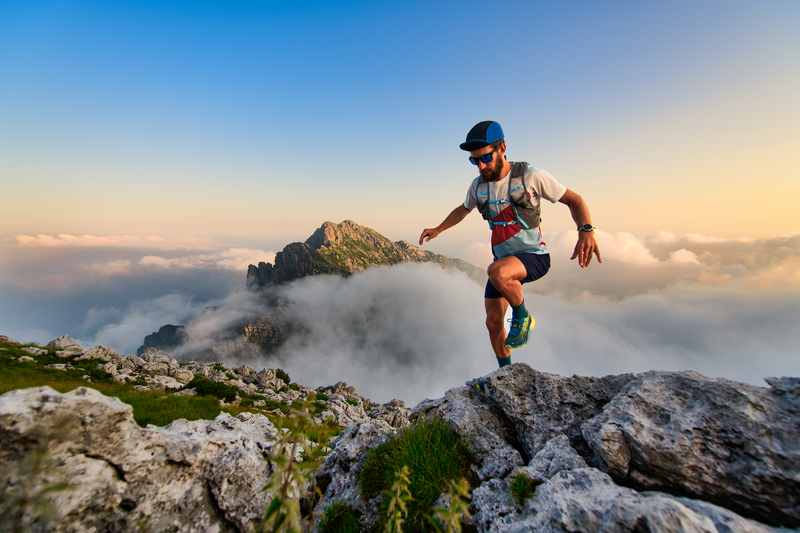
95% of researchers rate our articles as excellent or good
Learn more about the work of our research integrity team to safeguard the quality of each article we publish.
Find out more
SYSTEMATIC REVIEW article
Front. Neural Circuits , 05 October 2022
Volume 16 - 2022 | https://doi.org/10.3389/fncir.2022.984802
This article is part of the Research Topic GABAergic Circuits in Health and Disease View all 11 articles
Under physiological conditions, neuronal network synchronization leads to different oscillatory EEG patterns that are associated with specific behavioral and cognitive functions. Excessive synchronization can, however, lead to focal or generalized epileptiform activities. It is indeed well established that in both epileptic patients and animal models, focal epileptiform EEG patterns are characterized by interictal and ictal (seizure) discharges. Over the last three decades, employing in vitro and in vivo recording techniques, several experimental studies have firmly identified a paradoxical role of GABAA signaling in generating interictal discharges, and in initiating—and perhaps sustaining—focal seizures. Here, we will review these experiments and we will extend our appraisal to evidence suggesting that GABAA signaling may also contribute to epileptogenesis, i.e., the development of plastic changes in brain excitability that leads to the chronic epileptic condition. Overall, we anticipate that this information should provide the rationale for developing new specific pharmacological treatments for patients presenting with focal epileptic disorders such as mesial temporal lobe epilepsy (MTLE).
Neuronal synchronization reflects the integrated activity occurring over time among neuronal networks that are located in the brain (Niedermeyer and da Silva, 2005). Under physiological conditions, neuronal synchronization results in different EEG oscillations that are associated with specific behavioral states, which include cognitive functions and sleep (Steriade et al., 1990; Buzsáki, 2015). However, neuronal synchronization can become abnormally excessive thus leading to focal (Jefferys et al., 2012; Avoli et al., 2016) and/or generalized epileptic discharges (Timofeev and Steriade, 2004; Crunelli et al., 2012). In this review, we will address the cellular and pharmacological mechanisms that cause the generation of epileptiform discharges in in vivo and in vitro animal models of focal epilepsy as well as in epileptic patients who were investigated with invasive electrophysiological recordings (including single unit activity) before undergoing brain surgery. These studies were performed in limbic brain structures—including the hippocampus, the rhinal cortices and the amygdala—since these areas are known to play a role in mesial temporal lobe epilepsy (MTLE) (Gloor, 1997; Engel et al., 2012).
Interictal discharges or spikes (i.e., short-lasting events with duration less than 1 s and unaccompanied by any detectable clinical symptom) (Figure 1A) as well as ictal discharges (i.e., periods of abnormal, hypersynchronous activity lasting up to several minutes and thus disrupting normal brain function) (Figure 1B) are recorded in the EEG obtained from animals or patients presenting with a focal epileptic condition such as MTLE (Gloor, 1997; de Curtis and Avanzini, 2001; Jefferys et al., 2012; Avoli et al., 2016). More recently, it has been shown that focal epileptiform activity is accompanied by the occurrence of high frequency oscillations (HFOs) in the EEG
Figure 1. (A) Spontaneous interictal discharges recorded from the entorhinal cortex (EC), the hippocampal CA3 region and the amygdala (Amy) in a pilocarpine-treated epileptic rat. Note that only two interictal spikes are present in all regions. (B) Spontaneous ictal (seizure) discharge recorded in a pilocarpine-treated epileptic animal from the same areas as in (A); low-voltage fast activity (arrows) marks the onset of this seizure. (C) High-frequency oscillations (HFOs, 80–500 Hz) recorded in association with interictal spikes in a pilocarpine-treated epileptic animal. Interictal spikes are visible on the wideband signal (W/B) whereas high-frequency activity is detectable only after filtering the signals between 80 and 200 Hz (Ripples) and between 250 and 500 Hz (Fast ripples).
(field potential) recordings (Figure 1C); HFOs are not visible in standard EEG recordings but can be extracted by amplifying the appropriately filtered signals. Based on their frequency content, they have been categorized in two groups: (i) ripples, which include oscillatory events between 80 and 200 Hz and (ii) fast ripples, i.e., oscillatory events occurring between 250 and 500 Hz (Bragin et al., 1999a,b; Staba et al., 2004; Jirsch et al., 2006; Urrestarazu et al., 2006; Foffani et al., 2007; Ibarz et al., 2010; Lévesque et al., 2011, 2012; Zijlmans et al., 2011). It has been proposed that ripples may represent, mainly, summated IPSPs while fast ripples should mirror synchronized action potential firing generated by principal (glutamatergic) cells (Jefferys et al., 2012; Jiruska et al., 2017), although fast-spiking GABAergic interneurons could also contribute to the generation of fast ripples (Cepeda et al., 2020). To note how interictal and ictal discharges along with HFOs share some common synchronizing mechanisms.
The topic of our review is the surprisingly active role played by GABAA receptor signaling, in focal epileptiform synchronization. GABAA receptors, once activated, open ionotropic anionic channels that are permeable to Cl– and HCO3– (Kaila, 1994). Early clinical evidence indicated that interfering with GABA synthesis leads to convulsions (Coursin, 1954). In addition, experimental studies, which were mainly published in the 1980s, revealed that: (i) several convulsive drugs are GABAA receptor antagonists (Dingledine and Gjerstad, 1980; Schwartzkroin and Prince, 1980; Hablitz, 1984); (ii) inhibition is markedly reduced at the onset of electrographic hippocampal and neocortical seizures (Ben-Ari et al., 1979; Kostopoulos et al., 1983); (iii) functional disconnection of interneurons from excitatory inputs causes a decrease in inhibition in epileptic brains (Sloviter, 1987); (iv) inhibition in human MTLE may be reduced due to deficits in GABA transporter functions or alterations in GABAA receptor subunit composition (McDonald et al., 1991; Johnson et al., 1992; Olsen et al., 1992; Williamson et al., 1995). Therefore, in the early 1990s, weakening of inhibition was considered by the majority of epilepsy researchers as the main mechanism leading to focal interictal and ictal discharges and thus to epileptic disorders. This view has been, however, challenged by several successive studies that will be summarized here. To note, however, that we will limit the focus of our review to experimental studies involving electrophysiology methods as it is not meant to cover studies involving other investigative approaches.
Voskuyl and Albus (1985) were the first investigators to report that a pharmacological procedure that does not decrease GABAA receptor function—i.e., bath application of the K+blocker 4-aminopyridine (4AP)—can induce epileptiform activity in isolated rat hippocampal slices. By employing field potential recordings, they identified the spontaneous occurrence of two types of interictal spikes, with distinct shapes and rates of occurrence (Voskuyl and Albus, 1985). These two types of interictal patterns were confirmed to occur in successive studies in which field and intracellular potentials were simultaneously recorded from hippocampal slices (Perreault and Avoli, 1991, 1992). As shown in Figure 2A, field potential recordings obtained during 4AP application revealed: (i) “slow” interictal spikes occurring simultaneously in CA1, CA3, and dentate gyrus (DG), and (ii) “fast” interictal spikes that originate in CA3 and spread to CA1. Moreover, intracellular recordings from CA3 pyramidal cells demonstrated that “slow” interictal spikes were mirrored by slow depolarizations (which were abolished by GABAA receptor antagonists), while “fast” interictal spikes were associated to intracellular bursts of action potentials riding on depolarizations that were caused by ionotropic glutamatergic currents (Figure 2B; Perreault and Avoli, 1991, 1992). It was also confirmed in these experiments (cf., Buckle and Haas, 1982; Rutecki et al., 1987) that the postsynaptic responses caused by the activation of both GABAA and, presumably, GABAB receptors were not only preserved but greatly increased in amplitude and duration by 4AP (Figure 2C; Perreault and Avoli, 1991); to note as this complex, augmented response was characterized by a pronounced depolarizing component (asterisk in Figure 2C) that may be contributed by HCO3–—an anion that goes through the open GABAA receptor and has an equilibrium potential more positive than Cl– (Grover et al., 1993; Kaila, 1994)—as well as by the transient increase in extracellular [K+] caused by GABAA receptor postsynaptic activation (cf. Kaila et al., 1997). Presumptive ectopic, fractionated action potentials (arrow in Figure 2C) could consistently be recorded during these “slow” stimulus-induced or spontaneous events (Avoli et al., 1998), and this evidence has been confirmed in neocortical interneurons as well (Keros and Hablitz, 2005).
Figure 2. (A) Simultaneous field recordings obtained from CAI, CA3, and dentate gyrus in an adult rat hippocampal slice during 4AP application. Note that two types of spontaneous interictal spikes are spontaneously generated. The first type is long-lasting and less frequent, it is recorded in all three regions, and it is characterized by a late, “slow” wave; the second type is recorded in CA3 and CA1 only, it is characterized by a short burst of population spikes, and it occurs at a “fast” rate. (B) Simultaneous intracellular (top trace) and field potential (bottom trace) recordings obtained from the CA3 subfield during 4AP application. Note that intracellular bursts of action potentials correlated with the “fast” interictal field events, whereas a long-lasting depolarization corresponded to the “slow” interictal field event. (C) Responses to Schaffer collateral electrical stimulation (triangle) recorded intracellularly from a CA3 pyramidal cell under control conditions and in the presence of 4AP. Note that in control the presumptive recurrent hyperpolarizing IPSP lasts approx. 60 ms while, in the presence of 4AP, the same stimulation induces an initial hyperpolarizing IPSP followed by a slow depolarization (asterisk) and a long-lasting (almost 1 s long) hyperpolarization; the arrow indicates a fractionated, presumably ectopic, action potential that arises from the peak of the early hyperpolarizing IPSP. (D) Simultaneous field potential recordings obtained in an extended brain slice from the hippocampal CA3 area, the perirhinal cortex and the insular cortex under control (4AP) conditions and during application of NMDA and non-NMDA ionotropic glutamatergic receptor antagonists (+ CPP + CNQX). Note in the inset that during ionotropic glutamatergic receptor antagonism, “slow” field potentials continue to occur independently in CA3 from those seen quasi synchronously in PC and IC. (E) Under experimental conditions similar to those described for (D) (+ CPP + CNQX), addition of the GABAA receptor antagonist picrotoxin abolishes the presumptive, “slow” GABAergic field potentials in all areas of the brain slice. (F) Epileptiform activity induced by 4AP arterial application in the piriform cortex (PC), lateral entorhinal cortex (l-EC), hippocampal CA1 subfield, and medial entorhinal cortex (m-EC) of the isolated brain preparation is greatly reduced by ionotropic glutamatergic receptor antagonists (+ DNQX + AP5). The residual field potential events are then abolished by further administration of the GABAA receptor antagonist bicuculline (BIC). (A,B) Are modified from Perreault and Avoli (1992); (C) is modified from Perreault and Avoli (1991); (D,E) are modified from Sudbury and Avoli (2007); (F) is modified from Uva et al. (2009).
The two types of 4AP-induced interictal spikes were later recorded in extended brain slices—which included the hippocampus proper and other limbic or para-limbic areas such as the entorhinal/perirhinal cortices, the amygdala and the insular cortex (Figure 2D; Avoli et al., 1996a,b; Sudbury and Avoli, 2007)—as well as in the in vitro guinea pig isolated brain (Figure 2F; Uva et al., 2009). These studies (see also Morris et al., 1996; Lamsa and Kaila, 1997) have demonstrated that “fast” interictal spikes are abolished by ionotropic glutamatergic antagonists, a pharmacological procedure that does not appear to influence the recurrence of “slow” interictal spikes (Figures 2D,F), which are, however, eliminated by application of the GABAA; receptor antagonists picrotoxin (Figure 2E) or bicuculline (Figure 2F) as well as by activating μ-opioid receptors (Avoli et al., 1996a,b); this pharmacological procedure abolishes the presynaptic release of GABA (Capogna et al., 1993).
As shown in Figures 2D,F, slow, glutamatergic independent, interictal events continued to propagate through the extended brain slice and in the guinea pig isolated brain. As further discussed below, such propagation may depend on the increases in extracellular [K+] that accompany the slow interictal spikes induced by 4AP. To note as two types of interictal spikes have been identified in in vivo EEG recordings obtained from epileptic animals, and have been thereafter termed “type 1” and “type 2” (Bortel et al., 2010; Chauvière et al., 2012; Salami et al., 2014; Lévesque et al., 2021b). It should also be emphasized that preservation of inhibition is present in several in vitro models of epileptiform interictal synchronization such as those induced by application of Mg2+ free-medium (Mody et al., 1987; Tancredi et al., 1990), high K+ medium (Rutecki et al., 1985) or tetraethylammonium (Rutecki et al., 1990).
The likely role played by elevations in extracellular [K+] in the spread of the “slow,” mainly GABAergic, interictal spikes recorded during application of 4AP and ionotropic glutamatergic antagonists was originally proposed by Perreault and Avoli (1992). Shortly before, Barolet and Morris (1991) had discovered that GABAA receptor activation, resulting from the application of exogenous GABA or the GABAA receptor agonist THIP, led to increases in extracellular [K+] even when voltage-gated Na+ channels were blocked by tetrodotoxin, thus excluding any relevant contribution of action potential firing to such elevations in extracellular [K+]. As illustrated in Figure 3A, a few years later, Morris et al. (1996) reported that the “slow,” 4AP-induced spikes recorded from different regions of the isolated, adult rat hippocampal slice are mirrored by increases in extracellular [K+] that continue to occur in the presence of the ionotropic glutamate receptor antagonists 6- cyano-7-nitroquinoxalone-2,3-dione (CNQX) and DL-2- amino-5-phosphonovaleric acid (APV); however, these field events—along with their associated increases in extracellular [K+]—were reversibly blocked by the GABAA receptor antagonist bicuculline methiodide (BMI). Similar data have been obtained in successive studies that were aimed at analyzing the elevations in extracellular [K+] associated to the “slow” interictal spikes induced by 4AP in slices of the rat hippocampus (Avoli et al., 1996b; Lamsa and Kaila, 1997), the rat or mouse entorhinal cortex (Avoli et al., 1996a; Librizzi et al., 2017) and the human neocortex (Louvel et al., 2001; D’Antuono et al., 2004). Extracellular [K+] elevations associated to GABAA receptor-mediated spikes were also shown to occur in the entorhinal cortex of the in vitro isolated whole guinea pig brain (Librizzi et al., 2017). Overall, these data indicate that slow interictal spikes induced by 4AP mainly result from synchronous firing of interneurons that causes massive release of GABA, subsequent activation of post-synaptic GABAA receptors and thus sizeable increases in extracellular [K+] through the activation of the KCC2 cotransporter (Viitanen et al., 2010).
Figure 3. (A) Simultaneous extracellular [K+] and field potential recordings obtained from the CA1 subfield of an adult rat hippocampal slice during 4AP (Control) and successive application of ionotropic glutamatergic receptor antagonists (+ CNQX + CPP), GABAA receptor antagonist (BMI), and washout (i.e., return to 4AP application for over 3 h); note in control the occurrence of both “slow” (asterisks) and “fast” (arrows) interictal spikes as well as that only the “slow” spikes are associated with sizable increases in extracellular [K+]. Note also that these “slow” spikes continue to occur during CNQX + CPP but are abolished by (BMI). (B) Field potential recordings from the CA3 stratum radiatum of two hippocampal slices obtained from 19 and 30 day-old rats during application of 4AP; note that at day 19, “fast” (arrows) and “slow” (asterisks) interictal spikes occur along with ictal discharges (bars) that are shortly preceded by a low interictal; ictal discharges are, however, not recorded in the experiment performed at postnatal day 30. (C) Simultaneous field potential recordings obtained from the entorhinal cortex (EC) and from the hippocampal the dentate gyrus (DG) and CA1 and CA3 subfields during application of 4AP in an extended brain slice; note that the “slow” (asterisk), along with the subsequent ictal discharge, are recorded from all areas while the “fast” interictal spikes are clearly detected in CA3 only. (D) Simultaneous extracellular [K+] and intracellular recordings from fast-spiking interneurons (IN) and principal cells (PC) in the medial entorhinal cortex at the onset of two ictal discharges occurring during 4AP application. Current-clamp recordings from both IN and PC are shown in (a), while current -clamp and voltage-clamp recordings from and IN and a PC, respectively, are illustrated in (b). Note that in both examples the IN fires action potentials earlier than the PC and that these firings correspond to time-locked elevations in extracellular [K+]; note also in (b) that interneuron firing is mirrored by outward currents in the PC. (E) Effects induced by the μ-opioid receptor agonist DAGO on the epileptiform activity recorded from the CA3 of a 15-day-old rat brain slice during 4AP application; note that the negative-going, “slow” spikes and the subsequent ictal activity along with the associated increases in extracellular [K+] are abolished by DAGO, and are replaced by continuous “fast” interictal events. (A) Is modified from Morris et al. (1996); (B) is modified from Avoli (1990); (C) is modified from Avoli et al. (1996a); (D) is modified from Librizzi et al. (2017); (E) is modified from Avoli et al. (1996b).
A turning point on the role played by GABAA receptor signaling in epileptiform synchronization coincided with the discovery that the onset of ictal discharges recorded from juvenile (15–22 day-old) rat hippocampal slices during 4AP application, is shortly preceded, and thus presumably caused by a field event that resemble the “slow” GABAergic spike (asterisk in Figure 3B, 19 day-old field recording) (Avoli, 1990; Avoli et al., 1993, 1996b); to note how this interictal-ictal pattern disappeared with brain maturation to be replaced by a continuous pattern of “fast” and “slow” interictal spikes (Figure 3B, 30 day-old field recording) (cf. also Psarropoulou and Avoli, 1996). However, successive in vitro studies, which were performed in extended brain slices and in the isolated guinea pig brain revealed that ictal (seizure-like) discharges can occur in adult brain tissue during 4AP application as well as that they are initiated and presumably maintained by GABAA receptor signaling (Figure 3C; Avoli et al., 1996a,2004; Sudbury and Avoli, 2007; Carriero et al., 2010; Uva et al., 2013, 2015; Librizzi et al., 2017). The role of GABAA receptor signaling in the initiation of seizure-like activity has been confirmed by computational studies; Kurbatova et al. (2016) have indeed reported that before seizure onset, high frequency firing of GABAergic interneurons generates an increase of the depolarizing GABAA onto pyramidal cells, which induces a massive drop of inhibition that may allow seizure initiation. Interestingly, it has been also shown that the fast activity that occurs at seizure onset and characterizes low-voltage fast onset seizures is associated with interneuron firing, while pyramidal cells remain silent. Employing a biophysically network model (González et al., 2018), have also reported, that seizure-like activity triggered by interneuron firing would not depend on depolarizing GABAA signaling, but would instead rely on an increase of intracellular [Cl–], which is sufficient for KCC2 activation, the subsequent accumulation of extracellular [K+] and the development of epileptiform activity (González et al., 2018).
In line with the mechanism discussed above (i.e., that interneuron firing leading to GABAA receptor activation does, in turn, cause sizeable elevations in extracellular [K+]), several studies have reported that the initial (sentinel) spikes preceding the ictal events induced by 4AP is associated with interneuron action potential firing along with a large increase in extracellular [K+] (Avoli et al., 1996a,b; Ziburkus et al., 2006; Lévesque et al., 2016; Librizzi et al., 2017). This aspect is further illustrated in Figure 3D. First, double patch-clamp recordings of an interneuron and a principal cell in mouse entorhinal cortex slices demonstrated that interneuron burst discharges coupled with IPSPs (Figure 3Da) or IPSCs (Figure 3Db) in principal neurons occur at the onset of 4AP-induced ictal activity; second, such “pre-ictal” patterns were associated with rises in extracellular [K+] that were closely related to interneuron firing and further enhanced by the ensuing recruitment of neuronal networks into the seizure activity. These results firmly support the view that elevations in extracellular [K+] are caused by interneuron firing, which consistently precedes the initiation of ictal events as well as that these extracellular [K+] elevations contribute to seizure precipitation. Interestingly, the emergence of seizure-like activity during extracellular [K+] perturbations has been demonstrated by a realistic computational model of cortical networks (Fröhlich et al., 2010).
It is well known that elevating extracellular [K+] induces neuronal hyperexcitability along with seizure activity (Zuckermann and Glaser, 1968). Successive studies have demonstrated that increased extracellular [K+] causes a positive shift of the membrane reversal of the GABAA receptor-mediated currents thus weakening inhibition (Jensen et al., 1993); it has also been shown that neuronal network resonance, which leads to oscillatory patterns in the beta-gamma range, emerges during increased extracellular [K+] (Bartos et al., 2007). These data are therefore in line with the role played by GABAA receptor activation in promoting epileptiform synchronization and thus seizure-like activity. To be emphasized as pharmacological procedures that interfere with GABAA signaling (e.g., GABAA receptor antagonists or μ-opioid receptor agonists) halt ictal discharges induced in vitro by 4AP and replace them with a pattern of recurring, short-lasting interictal spikes (Figure 3E; Avoli et al., 1996a,b, 2004; Sudbury and Avoli, 2007). In human epileptic tissue, blockade of GABAA receptors also halts interictal discharges (Cohen et al., 2002; Blauwblomme et al., 2019) or modifies their spatial propagation (Sabolek et al., 2012). Suppression of interictal discharges can also be obtained with the application of the NKCC1 blocker bumetanide, in brain slices obtained from pediatric patients with focal cortical dysplasia (Blauwblomme et al., 2019) or in slices obtained from patients with temporal lobe epilepsy and hippocampal sclerosis (Huberfeld et al., 2007), therefore suggesting that the depolarizing responses to GABA in a subset of pyramidal cells during interictal spikes results from excessively high intracellular [Cl–]. A depolarizing action of GABA due to altered intracellular [Cl–] homeostasis has also been demonstrated in tissue obtained from pediatric patients with cortical dysplasia (Abdijadid et al., 2015).
The paradoxical role played by GABAA receptors in initiating 4AP-induced ictal (seizure-like) events (cf., de Curtis and Avoli, 2016) has been confirmed by studies in which optogenetic activation of parvalbumin- or somatostatin-positive interneurons was found capable of triggering ictal events with electrographic features similar to those occurring spontaneously (Shiri et al., 2015, 2016; Yekhlef et al., 2015; Lado et al., 2022). As illustrated in Figure 4A, optogenetic activation of parvalbumin-positive interneurons in the entorhinal cortex (panel b) initiates local ictal discharges that are characterized by an onset that is superimposable to what recorded during spontaneous events (panel a); in fact; the onset of both spontaneous and optogenetic-induced ictal events is typified by one-two interictal-like spikes that lead to fast, beta-gamma oscillations, which characterize the initial component of the seizure activity; these electrographic characteristics represent the hallmark of low-voltage fast onset ictal discharges recorded in patients presenting with focal epileptic disorders (Perucca et al., 2014) and in animal models in vivo (Lévesque et al., 2012). Moreover, it was found in these experiments that optogenetic activation of parvalbumin-positive interneurons could evoke slow interictal spikes both during application of 4AP and after blockade of ionotropic excitatory transmission (Figure 4B; Shiri et al., 2016).
Figure 4. (A) Ictal discharges recorded extracellularly from the mouse entorhinal cortex during application of 4AP can occur spontaneously (a) or be triggered by optogenetic activation of parvalbumin interneurons (b). One ictal event for each experimental condition is further expanded to show the onset patterns that are in both cases characterized by 1 or 2 negative-going interictal-like spikes. (B) Blockade of ionotropic glutamatergic receptors (+ CNQX + CPP) abolishes ictal discharges induced by the optogenetic activation of parvalbumin-positive interneurons in the presence of 4AP; however, under these experimental conditions optogenetic stimuli continue to evoke slow interictal spike. (A,B) Are modified from Shiri et al. (2016). The onset of the ictal discharge (*) is shown on an expanded time scale in the inset.
The surprisingly active role played by GABAA signaling in initiating and, perhaps, sustaining seizure activity in vitro has been identified under different experimental conditions, including perfusion of low doses of bicuculline in the isolated guinea pig brain (Gnatkovsky et al., 2008), perfusion of brain slices with Mg2+ free medium (Köhling et al., 2000), or high frequency electrical stimuli (Velazquez and Carlen, 1999; Fujiwara-Tsukamoto et al., 2010). The contribution of GABAA receptors to epileptiform synchronization is also supported by the ability of CA1 hippocampal networks in vitro to generate prolonged discharges following pharmacological blockade of both GABAB and ionotropic glutamatergic receptors (Uusisaari et al., 2002). Evidence obtained from in vivo models of MTLE have also shown that increased activity of GABA releasing interneurons (which in turns silences principal neurons) coincides with the onset of focal seizures (Grasse et al., 2013; Fujita et al., 2014; Toyoda et al., 2015; Karunakaran et al., 2016). Last but not least, seizure onsets recorded from epileptic patients undergoing presurgical depth electrode investigations, is associated with increased interneuron firing and marked reduction of principal cell excitability (Truccolo et al., 2011; Schevon et al., 2012; Elahian et al., 2018).
The kainic acid (KA) (Lévesque and Avoli, 2013) and the pilocarpine models of MTLE (Lévesque et al., 2021a) have been widely used to study how epileptic discharges are generated from mesial temporal lobe structures in vivo. Both models rely on the chemical induction of an initial brain insult (i.e., a status epilepticus, SE), that is followed a few days later by the development of a chronic epileptic condition. GABAA signaling could play a role in ictogenesis in these animal models, since alterations in GABAA receptor function and in GABA releasing interneurons have been reported (Friedman et al., 1994; Schwarzer et al., 1997; Tsunashima et al., 1997; Laurén et al., 2005; Fritsch et al., 2009; Drexel et al., 2013; Dubanet et al., 2021).
In the KA model, spontaneous seizures occurring in epileptic mice can be stopped, and the frequency of seizures with severe behavioral symptoms reduced, when optogenetic activation of ChR2-expressing PV-positive interneurons is performed in the hippocampus ipsilateral or contralateral to the hippocampus that was injected with KA (Krook-Magnuson et al., 2013). Similar findings were obtained by Chen et al. (2021), who used optogenetics in KA-treated epileptic animals to activate hippocampal PV-interneurons expressing ChRmine; this is a red-shifted opsin that exhibits high sensitivity to light stimulation (Marshel et al., 2019), thus making neurons expressing these opsins sensitive to transcranial optogenetic stimulation. The application of on-demand transcranial optogenetic stimulation to these ChRmine-expressing PV-positive interneurons during the chronic period induced a 51% decrease in seizure duration compared to sham treatment. Interestingly, optogenetic activation of PV-positive interneurons in the hippocampus of KA-treated animals also improves performance in cognitive tasks (Kim et al., 2020).
Such anti-ictogenic effect is not restricted to the hippocampus but it is also observed when optogenetic stimulation is applied to PV-expressing Purkinje cells of the cerebellum, a brain structure that is anatomically and functionally connected to the hippocampus (Watson et al., 2018) and that is known to modulate hippocampal function during cognitive tasks (Zeidler et al., 2020). Krook-Magnuson et al. (2014) found that optogenetic excitation or inhibition of PV-expressing Purkinje cells in the lateral or midline cerebellum of KA-treated animals during the chronic period shortens seizure duration. However, it remains unclear through which mechanisms cerebellar optogenetic stimulation controls hippocampal seizures, since both excitation and inhibition of cerebellar Purkinje cells could decrease seizure duration (Krook-Magnuson et al., 2014). Similar anti-ictogenic effects in the KA model resulting from the activation of GABAergic neuronal populations in remote regions were also reported recently by Hristova et al. (2021), who performed optogenetic stimulation of GABAergic populations in the medial septum, a region that sends GABAergic projections to hippocampal GABAergic interneurons (Unal et al., 2015).
In the pilocarpine model, Lévesque et al. (2019) investigated whether continuous, unilateral, optogenetic stimulation of ChR2-expressing PV-positive interneurons in the CA3 subfield of the hippocampus (Figure 5A) could decrease seizure rates in pilocarpine-treated epileptic mice. These results have revealed that activation of PV-ChR2 interneurons at 8 Hz for 30 s every 2 min for 14 continuous days induce a decrease in rates of spontaneous seizures compared to what was observed in PV-Cre (opsin-negative) animals (Figure 5B). Seizure duration (Figure 5C) and proportion of convulsive seizures (Figure 5D) were not decreased by PV optogenetic stimulation; however, rates of interictal spikes (Figure 5E), of interictal spikes with fast ripples (Figure 5F) and of isolated fast ripples (Figure 5G)—which are considered as markers of epileptogenesis (Jefferys et al., 2012)–were significantly lower in the PV-ChR2 group compared to the PV-Cre group.
Figure 5. (A) Schematic diagram showing the location of the optic fiber and electrode in the CA3 region of the hippocampus. The tip of the optic fiber was glued less than 1 mm above the tip of the electrode. Optogenetic stimulation of PV-positive interneurons (8 Hz for 30 s every 2 min) was performed for 14 continuous days, starting 3 days after SE. (B) Average daily rates of spontaneous seizures in PV-ChR2 and PV-Cre animals. PV-ChR2 animals showed significantly less seizures compared to PV-Cre animals (**p < 0.005). (C) Bar graph showing the average duration of seizures in both groups. No significant differences were observed. (D) Proportion of non-convulsive and convulsive seizures in both groups. No significant differences were observed. (E) Bar graph showing rates of interictal spikes in both groups. PV-Cre animals showed significantly higher rates of interictal spikes compared to PV-ChR2 animals (**p < 0.001). (F) Bar graph showing the average ratio of interictal spikes with fast ripples on the total number of interictal spikes for each group. PV-Cre animals showed a higher ratio compared to the PV-ChR2 group (**p < 0.001). A representative example of an interictal spike with a fast ripple is shown on the right. (G) Bar graph showing the average rate of isolated fast ripples in both groups. PV-ChR2 animals showed significantly lower rates of isolated fast ripples compared to PV-Cre animals (*p < 0.01). (H) Example of a spontaneous seizure that was triggered by optogenetic stimulation of PV-positive interneurons (blue rectangle) in a PV-ChR2 animal. Note that oscillations around 8 Hz in the field (arrow) were triggered by light stimulation and that the seizure occurred approximately 25 s after (arrowhead). (I) Cumulative probability curves showing that PV-ChR2 animals are more likely to show seizures between 0 and 30 s after the onset of optogenetic stimulation compared to PV-Cre animals. Modified from Lévesque et al. (2019).
These findings are in line with the evidence obtained by Krook-Magnuson et al. (2013), who reported a decrease in seizure rates in the KA model by using closed-loop activation of PV-positive interneurons. However, Lévesque et al. (2019) also found that the “residual” seizures that continued to occur, could be triggered by optogenetic stimuli (Figures 5H,I). These data are in line with what was reported in vitro (Shiri et al., 2015, 2016; Yekhlef et al., 2015; Chang et al., 2018; Botterill et al., 2019).
The studies reviewed here disclose an unexpected role played by GABAA receptors in epileptiform synchronization including the generation of interictal and ictal (seizure) events. Such paradoxical role depends on the large increases in extracellular [K+] that are caused by KCC2 activation due to massive release of GABA consequent to synchronous firing of inhibitory interneurons (Di Cristo et al., 2018). We have also summarized recent findings suggesting that activation of inhibitory interneurons can exert unexpected effects on the processes associated to epileptogenesis. These results reveal a complex pattern of participating mechanisms. Thus, while synaptic excitation and voltage-gated Na+ channels remain the key components of synchronous epileptiform discharges, GABAA receptors have emerged as surprising, paradoxical players in the generation of interictal spikes and in the initiation and maintenance of prolonged epileptiform phenomena (i.e., to ictogenesis).
The evidence that enhanced GABAA receptor function supports epileptiform synchronization and thus focal seizure generation may explain the disappointingly limited clinical efficacy of some antiepileptic compounds that were “mechanistically” developed to potentiate GABAA signaling during the 1980s and were introduced into clinical practice at the start of the 1990s. These compounds include γ-vinyl-GABA (which inhibits the breakdown of GABA by the enzyme GABA transaminase) (Rogawski and Löscher, 2004), tiagabine (which increases GABA levels by inhibiting GABA reuptake) (Brodie, 1995; Pollack et al., 2005), and progabide (Lloyd et al., 1983; Loiseau et al., 1983). It should also be emphasized that benzodiazepines, which can halt seizure activity and stop status epilepticus (Pang and Hirsch, 2005), increase GABAA receptor function by acting on an allosteric “benzodiazepine site” that is located in most of the α subunit-containing GABAA receptors (Costa et al., 1975; Choi et al., 1977; Olsen, 2015). However, and in line with a synchronizing action of GABA, benzodiazepines have been reported to precipitate seizures, when given intravenously in patients with Lennox-Gastaut syndrome (Perucca et al., 1998).
The original contributions presented in this study are included in the article/supplementary material, further inquiries can be directed to the corresponding author.
MA wrote the early draft of this review. All authors contributed to the manuscript revision, read, and approved the submitted version.
This review was based on experiments that were supported by the Canadian Institutes of Health Research (Grants PJT153310, PJT166178, and MOP130328), CURE and the Savoy Foundation to MA, and the Italian Ministry of Health (Current Research 2021 and Grant RF 2018-12365681), and the Paolo Zorzi Association for Neuroscience (Grant 2021-24 EPICARE project) to MC.
The authors declare that the research was conducted in the absence of any commercial or financial relationships that could be construed as a potential conflict of interest.
All claims expressed in this article are solely those of the authors and do not necessarily represent those of their affiliated organizations, or those of the publisher, the editors and the reviewers. Any product that may be evaluated in this article, or claim that may be made by its manufacturer, is not guaranteed or endorsed by the publisher.
Abdijadid, S., Mathern, G. W., Levine, M. S., and Cepeda, C. (2015). Basic mechanisms of epileptogenesis in pediatric cortical dysplasia. CNS Neurosci. Ther. 21, 92–103. doi: 10.1111/cns.12345
Avoli, M. (1990). Epileptiform discharges and a synchronous GABAergic potential induced by 4-aminopyridine in the rat immature hippocampus. Neurosci. Lett. 117, 93–98. doi: 10.1016/0304-3940(90)90125-s
Avoli, M., Benini, R., de Guzman, P., and Omar, A. (2004). GABA(B) receptor activation and limbic network ictogenesis. Neuropharmacology 46, 43–51. doi: 10.1016/s0028-3908(03)00307-1
Avoli, M., de Curtis, M., Gnatkovsky, V., Gotman, J., Köhling, R., Lévesque, M., et al. (2016). Specific imbalance of excitatory/inhibitory signaling establishes seizure onset pattern in temporal lobe epilepsy. J. Neurophysiol. 115, 3229–3237. doi: 10.1152/jn.01128.2015
Avoli, M., Barbarosie, M., Lücke, A., Nagao, T., Lopantsev, V., and Köhling, R. (1996a). Synchronous GABA-mediated potentials and epileptiform discharges in the rat limbic system in vitro. J. Neurosci. 16, 3912–3924.
Avoli, M., Louvel, J., Kurcewicz, I., Pumain, R., and Barbarosie, M. (1996b). Extracellular free potassium and calcium during synchronous activity induced by 4-aminopyridine in the juvenile rat hippocampus. J. Physiol. 493(Pt 3), 707–717. doi: 10.1113/jphysiol.1996.sp021416
Avoli, M., Methot, M., and Kawasaki, H. (1998). GABA-dependent generation of ectopic action potentials in the rat hippocampus. Eur. J. Neurosci. 10, 2714–2722. doi: 10.1046/j.1460-9568.1998.00275.x
Avoli, M., Psarropoulou, C., Tancredi, V., and Fueta, Y. (1993). On the synchronous activity induced by 4-aminopyridine in the CA3 subfield of juvenile rat hippocampus. J. Neurophysiol. 70, 1018–1029.
Barolet, A. W., and Morris, M. E. (1991). Changes in extracellular K+ evoked by GABA, THIP and baclofen in the guinea-pig hippocampal slice. Exp. Brain Res. 84, 591–598. doi: 10.1007/BF00230971
Bartos, M., Vida, I., and Jonas, P. (2007). Synaptic mechanisms of synchronized gamma oscillations in inhibitory interneuron networks. Nat. Rev. Neurosci. 8, 45–56. doi: 10.1038/nrn2044
Ben-Ari, Y., Krnjević, K., and Reinhardt, W. (1979). Hippocampal seizures and failure of inhibition. Can. J. Physiol. Pharmacol. 57, 1462–1466. doi: 10.1139/y79-218
Blauwblomme, T., Dossi, E., Pellegrino, C., Goubert, E., Iglesias, B. G., Sainte-Rose, C., et al. (2019). Gamma-aminobutyric acidergic transmission underlies interictal epileptogenicity in pediatric focal cortical dysplasia. Ann. Neurol. 85, 204–217. doi: 10.1002/ana.25403
Bortel, A., Lévesque, M., Biagini, G., Gotman, J., and Avoli, M. (2010). Convulsive status epilepticus duration as determinant for epileptogenesis and interictal discharge generation in the rat limbic system. Neurobiol. Dis. 40, 478–489. doi: 10.1016/j.nbd.2010.07.015
Botterill, J. J., Lu, Y.-L., LaFrancois, J. J., Bernstein, H. L., Alcantara-Gonzalez, D., Jain, S., et al. (2019). An excitatory and epileptogenic effect of dentate gyrus mossy cells in a mouse model of epilepsy. Cell Rep. 29, 2875–2889.e6. doi: 10.1016/j.celrep.2019.10.100
Bragin, A., Engel, J. Jr., Wilson, C. L., Fried, I., and Mathern, G. W. (1999a). Hippocampal and entorhinal cortex high-frequency oscillations (100–500 Hz) in human epileptic brain and in kainic acid–treated rats with chronic seizures. Epilepsia 40, 127–137. doi: 10.1111/j.1528-1157.1999.tb02065.x
Bragin, A., Engel, J., Wilson, C. L., Fried, I., and Buzsáki, G. (1999b). High-frequency oscillations in human brain. Hippocampus 9, 137–142. doi: 10.1002/(SICI)1098-106319999:2<137::AID-HIPO5<3.0.CO;2-0
Buckle, P. J., and Haas, H. L. (1982). Enhancement of synaptic transmission by 4-aminopyridine in hippocampal slices of the rat. J. Physiol. 326, 109–122.
Buzsáki, G. (2015). Hippocampal sharp wave-ripple: A cognitive biomarker for episodic memory and planning. Hippocampus 25, 1073–1188. doi: 10.1002/hipo.22488
Capogna, M., Gähwiler, B. H., and Thompson, S. M. (1993). Mechanism of mu-opioid receptor-mediated presynaptic inhibition in the rat hippocampus in vitro. J. Physiol. 470, 539–558. doi: 10.1113/jphysiol.1993.sp019874
Carriero, G., Uva, L., Gnatkovsky, V., Avoli, M., and de Curtis, M. (2010). Independent epileptiform discharge patterns in the olfactory and limbic areas of the in vitro isolated Guinea pig brain during 4-aminopyridine treatment. J. Neurophysiol. 103, 2728–2736. doi: 10.1152/jn.00862.2009
Cepeda, C., Levinson, S., Nariai, H., Yazon, V.-W., Tran, C., Barry, J., et al. (2020). Pathological high frequency oscillations associate with increased GABA synaptic activity in pediatric epilepsy surgery patients. Neurobiol. Dis. 134:104618. doi: 10.1016/j.nbd.2019.104618
Chang, M., Dian, J. A., Dufour, S., Wang, L., Moradi Chameh, H., Ramani, M., et al. (2018). Brief activation of GABAergic interneurons initiates the transition to ictal events through post-inhibitory rebound excitation. Neurobiol. Dis. 109, 102–116. doi: 10.1016/j.nbd.2017.10.007
Chauvière, L., Doublet, T., Ghestem, A., Siyoucef, S. S., Wendling, F., Huys, R., et al. (2012). Changes in interictal spike features precede the onset of temporal lobe epilepsy. Ann. Neurol. 71, 805–814. doi: 10.1002/ana.23549
Chen, R., Gore, F., Nguyen, Q.-A., Ramakrishnan, C., Patel, S., Kim, S. H., et al. (2021). Deep brain optogenetics without intracranial surgery. Nat. Biotechnol. 39, 161–164. doi: 10.1038/s41587-020-0679-9
Choi, D. W., Farb, D. H., and Fischbach, G. D. (1977). Chlordiazepoxide selectively augments GABA action in spinal cord cell cultures. Nature 269, 342–344. doi: 10.1038/269342a0
Cohen, I., Navarro, V., Clemenceau, S., Baulac, M., and Miles, R. (2002). On the origin of interictal activity in human temporal lobe epilepsy in vitro. Science 298, 1418–1421. doi: 10.1126/science.1076510
Costa, E., Guidotti, A., and Mao, C. C. (1975). “Evidence for involvement of GABA in the action of benzodiazepines: Studies on rat cerebellum,” in Advances in biochemical psychopharmacology, Vol. 14, eds E. Costa and P. Greengard (New York, NY: Raven), 113–130.
Coursin, D. B. (1954). Convulsive seizures in infants with pyridoxine-deficient diet. J. Am. Med. Assoc. 154, 406–408. doi: 10.1001/jama.1954.02940390030009
Crunelli, V., Leresche, N., and Cope, D. W. (2012). “GABA-A receptor function in typical absence seizures,” in Jasper’s basic mechanisms of the epilepsies, eds J. L. Noebels, M. Avoli, M. A. Rogawski, R. W. Olsen, and A. V. Delgado-Escueta (Bethesda, MD: National Center for Biotechnology Information (US)).
D’Antuono, M., Louvel, J., Köhling, R., Mattia, D., Bernasconi, A., Olivier, A., et al. (2004). GABAA receptor-dependent synchronization leads to ictogenesis in the human dysplastic cortex. Brain 127, 1626–1640. doi: 10.1093/brain/awh181
de Curtis, M., and Avanzini, G. (2001). Interictal spikes in focal epileptogenesis. Prog. Neurobiol. 63, 541–567.
de Curtis, M., and Avoli, M. (2016). GABAergic networks jump-start focal seizures. Epilepsia 57, 679–687. doi: 10.1111/epi.13370
Di Cristo, G., Awad, P. N., Hamidi, S., and Avoli, M. (2018). KCC2, epileptiform synchronization, and epileptic disorders. Prog. Neurobiol. 162, 1–16. doi: 10.1016/j.pneurobio.2017.11.002
Dingledine, R., and Gjerstad, L. (1980). Reduced inhibition during epileptiform activity in the in vitro hippocampal slice. J. Physiol. 305, 297–313.
Drexel, M., Kirchmair, E., and Sperk, G. (2013). Changes in the expression of GABAA receptor subunit mRNAs in parahippocampal areas after kainic acid induced seizures. Front. Neural Circuits 7:142. doi: 10.3389/fncir.2013.00142
Dubanet, O., Ferreira Gomes Da Silva, A., Frick, A., Hirase, H., Beyeler, A., and Leinekugel, X. (2021). Probing the polarity of spontaneous perisomatic GABAergic synaptic transmission in the mouse CA3 circuit in vivo. Cell Rep. 36:109381. doi: 10.1016/j.celrep.2021.109381
Elahian, B., Lado, N. E., Mankin, E., Vangala, S., Misra, A., Moxon, K., et al. (2018). Low-voltage fast seizures in humans begin with increased interneuron firing. Ann. Neurol. 84, 588–600. doi: 10.1002/ana.25325
Engel, J. Jr., McDermott, M. P., Wiebe, S., Langfitt, J. T., Stern, J. M., Dewar, S., et al. (2012). Early surgical therapy for drug-resistant temporal lobe epilepsy: A randomized trial. J. Am. Med. Assoc. 307, 922–930. doi: 10.1001/jama.2012.220
Foffani, G., Uzcategui, Y. G., Gal, B., and Menendez de la Prida, L. (2007). Reduced spike-timing reliability correlates with the emergence of fast ripples in the rat epileptic hippocampus. Neuron 55, 930–941. doi: 10.1016/j.neuron.2007.07.040
Friedman, L. K., Pellegrini-Giampietro, D. E., Sperber, E. F., Bennett, M. V., Moshé, S. L., and Zukin, R. S. (1994). Kainate-induced status epilepticus alters glutamate and GABAA receptor gene expression in adult rat hippocampus: An in situ hybridization study. J. Neurosci. 14, 2697–2707. doi: 10.1523/JNEUROSCI.14-05-02697.1994
Fritsch, B., Qashu, F., Figueiredo, T. H., Aroniadou-Anderjaska, V., Rogawski, M. A., and Braga, M. F. M. (2009). Pathological alterations in GABAergic interneurons and reduced tonic inhibition in the basolateral amygdala during epileptogenesis. Neuroscience 163, 415–429. doi: 10.1016/j.neuroscience.2009.06.034
Fröhlich, F., Sejnowski, T. J., and Bazhenov, M. (2010). Network bistability mediates spontaneous transitions between normal and pathological brain states. J. Neurosci. 30, 10734–10743. doi: 10.1523/JNEUROSCI.1239-10.2010
Fujita, S., Toyoda, I., Thamattoor, A. K., and Buckmaster, P. S. (2014). Preictal activity of subicular, CA1, and dentate gyrus principal neurons in the dorsal hippocampus before spontaneous seizures in a rat model of temporal lobe epilepsy. J. Neurosci. 34, 16671–16687. doi: 10.1523/JNEUROSCI.0584-14.2014
Fujiwara-Tsukamoto, Y., Isomura, Y., Imanishi, M., Ninomiya, T., Tsukada, M., Yanagawa, Y., et al. (2010). Prototypic seizure activity driven by mature hippocampal fast-spiking interneurons. J. Neurosci. 30, 13679–13689. doi: 10.1523/JNEUROSCI.1523-10.2010
Gnatkovsky, V., Librizzi, L., Trombin, F., and de Curtis, M. (2008). Fast activity at seizure onset is mediated by inhibitory circuits in the entorhinal cortex in vitro. Ann. Neurol. 64, 674–686. doi: 10.1002/ana.21519
González, O. C., Shiri, Z., Krishnan, G. P., Myers, T. L., Williams, S., Avoli, M., et al. (2018). Role of KCC2-dependent potassium efflux in 4-aminopyridine-induced epileptiform synchronization. Neurobiol. Dis. 109, 137–147. doi: 10.1016/j.nbd.2017.10.011
Grasse, D. W., Karunakaran, S., and Moxon, K. A. (2013). Neuronal synchrony and the transition to spontaneous seizures. Exp. Neurol. 248, 72–84. doi: 10.1016/j.expneurol.2013.05.004
Grover, L. M., Lambert, N. A., Schwartzkroin, P. A., and Teyler, T. J. (1993). Role of HCO3- ions in depolarizing GABAA receptor-mediated responses in pyramidal cells of rat hippocampus. J. Neurophysiol. 69, 1541–1555. doi: 10.1152/jn.1993.69.5.1541
Hablitz, J. J. (1984). Picrotoxin-induced epileptiform activity in hippocampus: Role of endogenous versus synaptic factors. J. Neurophysiol. 51, 1011–1027. doi: 10.1152/jn.1984.51.5.1011
Hristova, K., Martinez-Gonzalez, C., Watson, T. C., Codadu, N. K., Hashemi, K., Kind, P. C., et al. (2021). Medial septal GABAergic neurons reduce seizure duration upon optogenetic closed-loop stimulation. Brain J. Neurol. 144, 1576–1589. doi: 10.1093/brain/awab042
Huberfeld, G., Wittner, L., Clemenceau, S., Baulac, M., Kaila, K., Miles, R., et al. (2007). Perturbed chloride homeostasis and GABAergic signaling in human temporal lobe epilepsy. J. Neurosci. 27, 9866–9873. doi: 10.1523/JNEUROSCI.2761-07.2007
Ibarz, J. M., Foffani, G., Cid, E., Inostroza, M., and de la Prida, L. M. (2010). Emergent dynamics of fast ripples in the epileptic hippocampus. J. Neurosci. 30, 16249–16261. doi: 10.1523/JNEUROSCI.3357-10.2010
Jefferys, J. G. R., Menendez de la Prida, L., Wendling, F., Bragin, A., Avoli, M., Timofeev, I., et al. (2012). Mechanisms of physiological and epileptic HFO generation. Prog. Neurobiol. 98, 250–264. doi: 10.1016/j.pneurobio.2012.02.005
Jensen, M. S., Cherubini, E., and Yaari, Y. (1993). Opponent effects of potassium on GABAA-mediated postsynaptic inhibition in the rat hippocampus. J. Neurophysiol. 69, 764–771. doi: 10.1152/jn.1993.69.3.764
Jirsch, J. D., Urrestarazu, E., LeVan, P., Olivier, A., Dubeau, F., and Gotman, J. (2006). High-frequency oscillations during human focal seizures. Brain J. Neurol. 129, 1593–1608. doi: 10.1093/brain/awl085
Jiruska, P., Alvarado-Rojas, C., Schevon, C. A., Staba, R., Stacey, W., Wendling, F., et al. (2017). Update on the mechanisms and roles of high-frequency oscillations in seizures and epileptic disorders. Epilepsia 58, 1330–1339. doi: 10.1111/epi.13830
Johnson, E. W., de Lanerolle, N. C., Kim, J. H., Sundaresan, S., Spencer, D. D., Mattson, R. H., et al. (1992). “Central” and “peripheral” benzodiazepine receptors: Opposite changes in human epileptogenic tissue. Neurology 42, 811–815. doi: 10.1212/wnl.42.4.811
Kaila, K. (1994). Ionic basis of GABAA receptor channel function in the nervous system. Prog. Neurobiol. 42, 489–537.
Kaila, K., Lamsa, K., Smirnov, S., Taira, T., and Voipio, J. (1997). Long-lasting GABA-mediated depolarization evoked by high-frequency stimulation in pyramidal neurons of rat hippocampal slice is attributable to a network-driven, bicarbonate-dependent K+ transient. J. Neurosci. 17, 7662–7672. doi: 10.1523/JNEUROSCI.17-20-07662.1997
Karunakaran, S., Grasse, D. W., and Moxon, K. A. (2016). Role of CA3 theta-modulated interneurons during the transition to spontaneous seizures. Exp. Neurol. 283, 341–352. doi: 10.1016/j.expneurol.2016.06.027
Keros, S., and Hablitz, J. J. (2005). Ectopic action potential generation in cortical interneurons during synchronized GABA responses. Neuroscience 131, 833–842. doi: 10.1016/j.neuroscience.2004.12.010
Kim, H. K., Gschwind, T., Nguyen, T. M., Bui, A. D., Felong, S., Ampig, K., et al. (2020). Optogenetic intervention of seizures improves spatial memory in a mouse model of chronic temporal lobe epilepsy. Epilepsia 61, 561–571. doi: 10.1111/epi.16445
Köhling, R., Vreugdenhil, M., Bracci, E., and Jefferys, J. G. (2000). Ictal epileptiform activity is facilitated by hippocampal GABAA receptor-mediated oscillations. J. Neurosci. 20, 6820–6829. doi: 10.1523/JNEUROSCI.20-18-06820.2000
Kostopoulos, G., Avoli, M., and Gloor, P. (1983). Participation of cortical recurrent inhibition in the genesis of spike and wave discharges in feline generalized penicillin epilepsy. Brain Res. 267, 101–112. doi: 10.1016/0006-8993(83)91043-0
Krook-Magnuson, E., Armstrong, C., Oijala, M., and Soltesz, I. (2013). On-demand optogenetic control of spontaneous seizures in temporal lobe epilepsy. Nat. Commun. 4:1376. doi: 10.1038/ncomms2376
Krook-Magnuson, E., Szabo, G. G., Armstrong, C., Oijala, M., and Soltesz, I. (2014). Cerebellar directed optogenetic intervention inhibits spontaneous hippocampal seizures in a mouse model of temporal lobe epilepsy. eNeuro 1:ENEURO.0005-14.2014. doi: 10.1523/ENEURO.0005-14.2014
Kurbatova, P., Wendling, F., Kaminska, A., Rosati, A., Nabbout, R., Guerrini, R., et al. (2016). Dynamic changes of depolarizing GABA in a computational model of epileptogenic brain: Insight for Dravet syndrome. Exp. Neurol. 283, 57–72. doi: 10.1016/j.expneurol.2016.05.037
Lado, W. E., Xu, X., and Hablitz, J. J. (2022). Modulation of epileptiform activity by three subgroups of GABAergic interneurons in mouse somatosensory cortex. Epilepsy Res. 183:106937. doi: 10.1016/j.eplepsyres.2022.106937
Lamsa, K., and Kaila, K. (1997). Ionic mechanisms of spontaneous GABAergic events in rat hippocampal slices exposed to 4-aminopyridine. J. Neurophysiol. 78, 2582–2591. doi: 10.1152/jn.1997.78.5.2582
Laurén, H. B., Lopez-Picon, F. R., Korpi, E. R., and Holopainen, I. E. (2005). Kainic acid-induced status epilepticus alters GABA receptor subunit mRNA and protein expression in the developing rat hippocampus. J. Neurochem. 94, 1384–1394. doi: 10.1111/j.1471-4159.2005.03274.x
Lévesque, M., and Avoli, M. (2013). The kainic acid model of temporal lobe epilepsy. Neurosci. Biobehav. Rev. 37, 2887–2899. doi: 10.1016/j.neubiorev.2013.10.011
Lévesque, M., Bortel, A., Gotman, J., and Avoli, M. (2011). High-frequency (80–500 Hz) oscillations and epileptogenesis in temporal lobe epilepsy. Neurobiol. Dis. 42, 231–241. doi: 10.1016/j.nbd.2011.01.007
Lévesque, M., Chen, L.-Y., Etter, G., Shiri, Z., Wang, S., Williams, S., et al. (2019). Paradoxical effects of optogenetic stimulation in mesial temporal lobe epilepsy. Ann. Neurol. 86, 714–728. doi: 10.1002/ana.25572
Lévesque, M., Herrington, R., Hamidi, S., and Avoli, M. (2016). Interneurons spark seizure-like activity in the entorhinal cortex. Neurobiol. Dis. 87, 91–101. doi: 10.1016/j.nbd.2015.12.011
Lévesque, M., Macey-Dare, A. D. B., Wang, S., and Avoli, M. (2021b). Evolution of interictal spiking during the latent period in a mouse model of mesial temporal lobe epilepsy. Curr. Res. Neurobiol. 2:100008. doi: 10.1016/j.crneur.2021.100008
Lévesque, M., Biagini, G., de Curtis, M., Gnatkovsky, V., Pitsch, J., Wang, S., et al. (2021a). The pilocarpine model of mesial temporal lobe epilepsy: Over one decade later, with more rodent species and new investigative approaches. Neurosci. Biobehav. Rev. 130, 274–291. doi: 10.1016/j.neubiorev.2021.08.020
Lévesque, M., Salami, P., Gotman, J., and Avoli, M. (2012). Two seizure-onset types reveal specific patterns of high-frequency oscillations in a model of temporal lobe epilepsy. J. Neurosci. 32, 13264–13272. doi: 10.1523/JNEUROSCI.5086-11.2012
Librizzi, L., Losi, G., Marcon, I., Sessolo, M., Scalmani, P., Carmignoto, G., et al. (2017). Interneuronal network activity at the onset of seizure-like events in entorhinal cortex slices. J. Neurosci. 37, 10398–10407. doi: 10.1523/JNEUROSCI.3906-16.2017
Lloyd, K. G., Morselli, P. L., Depoortere, H., Fournier, V., Zivkovic, B., Scatton, B., et al. (1983). The potential use of GABA agonists in psychiatric disorders: Evidence from studies with progabide in animal models and clinical trials. Pharmacol. Biochem. Behav. 18, 957–966. doi: 10.1016/s0091-3057(83)80021-5
Loiseau, P., Bossi, L., Guyot, M., Orofiamma, B., and Morselli, P. L. (1983). Double-blind crossover trial of progabide versus placebo in severe epilepsies. Epilepsia 24, 703–715.
Louvel, J., Papatheodoropoulos, C., Siniscalchi, A., Kurcewicz, I., Pumain, R., Devaux, B., et al. (2001). GABA-mediated synchronization in the human neocortex: Elevations in extracellular potassium and presynaptic mechanisms. Neuroscience 105, 803–813. doi: 10.1016/s0306-4522(01)00247-0
Marshel, J. H., Kim, Y. S., Machado, T. A., Quirin, S., Benson, B., Kadmon, J., et al. (2019). Cortical layer-specific critical dynamics triggering perception. Science 365:eaaw5202. doi: 10.1126/science.aaw5202
McDonald, J. W., Garofalo, E. A., Hood, T., Sackellares, J. C., Gilman, S., McKeever, P. E., et al. (1991). Altered excitatory and inhibitory amino acid receptor binding in hippocampus of patients with temporal lobe epilepsy. Ann. Neurol. 29, 529–541. doi: 10.1002/ana.410290513
Mody, I., Lambert, J. D., and Heinemann, U. (1987). Low extracellular magnesium induces epileptiform activity and spreading depression in rat hippocampal slices. J. Neurophysiol. 57, 869–888. doi: 10.1152/jn.1987.57.3.869
Morris, M. E., Obrocea, G. V., and Avoli, M. (1996). Extracellular K+ accumulations and synchronous GABA-mediated potentials evoked by 4-aminopyridine in the adult rat hippocampus. Exp. Brain Res. 109, 71–82. doi: 10.1007/BF00228628
Niedermeyer, E., and da Silva, F. H. L. (2005). Electroencephalography: Basic principles, clinical applications, and related fields. Philadelphia, PA: Lippincott Williams & Wilkins.
Olsen, R. W. (2015). Allosteric ligands and their binding sites define γ-aminobutyric acid (GABA) type A receptor subtypes. Adv. Pharmacol. 73, 167–202. doi: 10.1016/bs.apha.2014.11.005
Olsen, R. W., Bureau, M., Houser, C. R., Delgado-Escueta, A. V., Richards, J. G., and Möhler, H. (1992). GABA/benzodiazepine receptors in human focal epilepsy. Epilepsy Res. Suppl. 8, 383–391.
Pang, T., and Hirsch, L. J. (2005). Treatment of convulsive and nonconvulsive status epilepticus. Curr. Treat. Options Neurol. 7, 247–259. doi: 10.1007/s11940-005-0035-x
Perreault, P., and Avoli, M. (1991). Physiology and pharmacology of epileptiform activity induced by 4-aminopyridine in rat hippocampal slices. J. Neurophysiol. 65, 771–785.
Perreault, P., and Avoli, M. (1992). 4-aminopyridine-induced epileptiform activity and a GABA-mediated long- lasting depolarization in the rat hippocampus. J. Neurosci. 12, 104–115. doi: 10.1523/JNEUROSCI.12-01-00104.1992
Perucca, E., Gram, L., Avanzini, G., and Dulac, O. (1998). Antiepileptic drugs as a cause of worsening seizures. Epilepsia 39, 5–17. doi: 10.1111/j.1528-1157.1998.tb01268.x
Perucca, P., Dubeau, F., and Gotman, J. (2014). Intracranial electroencephalographic seizure-onset patterns: Effect of underlying pathology. Brain J. Neurol. 137, 183–196. doi: 10.1093/brain/awt299
Pollack, M. H., Roy-Byrne, P. P., Van Ameringen, M., Snyder, H., Brown, C., Ondrasik, J., et al. (2005). The selective GABA reuptake inhibitor tiagabine for the treatment of generalized anxiety disorder: Results of a placebo-controlled study. J. Clin. Psychiatry 66, 1401–1408.
Psarropoulou, C., and Avoli, M. (1996). Developmental features of 4-aminopyridine induced epileptogenesis. Brain Res. Dev. Brain Res. 94, 52–59.
Rogawski, M. A., and Löscher, W. (2004). The neurobiology of antiepileptic drugs. Nat. Rev. Neurosci. 5, 553–564. doi: 10.1038/nrn1430
Rutecki, P. A., Lebeda, F. J., and Johnston, D. (1985). Epileptiform activity induced by changes in extracellular potassium in hippocampus. J. Neurophysiol. 54, 1363–1374.
Rutecki, P. A., Lebeda, F. J., and Johnston, D. (1987). 4-Aminopyridine produces epileptiform activity in hippocampus and enhances synaptic excitation and inhibition. J. Neurophysiol. 57, 1911–1924. doi: 10.1152/jn.1987.57.6.1911
Rutecki, P. A., Lebeda, F. J., and Johnston, D. (1990). Epileptiform activity in the hippocampus produced by tetraethylammonium. J. Neurophysiol. 64, 1077–1088.
Sabolek, H. R., Swiercz, W. B., Lillis, K. P., Cash, S. S., Huberfeld, G., Zhao, G., et al. (2012). A candidate mechanism underlying the variance of interictal spike propagation. J. Neurosci. 32, 3009–3021. doi: 10.1523/JNEUROSCI.5853-11.2012
Salami, P., Lévesque, M., Benini, R., Behr, C., Gotman, J., and Avoli, M. (2014). Dynamics of interictal spikes and high-frequency oscillations during epileptogenesis in temporal lobe epilepsy. Neurobiol. Dis. 67C, 97–106. doi: 10.1016/j.nbd.2014.03.012
Schevon, C. A., Weiss, S. A., McKhann, G., Goodman, R. R., Yuste, R., Emerson, R. G., et al. (2012). Evidence of an inhibitory restraint of seizure activity in humans. Nat. Commun. 3:1060. doi: 10.1038/ncomms2056
Schwartzkroin, P. A., and Prince, D. A. (1980). Changes in excitatory and inhibitory synaptic potentials leading to epileptogenic activity. Brain Res. 183, 61–76.
Schwarzer, C., Tsunashima, K., Wanzenböck, C., Fuchs, K., Sieghart, W., and Sperk, G. (1997). GABA(A) receptor subunits in the rat hippocampus II: Altered distribution in kainic acid-induced temporal lobe epilepsy. Neuroscience 80, 1001–1017. doi: 10.1016/s0306-4522(97)00145-0
Shiri, Z., Manseau, F., Lévesque, M., Williams, S., and Avoli, M. (2015). Interneuron activity leads to initiation of low-voltage fast-onset seizures. Ann. Neurol. 77, 541–546. doi: 10.1002/ana.24342
Shiri, Z., Manseau, F., Lévesque, M., Williams, S., and Avoli, M. (2016). Activation of specific neuronal networks leads to different seizure onset types. Ann. Neurol. 79, 354–365. doi: 10.1002/ana.24570
Sloviter, R. S. (1987). Decreased hippocampal inhibition and a selective loss of interneurons in experimental epilepsy. Science 235, 73–76. doi: 10.1126/science.2879352
Staba, R. J., Wilson, C. L., Bragin, A., Jhung, D., Fried, I., and Engel, J. Jr. (2004). High-frequency oscillations recorded in human medial temporal lobe during sleep. Ann. Neurol. 56, 108–115. doi: 10.1002/ana.20164
Steriade, M., Gloor, P., Llinás, R. R., Lopes de Silva, F. H., and Mesulam, M. M. (1990). Report of IFCN committee on basic mechanisms. Basic mechanisms of cerebral rhythmic activities. Electroencephalogr. Clin. Neurophysiol. 76, 481–508. doi: 10.1016/0013-4694(90)90001-z
Sudbury, J. R., and Avoli, M. (2007). Epileptiform synchronization in the rat insular and perirhinal cortices in vitro. Eur. J. Neurosci. 26, 3571–3582. doi: 10.1111/j.1460-9568.2007.05962.x
Tancredi, V., Hwa, G. G., Zona, C., Brancati, A., and Avoli, M. (1990). Low magnesium epileptogenesis in the rat hippocampal slice: Electrophysiological and pharmacological features. Brain Res. 511, 280–290. doi: 10.1016/0006-8993(90)90173-9
Timofeev, I., and Steriade, M. (2004). Neocortical seizures: Initiation, development and cessation. Neuroscience 123, 299–336. doi: 10.1016/j.neuroscience.2003.08.051
Toyoda, I., Fujita, S., Thamattoor, A. K., and Buckmaster, P. S. (2015). Unit activity of hippocampal interneurons before spontaneous seizures in an animal model of temporal lobe epilepsy. J. Neurosci. 35, 6600–6618. doi: 10.1523/JNEUROSCI.4786-14.2015
Truccolo, W., Donoghue, J. A., Hochberg, L. R., Eskandar, E. N., Madsen, J. R., Anderson, W. S., et al. (2011). Single-neuron dynamics in human focal epilepsy. Nat. Neurosci. 14, 635–641. doi: 10.1038/nn.2782
Tsunashima, K., Schwarzer, C., Kirchmair, E., Sieghart, W., and Sperk, G. (1997). GABA(A) receptor subunits in the rat hippocampus III: Altered messenger RNA expression in kainic acid-induced epilepsy. Neuroscience 80, 1019–1032. doi: 10.1016/s0306-4522(97)00144-9
Unal, G., Joshi, A., Viney, T. J., Kis, V., and Somogyi, P. (2015). Synaptic targets of medial septal projections in the hippocampus and extrahippocampal cortices of the mouse. J. Neurosci. 35, 15812–15826. doi: 10.1523/JNEUROSCI.2639-15.2015
Urrestarazu, E., Jirsch, J. D., LeVan, P., Hall, J., Avoli, M., Dubeau, F., et al. (2006). High-frequency intracerebral EEG activity (100-500 Hz) following interictal spikes. Epilepsia 47, 1465–1476. doi: 10.1111/j.1528-1167.2006.00618.x
Uusisaari, M., Smirnov, S., Voipio, J., and Kaila, K. (2002). Spontaneous epileptiform activity mediated by GABA(A) receptors and gap junctions in the rat hippocampal slice following long-term exposure to GABA(B) antagonists. Neuropharmacology 43, 563–572. doi: 10.1016/s0028-3908(02)00156-9
Uva, L., Avoli, M., and de Curtis, M. (2009). Synchronous GABA-receptor-dependent potentials in limbic areas of the in-vitro isolated adult guinea pig brain. Eur. J. Neurosci. 29, 911–920. doi: 10.1111/j.1460-9568.2009.06672.x
Uva, L., Breschi, G. L., Gnatkovsky, V., Taverna, S., and de Curtis, M. (2015). Synchronous inhibitory potentials precede seizure-like events in acute models of focal limbic seizures. J. Neurosci. 35, 3048–3055. doi: 10.1523/JNEUROSCI.3692-14.2015
Uva, L., Trombin, F., Carriero, G., Avoli, M., and de Curtis, M. (2013). Seizure-like discharges induced by 4-aminopyridine in the olfactory system of the in vitro isolated guinea pig brain. Epilepsia 54, 605–615. doi: 10.1111/epi.12133
Velazquez, J. L., and Carlen, P. L. (1999). Synchronization of GABAergic interneuronal networks during seizure-like activity in the rat horizontal hippocampal slice. Eur. J. Neurosci. 11, 4110–4118. doi: 10.1046/j.1460-9568.1999.00837.x
Viitanen, T., Ruusuvuori, E., Kaila, K., and Voipio, J. (2010). The K+–Cl– cotransporter KCC2 promotes GABAergic excitation in the mature rat hippocampus. J. Physiol. 588, 1527–1540. doi: 10.1113/jphysiol.2009.181826
Voskuyl, R. A., and Albus, H. (1985). Spontaneous epileptiform discharges in hippocampal slices induced by 4-aminopyridine. Brain Res. 342, 54–66.
Watson, T. C., Obiang, P., Torres-Herraez, A., Watilliaux, A., Coulon, P., Rochefort, C., et al. (2018). Anatomical and physiological foundations of cerebello-hippocampal interaction. eLife 8:e41896. doi: 10.7554/eLife.41896
Williamson, A., Telfeian, A. E., and Spencer, D. D. (1995). Prolonged GABA responses in dentate granule cells in slices isolated from patients with temporal lobe sclerosis. J. Neurophysiol. 74, 378–387. doi: 10.1152/jn.1995.74.1.378
Yekhlef, L., Breschi, G. L., Lagostena, L., Russo, G., and Taverna, S. (2015). Selective activation of parvalbumin- or somatostatin-expressing interneurons triggers epileptic seizurelike activity in mouse medial entorhinal cortex. J. Neurophysiol. 113, 1616–1630. doi: 10.1152/jn.00841.2014
Zeidler, Z., Hoffmann, K., and Krook-Magnuson, E. (2020). HippoBellum: Acute cerebellar modulation alters hippocampal dynamics and function. J. Neurosci. 40, 6910–6926. doi: 10.1523/JNEUROSCI.0763-20.2020
Ziburkus, J., Cressman, J. R., Barreto, E., and Schiff, S. J. (2006). Interneuron and pyramidal cell interplay during in vitro seizure-like events. J. Neurophysiol. 95, 3948–3954. doi: 10.1152/jn.01378.2005
Zijlmans, M., Jacobs, J., Kahn, Y. U., Zelmann, R., Dubeau, F., and Gotman, J. (2011). Ictal and interictal high frequency oscillations in patients with focal epilepsy. Clin. Neurophysiol. 122, 664–671. doi: 10.1016/j.clinph.2010.09.021
Keywords: epileptiform synchronization, excitatory transmission, GABAA receptor, inhibitory transmission, interictal spikes, mesial temporal lobe epilepsy, seizures
Citation: Avoli M, de Curtis M, Lévesque M, Librizzi L, Uva L and Wang S (2022) GABAA signaling, focal epileptiform synchronization and epileptogenesis. Front. Neural Circuits 16:984802. doi: 10.3389/fncir.2022.984802
Received: 02 July 2022; Accepted: 13 September 2022;
Published: 05 October 2022.
Edited by:
Lisa Topolnik, Laval University, CanadaReviewed by:
Sabato Santaniello, University of Connecticut, United StatesCopyright © 2022 Avoli, de Curtis, Lévesque, Librizzi, Uva and Wang. This is an open-access article distributed under the terms of the Creative Commons Attribution License (CC BY). The use, distribution or reproduction in other forums is permitted, provided the original author(s) and the copyright owner(s) are credited and that the original publication in this journal is cited, in accordance with accepted academic practice. No use, distribution or reproduction is permitted which does not comply with these terms.
*Correspondence: Massimo Avoli, bWFzc2ltby5hdm9saUBtY2dpbGwuY2E=
Disclaimer: All claims expressed in this article are solely those of the authors and do not necessarily represent those of their affiliated organizations, or those of the publisher, the editors and the reviewers. Any product that may be evaluated in this article or claim that may be made by its manufacturer is not guaranteed or endorsed by the publisher.
Research integrity at Frontiers
Learn more about the work of our research integrity team to safeguard the quality of each article we publish.