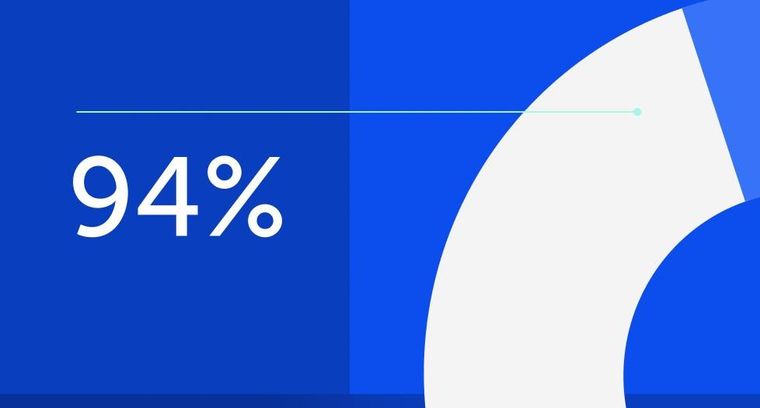
94% of researchers rate our articles as excellent or good
Learn more about the work of our research integrity team to safeguard the quality of each article we publish.
Find out more
BRIEF RESEARCH REPORT article
Front. Neural Circuits, 03 February 2023
Volume 17 - 2023 | https://doi.org/10.3389/fncir.2023.1098913
This article is part of the Research TopicHorizons in Neural Circuits, Volume IIView all 7 articles
The thalamic nuclear complex contains excitatory projection neurons and inhibitory local neurons, the two cell types driving the main circuits in sensory nuclei. While excitatory neurons are born from progenitors that reside in the proliferative zone of the developing thalamus, inhibitory local neurons are born outside the thalamus and they migrate there during development. In addition to these cell types, which occupy most of the thalamus, there are two small thalamic regions where inhibitory neurons target extra-thalamic regions rather than neighboring neurons, the intergeniculate leaflet and the parahabenular nucleus. Like excitatory thalamic neurons, these inhibitory neurons are derived from progenitors residing in the developing thalamus. The assembly of these circuits follows fine-tuned genetic programs and it is coordinated by extrinsic factors that help the cells find their location, associate with thalamic partners, and establish connections with their corresponding extra-thalamic inputs and outputs. In this review, we bring together what is currently known about the development of the excitatory and inhibitory components of the thalamocortical sensory system, in particular focusing on the visual pathway and thalamic interneurons in mice.
The thalamus has classically been considered a relay station in the brain due to its central location and patterns of connectivity. Excitatory neurons in sensory nuclei receive ascending information from peripheral organs and they project their axons beyond the thalamus, mainly into the sensory cortices and avoiding intrinsic connections (Petersen, 2007; Huberman et al., 2008; Tsukano et al., 2017). As the vast majority of cells in sensory nuclei are excitatory neurons, the thalamus could be considered to be merely a relay station of sensory information, transferring messages from the periphery to the cortex. However, there is an increasing body of evidence demonstrating a key role of thalamic nuclei in processing information and gating messages to the cortex. In addition to the ascending sensory information, excitatory neurons integrate signals from other brain structures and from the intrinsic thalamic networks. GABAergic neurons represent an important element in these intrinsic networks and despite their small number, the GABAergic neurons in the interconnected networks shape the output of the sensory thalamus (Hirsch et al., 2015).
Thalamic circuits develop progressively in embryonic stages and they finally assemble during postnatal life (Jhaveri et al., 1991; Schlaggar and O’Leary, 1994). Excitatory neurons are born in the ventricular zone of the developing thalamus, thereafter migrating toward the mantle zone where they start to extend dendrites and axons. Along their route toward the cortex, the axons of excitatory neurons project through different brain territories, bundling into fascicules, branching and making synaptic connections (Crowley and Katz, 2000; Hannan et al., 2001; Hevner et al., 2002; López-Bendito and Molnár, 2003; Gurung and Fritzsch, 2004; Hensch, 2004; Pfeiffenberger et al., 2005; Miko et al., 2008; Hanganu-Opatz, 2010). By contrast, the spatiotemporal developmental trajectory of local GABAergic neurons differs considerably. These GABAergic neurons are not derived from thalamic progenitors but rather, they are born and migrate from neighboring midbrain and pre-thalamic domains, invading the thalamus and integrating into its circuits some time after excitatory neurons. In this review, we bring together what is currently known about the development of the excitatory and inhibitory components of the thalamocortical sensory system, focusing particularly on the visual pathway and on thalamic interneurons in mice.
Thalamic neurons are organized into spatial clusters or nuclei that can be characterized through the subcortical origin of their afferents. Some of these afferents carry sensory information derived from peripheral organs, which adopt a modal organization to define the primary sensory nuclei of the thalamus: the dorsolateral geniculate nucleus (dLG) that receives visual information; the ventral posteromedial nucleus (VPM) for somatosensory input; and the ventral medial geniculate nucleus (MGv) for auditory input. While the dLG, VPM and MGv are classified as first-order (FO) nuclei since their main driving stimuli arrive directly or indirectly from the peripheral sensory organs (Sherman, 2017; Halassa and Sherman, 2019), the thalamus also contains higher-order (HO) nuclei that receive driving inputs from subpopulations of projection neurons in Layer 5b (L5b) of the respective cortical areas (Sherman and Guillery, 2002; Bickford, 2016). HO nuclei mainly process unimodal information, even though they can integrate inputs from other modalities too. Among the HO nuclei, the lateral posterior nucleus (LP, visual), posterior medial (PoM, somatosensory), and the dorsal medial geniculate nucleus (MGd, auditory) not only further process sensory information but they also help the thalamus to connect different cortical areas (Figure 1; Butler, 2008; Halassa and Sherman, 2019).
Figure 1. The thalamus transmits peripheral sensory information to primary sensory areas and retransmits the processed sensory message to secondary cortical areas. The schema represents the major feed-forward connections of the mouse whisker system. Stimuli from peripheral sensory organs arrive at first order thalamic nuclei (FO) which connects with L4 neurons in the primary sensory cortex. The information then spreads to other cortical areas both through direct cortico-cortical connections (red arrows) and through the trans-thalamic pathway using higher-order thalamic nuclei (HO) as relay stations (blue arrows).
The patterns of connectivity between the thalamus and cortex are similar for the different sensory modalities. Thus, sensory stimuli ascending from the peripheral organ arrives at the corresponding FO nucleus, which in turn projects to the L4, L5b, and L6 of the corresponding sensory cortex (Swadlow and Alonso, 2017). Within a cortical column, information flows from L4 to L2/3 and from there to L5 and L6, the layers that project out of the cortex. Neurons from L5b and L6b send projections to HO nuclei, and neurons in L6a project back to the FO nuclei (Sumser et al., 2017; Hoerder-Suabedissen et al., 2018). Therefore, these connections form feedback and feedforward loops that establish the basis of sensory processing in the thalamocortical system (Viaene et al., 2011). As a result, the thalamus represents a hub that can send information to and from different cortical areas (Sherman and Guillery, 2002; Reichova and Sherman, 2004; Lee and Murray Sherman, 2010; Theyel et al., 2010).
Early in development, the diencephalon subdivides into three transverse regions called prosomeres, each of which can be further subdivided into four longitudinal bands or plates: roof, alar, basal, and floor (in a dorsoventral order, Figure 2A). This percolation relies on the presence of gradients of diffusible molecules, such as the wingless-INT proteins (WNTs), bone morphogenetic proteins (BMPs), Sonic hedgehog (SHH), and fibroblast growth factor proteins (FGFs) (Kataoka and Shimogori, 2008; Martinez-Ferre and Martinez, 2009, 2012). Prosomeres are evident at E10 in mice and their respective alar plates develop into different brain structures: prosomere 1 gives rise to the pretectum, which includes multiple domains of the adult brain that are involved in processing visual information and in the execution of visual reflexes (Ferran et al., 2009); prosomere 2 develops into the epithalamus and thalamus; and lastly, prosomere 3 gives rise to the prethalamus, which includes GABAergic structures like the reticular nucleus (RTN) and the zona incerta (ZI) (Puelles and Rubenstein, 2003).
Figure 2. The patterning of the developing diencephalon. (A) The schema represents a sagittal view of the developing mouse brain highlighting the midbrain (Mid) and the subdivisions, known as prosomeres (P1, P2, and P3), of the diencephalon according to the prosomeric model. Ctx: cortex; Hy: hypothalamus; LV: lateral ventriculus; Poa: preoptic area; Rb: rhombencephalon; SC: spinal cord; Str: striatum; 4V: fourth ventriculus. (B) The two progenitor domains of the prosomere 2 or thalamus (Th): Th-c (caudal) and Th-r (rostral). PTh: prethalamus; PT: pretectum. (C) Representative expression patterns of transcription factors in the different the progenitor domains of prosomeres 2 and 3.
Prosomeres 2 and 3 are separated by the zona limitans intrathalamica (ZLI), an organization center that expresses high levels of SHH. During the early percolation of the diencephalon, SHH is a fundamental signaling molecule in prosomere 2 because it steers the differentiation of the prospective thalamus from the epithalamus (Chatterjee and Li, 2012; Chatterjee et al., 2014; Mallika et al., 2015). In the prospective thalamus, two progenitor zones are established by E10.5 in the mouse (Figure 2B): the rostral and the caudal progenitor domains (Jeong et al., 2011; Suzuki-Hirano et al., 2011). The progenitors in the rostral domain are exposed to higher concentrations of SHH and consequently, they express markers like Olig3, Nkx2.2, Ascl1, and Olig2 (Scholpp et al., 2009). On the other hand, the progenitors in the caudal domain are exposed to less SHH, such that they express markers like Olig3, Ngn1/2, and Dbx1 (Figure 2C; Barth and Wilson, 1995; Hashimoto-Torii et al., 2003; Kiecker and Lumsden, 2004; Scholpp et al., 2006; Szabó et al., 2009; Vue et al., 2009). Neurogenesis in these progenitor domains spans approximately from E10 to E13 (Wong et al., 2018) and the postmitotic progeny differentiate into two broad different cell types: the caudal domain differentiates into the glutamatergic projection neurons that populate the thalamic nuclei and form thalamocortical connections (Tou et al., 2007; Price et al., 2012), and the rostral domain differentiates into GABAergic projection neurons that populate the intergeniculate leaflet (IGL) and the perihabenular nucleus (pHB) (Delogu et al., 2012; Fernandez et al., 2018).
As most neurons in the adult thalamus derive from the caudal progenitor domain, they must undergo a complex process of specification to generate neuronal diversity and the distinct thalamic nuclei. Although it is not clear how this diversification occurs, some evidence indicates that the population of caudal progenitors is heterogeneous. Within this caudal progenitor domain, there are transcription factors that are expressed in opposite gradients through the antero-ventral to caudo-dorsal axis. For instance, the expression of Olig2 is high at the most anterior pole and the expression of Dbx1 in the most caudal pole. This polarization of the caudal progenitor domain has relevant implications for thalamic nucleogenesis. Lineage and birth-dating analysis demonstrated that antero-ventral progenitors give rise to neurons populating more latero-ventral nuclei, and caudo-dorsal progenitors to more medio-dorsal ones (Tou et al., 2007; Wong et al., 2018). In addition, to this spatial order, there is also a temporal sequence whereby the formation of the latero-ventral nuclei occurs earlier than the medio-dorsal nuclei, which is consistent with the earlier transition from symmetric to asymmetric division in the antero-ventral compared to the caudo-dorsal progenitors (Nakagawa and Shimogori, 2012; Wong et al., 2018).
If different subpopulations of progenitors give rise to diverse cell types populating thalamic nuclei, it is expected that they are controlled by different genetic programs. However, data from single-cell RNA sequencing of E12 mice suggests that the progenitors in the ventricular zone of the caudal domain (apical progenitors) comprises a unique cluster of cells based on their transcriptomic profile. There is, indeed, a second cluster of dividing cells that derives from the apical progenitors and corresponds to the basal (or intermediate) progenitors of the thalamus located away from the ventricular zone (Guo and Li, 2019). Apical progenitors generate larger clones than basal progenitors, 12 neurons on average, and both apical or basal-derived clones tend to occupy more than one nucleus (Wong et al., 2018). It has been suggested that sibling cells tend to occupy functionally related nuclei but further evidence is needed (Shi et al., 2017). Despite transcriptomic analysis does not reveal clear-cut internal subdivisions of progenitor domains, they do show a graded pattern of gene expression (Guo and Li, 2019), as previously observed in data obtained using labeling methods (Tou et al., 2007). In sum, to refine the classification of the apparently heterogeneous populations of progenitors in the caudal domain of the thalamus, it is necessary to generate larger databases of single-cell transcriptomic profiles, accompanied by more complete atlases of gene expression and sophisticated algorithms.
Although the projection neurons of thalamic nuclei are born in the caudal proliferative domain, it remains unclear whether their nucleus-specific identity is specified at the progenitor stage (Chatterjee et al., 2014). Rather, current evidence suggests that nucleus-specific identity is conferred when progenitors exit the cell-cycle. The gradual expression of the Gbx2 transcription factor by post-mitotic cells is one determinant of thalamic nuclei that project to the cortex (Figure 3B). The expression of Gbx2 starts at E9.5, following a very dynamic spatiotemporal pattern that ultimately defines the borders of the thalamus with the epithalamus, prethalamus and pretectum, as well as parcellating the thalamus into distinct nuclei (Nakagawa and O’Leary, 2001; Tou et al., 2007; Chen et al., 2009; Li et al., 2012). The absence of Gbx2 leads to a shrinking of the thalamus at its posterior and dorsal borders, enlarging the pretectum and epithalamus, respectively. In turn, there is a disruption in the histogenesis of the nuclear complexes and a significant loss of thalamocortical projections (Chen et al., 2009; Chatterjee and Li, 2012; Mallika et al., 2015; Nakagawa, 2019).
Figure 3. Integration of local thalamic interneurons from extra-thalamic sources. (A) Right, Schema showing the migration toward the thalamus of prospective local interneurons born in the midbrain and prethalamus. Left, Prospective local interneurons start invading the developing mouse dLGN shortly before birth. (B) Representative expression patterns of transcription factors, specially lineage selector genes, in precursor cells from prosomere 2 and GABA lineages from prosomere 3 and midbrain. (C) Schema of a mouse dLGN showing sequentially how local interneurons migrate and distribute into the nucleus in control conditions and upon deprivation of retinal input (Golding et al., 2014; Charalambakis et al., 2019; Su et al., 2020).
In the developing mouse thalamus, prospective thalamocortical cells begin to extend their axons toward the cortex at around E12, shortly after neurogenesis ceases (Figure 4). Growing thalamocortical axons are guided through different territories by molecular and cellular cues, as well as by activity-dependent mechanisms (Marcos-Mondéjar et al., 2012; Mire et al., 2012; Molnár et al., 2012; Leyva-Díaz et al., 2014; Castillo-Paterna et al., 2015). Thalamocortical axons start their journey toward the cortex by growing rostrally through the thalamus and heading toward the prethalamus. They navigate through the thalamic territory using prethalamic axons as scaffolds and following gradients of guidance cues, such as Sema3a and Netrin1 (Quintana-Urzainqui et al., 2020). Thalamocortical axons traverse the entire prethalamus and move through the Slit-free domain of the peduncular hypothalamus until they encounter the diencephalic-telencephalic boundary (DTB) (Callejas-Marin et al., 2022). Slit proteins guide thalamocortical axons out of the hypothalamus and they prevent them from crossing the midline (Bagri et al., 2002; López-Bendito et al., 2007; Braisted et al., 2009; Bielle et al., 2011). Subsequently, thalamocortical axons cross the DTB, attracted by Netrin1, and they enter the ventral telencephalon (Métin and Godement, 1996; Braisted et al., 2000).
Figure 4. Timeline showing milestones in mouse thalamic development (red boxes). The different patterns of spontaneous thalamic activity throughout development (blue boxes). First, thalamic spontaneous activity is characterized for being scarce and the events are uncorrelated (E12-E14). This is followed by waves of spontaneous activity (E14-P0) that propagate among FO and then HO nuclei. At perinatal stages, these waves disappear and instead, there are small events most likely triggered spontaneously from peripheral organs that engage cortical areas. Before eye opening, there is a switch to an adult-like pattern where spontaneous activity becomes dense, asynchronous and continuous (Colonnese et al., 2010). CP, cortical plate; CTAs, corticothalamic axons; E, embryonic day; Ins, interneurons; P, postnatal day; TCAs, thalamocortical axons.
Thalamic projections continue their journey through the telencephalon, reaching the internal capsule from where they follow a permissive corridor formed between two repulsive areas: the proliferative zone of the medial ganglionic eminence (MGE) and the globus pallidus. A large proportion of these corridor cells are GABAergic neurons derived from the lateral ganglionic eminence (LGE), cells that express membrane-bound Neuregulin1 and that migrate into the mantle of the MGE between E11-E14 (López-Bendito et al., 2006; Bielle et al., 2011). The topographic organization of thalamocortical axons is preserved throughout the ventral telencephalon, which is a result of complex interactions between cues like Netrin1, Sema3a/3f, Slit1, L1 cell-adhesion molecules and ephrinA5 (Dufour et al., 2003; Seibt et al., 2003; Mire et al., 2012; Leyva-Díaz et al., 2014; Castillo-Paterna et al., 2015).
By E14, thalamocortical axons meet corticofugal axons at the pallial-subpallial boundary (PSPB), and they use them as scaffolds to turn into the pallium and spread across the developing cortex (McConnell et al., 1989; Blakemore and Molnar, 1990; de Carlos and O’Leary, 1992). The timing of the arrival of thalamic axons is relevant for cortical development. As thalamic inputs arrive at a rather immature cortex, they can exert a major effect on ongoing processes such as neurogenesis, migration and differentiation (Cadwell et al., 2019). Thalamocortical axons start to invade the cortical plate at E17 when the granular layers are being formed (Allendoerfer and Shatz, 1994; del Río et al., 2000; Molyneaux et al., 2007; Little et al., 2009), and they finally reach their destination during the first postnatal week (Figure 4), mainly targeting neurons in L4 and to a lesser extent, neurons in L5b (López-Bendito and Molnár, 2003).
While invading the cortical plate, thalamic axons form functional synapses with subplate cells, a transient layer of rather mature neurons located below the cortical plate that coordinate the early maturation of thalamocortical networks (Kanold and Luhmann, 2010; Hoerder-Suabedissen and Molnár, 2015). As Cajal-Retzius cells, another transient population of cortical neurons, subplate neurons disappear by programmed cells death during the first postnatal days in mouse and thalamocortical afferents form direct contacts with neurons in L4 and deeper layers. Apart from subplate neurons, thalamocortical afferents form transient circuits with a specific subpopulation of developing interneruons. During the first postnatal week in mice, thalamocortical axons contact L5 somatostatin-positive interneurons that in turn contact spiny stellate neurons in layer 4 (Marques-Smith et al., 2016). This circuit becomes remodeled and disappears by the end of the first postnatal week. Despite its brief duration, the connection between L5 somatostatin-positive interneurons and L4 neurons orchestrates the assembly of local inhibition in layers 4. Also the density of thalamocortical input to infragranular interneurons varies during development. Somatostatin-positive interneurons receive a transient strong thalamic drive at immature stages that is required for the correct assembly of thalamic feed-forward inhibition mediated by parvalbumin-positive interneurons (Tuncdemir et al., 2016).
In mice, corticothalamic projections appear at E10 from post-mitotic neurons in the cortical plate. These corticofugal projections navigate laterally through the intermediate zone until they reach the PSPB between E13 and E15 (Jacobs et al., 2007). At this boundary, corticothalamic axons interact with the ascending thalamocortical axons, facilitating their invasion of the cortical territory. The corticothalamic axons then continue their journey through the ventral telencephalon toward the internal capsule, where molecular cues and corridor cells guide them toward the diencephalon (Bagri et al., 2002; López-Bendito et al., 2006, 2007). By E15, corticothalamic axons have crossed the DTB to enter into the prethalamus, where more-or-less a day later they interact with cells from the RTN and the perireticular nucleus (PRN) (Garel and Rubenstein, 2004; Molnár et al., 2012; Deck et al., 2013). These axons are sorted in the prethalamus and the majority of axons from L5 are directed toward the cerebral peduncle, while axons from L6 and the remaining axons from L5 are directed toward their thalamic targets (Clascá et al., 1995; Molnár and Cordery, 1999; Jacobs et al., 2007). Mouse corticothalamic axons invade the thalamus just prior birth (Figure 4), first entering the developing somatosensory nuclei, and then invading the auditory and visual nuclei, which are fully innervated by the end of the first postnatal week (Jacobs et al., 2007; Grant et al., 2012). However, the cellular and molecular mechanisms that guide the entry of corticothalamic axons into the thalamus remain poorly understood. Nevertheless, removal of retinal input alters corticothalamic innervation of the dLGN, inducing premature entry of L6 axons and an abnormal cross-hierarchical invasion of L5 axons that would otherwise be designated to the LP (Brooks et al., 2013; Grant et al., 2016; Moreno-Juan et al., 2022).
The thalamus and cortex develop at a relatively similar pace and consequently they may influence each other’s maturation. The thalamus clearly affects many aspects of cortical development, such as the radial organization, cell proliferation, specification of cortical areas, navigation of corticothalamic axons, interneuron maturation and circuit assembly (Rakic, 1991; Dehay et al., 1996; Zechel et al., 2016). More specifically, glutamate released by thalamocortical axons is required for Reelin-expressing cortical interneurons to develop (de Marco García et al., 2015). Similarly, thalamic inputs regulate the integration of somatostatin- and parvalbumin-expressing interneurons into cortical circuits (Wamsley and Fishell, 2017), and the segregation of pyramidal neurons in the L4 of the barrel cortex (Li et al., 2013; Assali et al., 2017). Thalamic input also affects cell identity in the cortex, since differentiation into primary or higher-order cortical areas relies on the arrival of thalamocortical axons (Chou et al., 2013). Indeed, when FO nuclei are removed genetically, primary sensory areas acquire the molecular and functional properties of secondary cortical areas (Chou et al., 2013; Vue et al., 2013; Pouchelon et al., 2014). Recently, somatostatin-expressing interneurons in the cortex were seen to be necessary for the correct arrival of thalamocortical inputs onto parvalbumin-expressing interneurons and pyramidal neurons during the first week of postnatal life in mice (de Marco García et al., 2015; Marques-Smith et al., 2016; Tuncdemir et al., 2016; Che et al., 2018; Takesian et al., 2018).
Neuronal transmission in the thalamocortical system can also influence the development of the cortex, as seen when normal synaptic transmission is disrupted in knock-out mice that lack key synaptic proteins, such as NMDA receptor 1, adenylyl cyclase 1, or metabotropic glutamate receptor 5. Such disruption of neurotransmission can provoke a lack of neuronal organization and smaller barrels with blurry borders in S1 (Iwasato et al., 2000; Datwani et al., 2002; Ballester-Rosado et al., 2010; Antón-Bolaños et al., 2019).
Neuronal activity in the developing thalamus evolves over different stages in mice (Figure 4). In a first stage, endogenous and uncorrelated activity spans mid-embryonic mouse development (E12-E14), which affects the expression of genes involved in thalamocortical axon growth and branching when manipulated (Herrmann and Shatz, 1995; Uesaka et al., 2007; Mire et al., 2012; Castillo-Paterna et al., 2015; Moreno-Juan et al., 2017). After this initial stage, the activity in the thalamus becomes more synchronous and by E14, spontaneous synchronic activity takes the form of waves of spontaneous activity that initially propagate through FO nuclei and that later also engage HO nuclei (Moreno-Juan et al., 2017). After birth, spontaneous activity becomes less correlated, especially in the somatosensory and auditory nuclei and at P2, in the visual nucleus as well (Colonnese et al., 2010). The waves of spontaneous activity observed in the thalamus are transmitted along thalamocortical axons to the developing cortical areas and consequently, early thalamocortical input could have an impact on cortical development through activity-dependent mechanisms. Indeed, altering patterns of activity through genetic manipulation provokes cross-modal changes in the development of sensory areas in the cortex (Moreno-Juan et al., 2017; Antón-Bolaños et al., 2018). The electrical properties of the thalamocortical circuit progressively mature during the first two postnatal weeks, undertaking more continuous and decorrelated spontaneous firing (Murata and Colonnese, 2016, 2018; Martini et al., 2021). This transition in spontaneous thalamic activity seems to be critical for the onset of the active processing of environmental information by the cortex. Moreover, it might be caused by changes in the sensory organs, synaptic maturation or circuit remodeling, such as the gradual integration of inhibitory components (Demas et al., 2003; Colonnese, 2014; Sokhadze et al., 2018).
Thalamic neurons receive inhibitory inputs from projecting neurons residing in the prethalamus (RN, ZI, and vLGN) and from other extra-thalamic sources, such as the superior colliculus, basal ganglia, hypothalamus and pontine reticular formation (Halassa and Acsády, 2016). In addition, they are also inhibited by local GABA-releasing neurons, although the number and distribution of these local interneurons is not conserved across species. In small mammals like mice, marsupials and bats, interneurons are sparse and mainly found in the dLGN, whereas they are abundant and widely distributed throughout the thalamus in large mammals. Inhibitory interneurons are absent from the dLGN of some non-mammalian amniotes, such as crocodiles, lizards and snakes, but they are present in birds (Butler, 2008). Local interneurons are not the only GABAergic cells in the mature thalamus, since there is a small subpopulation of GABAergic cells that reside within the IGL and pHB whose axons project to extra-thalamic targets (Harrington, 1997; Tou et al., 2007; Delogu et al., 2012; Inamura et al., 2012; Fernandez et al., 2018).
Local interneurons that integrate into thalamic circuits are not born in the proliferative zone of the developing thalamus but rather, they migrate into the thalamus from other brain regions. Across species, some regions that generate thalamic neurons are conserved but also, additional regions are observed as thalamic circuits increase in complexity and size. The midbrain proliferative zone generates a stream of cells that colonizes the developing thalamus and that is made up of cells that differentiate into local GABAergic cells (Figure 3A; Jones, 2002; Hayes et al., 2003; Bakken et al., 2015; Jager et al., 2021). In the mouse, the invasion of these local interneuron precursors begins at E17, starting from the caudal tier of the developing thalamus. Fate mapping experiments confirmed the midbrain origin of these cells, showing that they are born at approximately E10-E13 and that they belong to the Engrailed1 lineage (Jager et al., 2016, 2021), a transcription factor that is expressed in the midbrain and not the forebrain (Sgaier et al., 2007). The precursors generated from the Engrailed1 progenitors are also characterized by the expression of the transcription factors Sox14, Gata2, and Otx2 (Figure 3B). Once in the thalamus, these midbrain-derived interneurons adopt a specific spatial distribution, whereby they are enriched in FO nuclei but they also appear in HO and rostral nuclei (Jager et al., 2021). This subpopulation of GABAergic cells constitutes the largest of the local interneuron populations in the mature thalamus.
Another source of thalamic interneurons in the mouse is the developing prethalamus or prosomere 3 (Figure 3A). Located rostral to the thalamus, the developing prethalamus generates several GABAergic cell lineages, most of which populate prethalamic structures like the RTN and the vLGN, while others establish a stream of cells that invade the developing thalamus from its rostral tier around the time of birth (Golding et al., 2014; Jager et al., 2021). Approximately 20% of the population of local interneurons in the mature thalamus are specified in the developing prethalamus from a lineage that expresses Dlx1/2, Foxd1, and Dlx5/6, and that does not express Lhx6 or Nkx2.1 (Figure 3B). The prethalamus-derived interneurons that will invade the thalamus have features complementary to midbrain-derived interneurons, for example, they do not express Sox14 and they are enriched in HO nuclei (Jager et al., 2021).
Studying the origin of local thalamic interneurons is challenging because the thalamus does not generate local GABAergic neurons but it does generate projecting GABAergic neurons. The thalamic progenitors that give rise to projecting GABAergic neurons reside in the rostral tier of the proliferative neuroepithelium of prosomere 2, known as pTH-R (Tou et al., 2007). Indeed, the stream of cells derived from this progenitor domain can be distinguished from the neighboring caudal domain of thalamic progenitors (known as the pTH-C), and from prosomere 3, by the expression of post-mitotic markers like Sox14, Nkx2.2, and Tal1 (Jeong et al., 2011). The cells derived from pTH-R become GABAergic projection neurons that populate diencephalic regions, such as the pHB and the IGL. While IGL neurons project to the suprachiasmatic nucleus and other hypothalamic nuclei, pHB axons target the ventromedial prefrontal cortex, the dorsomedial striatum and the nucleus accumbens, and as such, they are involved in mechanisms that regulate mood (Moore et al., 2000; Fernandez et al., 2018; Anastasiades et al., 2021).
An additional challenge is that extra-thalamic sources of local thalamic GABAergic neurons, like the prethalamus and midbrain, also generate other GABAergic neurons. As well as the Sox14-negative local interneurons of the thalamus, the proliferative zone of the prethalamus gives rise to many other GABAergic cells. At E10 in mice, the progenitor cells found in the ventricular zone of the prethalamus are characterized by strong expression of transcription factors like Olig2, Dlx genes and Foxd1 (Tou et al., 2007; Blackshaw et al., 2010; Newman et al., 2018; Puelles et al., 2021). Before E14, the prethalamic lineage cells differentiate into neurons, and they start migrating laterally and dorsally to populate the nascent RTN, ZI and vLGN (Ono et al., 2008; Inamura et al., 2011). Almost all of these cells are either local or projecting GABAergic neurons, the latter targeting regions of the thalamus, pretectum and midbrain (Jones, 2007).
The neural tube of rodents exhibits three domains of GABAergic progenitors along its rostro-caudal axis (Achim et al., 2013), each characterized by specific genetic programs with distinct terminal selector genes and giving rise to three broad GABA lineages. The borders of these domains are defined by molecular markers and by secondary organizers. Firstly, the rostral domain expands caudally from the ganglionic eminences, through prosomere 3 up to the ZLI in the diencephalon, where GABAergic differentiation depends on the Dlx1/2 lineage selector genes (Delogu et al., 2012; Le et al., 2017). Secondly, the ZLI separates the rostral domain from the intermediate domain, which expands caudally up to the isthmic organizer at the midbrain-hindbrain boundary. The intermediate domain includes prosomere 2, prosomere 1 and the midbrain, and GABAergic neurogenesis in the intermediate domain relies on the lineage selector genes Tal2 and Gata2 (Virolainen et al., 2012). Finally, the caudal domain spans through the hindbrain and spinal cord, where GABAergic fate is acquired through the expression of lineage selector genes like Ptf1a and Tal1 (Hoshino et al., 2005; Muroyama et al., 2005; Fujiyama et al., 2009). Therefore, the combined mesencephalic and prethalamic origin of thalamic interneurons mean they constitute a population with two broad molecular identities: the Dlx1/2 (enriched in HO nuclei) and the Gata2/Tal2 lineage (enriched in FO nuclei) (Figures 3A, B).
The GABAergic cells derived from each lineage acquire their identity during development through different genetic programs. Although the molecular mechanisms that confer GABAergic identity have not specifically been studied in thalamic interneurons, it is likely that each subpopulation of thalamic interneurons unfolds genetic programs according to their site of origin. For instance, GABA precursors in the developing midbrain start expressing Gata2 and Tal2 after cell-cycle exit, fate determinants that directly regulate the acquisition of a GABAergic phenotype. These cells activate sustained expression of downstream transcription factors related to the maintenance of a GABAergic identity (Tal1, Gata3, Six3, and Gad1) and to the correct migration of midbrain precursors (Sox14) (Delogu et al., 2012). Indeed, in mice lacking GATA2 or TAL2, GABAergic precursors from the midbrain fail to express genes related to GABA neurotransmission and they switch fate, acquiring a glutamatergic identity (Kala et al., 2009; Achim et al., 2013). However, it remains unclear to what extent midbrain-derived thalamic interneurons share a similar developmental trajectory with other midbrain-derived GABAergic cells. A similar genetic program could also be established for the prethalamic-derived thalamic interneurons and although there are no direct studies on this subpopulation, the developmental program is likely to resemble that of other GABAergic neurons derived from the rostral GABAergic domain, such as cortical and striatal interneurons (Lindtner et al., 2019). As such, the Dlx1/2 transcription factors may contribute to their GABAergic phenotype, either by directly controlling the expression of the GAD isoforms or by indirectly activating Dlx5 and Dlx6 transcription, markers of more mature GABAergic precursors (Cobos et al., 2007; Le et al., 2017).
In addition to intrinsic gene regulatory networks, extrinsic factors also influence the development of thalamic interneurons. In the mouse visual system, different developmental processes are thought to be extrinsically influenced by the input that arrives from the retina, ranging from neurogenesis to network recruitment of interneurons (Golding et al., 2014; Charalambakis et al., 2019; Su et al., 2020). This is evident in animal models where retinal projections are absent or compromised. For example, in anophthalmic mice whose optic nerves were severed at birth and in mice with abnormal spontaneous retinal activity during development, thalamic interneurons accumulate in the upper tiers of the dLGN as opposed to adopting the homogenous distribution throughout the nucleus observed in control mice (Figure 3C; Golding et al., 2014). In these models, the synaptic properties of thalamic interneurons were also affected due to the downregulation of presynaptic and postsynaptic proteins, enhancing the excitability of dLGN neurons and disinhibiting the visual thalamocortical system. Similar results were reported in a transgenic mouse (Math5–/–) in which the optic tract does not develop, and following binocular enucleation in mice soon after birth (Charalambakis et al., 2019). In both scenarios, the distribution of thalamic interneurons was biased toward the dorsal part of the nucleus, failing to develop both mature intrinsic electrical properties and normal synaptic connectivity with relay neurons.
In addition to the alterations in the distribution of interneurons, there are fewer of these cells in the dLGN of Math5–/– mice than in control mice. This reduction in the number of interneurons correlates with abnormally weak FGF15 expression by some astrocytes residing in the visual thalamus (Su et al., 2020). Thus, as in other brain structures, it is likely that the release of FGFs contributes to the recruitment and maturation of inhibitory neurons. Accordingly, genetic ablation of FGF15 impairs the migration of thalamic interneurons into the dLGN and they become misrouted into the somatosensory nucleus. The expression of astrocytic FGF15 in the visual thalamus may be regulated by the SHH released from retinal axons (Deven Somaiya et al., 2022), and the expression of astrocytic FGF15 is reduced in the absence of retinal SHH, decimating the recruitment of interneurons. However, more evidence is needed regarding the interaction between SHH released from retinal axons, the SHH signaling cascades in astrocytes and FGF15 expression. Other effects of SHH might also be at play in these processes and for instance, SHH could exert a broader effect on thalamic astrocytes as it participates in astrocyte specification in brain regions like the retina (Dakubo et al., 2008).
In this review we first focus on the development of excitatory neurons of the thalamus, how they extend their axons and receive inputs from the cortex, and the role of spontaneous activity in the development of these projections. Next, we have delved into the information currently available regarding the development of the other main neuronal cell-type present in the thalamus, local GABAergic interneurons. The evidence compiled in this review establishes the state-of-the-art of the field but also, it poses important questions that need to be addressed. For instance, there are few studies that have investigated how the development of thalamocortical excitatory neurons and local GABAergic interneurons is orchestrated. Moreover, further studies are required to disentangle the precise origin of these local GABAergic neurons, as well as comparative studies using ancient species. It is still unclear what molecular signature determines the thalamic fate of interneurons derived from the midbrain, the prethalamus or other regions, as well as the guidance mechanisms that direct interneurons into the thalamus during development. Finally, since it is now well established that the thalamus presents different patterns of spontaneous activity and that changes in these patterns affect the development of other structures like the sensory cortices, it would be interesting to study the impact of this spontaneous thalamic activity on developing thalamic interneurons.
The original contributions presented in this study are included in this article/supplementary material, further inquiries can be directed to the corresponding authors.
GL-B, FM, and IH-G wrote the manuscript. All authors contributed to the article and approved the submitted version.
This work was supported by grants from the European Research Council (ERC-2014-CoG-647012), the PROMETEO grant 2021/52 from the Generalitat Valenciana and the Spanish Ministry of Science, and Innovation and Universities (grants: PGC2018/096631-B-I00 and PID2021-127112NB-I00).
The authors are grateful to the members of GL-B laboratory for stimulating discussions.
The authors declare that the research was conducted in the absence of any commercial or financial relationships that could be construed as a potential conflict of interest.
All claims expressed in this article are solely those of the authors and do not necessarily represent those of their affiliated organizations, or those of the publisher, the editors and the reviewers. Any product that may be evaluated in this article, or claim that may be made by its manufacturer, is not guaranteed or endorsed by the publisher.
Achim, K., Peltopuro, P., Lahti, L., Tsai, H. H., Zachariah, A., Åstrand, M., et al. (2013). The role of Tal2 and Tal1 in the differentiation of midbrain GABAergic neuron precursors. Biol. Open 2, 990–997. doi: 10.1242/bio.20135041
Allendoerfer, K. L., and Shatz, C. J. (1994). The subplate, a transient neocortical structure: Its role in the development of connections between thalamus and cortex. Annu. Rev. Neurosci. 17, 185–218. doi: 10.1146/ANNUREV.NE.17.030194.001153
Anastasiades, P. G., Collins, D. P., and Carter, A. G. (2021). Mediodorsal and ventromedial thalamus engage distinct L1 circuits in the prefrontal cortex. Neuron 109, 314–330.e4. doi: 10.1016/J.NEURON.2020.10.031
Antón-Bolaños, N., Espinosa, A., and López-Bendito, G. (2018). Developmental interactions between thalamus and cortex: A true love reciprocal story. Curr. Opin. Neurobiol. 52, 33–41. doi: 10.1016/J.CONB.2018.04.018
Antón-Bolaños, N., Sempere-Ferràndez, A., Guillamón-Vivancos, T., Martini, F. J., Pérez-Saiz, L., Gezelius, H., et al. (2019). Prenatal activity from thalamic neurons governs the emergence of functional cortical maps in mice. Science 364, 987–990. doi: 10.1126/science.aav7617
Assali, A., le Magueresse, C., Bennis, M., Nicol, X., Gaspar, P., and Rebsam, A. (2017). RIM1/2 in retinal ganglion cells are required for the refinement of ipsilateral axons and eye-specific segregation. Sci. Rep. 7:3236. doi: 10.1038/S41598-017-03361-0
Bagri, A., Marín, O., Plump, A. S., Mak, J., Pleasure, S. J., Rubenstein, J. L. R., et al. (2002). Slit proteins prevent midline crossing and determine the dorsoventral position of major axonal pathways in the mammalian forebrain. Neuron 33, 233–248. doi: 10.1016/S0896-6273(02)00561-5
Bakken, T. E., van Velthoven, C. T. J., Menon, V., Hodge, R. D., Yao, Z., Nguyen, T. N., et al. (2015). Single-cell RNA-seq uncovers shared and distinct axes of variation in dorsal LGN neurons in mice, non-human primates and humans. eLife 10:e64875. doi: 10.1101/2020.11.05.367482
Ballester-Rosado, C. J., Albright, M. J., Wu, C. S., Liao, C. C., Zhu, J., Xu, J., et al. (2010). mGluR5 in cortical excitatory neurons exerts both cell-autonomous and -nonautonomous influences on cortical somatosensory circuit formation. J. Neurosci. 30, 16896–16909. doi: 10.1523/JNEUROSCI.2462-10.2010
Barth, K. A., and Wilson, S. W. (1995). Expression of zebrafish nk2.2 is influenced by sonic hedgehog/vertebrate hedgehog-1 and demarcates a zone of neuronal differentiation in the embryonic forebrain. Development 121, 1755–1768. doi: 10.1242/DEV.121.6.1755
Bickford, M. E. (2016). Thalamic circuit diversity: Modulation of the driver/modulator framework. Front. Neural Circ. 9:86. doi: 10.3389/FNCIR.2015.00086
Bielle, F., Marcos-Mondejar, P., Keita, M., Mailhes, C., Verney, C., Nguyen Ba-Charvet, K., et al. (2011). Slit2 activity in the migration of guidepost neurons shapes thalamic projections during development and evolution. Neuron 69, 1085–1098. doi: 10.1016/J.NEURON.2011.02.026
Blackshaw, S., Scholpp, S., Placzek, M., Ingraham, H., Simerly, R., and Shimogori, T. (2010). Molecular pathways controlling development of thalamus and hypothalamus: From neural specification to circuit formation. J. Neurosci. 30, 14925–14930. doi: 10.1523/JNEUROSCI.4499-10.2010
Blakemore, C., and Molnar, Z. (1990). Factors involved in the establishment of specific interconnections between thalamus and cerebral cortex. Cold Spring Harb. Symp. Quant. Biol. 55, 491–504. doi: 10.1101/SQB.1990.055.01.048
Braisted, J. E., Catalano, S. M., Stimac, R., Kennedy, T. E., Tessier-Lavigne, M., Shatz, C. J., et al. (2000). Netrin-1 promotes thalamic axon growth and is required for proper development of the thalamocortical projection. J. Neurosci. 20, 5792–5801. doi: 10.1523/JNEUROSCI.20-15-05792.2000
Braisted, J. E., Ringstedt, T., and O’Leary, D. D. M. (2009). Slits are chemorepellents endogenous to hypothalamus and steer thalamocortical axons into ventral telencephalon. Cereb. Cortex 19(Suppl. 1), i144–i151. doi: 10.1093/CERCOR/BHP035
Brooks, J. M., Su, J., Levy, C., Wang, J. S., Seabrook, T. A., Guido, W., et al. (2013). A molecular mechanism regulating the timing of corticogeniculate innervation. Cell Rep. 5, 573–581. doi: 10.1016/J.CELREP.2013.09.041
Butler, A. B. (2008). Evolution of the thalamus: A morphological and functional review. Thalamus. Relat. Syst. 4, 35–58. doi: 10.1017/S1472928808000356
Cadwell, C. R., Bhaduri, A., Mostajo-Radji, M. A., Keefe, M. G., and Nowakowski, T. J. (2019). Development and arealization of the cerebral cortex. Neuron 103, 980–1004. doi: 10.1016/J.NEURON.2019.07.009
Callejas-Marin, A., Moreno-Bravo, J. A., Company, V., Madrigal, M. P., Almagro-García, F., Martínez, S., et al. (2022). Gli2-mediated Shh signaling is required for thalamocortical projection guidance. Front. Neuroanat. 16:830758. doi: 10.3389/FNANA.2022.830758
Castillo-Paterna, M., Moreno-Juan, V., Filipchuk, A., Rodríguez-Malmierca, L., Susín, R., and López-Bendito, G. (2015). DCC functions as an accelerator of thalamocortical axonal growth downstream of spontaneous thalamic activity. EMBO Rep. 16, 851–862. doi: 10.15252/EMBR.201439882
Charalambakis, N. E., Govindaiah, G., Campbell, P. W., and Guido, W. (2019). Developmental remodeling of thalamic interneurons requires retinal signaling. J. Neurosci. 39, 3856–3866. doi: 10.1523/JNEUROSCI.2224-18.2019
Chatterjee, M., and Li, J. Y. H. (2012). Patterning and compartment formation in the diencephalon. Front. Neurosci. 6:66. doi: 10.3389/FNINS.2012.00066
Chatterjee, M., Guo, Q., Weber, S., Scholpp, S., and Li, J. Y. H. (2014). Pax6 regulates the formation of the habenular nuclei by controlling the temporospatial expression of Shh in the diencephalon in vertebrates. BMC Biol. 12:13. doi: 10.1186/1741-7007-12-13
Che, A., Babij, R., Iannone, A. F., Fetcho, R. N., Ferrer, M., Liston, C., et al. (2018). Layer I interneurons sharpen sensory maps during neonatal development. Neuron 99, 98–116.e7. doi: 10.1016/J.NEURON.2018.06.002
Chen, L., Guo, Q., and Li, J. Y. H. (2009). Transcription factor Gbx2 acts cell-nonautonomously to regulate the formation of lineage-restriction boundaries of the thalamus. Development 136, 1317–1326. doi: 10.1242/DEV.030510
Chou, S. J., Babot, Z., Leingar̈tner, A., Studer, M., Nakagawa, Y., and O’Leary, D. D. M. (2013). Geniculocortical input drives genetic distinctions between primary and higher-order visual areas. Science 340, 1239–1242. doi: 10.1126/SCIENCE.1232806
Clascá, F., Angelucci, A., and Sur, M. (1995). Layer-specific programs of development in neocortical projection neurons. Proc. Natl. Acad. Sci. U.S.A. 92, 11145–11149. doi: 10.1073/PNAS.92.24.11145
Cobos, I., Borello, U., and Rubenstein, J. L. R. (2007). Dlx transcription factors promote migration through repression of axon and dendrite growth. Neuron 54, 873–888. doi: 10.1016/J.NEURON.2007.05.024
Colonnese, M. T. (2014). Rapid developmental emergence of stable depolarization during wakefulness by inhibitory balancing of cortical network excitability. J. Neurosci. 34, 5477–5485. doi: 10.1523/JNEUROSCI.3659-13.2014
Colonnese, M. T., Kaminska, A., Minlebaev, M., Milh, M., Bloem, B., Lescure, S., et al. (2010). A conserved switch in sensory processing prepares developing neocortex for vision. Neuron 67, 480–498.
Crowley, J. C., and Katz, L. C. (2000). Early development of ocular dominance columns. Science 290, 1321–1324. doi: 10.1126/SCIENCE.290.5495.1321
Dakubo, G. D., Beug, S. T., Mazerolle, C. J., Thurig, S., Wang, Y., and Wallace, V. A. (2008). Control of glial precursor cell development in the mouse optic nerve by sonic hedgehog from retinal ganglion cells. Brain Res. 1228, 27–42. doi: 10.1016/J.BRAINRES.2008.06.058
Datwani, A., Iwasato, T., Itohara, S., and Erzurumlu, R. S. (2002). NMDA receptor-dependent pattern transfer from afferents to postsynaptic cells and dendritic differentiation in the barrel cortex. Mol. Cell Neurosci. 21, 477–492. doi: 10.1006/mcne.2002.1195
de Carlos, J. A., and O’Leary, D. D. M. (1992). Growth and targeting of subplate axons and establishment of major cortical pathways. J. Neurosci. 12, 1194–1211. doi: 10.1523/JNEUROSCI.12-04-01194.1992
de Marco García, N. V., Priya, R., Tuncdemir, S. N., Fishell, G., and Karayannis, T. (2015). Sensory inputs control the integration of neurogliaform interneurons into cortical circuits. Nat. Neurosci. 18, 393–403. doi: 10.1038/NN.3946
Deck, M., Lokmane, L., Chauvet, S., Mailhes, C., Keita, M., Niquille, M., et al. (2013). Pathfinding of corticothalamic axons relies on a rendezvous with thalamic projections. Neuron 77, 472–484. doi: 10.1016/J.NEURON.2012.11.031
Dehay, C., Giroud, P., Berland, M., Killackey, H. P., and Kennedy, H. (1996). Phenotypic characterisation of respecified visual cortex subsequent to prenatal enucleation in the monkey: Development of acetylcholinesterase and cytochrome oxidase patterns. J. Comp. Neurol. 376, 386–402. doi: 10.1002/(SICI)1096-9861(19961216)376:3<386::AID-CNE3>3.0.CO;2-Z
del Río, J. A., Martínez, A., Auladell, C., and Soriano, E. (2000). Developmental history of the subplate and developing white matter in the murine neocortex. Neuronal organization and relationship with the main afferent systems at embryonic and perinatal stages. Cereb. Cortex 10, 784–801. doi: 10.1093/CERCOR/10.8.784
Delogu, A., Sellers, K., Zagoraiou, L., Bocianowska-Zbrog, A., Mandal, S., Guimera, J., et al. (2012). Subcortical visual shell nuclei targeted by ipRGCs develop from a Sox14+-GABAergic progenitor and require sox14 to regulate daily activity rhythms. Neuron 75, 648–662. doi: 10.1016/j.neuron.2012.06.013
Demas, J., Eglen, S. J., and Wong, R. O. L. (2003). Developmental loss of synchronous spontaneous activity in the mouse retina is independent of visual experience. J. Neurosci. 23, 2851–2860. doi: 10.1523/JNEUROSCI.23-07-02851.2003
Deven Somaiya, R., Stebbins, K., Xie, H., Denise, A., Garcia, R., and Fox, M. A. (2022). Sonic hedgehog-dependent recruitment of GABAergic interneurons into the developing visual thalamus. eLife 10:e79833. doi: 10.1101/2022.02.22.481508
Dufour, A., Seibt, J., Passante, L., Depaepe, V., Ciossek, T., Frisén, J., et al. (2003). Area specificity and topography of thalamocortical projections are controlled by ephrin/Eph genes. Neuron 39, 453–465. doi: 10.1016/S0896-6273(03)00440-9
Fernandez, L. M. J., Vantomme, G., Osorio-Forero, A., Cardis, R., Béard, E., and Lüthi, A. (2018). Thalamic reticular control of local sleep in mouse sensory cortex. eLife 7:e39111. doi: 10.7554/ELIFE.39111
Ferran, J. L., de Oliveira, E. D., Merchán, P., Sandoval, J. E., Sánchez-Arrones, L., Martínez-de-la-Torre, M., et al. (2009). Genoarchitectonic profile of developing nuclear groups in the chicken pretectum. J. Comp. Neurol. 517, 405–451. doi: 10.1002/CNE.22115
Fujiyama, T., Yamada, M., Terao, M., Terashima, T., Hioki, H., Inoue, Y. U., et al. (2009). Inhibitory and excitatory subtypes of cochlear nucleus neurons are defined by distinct bHLH transcription factors, Ptf1a and Atoh1. Development 136, 2049–2058. doi: 10.1242/DEV.033480
Garel, S., and Rubenstein, J. L. R. (2004). Intermediate targets in formation of topographic projections: Inputs from the thalamocortical system. Trends Neurosci. 27, 533–539. doi: 10.1016/J.TINS.2004.06.014
Golding, B., Pouchelon, G., Bellone, C., Murthy, S., di Nardo, A. A., Govindan, S., et al. (2014). Retinal input directs the recruitment of inhibitory interneurons into thalamic visual circuits. Neuron 81, 1057–1069. doi: 10.1016/j.neuron.2014.01.032
Grant, E., Hoerder-Suabedissen, A., and Molnár, Z. (2012). Development of the corticothalamic projections. Front. Neurosci. 6:53. doi: 10.3389/FNINS.2012.00053
Grant, E., Hoerder-Suabedissen, A., and Molnar, Z. (2016). The regulation of corticofugal fiber targeting by retinal inputs. Cereb. Cortex 26, 1336–1348. doi: 10.1093/CERCOR/BHV315
Guo, Q., and Li, J. Y. H. (2019). Defining developmental diversification of diencephalon neurons through single cell gene expression profiling. Development 146:dev174284. doi: 10.1242/DEV.174284
Gurung, B., and Fritzsch, B. (2004). Time course of embryonic midbrain and thalamic auditory connection development in mice as revealed by carbocyanine dye tracing. J. Comp. Neurol. 479, 309–327. doi: 10.1002/CNE.20328
Halassa, M. M., and Acsády, L. (2016). Thalamic inhibition: Diverse sources, diverse scales. Trends Neurosci. 39, 680–693. doi: 10.1016/j.tins.2016.08.001
Halassa, M. M., and Sherman, S. M. (2019). Thalamocortical circuit motifs: A general framework. Neuron 103, 762–770. doi: 10.1016/J.NEURON.2019.06.005
Hanganu-Opatz, I. L. (2010). Between molecules and experience: Role of early patterns of coordinated activity for the development of cortical maps and sensory abilities. Brain Res. Rev. 64, 160–176. doi: 10.1016/J.BRAINRESREV.2010.03.005
Hannan, A. J., Blakemore, C., Katsnelson, A., Vitalis, T., Huber, K. M., Bear, M., et al. (2001). PLC-beta1, activated via mGluRs, mediates activity-dependent differentiation in cerebral cortex. Nat. Neurosci. 4, 282–288. doi: 10.1038/85132
Harrington, M. E. (1997). The ventral lateral geniculate nucleus and the intergeniculate leaflet: Interrelated structures in the visual and circadian systems. Neurosci. Biobehav. Rev. 21, 705–727. doi: 10.1016/S0149-7634(96)00019-X
Hashimoto-Torii, K., Motoyama, J., Hui, C. C., Kuroiwa, A., Nakafuku, M., and Shimamura, K. (2003). Differential activities of Sonic hedgehog mediated by Gli transcription factors define distinct neuronal subtypes in the dorsal thalamus. Mech. Dev. 120, 1097–1111. doi: 10.1016/j.mod.2003.09.001
Hayes, S. G., Murray, K. D., and Jones, E. G. (2003). Two epochs in the development of gamma-aminobutyric acidergic neurons in the ferret thalamus. J. Comp. Neurol. 463, 45–65. doi: 10.1002/CNE.10749
Hensch, T. K. (2004). Critical period regulation. Annu. Rev. Neurosci. 27, 549–579. doi: 10.1146/ANNUREV.NEURO.27.070203.144327
Herrmann, K., and Shatz, C. J. (1995). Blockade of action potential activity alters initial arborization of thalamic axons within cortical layer 4. Proc. Natl. Acad. Sci. U.S.A. 92, 11244–11248. doi: 10.1073/PNAS.92.24.11244
Hevner, R. F., Miyashita-Lin, E., and Rubenstein, J. L. R. (2002). Cortical and thalamic axon pathfinding defects in Tbr1, Gbx2, and Pax6 mutant mice: Evidence that cortical and thalamic axons interact and guide each other. J. Comp. Neurol. 447, 8–17. doi: 10.1002/CNE.10219
Hirsch, J. A., Wang, X., Sommer, F. T., and Martinez, L. M. (2015). How inhibitory circuits in the thalamus serve vision. Annu. Rev. Neurosci. 38, 309–329. doi: 10.1146/annurev-neuro-071013-014229
Hoerder-Suabedissen, A., and Molnár, Z. (2015). Development, evolution and pathology of neocortical subplate neurons. Nat. Rev. Neurosci. 16, 133–146. doi: 10.1038/NRN3915
Hoerder-Suabedissen, A., Hayashi, S., Upton, L., Nolan, Z., Casas-Torremocha, D., Grant, E., et al. (2018). Subset of cortical layer 6b neurons selectively innervates higher order thalamic nuclei in mice. Cereb. Cortex 28, 1882–1897. doi: 10.1093/CERCOR/BHY036
Hoshino, M., Nakamura, S., Mori, K., Kawauchi, T., Terao, M., Nishimura, Y. V., et al. (2005). Ptf1a, a bHLH transcriptional gene, defines GABAergic neuronal fates in cerebellum. Neuron 47, 201–213. doi: 10.1016/J.NEURON.2005.06.007
Huberman, A. D., Feller, M. B., and Chapman, B. (2008). Mechanisms underlying development of visual maps and receptive fields. Annu. Rev. Neurosci. 31, 479–509. doi: 10.1146/ANNUREV.NEURO.31.060407.125533
Inamura, N., Kimura, T., Tada, S., Kurahashi, T., Yanagida, M., Yanagawa, Y., et al. (2012). Intrinsic and extrinsic mechanisms control the termination of cortical interneuron migration. J. Neurosci. 32, 6032–6042. doi: 10.1523/jneurosci.3446-11.2012
Inamura, N., Ono, K., Takebayashi, H., Zalc, B., and Ikenaka, K. (2011). Olig2 lineage cells generate GABAergic neurons in the prethalamic nuclei, including the zona incerta, ventral lateral geniculate nucleus and reticular thalamic nucleus. Dev. Neurosci. 33, 118–129. doi: 10.1159/000328974
Iwasato, T., Datwani, A., Wolf, A. M., Nishiyama, H., Taguchi, Y., Tonegawa, S., et al. (2000). Cortex-restricted disruption of NMDAR1 impairs neuronal patterns in the barrel cortex. Nature 406, 726–731. doi: 10.1038/35021059
Jacobs, E. C., Campagnoni, C., Kampf, K., Reyes, S. D., Kalra, V., Handley, V., et al. (2007). Visualization of corticofugal projections during early cortical development in a tau-GFP-transgenic mouse. Eur. J. Neurosci. 25, 17–30. doi: 10.1111/J.1460-9568.2006.05258.X
Jager, P., Moore, G., Calpin, P., Durmishi, X., Salgarella, I., Menage, L., et al. (2021). Dual midbrain and forebrain origins of thalamic inhibitory interneurons. eLife 10, 1–29. doi: 10.7554/ELIFE.59272
Jager, P., Ye, Z., Yu, X., Zagoraiou, L., Prekop, H. T., Partanen, J., et al. (2016). Tectal-derived interneurons contribute to phasic and tonic inhibition in the visual thalamus. Nat. Commun. 7:13579. doi: 10.1038/ncomms13579
Jeong, Y., Dolson, D. K., Waclaw, R. R., Matise, M. P., Sussel, L., Campbell, K., et al. (2011). Spatial and temporal requirements for sonic hedgehog in the regulation of thalamic interneuron identity. Development 138, 531–541. doi: 10.1242/dev.058917
Jhaveri, S., Edwards, M. A., and Schneider, G. E. (1991). Initial stages of retinofugal axon development in the hamster: Evidence for two distinct modes of growth. Exp. Brain Res. 87, 371–382. doi: 10.1007/BF00231854
Jones, E. G. (2002). Thalamic circuitry and thalamocortical synchrony. Philos. Trans. R. Soc. Lond. Ser. B Biol. Sci. 357, 1659–1673. doi: 10.1098/RSTB.2002.1168
Kala, K., Haugas, M., Lilleväli, K., Guimera, J., Wurst, W., Salminen, M., et al. (2009). Gata2 is a tissue-specific post-mitotic selector gene for midbrain GABAergic neurons. Development 136, 253–262. doi: 10.1242/DEV.029900
Kanold, P. O., and Luhmann, H. J. (2010). The subplate and early cortical circuits. Annu. Rev. Neurosci. 33, 23–48. doi: 10.1146/ANNUREV-NEURO-060909-153244
Kataoka, A., and Shimogori, T. (2008). Fgf8 controls regional identity in the developing thalamus. Development 135, 2873–2881. doi: 10.1242/DEV.021618
Kiecker, C., and Lumsden, A. (2004). Hedgehog signaling from the ZLI regulates diencephalic regional identity. Nat. Neurosci. 7, 1242–1249. doi: 10.1038/NN1338
Le, T. N., Zhou, Q. P., Cobos, I., Zhang, S., Zagozewski, J., Japoni, S., et al. (2017). GABAergic interneuron differentiation in the basal forebrain is mediated through direct regulation of glutamic acid decarboxylase isoforms by Dlx homeobox transcription factors. J. Neurosci. 37, 8816–8829. doi: 10.1523/JNEUROSCI.2125-16.2017
Lee, C. C., and Murray Sherman, S. (2010). Drivers and modulators in the central auditory pathways. Front. Neurosci. 4:79. doi: 10.3389/NEURO.01.014.2010
Leyva-Díaz, E., del Toro, D., Menal, M. J., Cambray, S., Susín, R., Tessier-Lavigne, M., et al. (2014). FLRT3 is a Robo1-interacting protein that determines Netrin-1 attraction in developing axons. Curr. Biol. 24, 494–508. doi: 10.1016/J.CUB.2014.01.042
Li, H., Fertuzinhos, S., Mohns, E., Hnasko, T. S., Verhage, M., Edwards, R., et al. (2013). Laminar and columnar development of barrel cortex relies on thalamocortical neurotransmission. Neuron 79, 970–986. doi: 10.1016/J.NEURON.2013.06.043
Li, K., Zhang, J., and Li, J. Y. H. (2012). Gbx2 plays an essential but transient role in the formation of thalamic nuclei. PLoS One 7:e47111. doi: 10.1371/JOURNAL.PONE.0047111
Lindtner, S., Catta-Preta, R., Tian, H., Su-Feher, L., Price, J. D., Dickel, D. E., et al. (2019). Genomic resolution of DLX-orchestrated transcriptional circuits driving development of forebrain GABAergic neurons. Cell Rep. 28, 2048–2063.e8. doi: 10.1016/J.CELREP.2019.07.022
Little, G. E., López-Bendito, G., Rünker, A. E., García, N., Piñon, M. C., Chédotal, A., et al. (2009). Specificity and plasticity of thalamocortical connections in Sema6A mutant mice. PLoS Biol. 7:e98. doi: 10.1371/JOURNAL.PBIO.1000098
López-Bendito, G., and Molnár, Z. (2003). Thalamocortical development: How are we going to get there? Nat. Rev. Neurosci. 4, 276–289. doi: 10.1038/NRN1075
López-Bendito, G., Cautinat, A., Sánchez, J. A., Bielle, F., Flames, N., Garratt, A. N., et al. (2006). Tangential neuronal migration controls axon guidance: A role for neuregulin-1 in thalamocortical axon navigation. Cell 125, 127–142. doi: 10.1016/J.CELL.2006.01.042
López-Bendito, G., Flames, N., Ma, L., Fouquet, C., di Meglio, T., Chedotal, A., et al. (2007). Robo1 and Robo2 cooperate to control the guidance of major axonal tracts in the mammalian forebrain. J. Neurosci. 27, 3395–3407. doi: 10.1523/JNEUROSCI.4605-06.2007
Mallika, C., Guo, Q., and Li, J. Y. H. (2015). Gbx2 is essential for maintaining thalamic neuron identity and repressing habenular characters in the developing thalamus. Dev. Biol. 407, 26–39. doi: 10.1016/J.YDBIO.2015.08.010
Marcos-Mondéjar, P., Peregrín, S., Li, J. Y., Carlsson, L., Tole, S., and López-Bendito, G. (2012). The lhx2 transcription factor controls thalamocortical axonal guidance by specific regulation of robo1 and robo2 receptors. J. Neurosci. 32, 4372–4385. doi: 10.1523/JNEUROSCI.5851-11.2012
Marques-Smith, A., Lyngholm, D., Kaufmann, A. K., Stacey, J. A., Hoerder-Suabedissen, A., Becker, E. B. E., et al. (2016). A transient translaminar GABAergic interneuron circuit connects thalamocortical recipient layers in neonatal somatosensory cortex. Neuron 89, 536–549. doi: 10.1016/j.neuron.2016.01.015
Martinez-Ferre, A., and Martinez, S. (2009). The development of the thalamic motor learning area is regulated by Fgf8 expression. J. Neurosci. 29, 13389–13400. doi: 10.1523/JNEUROSCI.2625-09.2009
Martinez-Ferre, A., and Martinez, S. (2012). Molecular regionalization of the diencephalon. Front. Neurosci. 6:73. doi: 10.3389/FNINS.2012.00073
Martini, F. J., Guillamón-Vivancos, T., Moreno-Juan, V., Valdeolmillos, M., and López-Bendito, G. (2021). Spontaneous activity in developing thalamic and cortical sensory networks. Neuron 109, 2519–2534. doi: 10.1016/j.neuron.2021.06.026
McConnell, S. K., Ghosh, A., and Shatz, C. J. (1989). Subplate neurons pioneer the first axon pathway from the cerebral cortex. Science 245, 978–982. doi: 10.1126/SCIENCE.2475909
Métin, C., and Godement, P. (1996). The ganglionic eminence may be an intermediate target for corticofugal and thalamocortical axons. J. Neurosci. 16, 3219–3235. doi: 10.1523/JNEUROSCI.16-10-03219.1996
Miko, I. J., Henkemeyer, M., and Cramer, K. S. (2008). Auditory brainstem responses are impaired in EphA4 and ephrin-B2 deficient mice. Hear. Res. 235, 39–46. doi: 10.1016/J.HEARES.2007.09.003
Mire, E., Mezzera, C., Leyva-Diáz, E., Paternain, A. V., Squarzoni, P., Bluy, L., et al. (2012). Spontaneous activity regulates Robo1 transcription to mediate a switch in thalamocortical axon growth. Nat. Neurosci. 15, 1134–1143. doi: 10.1038/NN.3160
Molnár, Z., and Cordery, P. (1999). Connections between cells of the internal capsule, thalamus, and cerebral cortex in embryonic rat. J. Comp. Neurol. 413, 1–25.
Molnár, Z., Garel, S., López-Bendito, G., Maness, P., and Price, D. J. (2012). Mechanisms controlling the guidance of thalamocortical axons through the embryonic forebrain. Eur. J. Neurosci. 35, 1573–1585. doi: 10.1111/J.1460-9568.2012.08119.X
Molyneaux, B. J., Arlotta, P., Menezes, J. R. L., and Macklis, J. D. (2007). Neuronal subtype specification in the cerebral cortex. Nat. Rev. Neurosci. 8, 427–437. doi: 10.1038/NRN2151
Moore, R. Y., Weis, R., and Moga, M. M. (2000). Efferent projections of the intergeniculate leaflet and the ventral lateral geniculate nucleus in the rat. J. Comp. Neurol. 420, 398–418.
Moreno-Juan, V., Aníbal-Martínez, M., Herrero-Navarro, Á, Valdeolmillos, M., Martini, F. J., and López-Bendito, G. (2022). Spontaneous thalamic activity modulates the cortical innervation of the primary visual nucleus of the thalamus. Neuroscience 508, 87–97. doi: 10.1016/J.NEUROSCIENCE.2022.07.022
Moreno-Juan, V., Filipchuk, A., Antón-Bolaños, N., Mezzera, C., Gezelius, H., Andrés, B., et al. (2017). Prenatal thalamic waves regulate cortical area size prior to sensory processing. Nat. Commun. 8:14172. doi: 10.1038/NCOMMS14172
Murata, Y., and Colonnese, M. T. (2016). An excitatory cortical feedback loop gates retinal wave transmission in rodent thalamus. eLife 5:e18816. doi: 10.7554/ELIFE.18816
Murata, Y., and Colonnese, M. T. (2018). Thalamus controls development and expression of arousal states in visual cortex. J. Neurosci. 38, 8772–8786. doi: 10.1523/JNEUROSCI.1519-18.2018
Muroyama, Y., Fujiwara, Y., Orkin, S. H., and Rowitch, D. H. (2005). Specification of astrocytes by bHLH protein SCL in a restricted region of the neural tube. Nature 43, 360–363. doi: 10.1038/NATURE04139
Nakagawa, Y. (2019). Development of the thalamus: From early patterning to regulation of cortical functions. Wiley Interdiscip. Rev. Dev. Biol. 8:e345. doi: 10.1002/WDEV.345
Nakagawa, Y., and O’Leary, D. D. M. (2001). Combinatorial expression patterns of LIM-homeodomain and other regulatory genes parcellate developing thalamus. J. Neurosci. 21, 2711–2725. doi: 10.1523/JNEUROSCI.21-08-02711.2001
Nakagawa, Y., and Shimogori, T. (2012). Diversity of thalamic progenitor cells and postmitotic neurons. Eur. J. Neurosci. 35, 1554–1562. doi: 10.1111/J.1460-9568.2012.08089.X
Newman, E. A., Wu, D., Taketo, M. M., Zhang, J., and Blackshaw, S. (2018). Canonical Wnt signaling regulates patterning, differentiation and nucleogenesis in mouse hypothalamus and prethalamus. Dev. Biol. 442, 236–248. doi: 10.1016/J.YDBIO.2018.07.021
Ono, K., Takebayashi, H., Ikeda, K., Furusho, M., Nishizawa, T., Watanabe, K., et al. (2008). Regional- and temporal-dependent changes in the differentiation of Olig2 progenitors in the forebrain, and the impact on astrocyte development in the dorsal pallium. Dev. Biol. 320, 456–468. doi: 10.1016/J.YDBIO.2008.06.001
Petersen, C. C. H. (2007). The functional organization of the barrel cortex. Neuron 56, 339–355. doi: 10.1016/J.NEURON.2007.09.017
Pfeiffenberger, C., Cutforth, T., Woods, G., Yamada, J., Rentería, R. C., Copenhagen, D. R., et al. (2005). Ephrin-As and neural activity are required for eye-specific patterning during retinogeniculate mapping. Nat. Neurosci. 8, 1022–1027. doi: 10.1038/NN1508
Pouchelon, G., Gambino, F., Bellone, C., Telley, L., Vitali, I., Lüscher, C., et al. (2014). Modality-specific thalamocortical inputs instruct the identity of postsynaptic L4 neurons. Nature 511, 471–474. doi: 10.1038/NATURE13390
Price, D. J., Clegg, J., Duocastella, X. O., Willshaw, D., and Pratt, T. (2012). The importance of combinatorial gene expression in early mammalian thalamic patterning and thalamocortical axonal guidance. Front. Neurosci. 6:37. doi: 10.3389/FNINS.2012.00037
Puelles L, Diaz C, Stühmer T, Ferran JL, Martínez-de la Torre M, Rubenstein, JLR. LacZ-reporter mapping of Dlx5/6 expression and genoarchitectural analysis of the postnatal mouse prethalamus. J. Comp. Neurol. (2021). 529:367–420. doi: 10.1002/CNE.24952
Puelles, L., and Rubenstein, J. L. R. (2003). Forebrain gene expression domains and the evolving prosomeric model. Trends Neurosci. 26, 469–476. doi: 10.1016/S0166-2236(03)00234-0
Quintana-Urzainqui, I., Hernández-Malmierca, P., Clegg, J. M., Li, Z., Kozić, Z., and Price, D. J. (2020). The role of the diencephalon in the guidance of thalamocortical axons in mice. Development 147:dev184523. doi: 10.1242/DEV.184523
Rakic, P. (1991). Experimental manipulation of cerebral cortical areas in primates. Philos. Trans. R Soc. Lond. Ser. B Biol. Sci. 331, 291–294. doi: 10.1098/RSTB.1991.0019
Reichova, I., and Sherman, S. M. (2004). Somatosensory corticothalamic projections: Distinguishing drivers from modulators. J. Neurophysiol. 92, 2185–2197. doi: 10.1152/JN.00322.2004
Schlaggar, B. L., and O’Leary, D. D. M. (1994). Early development of the somatotopic map and barrel patterning in rat somatosensory cortex. J. Comp. Neurol. 346, 80–96. doi: 10.1002/CNE.903460106
Scholpp, S., Delogu, A., Gilthorpe, J., Peukert, D., Schindler, S., and Lumsden, A. (2009). Her6 regulates the neurogenetic gradient and neuronal identity in the thalamus. Proc. Natl. Acad. Sci. U.S.A. 106, 19895–19900. doi: 10.1073/PNAS.0910894106
Scholpp, S., Wolf, O., Brand, M., and Lumsden, A. (2006). Hedgehog signalling from the zona limitans intrathalamica orchestrates patterning of the zebrafish diencephalon. Development 133, 855–864. doi: 10.1242/DEV.02248
Seibt, J., Schuurmans, C., Gradwhol, G., Dehay, C., Vanderhaeghen, P., Guillemot, F., et al. (2003). Neurogenin2 specifies the connectivity of thalamic neurons by controlling axon responsiveness to intermediate target cues. Neuron 39, 439–452. doi: 10.1016/S0896-6273(03)00435-5
Sgaier, S. K., Lao, Z., Villanueva, M. P., Berenshteyn, F., Stephen, D., Turnbull, R. K., et al. (2007). Genetic subdivision of the tectum and cerebellum into functionally related regions based on differential sensitivity to engrailed proteins. Development 134, 2325–2335. doi: 10.1242/DEV.000620
Sherman, S. M. (2017). Functioning of circuits connecting thalamus and cortex. Compr. Physiol. 7, 713–739. doi: 10.1002/CPHY.C160032
Sherman, S. M., and Guillery, R. W. (2002). The role of the thalamus in the flow of information to the cortex. Philos. Trans. R. Soc. Lond. Ser. B Biol. Sci. 357, 1695–1708. doi: 10.1098/RSTB.2002.1161
Shi, W., Xianyu, A., Han, Z., Tang, X., Li, Z., Zhong, H., et al. (2017). Ontogenetic establishment of order-specific nuclear organization in the mammalian thalamus. Nat. Neurosci. 20, 516–528. doi: 10.1038/NN.4519
Sokhadze, G., Seabrook, T. A., and Guido, W. (2018). The absence of retinal input disrupts the development of cholinergic brainstem projections in the mouse dorsal lateral geniculate nucleus 11 Medical and Health Sciences 1109 Neurosciences. Neural Dev. 13:27. doi: 10.1186/s13064-018-0124-7
Su, J., Charalambakis, N. E., Sabbagh, U., Somaiya, R. D., Monavarfeshani, A., Guido, W., et al. (2020). Retinal inputs signal astrocytes to recruit interneurons into visual thalamus. Proc. Natl. Acad. Sci. U.S.A. 117, 2671–2682. doi: 10.1073/PNAS.1913053117
Sumser, A., Mease, R. A., Sakmann, B., and Groh, A. (2017). Organization and somatotopy of corticothalamic projections from L5B in mouse barrel cortex. Proc. Natl. Acad. Sci. U.S.A. 114, 8853–8858. doi: 10.1073/PNAS.1704302114
Suzuki-Hirano, A., Ogawa, M., Kataoka, A., Yoshida, A. C., Itoh, D., Ueno, M., et al. (2011). Dynamic spatiotemporal gene expression in embryonic mouse thalamus. J. Comp. Neurol. 519, 528–543. doi: 10.1002/CNE.22531
Swadlow, H. A., and Alonso, J. M. (2017). Multielectrodes join the connectome. Nat. Methods 14, 847–848. doi: 10.1038/NMETH.4424
Szabó, N. E., Zhao, T., Zhou, X., and Alvarez-Bolado, G. (2009). The role of Sonic hedgehog of neural origin in thalamic differentiation in the mouse. J. Neurosci. 29, 2453–2466. doi: 10.1523/JNEUROSCI.4524-08.2009
Takesian, A. E., Bogart, L. J., Lichtman, J. W., and Hensch, T. K. (2018). Inhibitory circuit gating of auditory critical-period plasticity. Nat. Neurosci. 21, 218–227. doi: 10.1038/s41593-017-0064-2
Theyel, B. B., Lee, C. C., and Sherman, S. M. (2010). Specific and nonspecific thalamocortical connectivity in the auditory and somatosensory thalamocortical slices. Neuroreport 21, 861–864. doi: 10.1097/WNR.0B013E32833D7CEC
Tou, Y. V., Aaker, J., Taniguchi, A., Kazemzadeh, C., Skidmore, J. M., Martin, D. M., et al. (2007). Characterization of progenitor domains in the developing mouse thalamus. J. Comp. Neurol. 505, 73–91. doi: 10.1002/CNE.21467
Tsukano, H., Horie, M., Ohga, S., Takahashi, K., Kubota, Y., Hishida, R., et al. (2017). Reconsidering tonotopic maps in the auditory cortex and lemniscal auditory thalamus in mice. Front. Neural Circuits 11:14. doi: 10.3389/FNCIR.2017.00014
Tuncdemir, S. N., Wamsley, B., Stam, F. J., Osakada, F., Goulding, M., Callaway, E. M., et al. (2016). Early somatostatin interneuron connectivity mediates the maturation of deep layer cortical circuits. Neuron 89, 521–535. doi: 10.1016/j.neuron.2015.11.020
Uesaka, N., Hayano, Y., Yamada, A., and Yamamoto, N. (2007). Interplay between laminar specificity and activity-dependent mechanisms of thalamocortical axon branching. J. Neurosci. 27, 5215–5223. doi: 10.1523/JNEUROSCI.4685-06.2007
Viaene, A. N., Petrof, I., and Murray Sherman, S. (2011). Synaptic properties of thalamic input to the subgranular layers of primary somatosensory and auditory cortices in the mouse. J. Neurosci. 31, 12738–12747. doi: 10.1523/JNEUROSCI.1565-11.2011
Virolainen, S. M., Achim, K., Peltopuro, P., Salminen, M., and Partanen, J. (2012). Transcriptional regulatory mechanisms underlying the GABAergic neuron fate in different diencephalic prosomeres. Development 139, 3795–3805. doi: 10.1242/dev.075192
Vue, T. Y., Bluske, K., Alishahi, A., Yang, L. L., Koyano-Nakagawa, N., Novitch, B., et al. (2009). Sonic hedgehog signaling controls thalamic progenitor identity and nuclei specification in mice. J. Neurosci. 29, 4484–4497. doi: 10.1523/JNEUROSCI.0656-09.2009
Vue, T. Y., Lee, M., Tan, Y. E., Werkhoven, Z., Wang, L., and Nakagawa, Y. (2013). Thalamic control of neocortical area formation in mice. J. Neurosci. 33, 8442–8453. doi: 10.1523/JNEUROSCI.5786-12.2013
Wamsley, B., and Fishell, G. (2017). Genetic and activity-dependent mechanisms underlying interneuron diversity. Nat. Rev. Neurosci. 18, 299–309. doi: 10.1038/NRN.2017.30
Wong, F. K., Bercsenyi, K., Sreenivasan, V., Portalés, A., Fernández-Otero, M., and Marín, O. (2018). Pyramidal cell regulation of interneuron survival sculpts cortical networks. Nature 557, 668–673. doi: 10.1038/s41586-018-0139-6
Keywords: thalamus, development, mouse, thalamocortical, interneurons
Citation: Huerga-Gómez I, Martini FJ and López-Bendito G (2023) Building thalamic neuronal networks during mouse development. Front. Neural Circuits 17:1098913. doi: 10.3389/fncir.2023.1098913
Received: 15 November 2022; Accepted: 18 January 2023;
Published: 03 February 2023.
Edited by:
Edward S. Ruthazer, McGill University, CanadaReviewed by:
Fernando Garcia-Moreno, Achucarro Basque Center for Neuroscience, SpainCopyright © 2023 Huerga-Gómez, Martini and López-Bendito. This is an open-access article distributed under the terms of the Creative Commons Attribution License (CC BY). The use, distribution or reproduction in other forums is permitted, provided the original author(s) and the copyright owner(s) are credited and that the original publication in this journal is cited, in accordance with accepted academic practice. No use, distribution or reproduction is permitted which does not comply with these terms.
*Correspondence: Francisco J. Martini, Zm1hcnRpbmlAdW1oLmVz; Guillermina López-Bendito, Zy5sYmVuZGl0b0B1bWguZXM=
Disclaimer: All claims expressed in this article are solely those of the authors and do not necessarily represent those of their affiliated organizations, or those of the publisher, the editors and the reviewers. Any product that may be evaluated in this article or claim that may be made by its manufacturer is not guaranteed or endorsed by the publisher.
Research integrity at Frontiers
Learn more about the work of our research integrity team to safeguard the quality of each article we publish.