neuron.2005.10.028
Cohen, N. J., and Squire, L. R. (1980). Preserved learning and retention of pattern-analyzing skill in amnesia: dissociation of knowing how and knowing that. Science 210, 207–210. doi: 10.1126/science.7414331
Colangelo, V., Schurr, J., Ball, M. J., Pelaez, R. P., Bazan, N. G., and Lukiw, W. J. (2002). Gene expression profiling of 12633 genes in Alzheimer hippocampal CA1: transcription and neurotrophic factor down-regulation and up-regulation of apoptotic and pro-inflammatory signaling. J. Neurosci. Res. 70, 462–473. doi: 10.1002/jnr.10351
Cope, D. W., Maccaferri, G., Márton, L. F., Roberts, J. D. B., Cobden, P. M., and Somogyi, P. (2002). Cholecystokinin-immunopositive basket and Schaffer collateral-associated interneurones target different domains of pyramidal cells in the CA1 area of the rat hippocampus. Neuroscience 109, 63–80. doi: 10.1016/S0306-4522(01)00440-7
Counts, S. E., Alldred, M. J., Che, S., Ginsberg, S. D., and Mufson, E. J. (2014). Synaptic gene dysregulation within hippocampal CA1 pyramidal neurons in mild cognitive impairment. Neuropharmacology 79, 172–179. doi: 10.1016/j.neuropharm.2013.10.018
Csicsvari, J., Hirase, H., Czurkó, A., Mamiya, A., and Buzsáki, G. (1999). Oscillatory coupling of hippocampal pyramidal cells and interneurons in the behaving rat. J. Neurosci. 19:274. doi: 10.1523/JNEUROSCI.19-01-00274.1999
Cuestas Torres, D. M., and Cardenas, F. P. (2020). Synaptic plasticity in Alzheimer’s disease and healthy aging. Rev. Neurosci. 31, 245–268. doi: 10.1515/REVNEURO-2019-0058
Cutsuridis, V., Cobb, S., and Graham, B. P. (2010). Encoding and retrieval in a model of the hippocampal CA1 microcircuit. Hippocampus 20, 423–446. doi: 10.1002/hipo.20661
Cutsuridis, V., and Taxidis, J. (2013). Deciphering the role of CA1 inhibitory circuits in sharp wave-ripple complexes. Front. Syst. Neurosci. 7:13. doi: 10.3389/FNSYS.2013.00013
De Calignon, A., Polydoro, M., Suárez-Calvet, M., William, C., Adamowicz, D. H., Kopeikina, K. J., et al. (2012). Propagation of tau pathology in a model of early Alzheimer’s disease. Neuron 73, 685–697. doi: 10.1016/j.neuron.2011.11.033
Degro, C. E., Kulik, A., Booker, S. A., and Vida, I. (2015). Compartmental distribution of GABAB receptor-mediated currents along the somatodendritic axis of hippocampal principal cells. Front. Synap. Neurosci. 7:6. doi: 10.3389/FNSYN.2015.00006
Del Pino, I., Brotons-Mas, J. R., Marques-Smith, A., Marighetto, A., Frick, A., Marín, O., et al. (2017). Abnormal wiring of CCK+ basket cells disrupts spatial information coding. Nat. Neurosci. 20:784. doi: 10.1038/nn.4544
Dimidschstein, J., Chen, Q., Tremblay, R., Rogers, S. L., Saldi, G. A., Guo, L., et al. (2016). A viral strategy for targeting and manipulating interneurons across vertebrate species. Nat. Neurosci. 19:1743. doi: 10.1038/nn.4430
Dudok, B., Klein, P. M., Hwaun, E., Lee, B. R., Yao, Z., Fong, O., et al. (2021a). Alternating sources of perisomatic inhibition during behavior. Neuron 109, 997–1012.e9. doi: 10.1016/J.NEURON.2021.01.003
Dudok, B., Klein, P. M., and Soltesz, I. (2022). Toward understanding the diverse roles of Perisomatic interneurons in epilepsy. Epilepsy Curr. 22:54. doi: 10.1177/15357597211053687
Dudok, B., and Soltesz, I. (2022). Imaging the endocannabinoid signaling system. J. Neurosci. Methods 367:109451. doi: 10.1016/j.jneumeth.2021.109451
Dudok, B., Szoboszlay, M., Paul, A., Klein, P. M., Liao, Z., Hwaun, E., et al. (2021b). Recruitment and inhibitory action of hippocampal axo-axonic cells during behavior. Neuron 109, 3838–3850.e8. doi: 10.1016/J.NEURON.2021.09.033
Eichenbaum, H. (2000). A cortical-hippocampal system for declarative memory. Neuroscience 1, 41–50. doi: 10.1038/35036213
Eichenbaum, H., Sauvage, M., Fortin, N., Komorowski, R., and Lipton, P. (2012). Towards a functional organization of episodic memory in the medial temporal lobe. Neurosci. Biobehav. Rev. 36, 1597–1608. doi: 10.1016/j.neubiorev.2011.07.006
Elfant, D., Pál, B. Z., Emptage, N., and Capogna, M. (2008). Specific inhibitory synapses shift the balance from feedforward to feedback inhibition of hippocampal CA1 pyramidal cells. Eur. J. Neurosci. 27, 104–113. doi: 10.1111/j.1460-9568.2007.06001.x
English, D. F., McKenzie, S., Evans, T., Kim, K., Yoon, E., and Buzsáki, G. (2017). Pyramidal cell-interneuron circuit architecture and dynamics in hippocampal networks. Neuron 96, 505–520.e7. doi: 10.1016/j.neuron.2017.09.033
Evangelista, R., Cano, G., Cooper, C., Schmitz, D., Maier, N., and Kempter, R. (2020). Generation of sharp wave-ripple events by disinhibition. J. Neurosci. 40, 7811–7836. doi: 10.1523/JNEUROSCI.2174-19.2020
Eyre, M. D., and Bartos, M. (2019). Somatostatin-expressing interneurons form axonal projections to the contralateral Hippocampus. Front. Neural Circ. 13:56. doi: 10.3389/fncir.2019.00056
Fanselow, M. S., and Dong, H. W. (2010). Are the dorsal and ventral hippocampus functionally distinct structures? Neuron 65, 7–19. doi: 10.1016/j.neuron.2009.11.031
Ferrante, M., and Ascoli, G. A. (2015). Distinct and synergistic feedforward inhibition of pyramidal cells by basket and bistratified interneurons. Front. Cell. Neurosci. 9:165037. doi: 10.3389/FNCEL.2015.00439/BIBTEX
Francavilla, R., Luo, X., Magnin, E., Tyan, L., and Topolnik, L. (2015). Coordination of dendritic inhibition through local disinhibitory circuits. Front. Synap. Neurosci. 7:5. doi: 10.3389/FNSYN.2015.00005/BIBTEX
Francavilla, R., Villette, V., Luo, X., Chamberland, S., Muñoz-Pino, E., Camiré, O., et al. (2018). Connectivity and network state-dependent recruitment of long-range VIP-GABAergic neurons in the mouse hippocampus. Nat. Commun. 9. doi: 10.1038/s41467-018-07162-5
Freund, T. F., and Katona, I. (2007). Perisomatic inhibition. Neuron 56, 33–42. doi: 10.1016/j.neuron.2007.09.012
Fuchs, E. C., Zivkovic, A. R., Cunningham, M. O., Middleton, S., LeBeau, F. E. N., Bannerman, D. M. M., et al. (2007). Recruitment of parvalbumin-positive interneurons determines hippocampal function and associated behavior. Neuron 53, 591–604. doi: 10.1016/j.neuron.2007.01.031
Geiller, T., Vancura, B., Terada, S., Troullinou, E., Chavlis, S., Tsagkatakis, G., et al. (2020). Large-scale 3D two-photon imaging of molecularly identified CA1 interneuron dynamics in behaving mice. Neuron 108, 968–983.e9. doi: 10.1016/j.neuron.2020.09.013
Germroth, P., Schwerdtfeger, W. K., and Buhl, E. H. (1989). GABAergic neurons in the entorhinal cortex project to the hippocampus. Brain Res. 494, 187–192. doi: 10.1016/0006-8993(89)90162-5
Gervais, É., Iloun, P., Martianova, E., Gonçalves Bessa, A. C., Rivest, S., and Topolnik, L. (2022). Structural analysis of the microglia–interneuron interactions in the CA1 hippocampal area of the APP/PS1 mouse model of Alzheimer’s disease. J. Comp. Neurol. 530, 1423–1437. doi: 10.1002/cne.25289
Gidon, A., and Segev, I. (2012). Principles governing the operation of synaptic inhibition in dendrites. Neuron 75, 330–341. doi: 10.1016/j.neuron.2012.05.015
Giesers, N. K., and Wirths, O. (2020). Loss of hippocampal calretinin and parvalbumin interneurons in the 5XFAD mouse model of Alzheimer’s disease. ASN Neuro 12. doi: 10.1177/1759091420925356
Ginsberg, S. D., Alldred, M. J., and Che, S. (2012). Gene expression levels assessed by CA1 pyramidal neuron and regional hippocampal dissections in Alzheimer’s disease. Neurobiol. Dis. 45, 99–107. doi: 10.1016/j.nbd.2011.07.013
Ginsberg, S. D., Alldred, M. J., Counts, S. E., Cataldo, A. M., Neve, R. L., Jiang, Y., et al. (2010). Microarray analysis of hippocampal CA1 neurons implicates early endosomal dysfunction during Alzheimer’s disease progression. Biol. Psychiatry 68, 885–893. doi: 10.1016/j.biopsych.2010.05.030
Giustiniani, A., Danesin, L., Bozzetto, B., Macina, A., Benavides-Varela, S., and Burgio, F. (2022). Functional changes in brain oscillations in dementia: a review. Rev. Neurosci. 34, 25–47. doi: 10.1515/REVNEURO-2022-0010
Glickfeld, L. L., Atallah, B. V., and Scanziani, M. (2008). Complementary modulation of somatic inhibition by opioids and cannabinoids. J. Neurosci. 28, 1824–1832. doi: 10.1523/JNEUROSCI.4700-07.2008
Glickfeld, L. L., Roberts, J. D., Somogyi, P., and Scanziani, M. (2009). Interneurons hyperpolarize pyramidal cells along their entire somatodendritic axis. Nat. Neurosci. 12, 21–23. doi: 10.1038/nn.2230
Glickfeld, L. L., and Scanziani, M. (2006). Distinct timing in the activity of cannabinoid-sensitive and cannabinoid-insensitive basket cells. Nat. Neurosci. 9:807. doi: 10.1038/nn1688
Grienberger, C., Milstein, A. D., Bittner, K. C., Romani, S., and Magee, J. C. (2017). Inhibitory suppression of heterogeneously tuned excitation enhances spatial coding in CA1 place cells. Nat. Neurosci. 20, 417–426. doi: 10.1038/nn.4486
Guet-McCreight, A., Skinner, F. K., and Topolnik, L. (2020). Common principles in functional organization of VIP/Calretinin cell-driven Disinhibitory circuits across cortical areas. Front. Neural Circ. 14:32. doi: 10.3389/FNCIR.2020.00032/BIBTEX
Gulyás, A. I., Hájos, N., Katona, I., and Freund, T. F. (2003). Interneurons are the local targets of hippocampal inhibitory cells which project to the medial septum. Eur. J. Neurosci. 17, 1861–1872. doi: 10.1046/j.1460-9568.2003.02630.x
Guo, J., Oliveros, H., Jung, O. S., Liang, B., Li, Y., Kavalali, E., et al. (2021). Stratum Lacunosum-moleculare interneurons of the Hippocampus coordinate memory encoding and retrieval. bioRxiv. doi: 10.21203/rs.3.rs-310894/v1
Han, S., Tai, C., Westenbroek, R. E., Yu, F. H., Cheah, C. S., Potter, G. B., et al. (2012). Autistic-like behaviour in Scn1a+/− mice and rescue by enhanced GABA-mediated neurotransmission. Nature 489, 385–390. doi: 10.1038/nature11356
Harris, K. D., Hochgerner, H., Skene, N. G., Magno, L., Katona, L., Bengtsson Gonzales, C., et al. (2018). Classes and continua of hippocampal CA1 inhibitory neurons revealed by single-cell transcriptomics. PLoS Biol. 16:e2006387. doi: 10.1371/journal.pbio.2006387
Hartzell, A. L., Martyniuk, K. M., Brigidi, G. S., Heinz, D. A., Djaja, N. A., Payne, A., et al. (2018). NPAS4 recruits CCK basket cell synapses and enhances cannabinoid-sensitive inhibition in the mouse hippocampus. elife 7. doi: 10.7554/eLife.35927
He, Q., Jiang, L., Zhang, Y., Yang, H., Zhou, C. N., Xie, Y., et al. (2021). Anti-LINGO-1 antibody ameliorates cognitive impairment, promotes adult hippocampal neurogenesis, and increases the abundance of CB1R-rich CCK-GABAergic interneurons in AD mice. Neurobiology of Disease 156:105406. doi: 10.1016/J.NBD.2021.105406
Hefft, S., and Jonas, P. (2005). Asynchronous GABA release generates long-lasting inhibition at a hippocampal interneuron–principal neuron synapse. Nat. Neurosci. 8, 1319–1328. doi: 10.1038/nn1542
Heifets, B. D., and Castillo, P. E. (2009). Endocannabinoid signaling and long-term synaptic plasticity. Annu. Rev. Physiol. 71, 283–306. doi: 10.1146/annurev.physiol.010908.163149
Hijazi, S., Heistek, T. S., Scheltens, P., Neumann, U., Shimshek, D. R., Mansvelder, H. D., et al. (2019). Early restoration of parvalbumin interneuron activity prevents memory loss and network hyperexcitability in a mouse model of Alzheimer’s disease. Mol. Psychiatry 25, 3380–3398. doi: 10.1038/s41380-019-0483-4
Hijazi, S., Heistek, T. S., van der Loo, R., Mansvelder, H. D., Smit, A. B., and van Kesteren, R. E. (2020). Hyperexcitable Parvalbumin interneurons render hippocampal circuitry vulnerable to amyloid Beta. iScience 23. doi: 10.1016/j.isci.2020.101271
Hollnagel, J. O., Elzoheiry, S., Gorgas, K., Kins, S., Beretta, C. A., Kirsch, J., et al. (2019). Early alterations in hippocampal perisomatic GABAergic synapses and network oscillations in a mouse model of Alzheimer’s disease amyloidosis. PLoS One 14. doi: 10.1371/journal.pone.0209228
Hondius, D. C., Van Nierop, P., Li, K. W., Hoozemans, J. J. M., Van Der Schors, R. C., Van Haastert, E. S., et al. (2016). Profiling the human hippocampal proteome at all pathologic stages of Alzheimer’s disease. Alzheimers Dement. 12, 654–668. doi: 10.1016/J.JALZ.2015.11.002
Hu, H., Gan, J., and Jonas, P. (2014). Fast-spiking, parvalbumin+ GABAergic interneurons: from cellular design to microcircuit function. Science 345. doi: 10.1126/science.1255263
Huh, S., Baek, S. J., Lee, K. H., Whitcomb, D. J., Jo, J., Choi, S. M., et al. (2016). The reemergence of long-term potentiation in aged Alzheimer’s disease mouse model. Sci. Rep. 6. doi: 10.1038/srep29152
Ino, T., Matsuzaki, S., Shinonaga, Y., Ohishi, H., Ogawa-Meguro, R., and Mizuno, N. (1990). Direct projections of non-pyramidal neurons of Ammon’s horn to the amygdala and the entorhinal cortex. Neurosci. Lett. 115, 161–166. doi: 10.1016/0304-3940(90)90448-I
Jack, C. R., Bennett, D. A., Blennow, K., Carrillo, M. C., Dunn, B., Haeberlein, S. B., et al. (2018). NIA-AA research framework: toward a biological definition of Alzheimer’s disease. Alzheimer’s Dement. 14, 535–562. doi: 10.1016/j.jalz.2018.02.018
Jinno, S., Klausberger, T., Marton, L. F., Dalezios, Y., Roberts, J. D. B., Fuentealba, P., et al. (2007). Neuronal diversity in GABAergic long-range projections from the hippocampus. J. Neurosci. 27, 8790–8804. doi: 10.1523/JNEUROSCI.1847-07.2007
Jonas, P., and Lisman, J. (2014). Structure, function, and plasticity of hippocampal dentate gyrus microcircuits. Front. Neural Circ. 8:107. doi: 10.3389/fncir.2014.00107
Joshi, A., Salib, M., Viney, T. J., Dupret, D., and Somogyi, P. (2017). Behavior-dependent activity and synaptic Organization of Septo-hippocampal GABAergic neurons selectively targeting the hippocampal CA3 area. Neuron 96, 1342–1357.e5. doi: 10.1016/j.neuron.2017.10.033
Jun, H., Bramian, A., Soma, S., Saito, T., Saido, T. C., and Igarashi, K. M. (2020). Disrupted place cell remapping and impaired grid cells in a knockin model of Alzheimer’s disease. Neuron 107, 1095–1112.e6. doi: 10.1016/j.neuron.2020.06.023
Kamenetz, F., Tomita, T., Hsieh, H., Seabrook, G., Borchelt, D., Iwatsubo, T., et al. (2003). APP processing and synaptic function. Neuron 37, 925–937. doi: 10.1016/S0896-6273(03)00124-7
Karson, M. A., Tang, A. H., Milner, T. A., and Alger, B. E. (2009). Synaptic cross talk between perisomatic-targeting interneuron classes expressing cholecystokinin and parvalbumin in hippocampus. J. Neurosci. 29, 4140–4154. doi: 10.1523/JNEUROSCI.5264-08.2009
Katona, I., Acsády, L., and Freund, T. F. (1999). Postsynaptic targets of somatostatin-immunoreactive interneurons in the rat hippocampus. Neuroscience 88, 37–55. doi: 10.1016/S0306-4522(98)00302-9
Katona, L., Lapray, D., Viney, T. J., Oulhaj, A., Borhegyi, Z., Micklem, B. R., et al. (2014). Sleep and movement differentiates actions of two types of somatostatin-expressing GABAergic interneuron in rat hippocampus. Neuron 82, 872–886. doi: 10.1016/j.neuron.2014.04.007
Kepecs, A., and Fishell, G. (2014). Interneuron cell types are fit to function. Nature 505, 318–326. doi: 10.1038/nature12983
Kerchner, G. A., Hess, C. P., Hammond-Rosenbluth, K. E., Xu, D., Rabinovici, G. D., Kelley, D. A. C., et al. (2010). Hippocampal CA1 apical neuropil atrophy in mild Alzheimer disease visualized with 7-T MRI. Neurology 75, 1381–1387. doi: 10.1212/WNL.0b013e3181f736a1
Khan, U. A., Liu, L., Provenzano, F. A., Berman, D. E., Profaci, C. P., Sloan, R., et al. (2014). Molecular drivers and cortical spread of lateral entorhinal cortex dysfunction in preclinical Alzheimer’s disease. Nat. Neurosci. 17:304. doi: 10.1038/nn.3606
Khavandi, M., Rao, P. P. N., and Beazely, M. A. (2023). Differential effects of endocannabinoids on amyloid-Beta aggregation and toxicity. Int. J. Mol. Sci. 24. doi: 10.3390/ijms24020911
Kiss, J., Buzsaki, G., Morrow, J. S., Glantz, S. B., and Leranth, C. (1996). Entorhinal cortical innervation of Parvalbumin-containing neurons (basket and chandelier cells) in the rat Ammon’s horn. Hippocampus 6, 239–246. doi: 10.1002/(SICI)1098-1063(1996)6:3<239::AID-HIPO3>3.0.CO;2-I
Klausberger, T. (2009). GABAergic interneurons targeting dendrites of pyramidal cells in the CA1 area of the hippocampus. Eur. J. Neurosci. 30, 947–957. doi: 10.1111/j.1460-9568.2009.06913.x
Klausberger, T., Marton, L. F., O’Neill, J., Huck, J. H. J., Dalezios, Y., Fuentealba, P., et al. (2005). Complementary roles of cholecystokinin- and parvalbumin-expressing GABAergic neurons in hippocampal network oscillations. J. Neurosci. 25, 9782–9793. doi: 10.1523/JNEUROSCI.3269-05.2005
Klausberger, T., and Somogyi, P. (2008). Neuronal diversity and temporal dynamics: the unity of hippocampal circuit operations. Science 321, 53–57. doi: 10.1126/science.1149381
Korkmaz, O. T., Ay, H., Aytan, N., Carreras, I., Kowall, N. W., Dedeoglu, A., et al. (2019). Vasoactive intestinal peptide decreases β-amyloid accumulation and prevents brain atrophy in the 5xFAD mouse model of Alzheimer’s disease. J. Mol. Neurosci. 68, 389–396. doi: 10.1007/s12031-018-1226-8
Kullander, K., and Topolnik, L. (2021). Cortical disinhibitory circuits: cell types, connectivity and function. Trends Neurosci. 44, 643–657. doi: 10.1016/j.tins.2021.04.009
Kullmann, D. M. (2011). Interneuron networks in the hippocampus. Curr. Opin. Neurobiol. 21, 709–716. doi: 10.1016/j.conb.2011.05.006
Lacaille, J. C., and Schwartzkroin, P. A. (1988). Stratum lacunosum-moleculare interneurons of hippocampal CA1 region. II. Intrasomatic and intradendritic recordings of local circuit synaptic interactions. J. Neurosci. 8, 1411–1424. doi: 10.1523/JNEUROSCI.08-04-01411.1988
Lace, G., Savva, G. M., Forster, G., De Silva, R., Brayne, C., Matthews, F. E., et al. (2009). Hippocampal tau pathology is related to neuroanatomical connections: an ageing population-based study. Brain J. Neurol. 132, 1324–1334. doi: 10.1093/brain/awp059
Le Magueresse, C., and Monyer, H. (2013). GABAergic interneurons shape the functional maturation of the cortex. Neuron 77, 388–405. doi: 10.1016/j.neuron.2013.01.011
Leão, R. N., Mikulovic, S., Leão, K. E., Munguba, H., Gezelius, H., Enjin, A., et al. (2012). OLM interneurons differentially modulate CA3 and entorhinal inputs to hippocampal CA1 neurons. Nat. Neurosci. 15, 1524–1530. doi: 10.1038/nn.3235
Lee, S., Kruglikov, I., Huang, Z. J., Fishell, G., and Rudy, B. (2013). A disinhibitory circuit mediates motor integration in the somatosensory cortex. Nat. Neurosci. 16:1662. doi: 10.1038/nn.3544
Lee, S. H., Marchionni, I., Bezaire, M., Varga, C., Danielson, N., Lovett-Barron, M., et al. (2014). Parvalbumin-positive basket cells differentiate among hippocampal pyramidal cells. Neuron 82, 1129–1144. doi: 10.1016/j.neuron.2014.03.034
Lee, A. T., Vogt, D., Rubenstein, J. L., and Sohal, V. S. (2014). A class of GABAergic neurons in the prefrontal cortex sends long-range projections to the nucleus Accumbens and elicits acute avoidance behavior. J. Neurosci. 34, 11519–11525. doi: 10.1523/JNEUROSCI.1157-14.2014
Lenders, M. B., Peers, M. C., Tramu, G., Delacourte, A., Defossez, A., Petit, H., et al. (1989). Dystrophic peptidergic neurites in senile plaques of Alzheimer’s disease hippocampus precede formation of paired helical filaments. Brain Res. 481, 344–349. doi: 10.1016/0006-8993(89)90812-3
Li, Y., Xu, J., Liu, Y., Zhu, J., Liu, N., Zeng, W., et al. (2017). A distinct entorhinal cortex to hippocampal CA1 direct circuit for olfactory associative learning. Nat. Neurosci. 20, 559–570. doi: 10.1038/nn.4517
Li, H., Zhao, J., Lai, L., Xia, Y., Wan, C., Wei, S., et al. (2022). Loss of SST and PV positive interneurons in the ventral hippocampus results in anxiety-like behavior in 5xFAD mice. Neurobiol. Aging 117, 165–178. doi: 10.1016/j.neurobiolaging.2022.05.013
Limon, A., Reyes-Ruiz, J. M., and Miledi, R. (2012). Loss of functional GABA a receptors in the Alzheimer diseased brain. Proc. Natl. Acad. Sci. U. S. A. 109, 10071–10076. doi: 10.1073/pnas.1204606109
Liu, L., Drouet, V., Wu, J. W., Witter, M. P., Small, S. A., Clelland, C., et al. (2012). Trans-synaptic spread of tau pathology in vivo. PLoS One 7. doi: 10.1371/journal.pone.0031302
Loreth, D., Ozmen, L., Revel, F. G., Knoflach, F., Wetzel, P., Frotscher, M., et al. (2012). Selective degeneration of septal and hippocampal GABAergic neurons in a mouse model of amyloidosis and tauopathy. Neurobiol. Dis. 47, 1–12. doi: 10.1016/j.nbd.2012.03.011
Lovett-Barron, M., Kaifosh, P., Kheirbek, M. A., Danielson, N., Zaremba, J. D., Reardin, T. R., et al. (2014). Dendritic inhibition in the Hippocampus supports fear learning. Science 343, 857–863. doi: 10.1126/science.1247485
Lovett-Barron, M., Turi, G. F., Kaifosh, P., Lee, P. H., Bolze, F., Sun, X. H., et al. (2012). Regulation of neuronal input transformations by tunable dendritic inhibition. Nat. Neurosci. 15, 423–430. doi: 10.1038/nn.3024
Lübkemann, R., Eberhardt, J., Röhl, F. W., Janitzky, K., Nullmeier, S., Stork, O., et al. (2015). Identification and characterization of GABAergic projection neurons from ventral hippocampus to amygdala. Brain Sci. 5, 299–317. doi: 10.3390/brainsci5030299
Luo, X., Guet-Mccreight, A., Villette, V., Francavilla, R., Marino, B., Chamberland, S., et al. (2020). Synaptic mechanisms underlying the network state-dependent recruitment of VIP-expressing interneurons in the CA1 Hippocampus. Cereb. Cortex 30:3667. doi: 10.1093/cercor/bhz334
Mably, A. J., Gereke, B. J., Jones, D. T., and Colgin, L. L. (2017). Impairments in spatial representations and rhythmic coordination of place cells in the 3xTg mouse model of Alzheimer’s disease. Hippocampus 27, 378–392. doi: 10.1002/hipo.22697
Maccaferri, G. (2005). Stratum oriens horizontal interneurone diversity and hippocampal network dynamics. J. Physiol. 562, 73–80. doi: 10.1113/jphysiol.2004.077081
Mackenzie-Gray Scott, C. A., Pelkey, K. A., Caccavano, A. P., Abebe, D., Lai, M., Black, K. N., et al. (2022). Resilient hippocampal gamma rhythmogenesis and parvalbumin-expressing interneuron function before and after plaque burden in 5xFAD Alzheimer’s disease model. Front. Synap. Neurosci. 14:857608. doi: 10.3389/fnsyn.2022.857608
Magnin, E., Francavilla, R., Amalyan, S., Gervais, E., David, L. S., Luo, X., et al. (2019). Input-specific synaptic location and function of the α5 GABAA receptor subunit in the mouse CA1 hippocampal neurons. J. Neurosci. 39, 788–801. doi: 10.1523/JNEUROSCI.0567-18.2018
Mahar, I., Albuquerque, M. S., Mondragon-Rodriguez, S., Cavanagh, C., Davoli, M. A., Chabot, J. G., et al. (2016). Phenotypic alterations in hippocampal NPY- and PV-expressing interneurons in a Presymptomatic transgenic mouse model of Alzheimer’s disease. Front. Aging Neurosci. 8:327. doi: 10.3389/FNAGI.2016.00327
Malik, R., Li, Y., Schamiloglu, S., and Sohal, V. S. (2022). Top-down control of hippocampal signal-to-noise by prefrontal long-range inhibition. Cells 185, 1602–1617.e17. doi: 10.1016/j.cell.2022.04.001
Marín, O. (2012). Interneuron dysfunction in psychiatric disorders. Nat. Rev. Neurosci. 13, 107–120. doi: 10.1038/nrn3155
Marlin, J. J., and Carter, A. G. (2014). GABA-A receptor inhibition of local calcium signaling in spines and dendrites. J. Neurosci. 34, 15898–15911. doi: 10.1523/JNEUROSCI.0869-13.2014
Masurkar, A. V. (2018). Towards a circuit-level understanding of hippocampal CA1 dysfunction in Alzheimer’s disease across anatomical axes. J. Alzheimer’s Dis. Parkin. 8. doi: 10.4172/2161-0460.1000412
Mátyás, F., Freund, T. F., and Gulyás, A. I. (2004). Convergence of excitatory and inhibitory inputs onto CCK-containing basket cells in the CA1 area of the rat hippocampus. Eur. J. Neurosci. 19, 1243–1256. doi: 10.1111/j.1460-9568.2004.03225.x
Megías, M., Emri, Z., Freund, T. F., and Gulyás, A. I. (2001). Total number and distribution of inhibitory and excitatory synapses on hippocampal CA1 pyramidal cells. Neuroscience 102, 527–540. doi: 10.1016/S0306-4522(00)00496-6
Melzer, S., Michael, M., Caputi, A., Eliava, M., Fuchs, E. C., Whittington, M., et al. (2012). Long-range–projecting GABAergic neurons modulate inhibition in Hippocampus and entorhinal cortex. Science 335, 1506–1510. doi: 10.1126/science.1217139
Melzer, S., and Monyer, H. (2020). Diversity and function of corticopetal and corticofugal GABAergic projection neurons. Nat. Rev. Neurosci. 21, 499–515. doi: 10.1038/s41583-020-0344-9
Mercier, M. S., Magloire, V., Cornford, J. H., and Kullmann, D. M. (2022). Long-term potentiation in neurogliaform interneurons modulates excitation–inhibition balance in the temporoammonic pathway. J. Physiol. 600:4001. doi: 10.1113/JP282753
Miles, R., Tóth, K., Gulyás, A. I., Hájos, N., and Freund, T. F. (1996). Differences between somatic and dendritic inhibition in the hippocampus. Neuron 16, 815–823. doi: 10.1016/S0896-6273(00)80101-4
Milstein, A. D., Bloss, E. B., Apostolides, P. F., Vaidya, S. P., Dilly, G. A., Zemelman, B. V., et al. (2015). Inhibitory gating of input comparison in the CA1 microcircuit. Neuron 87, 1274–1289. doi: 10.1016/j.neuron.2015.08.025
Miyashita, T., and Rockland, K. S. (2007). GABAergic projections from the hippocampus to the retrosplenial cortex in the rat. Eur. J. Neurosci. 26, 1193–1204. doi: 10.1111/j.1460-9568.2007.05745.x
Morrone, C. D., Lai, A. Y., Bishay, J., Hill, M. E., and McLaurin, J. A. (2022). Parvalbumin neuroplasticity compensates for somatostatin impairment, maintaining cognitive function in Alzheimer’s disease. Trans. Neurodegen. 11. doi: 10.1186/s40035-022-00300-6
Müller, M., Faber-Zuschratter, H., Yanagawa, Y., Stork, O., Schwegler, H., and Linke, R. (2012). Synaptology of ventral CA1 and subiculum projections to the basomedial nucleus of the amygdala in the mouse: relation to GABAergic interneurons. Brain Struct. Funct. 217, 5–17. doi: 10.1007/s00429-011-0326-9
Müller, C., and Remy, S. (2014). Dendritic inhibition mediated by O-LM and bistratified interneurons in the hippocampus. Front. Synap. Neurosci. 6:23. doi: 10.3389/FNSYN.2014.00023
Müllner, F. E., Wierenga, C. J., and Bonhoeffer, T. (2015). Precision of inhibition: dendritic inhibition by individual GABAergic synapses on hippocampal pyramidal cells is confined in space and time. Neuron 87, 576–589. doi: 10.1016/j.neuron.2015.07.003
Murray, A. J., Sauer, J. F., Riedel, G., McClure, C., Ansel, L., Cheyne, L., et al. (2011). Parvalbumin-positive CA1 interneurons are required for spatial working but not for reference memory. Nat. Neurosci. 14, 297–299. doi: 10.1038/nn.2751
Noguchi, A., Huszár, R., Morikawa, S., Buzsáki, G., and Ikegaya, Y. (2022). Inhibition allocates spikes during hippocampal ripples. Nat. Commun. 13, 1–14. doi: 10.1038/s41467-022-28890-9
O’Donnell, C., Tiago Gonçalves, J., Portera-Cailliau, C., and Sejnowski, T. J. (2017). Beyond excitation/inhibition imbalance in multidimensional models of neural circuit changes in brain disorders. elife 6. doi: 10.7554/eLife.26724
Oláh, S., Füle, M., Komlósi, G., Varga, C., Báldi, R., Barzó, P., et al. (2009). Regulation of cortical microcircuits by unitary GABAergic volume transmission. Nature 461:1278. doi: 10.1038/nature08503
Oliva, A. A., Jiang, M., Lam, T., Smith, K. L., and Swann, J. W. (2000). Novel hippocampal interneuronal subtypes identified using transgenic mice that express green fluorescent protein in GABAergic interneurons. J. Neurosci. 20:3354. doi: 10.1523/JNEUROSCI.20-09-03354.2000
Owen, S. F., Tuncdemir, S. N., Bader, P. L., Tirko, N. N., Fishell, G., and Tsien, R. W. (2013). Oxytocin enhances hippocampal spike transmission by modulating fast-spiking interneurons. Nature 500, 458–462. doi: 10.1038/nature12330
Pacheco-Quinto, J., Eckman, C. B., and Eckman, E. A. (2016). Major amyloid-β-degrading enzymes, endothelin-converting enzyme-2 and neprilysin, are expressed by distinct populations of GABAergic interneurons in hippocampus and neocortex. Neurobiol. Aging 48, 83–92. doi: 10.1016/j.neurobiolaging.2016.08.011
Palop, J. J., Chin, J., Roberson, E. D., Wang, J., Thwin, M. T., Bien-Ly, N., et al. (2007). Aberrant excitatory neuronal activity and compensatory remodeling of inhibitory hippocampal circuits in mouse models of Alzheimer’s disease. Neuron 55:697. doi: 10.1016/j.neuron.2007.07.025
Park, K., Lee, J., Jang, H. J., Richards, B. A., Kohl, M. M., and Kwag, J. (2020). Optogenetic activation of parvalbumin and somatostatin interneurons selectively restores theta-nested gamma oscillations and oscillation-induced spike timing-dependent long-term potentiation impaired by amyloid β oligomers. BMC Biol. 18. doi: 10.1186/s12915-019-0732-7
Pawelzik, H., Hughes, D. I., and Thomson, A. M. (2002). Physiological and morphological diversity of immunocytochemically defined parvalbumin- and cholecystokinin-positive interneurones in CA1 of the adult rat hippocampus. J. Comp. Neurol. 443, 346–367. doi: 10.1002/cne.10118
Pelkey, K. A., Chittajallu, R., Craig, M. T., Tricoire, L., Wester, J. C., and McBain, C. J. (2017). Hippocampal gabaergic inhibitory interneurons. Physiol. Rev. 97, 1619–1747. doi: 10.1152/physrev.00007.2017
Petrache, A. L., Khan, A. A., Nicholson, M. W., Monaco, A., Kuta-Siejkowska, M., Haider, S., et al. (2020). Selective modulation of α5 GABAA receptors exacerbates aberrant inhibition at key hippocampal neuronal circuits in APP mouse model of Alzheimer’s disease. Front. Cell. Neurosci. 14:568194. doi: 10.3389/fncel.2020.568194
Pfeffer, C. K., Xue, M., He, M., Huang, Z. J., and Scanziani, M. (2013). Inhibition of inhibition in visual cortex: the logic of connections between molecularly distinct interneurons. Nat. Neurosci. 16:1068. doi: 10.1038/nn.3446
Pi, H. J., Hangya, B., Kvitsiani, D., Sanders, J. I., Huang, Z. J., and Kepecs, A. (2013). Cortical interneurons that specialize in disinhibitory control. Nature 503:521. doi: 10.1038/nature12676
Plagman, A., Hoscheidt, S., McLimans, K. E., Klinedinst, B., Pappas, C., Anantharam, V., et al. (2019). Cholecystokinin and Alzheimer’s disease: a biomarker of metabolic function, neural integrity, and cognitive performance. Neurobiol. Aging 76, 201–207. doi: 10.1016/j.neurobiolaging.2019.01.002
Pouille, F., and Scanziani, M. (2001). Enforcement of temporal fidelity in pyramidal cells by somatic feed-forward inhibition. Science 293, 1159–1163. doi: 10.1126/science.1060342
Pouille, F., Watkinson, O., Scanziani, M., and Trevelyan, A. J. (2013). The contribution of synaptic location to inhibitory gain control in pyramidal cells. Phys. Rep. 1:67. doi: 10.1002/PHY2.67
Pozueta, J., Lefort, R., and Shelanski, M. L. (2013). Synaptic changes in Alzheimer’s disease and its models. Neuroscience 251, 51–65. doi: 10.1016/j.neuroscience.2012.05.050
Price, C. J., Cauli, B., Kovacs, E. R., Kulik, A., Lambolez, B., Shigemoto, R., et al. (2005). Neurogliaform neurons form a novel inhibitory network in the hippocampal CA1 area. J. Neurosci. 25:6775. doi: 10.1523/JNEUROSCI.1135-05.2005
Price, J. L., Ko, A. I., Wade, M. J., Tsou, S. K., McKeel, D. W., and Morris, J. C. (2001). Neuron number in the entorhinal cortex and CA1 in preclinical Alzheimer disease. Arch. Neurol. 58, 1395–1402. doi: 10.1001/archneur.58.9.1395
Price, C. J., Scott, R., Rusakov, D. A., and Capogna, M. (2008). GABAB receptor modulation of feedforward inhibition through hippocampal neurogliaform cells. J. Neurosci. 28:6974. doi: 10.1523/JNEUROSCI.4673-07.2008
Ramos, B., Baglietto-Vargas, D., del Rio, J. C., Moreno-Gonzalez, I., Santa-Maria, C., Jimenez, S., et al. (2006). Early neuropathology of somatostatin/NPY GABAergic cells in the hippocampus of a PS1xAPP transgenic model of Alzheimer’s disease. Neurobiol. Aging 27, 1658–1672. doi: 10.1016/j.neurobiolaging.2005.09.022
Ren, C., Peng, K., Yang, R., Liu, W., Liu, C., and Komiyama, T. (2022). Global and subtype-specific modulation of cortical inhibitory neurons regulated by acetylcholine during motor learning. Neuron 110, 2334–2350.e8. doi: 10.1016/j.neuron.2022.04.031
Ribak, C. E., Seress, L., and Leranth, C. (1993). Electron microscopic immunocytochemical study of the distribution of parvalbumin-containing neurons and axon terminals in the primate dentate gyrus and Ammon’s horn. J. Comp. Neurol. 327, 298–321. doi: 10.1002/cne.903270211
Rice, H. C., Marcassa, G., Chrysidou, I., Horré, K., Young-Pearse, T. L., Müller, U. C., et al. (2020). Contribution of GABAergic interneurons to amyloid-β plaque pathology in an APP knock-in mouse model. Mol. Neurodegener. 15, 1–8. doi: 10.1186/S13024-019-0356-Y/FIGURES/3
Rosen, Z. B., Cheung, S., and Siegelbaum, S. A. (2015). Midbrain dopamine neurons bidirectionally regulate CA3-CA1 synaptic drive. Nat. Neurosci. 18, 1763–1771. doi: 10.1038/nn.4152
Royer, S., Zemelman, B. V., Losonczy, A., Kim, J., Chance, F., Magee, J. C., et al. (2012). Control of timing, rate and bursts of hippocampal place cells by dendritic and somatic inhibition. Nat. Neurosci. 15, 769–775. doi: 10.1038/nn.3077
Sans-Dublanc, A., Razzauti, A., Desikan, S., Pascual, M., Monyer, H., and Sindreu, C. (2020). Septal GABAergic inputs to CA1 govern contextual memory retrieval. Sci. Adv. 6:5003. doi: 10.1126/sciadv.aba5003
Scaduto, P., Lauterborn, J. C., Cox, C. D., Fracassi, A., Zeppillo, T., Gutierrez, B. A., et al. (2023). Functional excitatory to inhibitory synaptic imbalance and loss of cognitive performance in people with Alzheimer’s disease neuropathologic change. Acta Neuropathol. 145, 303–324. doi: 10.1007/s00401-022-02526-0
Scheff, S. W., Price, D. A., Schmitt, F. A., Dekosky, S. T., and Mufson, E. J. (2007). Synaptic alterations in CA1 in mild Alzheimer disease and mild cognitive impairment. Neurology 68, 1501–1508. doi: 10.1212/01.wnl.0000260698.46517.8f
Schlingloff, D., Káli, S., Freund, T. F., Hájos, N., and Gulyás, A. I. (2014). Mechanisms of sharp wave initiation and ripple generation. J. Neurosci. 34:11385. doi: 10.1523/JNEUROSCI.0867-14.2014
Schroeder, A., Pardi, M. B., Keijser, J., Dalmay, T., Groisman, A. I., Schuman, E. M., et al. (2023). Inhibitory top-down projections from zona incerta mediate neocortical memory. Neuron 111, 727–738.e8. doi: 10.1016/j.neuron.2022.12.010
Schulz, J. M., Knoflach, F., Hernandez, M. C., and Bischofberger, J. (2018). Dendrite-targeting interneurons control synaptic NMDA-receptor activation via nonlinear α5-GABAA receptors. Nat. Commun. 9, 1–16. doi: 10.1038/s41467-018-06004-8
Seo, H. J., Park, J. E., Choi, S. M., Kim, T., Cho, S. H., Lee, K. H., et al. (2021). Inhibitory neural Network’s impairments at hippocampal CA1 LTP in an aged transgenic mouse model of Alzheimer’s disease. Int. J. Mol. Sci. 22, 1–15. doi: 10.3390/IJMS22020698
Sheffield, M. E. J., Adoff, M. D., and Dombeck, D. A. (2017). Increased prevalence of calcium transients across the dendritic arbor during place field formation. Neuron 96:490. doi: 10.1016/j.neuron.2017.09.029
Shi, A., Petrache, A. L., Shi, J., and Ali, A. B. (2020). Preserved Calretinin interneurons in an app model of Alzheimer’s disease disrupt hippocampal inhibition via upregulated P2Y1 purinoreceptors. Cereb. Cortex 30, 1272–1290. doi: 10.1093/cercor/bhz165
Siddhartha, M. R., Anahi, S. G., Perla, G. P., Macías, M., Ordaz, B., Fernando, P. O., et al. (2018). Phosphorylation of tau protein correlates with changes in hippocampal theta oscillations and reduces hippocampal excitability in Alzheimer’s model. J. Biol. Chem. 293, 8462–8472. doi: 10.1074/jbc.RA117.001187
Sik, A., Penttonen, M., Ylinen, A., and Buzsáki, G. (1995). Hippocampal CA1 interneurons: an in vivo intracellular labeling study. J. Neurosci. 15, 6651–6665. doi: 10.1523/JNEUROSCI.15-10-06651.1995
Silva Albequerque, M., Mahar, I., Davoli, M. A., Chabot, J. G., Mechawar, N., Quirion, R., et al. (2015). Regional and sub-regional differences in hippocampal GABAergic neuronal vulnerability in the TgCRND8 mouse model of Alzheimer’s disease. Front. Aging Neurosci. 7:30. doi: 10.3389/FNAGI.2015.00030
Siwani, S., França, A. S. C., Mikulovic, S., Reis, A., Hilscher, M. M., Edwards, S. J., et al. (2018). OLMα2 cells bidirectionally modulate learning. Neuron 99, 404–412.e3. doi: 10.1016/j.neuron.2018.06.022
Soler, H., Dorca-Arévalo, J., González, M., Rubio, S. E., Ávila, J., Soriano, E., et al. (2017). The GABAergic septohippocampal connection is impaired in a mouse model of tauopathy. Neurobiol. Aging 49, 40–51. doi: 10.1016/j.neurobiolaging.2016.09.006
Somogyi, J., Baude, A., Omori, Y., Shimizu, H., El Mestikawy, S., Fukaya, M., et al. (2004). GABAergic basket cells expressing cholecystokinin contain vesicular glutamate transporter type3 (VGLUT3) in their synaptic terminals in hippocampus and isocortex of the rat. Eur. J. Neurosci. 19, 552–569. doi: 10.1111/j.0953-816X.2003.03091.x
Somogyi, P., Katona, L., Klausberger, T., Lasztóczi, B., and Viney, T. J. (2014). Temporal redistribution of inhibition over neuronal subcellular domains underlies state-dependent rhythmic change of excitability in the hippocampus. Philos. Trans. R. Soc. Lond., B, Biol. Sci. 369. doi: 10.1098/rstb.2012.0518
Song, M., Xiong, J., Wang, Y. Y., Tang, J., Zhang, B., and Bai, Y. (2012). VIP enhances phagocytosis of fibrillar beta-amyloid by microglia and attenuates amyloid deposition in the brain of APP/PS1 mice. PLoS One 7. doi: 10.1371/journal.pone.0029790
Sos, K. E., Mayer, M. I., Takács, V. T., Major, A., Bardóczi, Z., Beres, B. M., et al. (2020). Amyloid β induces interneuron-specific changes in the hippocampus of APPNL-F mice. PLoS One 15. doi: 10.1371/journal.pone.0233700
Squire, L. R. (2004). Memory systems of the brain: a brief history and current perspective. Neurobiol. Learn. Mem. 82, 171–177. doi: 10.1016/j.nlm.2004.06.005
Stark, E., Roux, L., Eichler, R., Senzai, Y., Royer, S., and Buzsáki, G. (2014). Pyramidal cell-interneuron interactions underlie hippocampal ripple oscillations. Neuron 83, 467–480. doi: 10.1016/j.neuron.2014.06.023
Sun, X., Bernstein, M. J., Meng, M., Rao, S., Sørensen, A. T., Yao, L., et al. (2020). Functionally distinct neuronal ensembles within the memory engram. Cells 181:410. doi: 10.1016/j.cell.2020.02.055
Szabo, G. G., Farrell, J. S., Dudok, B., Hou, W. H., Ortiz, A. L., Varga, C., et al. (2022). Ripple-selective GABAergic projection cells in the hippocampus. Neuron 110, 1959–1977.e9. doi: 10.1016/j.neuron.2022.04.002
Takács, V. T., Szőnyi, A., Freund, T. F., Nyiri, G., and Gulyás, A. I. (2015). Quantitative ultrastructural analysis of basket and axo-axonic cell terminals in the mouse hippocampus. Brain Struct. Funct. 220, 919–940. doi: 10.1007/s00429-013-0692-6
Takahashi, H., Brasnjevic, I., Rutten, B. P. F., Van Der Kolk, N., Perl, D. P., Bouras, C., et al. (2010). Hippocampal interneuron loss in an APP/PS1 double mutant mouse and in Alzheimer’s disease. Brain Struct. Funct. 214:145. doi: 10.1007/S00429-010-0242-4
Taniguchi, H., He, M., Wu, P., Kim, S., Paik, R., Sugino, K., et al. (2011). A resource of Cre driver lines for genetic targeting of GABAergic neurons in cerebral cortex. Neuron 71, 995–1013. doi: 10.1016/j.neuron.2011.07.026
Tasic, B., Menon, V., Nguyen, T. N., Kim, T. K., Jarsky, T., Yao, Z., et al. (2016). Adult mouse cortical cell taxonomy by single cell transcriptomics. Nat. Neurosci. 19:335. doi: 10.1038/nn.4216
Tremblay, R., Lee, S., and Rudy, B. (2016). GABAergic interneurons in the neocortex: from cellular properties to circuits. Neuron 91:260. doi: 10.1016/j.neuron.2016.06.033
Tricoire, L., Pelkey, K. A., Daw, M. I., Sousa, V. H., Miyoshi, G., Jeffries, B., et al. (2010). Common origins of hippocampal ivy and Nitric oxide synthase expressing Neurogliaform cells. J. Neurosci. 30:2165. doi: 10.1523/JNEUROSCI.5123-09.2010
Turi, G. F., Li, W. K., Chavlis, S., Pandi, I., O’Hare, J., Priestley, J. B., et al. (2019). Vasoactive intestinal polypeptide-expressing interneurons in the hippocampus support goal-oriented spatial learning. Neuron 101, 1150–1165.e8. doi: 10.1016/j.neuron.2019.01.009
Tyan, L., Chamberland, S., Magnin, E., Camiré, O., Francavilla, R., Suzanne David, L., et al. (2014). Dendritic inhibition provided by interneuron-specific cells controls the firing rate and timing of the hippocampal feedback inhibitory circuitry. J. Neurosci. 34, 4534–4547. doi: 10.1523/JNEUROSCI.3813-13.2014
Udakis, M., Pedrosa, V., Chamberlain, S. E. L., Clopath, C., and Mellor, J. R. (2020). Interneuron-specific plasticity at parvalbumin and somatostatin inhibitory synapses onto CA1 pyramidal neurons shapes hippocampal output. Nat. Commun. 11. doi: 10.1038/s41467-020-18074-8
Valero, M., Cid, E., Averkin, R. G., Aguilar, J., Sanchez-Aguilera, A., Viney, T. J., et al. (2015). Determinants of different deep and superficial CA1 pyramidal cell dynamics during sharp-wave ripples. Nat. Neurosci. 18, 1281–1290. doi: 10.1038/nn.4074
Van Strien, N. M., Cappaert, N. L. M., and Witter, M. P. (2009). The anatomy of memory: an interactive overview of the parahippocampal–hippocampal network. Nat. Rev. Neurosci. 10, 272–282. doi: 10.1038/nrn2614
Verdaguer, E., Brox, S., Petrov, D., Olloquequi, J., Romero, R., de Lemos, M. L., et al. (2015). Vulnerability of calbindin, calretinin and parvalbumin in a transgenic/knock-in APPswe/PS1dE9 mouse model of Alzheimer disease together with disruption of hippocampal neurogenesis. Exp. Gerontol. 69, 176–188. doi: 10.1016/j.exger.2015.06.013
Verret, L., Mann, E. O., Hang, G. B., Barth, A. M. I., Cobos, I., Ho, K., et al. (2012). Inhibitory interneuron deficit links altered network activity and cognitive dysfunction in Alzheimer model. Cells 149, 708–721. doi: 10.1016/j.cell.2012.02.046
Villette, V., Poindessous-Jazat, F., Bellessort, B., Roullot, E., Peterschmitt, Y., Epelbaum, J., et al. (2012). A new neuronal target for beta-amyloid peptide in the rat hippocampus. Neurobiol. Aging 33, 1126.e1–1126.e14. doi: 10.1016/j.neurobiolaging.2011.11.024
Waller, R., Mandeya, M., Viney, E., Simpson, J. E., and Wharton, S. B. (2020). Histological characterization of interneurons in Alzheimer’s disease reveals a loss of somatostatin interneurons in the temporal cortex. Neuropathology 40, 336–346. doi: 10.1111/neup.12649
Webster, S. J., Bachstetter, A. D., Nelson, P. T., Schmitt, F. A., and Van Eldik, L. J. (2014). Using mice to model Alzheimer’s dementia: an overview of the clinical disease and the preclinical behavioral changes in 10 mouse models. Front. Genet. 5:88. doi: 10.3389/FGENE.2014.00088
Whissell, P. D., Bang, J. Y., Khan, I., Xie, Y. F., Parfitt, G. M., Grenon, M., et al. (2019). Selective activation of cholecystokinin-expressing GABA (CCK-GABA) neurons enhances memory and cognition. eNeuro 6. doi: 10.1523/ENEURO.0360-18.2019
Wick, Z. C., Tetzlaff, M. R., and Krook-Magnuson, E. (2019). Novel long-range inhibitory nNOS-expressing hippocampal cells. elife 8. doi: 10.7554/eLife.46816
Wilson, R. I., Kunos, G., and Nicoll, R. A. (2001). Presynaptic specificity of endocannabinoid signaling in the hippocampus. Neuron 31, 453–462. doi: 10.1016/S0896-6273(01)00372-5
Witter, M. P., Doan, T. P., Jacobsen, B., Nilssen, E. S., and Ohara, S. (2017). Architecture of the entorhinal cortex a review of entorhinal anatomy in rodents with some comparative notes. Front. Syst. Neurosci. 11:46. doi: 10.3389/fnsys.2017.00046
Witter, M. P., Wouterlood, F. G., Naber, P. A., and Van Haeften, T. (2000). Anatomical Organization of the Parahippocampal-Hippocampal Network. Ann. N. Y. Acad. Sci. 911, 1–24. doi: 10.1111/J.1749-6632.2000.TB06716.X
Wu, J. W., Hussaini, S. A., Bastille, I. M., Rodriguez, G. A., Mrejeru, A., Rilett, K., et al. (2016). Neuronal activity enhances tau propagation and tau pathology in vivo. Nat. Neurosci. 19:1085. doi: 10.1038/nn.4328
Wulff, P., Ponomarenko, A. A., Bartos, M., Korotkova, T. M., Fuchs, E. C., Bähner, F., et al. (2009). Hippocampal theta rhythm and its coupling with gamma oscillations require fast inhibition onto parvalbumin-positive interneurons. Proc. Natl. Acad. Sci. U. S. A. 106, 3561–3566. doi: 10.1073/pnas.0813176106
Xiao, M. F., Xu, D., Craig, M. T., Pelkey, K. A., Chien, C. C., Shi, Y., et al. (2017). NPTX2 and cognitive dysfunction in Alzheimer’s disease. elife 6, 1–27. doi: 10.7554/ELIFE.23798
Xiong, Y., and Lim, C. S. (2021). Understanding the modulatory effects of Cannabidiol on Alzheimer’s disease. Brain Sci. 11. doi: 10.3390/brainsci11091211
Xu, Y., Zhao, M., Han, Y., and Zhang, H. (2020). GABAergic inhibitory interneuron deficits in Alzheimer’s disease: implications for treatment. Front. Neurosci. 14:660. doi: 10.3389/fnins.2020.00660
Xue, M., Atallah, B. V., and Scanziani, M. (2014). Equalizing excitation-inhibition ratios across visual cortical neurons. Nature 511, 596–600. doi: 10.1038/nature13321
Yang, X., Yao, C., Tian, T., Li, X., Yan, H., Wu, J., et al. (2018). A novel mechanism of memory loss in Alzheimer’s disease mice via the degeneration of entorhinal-CA1 synapses. Mol. Psychiatry 23, 199–210. doi: 10.1038/mp.2016.151
Yen, T. Y., Huang, X., MacLaren, D. A. A., Schlesiger, M. I., Monyer, H., and Lien, C. C. (2022). Inhibitory projections connecting the dentate gyri in the two hemispheres support spatial and contextual memory. Cell Rep. 39. doi: 10.1016/j.celrep.2022.110831
Yoshida, T., Hashimoto, K., Zimmer, A., Maejima, T., Araishi, K., and Kano, M. (2002). The cannabinoid CB1 receptor mediates retrograde signals for depolarization-induced suppression of inhibition in cerebellar Purkinje cells. J. Neurosci. 22:1690. doi: 10.1523/JNEUROSCI.22-05-01690.2002
Yuan, M., Meyer, T., Benkowitz, C., Savanthrapadian, S., Ansel-Bollepalli, L., Foggetti, A., et al. (2017). Somatostatin-positive interneurons in the dentate gyrus of mice provide local-and long-range septal synaptic inhibition. elife 6. doi: 10.7554/eLife.21105
Zallo, F., Gardenal, E., Verkhratsky, A., and Rodríguez, J. J. (2018). Loss of calretinin and parvalbumin positive interneurones in the hippocampal CA1 of aged Alzheimer’s disease mice. Neurosci. Lett. 681, 19–25. doi: 10.1016/j.neulet.2018.05.027
Zemla, R., and Basu, J. (2017). Hippocampal function in rodents. Curr. Opin. Neurobiol. 43, 187–197. doi: 10.1016/j.conb.2017.04.005
Zeng, H. (2022). What is a cell type and how to define it? Cells 185, 2739–2755. doi: 10.1016/j.cell.2022.06.031
Zhang, Z., Li, H., Su, Y., Ma, J., Yuan, Y., Yu, Z., et al. (2022). Neuroprotective effects of a cholecystokinin analogue in the 1-methyl-4-phenyl-1,2,3,6-tetrahydropyridine Parkinson’s disease mouse model. Front. Neurosci. 16:814430. doi: 10.3389/fnins.2022.814430
Zhang, H., Zhang, L., Zhou, D., He, X., Wang, D., Pan, H., et al. (2017). Ablating ErbB4 in PV neurons attenuates synaptic and cognitive deficits in an animal model of Alzheimer’s disease. Neurobiol. Dis. 106, 171–180. doi: 10.1016/j.nbd.2017.07.001
Zhao, R., Fowler, S. W., Chiang, A. C. A., Ji, D., and Jankowsky, J. L. (2014). Impairments in experience-dependent scaling and stability of hippocampal place fields limit spatial learning in a mouse model of Alzheimer’s disease. Hippocampus 24, 963–978. doi: 10.1002/hipo.22283
Zott, B., Busche, M. A., Sperling, R. A., and Konnerth, A. (2018). What happens with the circuit in Alzheimer’s disease in mice and humans? Annu. Rev. Neurosci. 41, 277–297. doi: 10.1146/annurev-neuro-080317-061725
Keywords: GABAergic circuits, Alzheimer’s disease, hippocampus (CA1), long-range GABAergic neurons, disinhibition
Citation: Hernández-Frausto M, Bilash OM, Masurkar AV and Basu J (2023) Local and long-range GABAergic circuits in hippocampal area CA1 and their link to Alzheimer’s disease. Front. Neural Circuits. 17:1223891. doi: 10.3389/fncir.2023.1223891
Received: 16 May 2023; Accepted: 08 September 2023;
Published: 29 September 2023.
Edited by:
Lisa Topolnik, Laval University, CanadaReviewed by:
Claudio Elgueta, University of Freiburg, GermanyCopyright © 2023 Hernández-Frausto, Bilash, Masurkar and Basu. This is an open-access article distributed under the terms of the Creative Commons Attribution License (CC BY). The use, distribution or reproduction in other forums is permitted, provided the original author(s) and the copyright owner(s) are credited and that the original publication in this journal is cited, in accordance with accepted academic practice. No use, distribution or reproduction is permitted which does not comply with these terms.
*Correspondence: Jayeeta Basu, amF5ZWV0YS5iYXN1QG55dWxhbmdvbmUub3Jn; Arjun V. Masurkar, YXJqdW4ubWFzdXJrYXJAbnl1bGFuZ29uZS5vcmc=
Disclaimer: All claims expressed in this article are solely those of the authors and do not necessarily represent those of their affiliated organizations, or those of the publisher, the editors and the reviewers. Any product that may be evaluated in this article or claim that may be made by its manufacturer is not guaranteed or endorsed by the publisher.
Research integrity at Frontiers
Learn more about the work of our research integrity team to safeguard the quality of each article we publish.