- Centre for Interdisciplinary Research in Biology (CIRB), Collège de France, CNRS, INSERM, Labex MemoLife, PSL Research University, Paris, France
From birth to adolescence, the brain adapts to its environmental stimuli through structural and functional remodeling of neural circuits during critical periods of heightened plasticity. They occur across modalities for proper sensory, motor, linguistic, and cognitive development. If they are disrupted by early-life adverse experiences or genetic deficiencies, lasting consequences include behavioral changes, physiological and cognitive deficits, or psychiatric illness. Critical period timing is orchestrated not only by appropriate neural activity but also by a multitude of signals that participate in the maturation of fast-spiking parvalbumin interneurons and the consolidation of neural circuits. In this review, we describe the various signaling factors that initiate critical period onset, such as BDNF, SPARCL1, or OTX2, which originate either from local neurons or glial cells or from extracortical sources such as the choroid plexus. Critical period closure is established by signals that modulate extracellular matrix and myelination, while timing and plasticity can also be influenced by circadian rhythms and by hormones and corticosteroids that affect brain oxidative stress levels or immune response. Molecular outcomes include lasting epigenetic changes which themselves can be considered signals that shape downstream cross-modal critical periods. Comprehensive knowledge of how these signals and signaling factors interplay to influence neural mechanisms will help provide an inclusive perspective on the effects of early adversity and developmental defects that permanently change perception and behavior.
Introduction
Critical periods (CPs) of heightened plasticity shape neural circuits according to experience during postnatal brain development (Reh et al., 2020). These distinct plasticity windows occur not only across primary sensory areas, such as the primary visual and auditory cortices but also in multimodal areas, such as the insular cortex and the medial prefrontal cortex (mPFC; Testa-Silva et al., 2012; Gogolla et al., 2014). Separate CPs can occur at the same time in different brain areas, but complex functions may depend on the closure of an upstream “primary” CP and thus require sequential CPs (Nakamura et al., 2020). CP timing is driven by the maturation of fast-spiking (FS) inhibitory interneurons that express parvalbumin (PV). While PV cells are located throughout the brain, FS-PV cells residing in supragranular cortical layers drive CP onset and circuit rewiring (Fagiolini et al., 2004). They receive excitatory input from local pyramidal cells as well as long-range inputs from the thalamus and hippocampus (Faini et al., 2018; Yang et al., 2021), and they receive inhibitory inputs from themselves (autapses), from other FS-PV cells, and from SST, VIP, and CCK interneurons (Méndez and Bacci, 2011). They also connect with dopaminergic, serotonergic, and cholinergic fibers (Sun et al., 2019). The physiological maturation of FS-PV cells results in strong inhibitory output on the soma or the axon initial segment of nearby pyramidal cells, which provides control of excitatory currents, alters the excitatory–inhibitory (E/I) balance, permits large-scale changes in neural circuitry, and influences rhythmic oscillations (Sohal et al., 2009; Hu et al., 2014).
As FS-PV cells mature, they become enwrapped by perineuronal nets (PNNs) composed of glycans, proteoglycans, and proteins originating from either FS-PV cells or surrounding cells. PNNs are a condensed extracellular matrix (ECM) providing specific electrical properties and a specialized micro-environment that stabilizes synapses and attracts non-cell-autonomous factors (for reviews, see Testa et al., 2019; Carulli and Verhaagen, 2021). Precise PNN accumulation, in terms of both composition and timing, is implicated in CP onset but plays a major role as a molecular brake limiting structural plasticity for CP closure (for review, see Fawcett et al., 2019). Removal of PNNs can allow for functional plasticity in adult rodents (Pizzorusso et al., 2002; Beurdeley et al., 2012).
Non-cell-autonomous factors (summarized in Figure 1) also shape the connectivity and the electrical properties of FS-PV cells during CPs and determine the extent to which the environment—local, systemic, and external—can affect cortical circuit dynamics throughout life. A precise and detailed understanding of such factors will help identify potential modulators of plasticity in both childhood and adulthood in order to reverse or repair brain disorders and trauma.
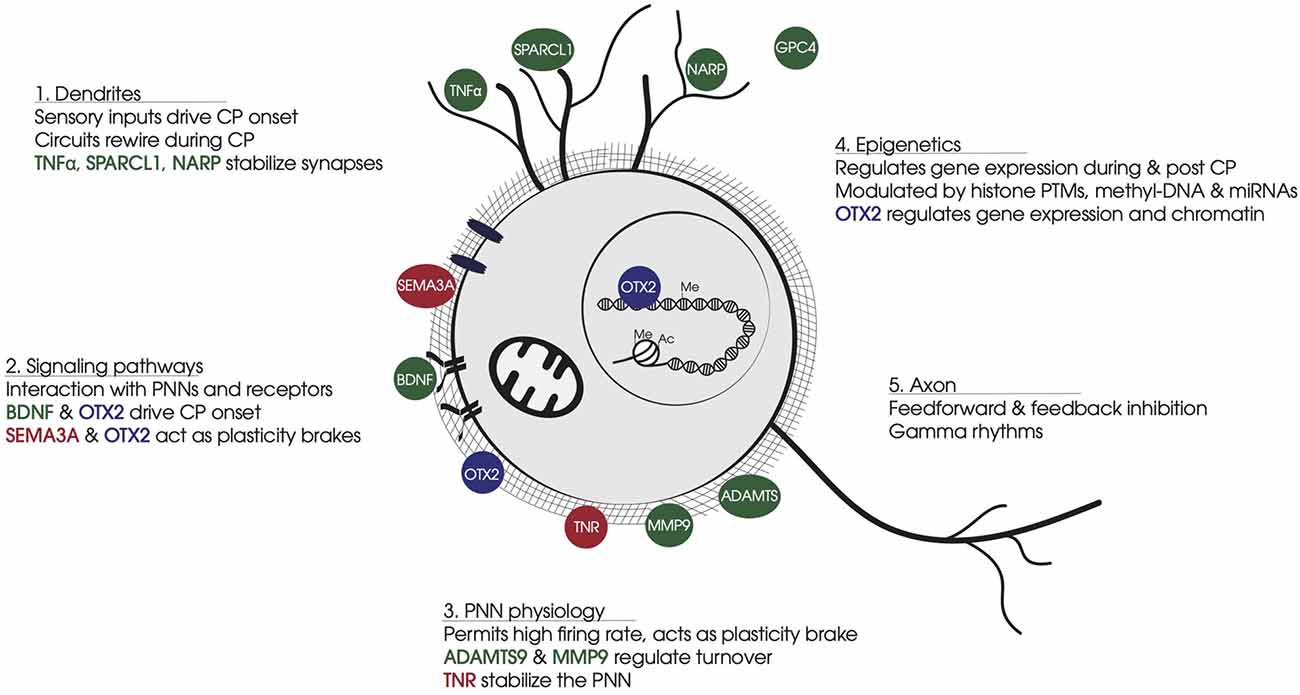
Figure 1. Summary of non-cell-autonomous factors involved in FS-PV cell physiology. (1) Dendrites: Non-cell-autonomous factors stabilize synapse number and strength of sensory inputs that drive CP onset and FS-PV cell synapse maturation. (2) Signaling pathways: Non-cell-autonomous factors bind specific receptors or glycosaminoglycan motifs in PNNs. BDNF-TRKB triggers activity-dependent pathways. OTX2 binding to CSPGs leads to cell internalization and translocation to the nucleus. SEMA3A interacts with CSPGs and stabilizes synapses on FS-PV cell soma. (3) PNN physiology: PNNs develop around FS-PV cells during CP, providing neuroprotection against oxidative damage induced by the fast-spiking activity of FS-PV cells. PNNs stabilize neural networks and limit synapses formation. Secreted ECM enzymes provide turnover for PNN dynamics, while other non-cell-autonomous factors participate in PNN stabilization. (4) Epigenetics: During the CP, the FS-PV cell transcriptome is regulated in response to the cellular environment via histone PTMs and DNA methylation. OTX2 affects gene expression and chromatin conformation in part through transcription regulation Gadd45b/g. (5) Axon: FS-PV cell outputs on principal cells generate gamma rhythms. Non-cell-autonomous factors participate in axon myelination and the stabilization of pre-synaptic receptors. Color code: In green, non-cell-autonomous factors contributing to CP onset; in red, non-cell-autonomous factors contributing to CP closure; in blue, non-cell-autonomous factors contributing in both opening and closure of CP. Abbreviations: CP, critical period; PNN, perineuronal net; FS-PV, fast-spiking-parvalbumin; CSPG, chondroitin sulfate proteoglycans; PTM, post-translational modification.
Non-Cell-Autonomous Factors from Local Cells for Short-Range Interactions
Cellular Sources
Short-range non-cell-autonomous proteins that affect FS-PV cell function come from surrounding neurons, astrocytes, microglia, and oligodendrocytes. While these cells can interact and exchange factors within the synaptic compartment to guide synapse formation and function, they also secrete factors that signal through the ECM (Table 1). All of these cell types secrete neurotrophic factors, proteases, and PNN components, while astrocytes and microglia also release proteins that shape synaptic function. Astrocytes participate in synapse formation, connectivity, transmission, and plasticity by regulating the extracellular ionic environment and recycling neurotransmitters (for review, see Santello et al., 2019), and by also secreting proteoglycans (for review, see Wiese et al., 2012). Microglia respond to changes in neural activity and help shape circuitry through various mechanisms including phagocytosis or through the secretion of active peptides and enzymes (for review, see Salter and Beggs, 2014). Both astrocytes and microglia have recently been implicated as active participants in CP plasticity (Sipe et al., 2016; Kalish et al., 2020; Ackerman et al., 2021; Ribot et al., 2021). Beyond secreting some of the above factors, oligodendrocytes provide myelination, which initially coincides with CP opening in the sensory cortices but ultimately participates as an important molecular brake for CP closure (for review, see Fletcher et al., 2021). In order to focus on more widely dispersed factors, we chose to not elaborate further on neurotransmitters, synaptic pruning, adhesion molecules, and myelination.
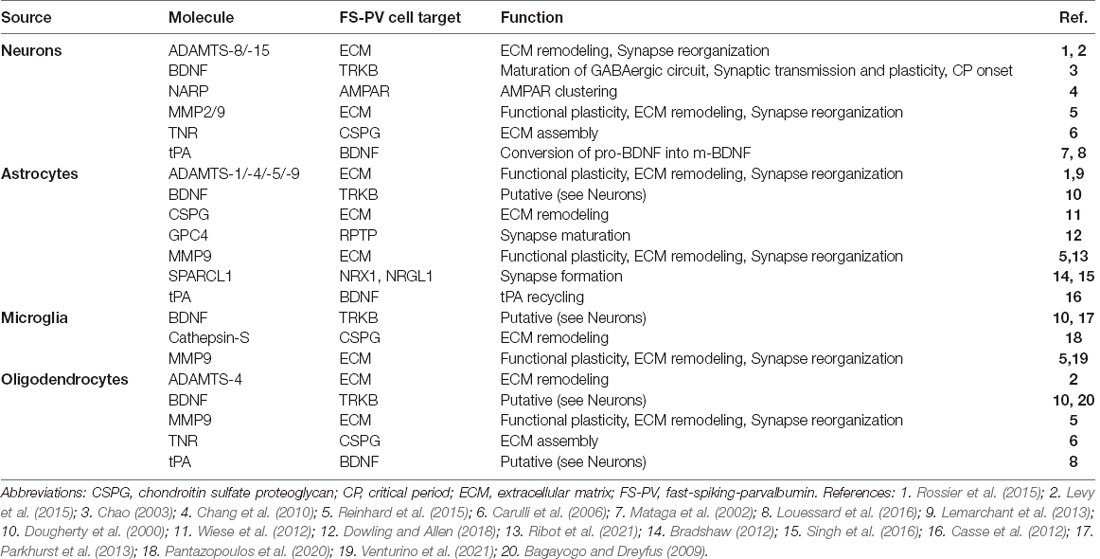
Table 1. Sources, targets, and functions of non-cell-autonomous molecules affecting FS-PV cell activity.
Growth Factors
One of the first non-cell-autonomous molecules discovered to be involved in CPs was brain-derived neurotrophic factor (BDNF), which signals through interaction with its canonical receptor TRKB on FS-PV cells. BDNF is typically secreted as pro-BDNF which is generally cleaved by plasmin to release BDNF (Lu et al., 2005) that then activates TRKB phosphorylation to initiate intracellular cascades of early/immediate-genes leading ultimately to FS-PV cell maturation for CP plasticity onset (Huang et al., 1999). Loss of function studies of TRKB receptors in FS-PV cells demonstrate global changes in network connectivity, as well as behavioral, autistic-like phenotypes (Xenos et al., 2018), probably caused by brain-wide dysregulation of CPs. The sensitivity of FS-PV cells to BDNF may be dampened by PNNs, which activate protein tyrosine phosphatase sigma (PTPσ) receptors (Lesnikova et al., 2021). When bound to chondroitin sulfate proteoglycan (CSPG), PTPσ promotes TRKB dephosphorylation to abolish signaling and limit BDNF-induced plasticity in FS-PV cells. Thus FS-PV cells are likely less responsive to BDNF after CP closure. Although astrocytes, microglia, and oligodendrocytes also secrete pro-BDNF (Dougherty et al., 2000), these cell types have not been formally shown to affect FS-PV TRKB signaling in cortical CPs. Nevertheless, it remains highly likely that they participate. For example, secretion of BDNF from microglia is critical for learning-dependent synaptic plasticity in the motor cortex (Parkhurst et al., 2013), and affects inhibitory synaptic transmission via TrkB signaling in the spinal cord (Coull et al., 2005) and hippocampus (Zheng et al., 2011). Also, oligodendrocytes actively participate in synaptic transmission and plasticity through BDNF signaling in the developing brain (Jang et al., 2019).
Neuregulin-1 (NRG1), a trophic factor containing an epidermal growth factor (EGF) domain, is expressed in astrocytes and in both inhibitory and excitatory cortical neurons, and exists in multiple isoforms including the soluble types I and II (Liu et al., 2011). NRG1 signals through the ErbB tyrosine receptor kinase family and specific NRG1/ErbB4 signaling in FS-PV cells regulate connectivity (Fazzari et al., 2010) and CP plasticity (Gu et al., 2016; Sun et al., 2016). While ErbB4 is expressed by FS-PV cells, it remains unclear whether NRG1 signaling is autocrine or paracrine, given that both FS-PV cells and excitatory neurons can release NRG1 into the extracellular space (Grieco et al., 2020). Interestingly, NRG1 signaling also involves oligodendrocytes that express ErbB3. In the mouse mPFC, NRG1/ErbB3 signaling is essential during a post-weaning CP for social learning which affects oligodendrocyte maturation and lifelong myelination (Makinodan et al., 2012). The NRG1/ErbB4 signal also involves VIP interneurons in the mPFC, and NRG1 is gaining attention as a therapeutic target for psychiatric disorders (Shi and Bergson, 2020).
Perineuronal Nets
Built with diverse components originating from neurons, astrocytes, and oligodendrocytes, PNNs are lattice-like structures primarily composed of hyaluronan (HA), CSPGs, and cross-linking proteins from the link protein family (HAPLNs) and tenascin-R (TNR; for reviews, see Fawcett et al., 2019; Testa et al., 2019). PNN assembly is activity-dependent (Dityatev et al., 2007), requiring sensory input during postnatal development in order to form (Pizzorusso et al., 2002; McRae et al., 2007; Kind et al., 2013), and their maintenance in adulthood can be regulated by local network activity (Devienne et al., 2021). Physiologically, PNNs act as molecular brakes that progressively decrease plasticity as they condense around FS-PV cell soma and proximal dendrites during the CP, and eventually restrict plasticity in adulthood (Pizzorusso et al., 2002). Through enzymatic removal of PNNs in vivo, it has been shown that PNNs can affect FS-PV cell excitability and spontaneous activity (Lensjø et al., 2017; Hayani et al., 2018; Carceller et al., 2020), and selectively dampen thalamic excitation (Faini et al., 2018).
PNNs protect neurons from oxidative stress and toxic proteins (Miyata et al., 2007; Cabungcal et al., 2013; Suttkus et al., 2014), and their polyanionic structure limits the toxicity inherent to the high activity of FS-PV cells by buffering the cations involved in neurotransmission (Brückner et al., 1993; Härtig et al., 1999). They also interact with signaling molecules, such as SEMA3A and OTX2 (described below), and potentially affect the dynamics of membrane-bound proteins. While OTX2 has been shown to regulate the expression of CSPGs within FS-PV cells (Hou et al., 2017; Lee et al., 2017), a complete picture of PNN molecule expression regulation has been difficult to obtain given that their components come from multiple sources. While FS-PV cells express all of the required molecules, including HA and HAPLNs, surrounding glial cells and other neurons can also contribute CSPGs and TNR (Testa et al., 2019). Thus, the assembly of PNNs around FS-PV cells has the potential to be greatly influenced by changes in the expression of neighboring cells. The picture is further complicated by the regulation of PNN structure by multiple metalloproteases (described below) that again come from multiple sources. In fact, although PNNs are often thought of as fixed structures, they remain dynamic in adulthood in response to changes in FS-PV activity and diurnal fluctuations (Pantazopoulos et al., 2020; Devienne et al., 2021; Harkness et al., 2021).
Proteases
Tissue-type plasminogen activator protein (tPA), originally identified as an anti-clotting agent in the blood, is a major serine protease in the brain that plays several roles in brain plasticity (for review, see Hensch, 2005) and is expressed by neurons and oligodendrocytes (Louessard et al., 2016). Proteolysis by tPA permits experience-dependent spine motility by degrading ECM and cell-adhesion proteins. It converts plasminogen to plasmin, which in turn not only degrades ECM but also activates metalloproteases, chemokines, and neurotrophic factors, such as BDNF. tPA is released by both axons and dendrites through exosomal vesicles, with axonal release being activity-dependent (for review, see Lenoir et al., 2019). Astrocytes also regulate tPA levels, possibly by expressing tPA, but also by recycling the tPA secreted by neurons in the synaptic cleft so that it can then bind to various receptors and act as a neuromodulator (Casse et al., 2012). Permissive amounts of tPA may therefore integrate functional and structural changes downstream of the E/I balance for CP plasticity.
Matrix metalloproteinases (MMPs) are a family of zinc-binding endopeptidases that selectively degrade proteoglycans, growth factors, cytokines, chemokines, myelin-associated proteins, and cell adhesion molecules in the ECM and PNNs (for review, see Huntley, 2012). MMPs can be activated by tPA and, in turn, be removed by tissue inhibitors of metalloproteinases (TIMPs). While several MMPs are found in the brain, MMP9 is the only member known to be secreted by astrocytes, oligodendrocytes, microglia, and neurons (for review, see Reinhard et al., 2015). MMP9 regulates both functional plasticity and ECM remodeling by shaping the pericellular and synaptic environment and by activating signaling molecules. CP closure is accompanied by changes in astrocytic networks that dampen their secretion of MMP9 and favor PNN formation (Ribot et al., 2021). Conversely, MMP9 secreted by microglia can remodel PNNs in adult plasticity paradigms (Venturino et al., 2021). MMP9 knock-out mice show attenuated plasticity during CPs and show subtle alterations in microglia morphology suggesting microglia function is changed (Kelly et al., 2015). In keeping with the possibility that MMPs can also liberate molecules that impede neurite outgrowth, recent evidence shows that inhibition of MMP2 and MMP9 can either limit or promote adult visual cortex plasticity depending on the nature of the insult (Akol et al., 2022).
Other proteases include cathepsin-S and enzymes from the ADAMTS (A Disintegrin and Metalloproteinase with Thrombospondin motifs) family. Cathepsin-S, which is expressed and secreted by microglia throughout the adult brain, is a member of the lysosomal cysteine protease family that can degrade proteoglycans, and was recently shown to digest PNNs in a circadian manner (see below; Pantazopoulos et al., 2020). Similar to MMPs, ADAMTSs are also zinc-binding endopeptidases, but select members expressed in the brain show substrate specificity for aggrecan and other CSPGs within the ECM and the PNNs of FS-PV cells (Kelwick et al., 2015). While they are mainly expressed by astrocytes (Lemarchant et al., 2013), ADAMTS-8 and -15 were found to be expressed in somatosensory FS-PV cells (Rossier et al., 2015), suggesting more widespread expression patterns are possible. Although ADAMTS8 and ADAMTS9 have been shown to be upregulated during CPs (Lee et al., 2017; Apulei et al., 2019), how this family of proteases is regulated remains unknown.
Glycoproteins
Several secreted glycoproteins, including glypicans (GPC), pentraxin 1 (NPTX1), and neuronal activity-regulated pentraxin (NARP or NPTX2), fall within related pathways that may affect FS-PV cell function. Glypicans are a family of heparan sulfate proteoglycans that are localized to the neuronal membrane via glycosylphosphatidylinositol (GPI) anchor and can be released from the cell surface upon cleavage (Filmus et al., 2008). Although not formally implicated in CP regulation, GPC4 is an astrocyte-secreted protein expressed throughout early postnatal development (Dowling and Allen, 2018), and has NARP as a downstream target that regulates FS-PV cells (see below). GPC4 binds to presynaptic type 2a receptor protein tyrosine phosphatases (RPTPs) inducing the release of NPTX1 (Farhy-Tselnicker et al., 2017). NPTX1 makes a heterocomplex with NARP, which binds to AMPA receptors and triggers their clustering in post-synaptic neurons. NARP is an immediate early gene that regulates synaptic strength and is enriched selectively at excitatory synapses impinging on FS-PV cells (Chang et al., 2010). In Narp−/− mice, inhibition from FS-PV cells is impaired at the onset of ocular dominance CP plasticity (Gu et al., 2013). Interestingly, enzymatic treatment to remove PNNs also removes NARP from the surface of neuronal dendrites (Gu et al., 2013). Conversely, NARP enhances PNN formation (Van’t Spijker et al., 2019), suggesting that there is a feedback interaction between NARP and PNNs. Given that GPCs are expressed in the choroid plexus and secreted into the cerebrospinal fluid (CSF; Lugert et al., 2017; Dani et al., 2021), their involvement in CP regulation may have been missed due to long-range signaling (see below).
In the visual cortex, the synapse-regulating protein SPARCL1 (Hevin) is a glycoprotein secreted by astrocytes and implicated in experience-dependent plasticity. Once secreted, it can participate in the formation of synapses by linking presynaptic Neurexin-1 (NRX1) to postsynaptic Neuroligin 1 (NLGN1), which together regulate synaptic signal transmission (Gan and Südhof, 2020). In the visual cortex, SPARCL1 has been shown to bridge thalamocortical afferents and be required for ocular dominance CP plasticity (Singh et al., 2016; Ribic et al., 2019). SPARCL1 also has a synaptogenic activity that can be inhibited by ADAMTS4 and MMPs (1, 3, and 9), which cleave SPARCL1 to generate a SPARC-like fragment (SLF) that in turn competes with SPARCL1 (Bradshaw, 2012).
Plasticity states and interneuron maturation are also influenced by levels of long polysialic acid (PSA) chains attached to neural cell adhesion molecules (NCAM). PSA-NCAM is expressed by both glial cells and neurons and provides a highly hydrated polymer that lubricates extracellular space and minimizes surface interactions between cells, and the NCAM extracellular region (NCAM-EC) can be released as a soluble fragment (for review, see Rutishauser, 2008). In the juvenile visual cortex, the expression of PSA-NCAM undergoes a dramatic activity-dependent decline that is permissive for the maturation of FS-PV cells (Di Cristo et al., 2007). In the rodent mPFC, PSA-NCAM expression is restricted to interneurons, at least in the adult, and plays a dopamine-dependent permissive role for inhibitory circuit structural plasticity (Castillo-Gómez et al., 2011). Conversely, non-PSA NCAM participates in the removal of interneuron synapses, but this process can be inhibited through NCAM interaction with CSPGs, further highlighting the role of PNNs for synaptic stability (Sullivan et al., 2018). Finally, NCAM-EC has been shown to restrict neurite branching and outgrowth, and its overexpression stunts FS-PV cell maturation in the mPFC (Brennaman and Maness, 2008). Thus, while NCAM is not strictly a non-cell-autonomous factor, its various forms and fragments can impact the ECM and FS-PV cell plasticity, thereby influencing juvenile CPs and adult functions. Indeed, its misexpression is linked with chronic stress and psychiatric disorders (Bueno-Fernandez et al., 2021).
Systemic and Non-Cell-Autonomous Factors for Long-Range Interactions
Cellular and Systemic Sources
Brain plasticity can be guided by signals coming from the periphery. Certain molecules in the cortical vasculature can pass the blood-brain barrier (BBB), either through transmembrane diffusion, active transport, or transcytosis (for reviews, see Abbott et al., 2010; Sweeney et al., 2019). Some diffuse through the extracellular space while others are mediated by astrocytic endfeet and local microglia. The choroid plexus is also highly vascularized and provides the blood-cerebrospinal fluid barrier (BCSFB) that is permissively different than the BBB and controls leukocyte entry into the CSF (for reviews, see Redzic, 2011; Ghersi-Egea et al., 2018; Cui et al., 2021). These structures are also responsive to corticosteroids and hormones. Furthermore, the choroid plexus secretes CSF which provides factors that regulate neural function and participates in the clearance of waste metabolites from the extracellular space (for reviews, see Praetorius and Damkier, 2017; Fame and Lehtinen, 2020). Diurnal rhythms also affect choroid plexus function, resulting in altered CSF composition, parenchyma clearance, and brain homeostasis (Myung et al., 2018).
Circadian Rhythms
Repeated daily patterns in gene expression, physiology, and behavior are driven by self-sustained biological clocks of approximately 24 h (circadian). A feedback loop between the transcription factors CLOCK and BMAL1 drives not only the central diurnal oscillator within the hypothalamic suprachiasmatic nucleus but is also observed in nearly all mammalian tissues (for review, see Lowrey and Takahashi, 2011). The choroid plexus also provides a strong circadian clock component that imparts diurnal changes in CSF composition and production, which affects not only the timing of metabolite clearance in the brain but also the distribution of clock signals to other brain regions (Myung et al., 2018). By using Clock knock-out mouse models, it was revealed that circadian-dependent expression of Per1 and Dbp in the primary visual cortex is mediated by the CLOCK:BMAL1 oscillator (Kobayashi et al., 2015). These mice have delayed CP timing, and it was further shown that Clock and Bmal1 expression in FS-PV cells participates in their maturation (Kobayashi et al., 2015). Mice mutants of Clock have altered nursing behavior that impacts cross-fostered wild-type pups. At postnatal day 14, these pups have reduced levels of brain serotonin, whose homeostasis during the early postnatal period is critical for normal emotional behavior in adulthood (Koizumi et al., 2013). Indeed, these animals have increased adult anxiety-related behavior. Furthermore, the photoperiod received during pre- and postnatal periods imprints the intrinsic electrical properties of serotonergic neurons of the dorsal raphe that can impact depression- and anxiety-related behavior later in life even after several subsequent photoperiod shifting (Green et al., 2015). Conversely, early-life adversity can change the hormonal milieu to increase the circulating levels of both glucocorticoids and pro-inflammatory cytokines that in turn deregulate circadian rhythms, leading to lasting epigenetic, physiological, and behavioral changes (Masri and Sassone-Corsi, 2010; Marco et al., 2016). Recent rodent and human studies have also revealed that PV expression and PNN accumulation have diurnal fluctuations in the adult (Pantazopoulos et al., 2020; Harkness et al., 2021), suggesting that circadian rhythms play a role in FS-PV function throughout life.
Hormones
CPs can occur in parallel to developmental changes in hormone levels, along the adrenal, thyroid, and gonadal axes, which have the potential to calibrate neural circuits. Adverse experiences during early life can elicit stress responses from the hypothalamic-pituitary-adrenal (HPA) axis, which results in the release of corticosteroids from the adrenal glands. Exposure during CPs may result in maladaptation of the HPA-axis that will affect basal and stress-induced activity in adulthood (Van Bodegom et al., 2017). The effects of early-life stress are also manifested by changes in FS-PV cell development and maturation (Chen et al., 2018; Page et al., 2019; Nawreen et al., 2020; Vasistha et al., 2020), typically within the mPFC (Bueno-Fernandez et al., 2021), which has a negative feedback function on the HPA-axis (Van Bodegom et al., 2017). Implicated in behavioral and psychiatric disorders, the maturation of mPFC connectivity occurs in multiple CP windows from childhood through to adolescence enabling complex brain functions such as memory, cognition, decision making, social behaviors, and mood (for review, see Klune et al., 2021). Consequently, mPFC development is also impacted by gonadal hormones during adolescence, with ovarian hormones having been shown to drive both mouse pubescence and mPFC inhibitory activity independently of age (Piekarski et al., 2017). Progesterone is also required in early neonatal life for proper innervation of the mPFC that affects adult mouse behavioral impulses and cognitive flexibility (Willing and Wagner, 2016). In song birds, both estrogen and testosterone have been shown to impact inhibitory neuron development affecting either language acquisition and processing or song crystallization (Vahaba and Remage-Healey, 2018; Cornez et al., 2020). Along the hypothalamic-pituitary-thyroid (HPT) axis, it has been hypothesized that thyroid hormone levels could affect cholinergic activity that shapes FS-PV cell maturation (Batista and Hensch, 2019). Indeed, reduction of thyroid levels has been shown to reduce cortical PV expression in an age-dependent manner, suggesting there is a CP of thyroid hormone action (Uchida et al., 2021). Together or separately, these hormonal signals clearly have the potential to influence FS-PV cell maturation during multiple CP windows thereby affecting the etiology of behavioral and psychiatric disorders such as autism spectrum disorder, depression, and schizophrenia.
Guidance Molecules and Morphogens
Implicated in axon guidance during development, semaphorins are chemorepulsive proteins that can be secreted and signal by binding to plexin receptors. In the postnatal rodent cortex, SEMA3A accumulates around FS-PV cells, owing to interaction with CSPGs, and colocalizes with various PNN components such as CSPGs and TNR (Dick et al., 2013; Vo et al., 2013; Nadanaka et al., 2020). It forms a complex with Plexin-A1 or Plexin-A4 receptors that is stabilized by Neuropilin-1 (NRP1; Lu et al., 2021). While Sema3A mRNA is found in neurons, there is no correlation between neuronal expression and PNN accumulation; instead, the choroid plexus has been proposed as a potential source via SEMA3A secretion into the CSF (de Winter et al., 2016). Regardless, SEMA3A accumulates in rodent visual cortex PNNs in an experience-dependent manner, and this accumulation participates in CP closure (Boggio et al., 2019). In the adult rat, blocking SEMA3A-NRP1 interaction can promote ocular dominance plasticity, showing that it is a required PNN-dependent component for maintaining low-plasticity (Boggio et al., 2019).
The OTX2 homeoprotein is expressed in the choroid plexus, secreted into the CSF, and accumulates within FS-PV cells owing to interactions with CSPGs in PNNs (Beurdeley et al., 2012; Spatazza et al., 2013). This signaling induces CP onset and mediates CP closure in primary visual and auditory cortices and in the mPFC (for review, see Di Nardo et al., 2020). Blocking OTX2-PNN interaction or sequestering OTX2 in the CSF in adult mice can promote ocular dominance plasticity (Beurdeley et al., 2012; Bernard et al., 2016), while overexpression of OTX2 in the choroid plexus can rescue plasticity-dependent anxiety-like behavior deficits regulated in the mPFC (Vincent et al., 2021). Upon accumulation within FS-PV cell PNNs, OTX2 can gain direct access to the cytoplasm and nucleus where it has been shown to regulate the translation of CSPGs and the transcription of proteins regulating oxidative stress response and DNA methylation (Hou et al., 2017; Sakai et al., 2017; Apulei et al., 2019). Thus, OTX2 participates in PNN growth, in cell metabolism, and in the regulation of FS-PV epigenetic states that directly impact FS-PV cell function. The accumulation of OTX2 in mPFC FS-PV cells has also been found to occur with diurnal fluctuations (Harkness et al., 2021), suggesting OTX2 may serve a role in coordinating diurnal changes in gene expression needed for optimal PV cell function during sleep and wakefulness.
Insight on Epigenetic Mechanisms Within FS-PV Cells
One of the final downstream outcomes of some non-cell-autonomous factors for FS-PV cell maturation may be to change chromatin states. DNA methylation and hydroxy-methylation are fast and precise systems of expression regulation, mainly repression (Greenberg and Bourc’his, 2019). In the brain, methylation occurs in both CpG islands and non-CpG contexts and may have diverse roles in CP regulation. DNA methyltransferase (DNMT) activity is required for experience-dependent methylation of key plasticity genes, and mediates ocular dominance shift after monocular deprivation during CP (Tognini et al., 2015). DNA methylation also acts as a marker for DNA binding proteins such as Methyl-CpG-binding protein 2 (MeCP2), a key methyl-DNA binding protein with a causal role in Rett syndrome. MeCP2 is also necessary for correct CP timing, as heterozygous mice show premature CP timing (Krishnan et al., 2015; Patrizi et al., 2020). This phenotype suggests accelerated FS-PV cell maturation, hypothesized to underly psychiatric disorders (Morishita et al., 2015). Furthermore, MeCP2 knock-out mice have increased basal and learning-induced PV expression, which is associated with CP dysfunction and anxiety (Morello et al., 2018). Histological analysis of MeCP2 distribution in FS-PV cells shows broad changes between plastic and non-plastic states, suggestive of global changes in chromatin structure. This distribution is influenced by the expression of GADD45b/g, which regulate the methylation of immediate-early genes implicated in plasticity (Apulei et al., 2019).
Histone post-translational modifications (hPTMs) impact chromatin structure and accessibility, which ultimately influence transcription regulation. The histone code is finely regulated and particularly difficult to study as it can include more than 100 different hPTMs that are often gene- and cell-specific (Millán-Zambrano et al., 2022). However, some experience-dependent marks have been linked to CP regulation, such as phosphorylated H3 and H3/H4 acetylation (Putignano et al., 2007). Functionally, broad pharmacological modulations of the enzymes responsible for acetylated hPTM maintenance such as histone deacetylases (HDAC) can restore plasticity in the adult in the primary visual cortex (Silingardi et al., 2010; Lennartsson et al., 2015). In the other direction, conditional HDAC2 knock-out specifically in FS-PV cells delays CP closure (Nott et al., 2015). Taken together, these results indicate that dynamic regulation of acetylation may be necessary to maintain a low-plastic state, but also that some degree of redundancy is possible.
Other potential epigenetic factors include microRNAs and long non-coding RNAs (lncRNA). In the primary visual cortex, Mir-29a expression increases with age independent of visual experience, yet is hypothesized to coordinate the expression of different epigenetic and ECM-related genes in FS-PV cells to facilitate juvenile CP or adult plasticity (Napoli et al., 2020). While lncRNAs are gaining attention in epigenetic research, only one study to date has investigated their involvement in CP visual cortex plasticity, highlighting specificity for cortical layer and plasticity state (Benoit et al., 2015). While their function in genomic regulation remains unknown, one hypothesis is that they regulate alternative splicing of transcripts in the maturing cortex. Further single-cell studies will be necessary to uncover the specificity and functions of these transcripts.
Outlook
Non-cell-autonomous factors play a particular role in the regulation of brain CPs owing to the involvement of FS-PV cells, whose maturation includes epigenetic changes and complex changes in their local ECM, which in turn modify their response to these signaling factors. As a result, factors considered permissive in the juvenile brain, such as BDNF and OTX2, may be ignored or even be repressive in the adult brain. Indeed, perturbations of many of these non-cell-autonomous signals are sufficient to delay or accelerate CP, and some of them are crucial for CP onset or closure. Thus, the regulation of FS-PV cell activity is uniquely positioned by integrating information coming from systemic and circadian signals, from local and choroid-plexus-derived molecular signals, and from local and long-range synaptic inputs. Although not highlighted in this review, other factors, such as changes in inflammatory response due to early-life stress or changes in gut bacteria, have recently been shown to affect CPs (Smith et al., 2016; Ikezu et al., 2021; Lupori et al., 2022). Many questions remain, such as whether FS-PV cells act in concert with local glial cells by regulating the secretion of glial-derived factors. It is also unknown how the maturation-dependent changes in DNA methylation and chromatin conformation directly affect the function of FS-PV cells and their response to these factors.
An important point to consider is the implication of FS-PV cells not only in neurodevelopmental disorders, such as amblyopia but also in psychiatric diseases, due in part to their influence on global excitation/inhibition balance in cognitive development (Marín, 2012; Ferguson and Gao, 2018; Hensch and Quinlan, 2018). The re-opening of heightened plasticity in the adult may be a promising therapeutic strategy for these diseases. However, animal models exploring these strategies currently rely on either broad or non-specific methods, such as chondroitinase ABC, fluoxetine, and valproate, or on cell-specific strategies requiring virus-based gene therapy through stereotaxic injections (Dehorter and Pino, 2020; Nelson and Gabard-Durnam, 2020). The panoply of non-cell-autonomous factors for FS-PV cell maturation states include potential modulators of plasticity that may overcome plasticity brakes in adulthood. Improved understanding of the mechanisms involving these factors may lead to new molecules for the precise and gentle re-opening of CPs in therapeutic contexts.
Author Contributions
All authors contributed equally to the writing of this review. All authors contributed to the article and approved the submitted version.
Funding
Funding was provided by the Agence Nationale de la Recherche (ANR-18-CE16-0013-01) and the NeuroGlia Foundation for supporting research costs and salaries.
Conflict of Interest
The authors declare that the research was conducted in the absence of any commercial or financial relationships that could be construed as a potential conflict of interest.
Publisher’s Note
All claims expressed in this article are solely those of the authors and do not necessarily represent those of their affiliated organizations, or those of the publisher, the editors and the reviewers. Any product that may be evaluated in this article, or claim that may be made by its manufacturer, is not guaranteed or endorsed by the publisher.
References
Abbott, N. J., Patabendige, A. A. K., Dolman, D. E. M., Yusof, S. R., and Begley, D. J. (2010). Structure and function of the blood-brain barrier. Neurobiol. Dis. 37, 13–25. doi: 10.1016/j.nbd.2009.07.030
Ackerman, S. D., Perez-Catalan, N. A., Freeman, M. R., and Doe, C. Q. (2021). Astrocytes close a motor circuit critical period. Nature 592, 414–420. doi: 10.1038/s41586-021-03441-2
Akol, I., Kalogeraki, E., Pielecka-Fortuna, J., Fricke, M., and Löwel, S. (2022). MMP2 and MMP9 activity is crucial for adult visual cortex plasticity in healthy and stroke-affected mice. J. Neurosci. 42, 16–32. doi: 10.1523/JNEUROSCI.0902-21.2021
Apulei, J., Kim, N., Testa, D., Ribot, J., Morizet, D., Bernard, C., et al. (2019). Non-cell autonomous OTX2 homeoprotein regulates visual cortex plasticity through Gadd45b/g. Cereb. Cortex 29, 2384–2395. doi: 10.1093/cercor/bhy108
Bagayogo, I. P., and Dreyfus, C. F. (2009). Regulated release of BDNF by cortical oligodendrocytes is mediated through metabotropic glutamate receptors and the PLC pathway. ASN Neuro 1:e00001. doi: 10.1042/AN20090006
Batista, G., and Hensch, T. K. (2019). Critical period regulation by thyroid hormones: Potential mechanisms and sex-specific aspects. Front. Mol. Neurosci. 12:77. doi: 10.3389/fnmol.2019.00077
Benoit, J., Ayoub, A. E., and Rakic, P. (2015). Transcriptomics of critical period of visual cortical plasticity in mice. Proc. Natl. Acad. Sci. U S A 112, 8094–8099. doi: 10.1073/pnas.1509323112
Bernard, C., Vincent, C., Testa, D., Bertini, E., Ribot, J., Di Nardo, A. A., et al. (2016). A mouse model for conditional secretion of specific single-chain antibodies provides genetic evidence for regulation of cortical plasticity by a non-cell autonomous homeoprotein transcription factor. PLoS Genet. 12:e1006035. doi: 10.1371/journal.pgen.1006035
Beurdeley, M., Spatazza, J., Lee, H. H. C., Sugiyama, S., Bernard, C., Di Nardo, A. A., et al. (2012). Otx2 binding to perineuronal nets persistently regulates plasticity in the mature visual cortex. J. Neurosci. 32, 9429–9437. doi: 10.1523/JNEUROSCI.0394-12.2012
Boggio, E. M., Ehlert, E. M., Lupori, L., Moloney, E. B., De Winter, F., Vander Kooi, C. W., et al. (2019). Inhibition of Semaphorin3A promotes ocular dominance plasticity in the adult rat visual cortex. Mol. Neurobiol. 56, 5987–5997. doi: 10.1007/s12035-019-1499-0
Bradshaw, A. D. (2012). Diverse biological functions of the SPARC family of proteins. Int. J. Biochem. Cell Biol. 44, 480–488. doi: 10.1016/j.biocel.2011.12.021
Brennaman, L. H., and Maness, P. F. (2008). Developmental regulation of GABAergic interneuron branching and synaptic development in the prefrontal cortex by soluble neural cell adhesion molecule. Mol. Cell. Neurosci. 37, 781–793. doi: 10.1016/j.mcn.2008.01.006
Brückner, G., Brauer, K., Härtig, W., Wolff, J. R., Rickmann, M. J., Derouiche, A., et al. (1993). Perineuronal nets provide a polyanionic, glia-associated form of microenvironment around certain neurons in many parts of the rat brain. Glia 8, 183–200. doi: 10.1002/glia.440080306
Bueno-Fernandez, C., Perez-Rando, M., Alcaide, J., Coviello, S., Sandi, C., Castillo-Gómez, E., et al. (2021). Long term effects of peripubertal stress on excitatory and inhibitory circuits in the prefrontal cortex of male and female mice. Neurobiol. Stress 14:100322. doi: 10.1016/j.ynstr.2021.100322
Cabungcal, J. H., Steullet, P., Morishita, H., Kraftsik, R., Cuenod, M., Hensch, T. K., et al. (2013). Perineuronal nets protect fast-spiking interneurons against oxidative stress. Proc. Natl. Acad. Sci. U S A 110, 9130–9135. doi: 10.1073/pnas.1300454110
Carceller, H., Guirado, R., Ripolles-Campos, E., Teruel-Marti, V., and Nacher, J. (2020). Perineuronal nets regulate the inhibitory perisomatic input onto parvalbumin interneurons and γ activity in the prefrontal cortex. J. Neurosci. 40, 5008–5018. doi: 10.1523/JNEUROSCI.0291-20.2020
Carulli, D., Rhodes, K. E., Brown, D. J., Bonnert, T. P., Pollack, S. J., Oliver, K., et al. (2006). Composition of perineuronal nets in the adult rat cerebellum and the cellular origin of their components. J. Comp. Neurol. 494, 559–577. doi: 10.1002/cne.20822
Carulli, D., and Verhaagen, J. (2021). An extracellular perspective on cns maturation: perineuronal nets and the control of plasticity. Int. J. Mol. Sci. 22:2434. doi: 10.3390/ijms22052434
Casse, F., Bardou, I., Danglot, L., Briens, A., Montagne, A., Parcq, J., et al. (2012). Glutamate controls tPA recycling by astrocytes, which in turn influences glutamatergic signals. J. Neurosci. 32, 5186–5199. doi: 10.1523/JNEUROSCI.5296-11.2012
Castillo-Gómez, E., Varea, E., Blasco-Ibáñez, J. M., Crespo, C., and Nacher, J. (2011). Polysialic acid is required for Dopamine D2 receptor-mediated plasticity involving inhibitory circuits of the rat medial prefrontal cortex. PLoS One 6:e29516. doi: 10.1371/journal.pone.0029516
Chang, M. C., Park, J. M., Pelkey, K. A., Grabenstatter, H. L., Xu, D., Linden, D. J., et al. (2010). Narp regulates homeostatic scaling of excitatory synapses on parvalbumin-expressing interneurons. Nat. Neurosci. 13, 1090–1097. doi: 10.1038/nn.2621
Chao, M. V. (2003). Neurotrophins and their receptors: a convergence point for many signalling pathways. Nat. Rev. Neurosci. 4, 299–309. doi: 10.1038/nrn1078
Chen, C.-C., Lu, J., Yang, R., Ding, J. B., and Zuo, Y. (2018). Selective activation of parvalbumin interneurons prevents stress-induced synapse loss and perceptual defects. Mol. Psychiatry 23, 1614–1625. doi: 10.1038/mp.2017.159
Cornez, G., Shevchouk, O. T., Ghorbanpoor, S., Ball, G. F., Cornil, C. A., and Balthazart, J. (2020). Testosterone stimulates perineuronal nets development around parvalbumin cells in the adult canary brain in parallel with song crystallization. Horm. Behav. 119:104643. doi: 10.1016/j.yhbeh.2019.104643
Coull, J. A. M., Beggs, S., Boudreau, D., Boivin, D., Tsuda, M., Inoue, K., et al. (2005). BDNF from microglia causes the shift in neuronal anion gradient underlying neuropathic pain. Nature 438, 1017–1021. doi: 10.1038/nature04223
Cui, J., Xu, H., and Lehtinen, M. K. (2021). Macrophages on the margin: choroid plexus immune responses. Trends Neurosci. 44, 864–875. doi: 10.1016/j.tins.2021.07.002
Dani, N., Herbst, R. H., McCabe, C., Green, G. S., Kaiser, K., Head, J. P., et al. (2021). A cellular and spatial map of the choroid plexus across brain ventricles and ages. Cell 184, 3056–3074.e21. doi: 10.1016/j.cell.2021.04.003
de Winter, F., Kwok, J. C. F., Fawcett, J. W., Vo, T. T., Carulli, D., and Verhaagen, J. (2016). The chemorepulsive protein semaphorin 3A and perineuronal net-mediated plasticity. Neural Plast. 2016:3679545. doi: 10.1155/2016/3679545
Dehorter, N., and Pino, I. D. (2020). Shifting developmental trajectories during critical periods of brain formation. Front. Cell. Neurosci. 14:283. doi: 10.3389/fncel.2020.00283
Devienne, G., Picaud, S., Cohen, I., Piquet, J., Tricoire, L., Testa, D., et al. (2021). Regulation of perineuronal nets in the adult cortex by the activity of the cortical network. J. Neurosci. 41, 5779–5790. doi: 10.1523/JNEUROSCI.0434-21.2021
Di Cristo, G., Chattopadhyaya, B., Kuhlman, S. J., Fu, Y., Bélanger, M.-C., Wu, C. Z., et al. (2007). Activity-dependent PSA expression regulates inhibitory maturation and onset of critical period plasticity. Nat. Neurosci. 10, 1569–1577. doi: 10.1038/nn2008
Di Nardo, A. A., Joliot, A., and Prochiantz, A. (2020). Homeoprotein transduction in neurodevelopment and physiopathology. Sci. Adv. 6:eabc6374. doi: 10.1126/sciadv.abc6374
Dick, G., Liktan, C., Alves, J. N., Ehlert, E. M. E., Miller, G. M., Hsieh-Wilson, L. C., et al. (2013). Semaphorin 3A binds to the perineuronal nets via chondroitin sulfate type E motifs in rodent brains. J. Biol. Chem. 288, 27384–27395. doi: 10.1074/jbc.M111.310029
Dityatev, A., Brückner, G., Dityateva, G., Grosche, J., Kleene, R., and Schachner, M. (2007). Activity-dependent formation and functions of chondroitin sulfate-rich extracellular matrix of perineuronal nets. Dev. Neurobiol. 67, 570–588. doi: 10.1002/dneu.20361
Dougherty, K. D., Dreyfus, C. F., and Black, I. B. (2000). Brain-derived neurotrophic factor in astrocytes, oligodendrocytes and microglia/macrophages after spinal cord injury. Neurobiol. Dis. 7, 574–585. doi: 10.1006/nbdi.2000.0318
Dowling, C., and Allen, N. J. (2018). Mice lacking glypican 4 display juvenile hyperactivity and adult social interaction deficits. Brain Plast. 4, 197–209. doi: 10.3233/BPL-180079
Fagiolini, M., Fritschy, J.-M., Löw, K., Möhler, H., Rudolph, U., and Hensch, T. K. (2004). Specific GABAA circuits for visual cortical plasticity. Science 303, 1681–1683. doi: 10.1126/science.1091032
Faini, G., Aguirre, A., Landi, S., Lamers, D., Pizzorusso, T., Ratto, G. M., et al. (2018). Perineuronal nets control visual input via thalamic recruitment of cortical PV interneurons. eLife 7:e41520. doi: 10.7554/eLife.41520
Fame, R. M., and Lehtinen, M. K. (2020). Emergence and developmental roles of the cerebrospinal fluid system. Dev. Cell 52, 261–275. doi: 10.1016/j.devcel.2020.01.027
Farhy-Tselnicker, I., van Casteren, A. C. M., Lee, A., Chang, V. T., Aricescu, A. R., and Allen, N. J. (2017). Astrocyte-secreted glypican 4 regulates release of neuronal pentraxin 1 from axons to induce functional synapse formation. Neuron 96, 428–445.e13. doi: 10.1016/j.neuron.2017.09.053
Fawcett, J. W., Oohashi, T., and Pizzorusso, T. (2019). The roles of perineuronal nets and the perinodal extracellular matrix in neuronal function. Nat. Rev. Neurosci. 20, 451–465. doi: 10.1038/s41583-019-0196-3
Fazzari, P., Paternain, A. V., Valiente, M., Pla, R., Luján, R., Lloyd, K., et al. (2010). Control of cortical GABA circuitry development by Nrg1 and ErbB4 signalling. Nature 464, 1376–1380. doi: 10.1038/nature08928
Ferguson, B. R., and Gao, W.-J. (2018). PV interneurons: critical regulators of E/I balance for prefrontal cortex-dependent behavior and psychiatric disorders. Front. Neural Circuits 12:37. doi: 10.3389/fncir.2018.00037
Filmus, J., Capurro, M., and Rast, J. (2008). Glypicans. Genome Biol. 9:224. doi: 10.1186/gb-2008-9-5-224
Fletcher, J. L., Makowiecki, K., Cullen, C. L., and Young, K. M. (2021). Oligodendrogenesis and myelination regulate cortical development, plasticity and circuit function. Semin. Cell Dev. Biol. 118, 14–23. doi: 10.1016/j.semcdb.2021.03.017
Gan, K. J., and Südhof, T. C. (2020). SPARCL1 promotes excitatory but not inhibitory synapse formation and function independent of neurexins and neuroligins. J. Neurosci. 40, 8088–8102. doi: 10.1523/JNEUROSCI.0454-20.2020
Ghersi-Egea, J.-F., Strazielle, N., Catala, M., Silva-Vargas, V., Doetsch, F., and Engelhardt, B. (2018). Molecular anatomy and functions of the choroidal blood-cerebrospinal fluid barrier in health and disease. Acta Neuropathol. 135, 337–361. doi: 10.1007/s00401-018-1807-1
Gogolla, N., Takesian, A. E., Feng, G., Fagiolini, M., and Hensch, T. K. (2014). Sensory integration in mouse insular cortex reflects GABA circuit maturation. Neuron 83, 894–905. doi: 10.1016/j.neuron.2014.06.033
Green, N. H., Jackson, C. R., Iwamoto, H., Tackenberg, M. C., and McMahon, D. G. (2015). Photoperiod programs dorsal raphe serotonergic neurons and affective behaviors. Curr. Biol. 25, 1389–1394. doi: 10.1016/j.cub.2015.03.050
Greenberg, M. V. C., and Bourc’his, D. (2019). The diverse roles of DNA methylation in mammalian development and disease. Nat. Rev. Mol. Cell Biol. 20, 590–607. doi: 10.1038/s41580-019-0159-6
Grieco, S. F., Wang, G., Mahapatra, A., Lai, C., Holmes, T. C., and Xu, X. (2020). Neuregulin and ErbB expression is regulated by development and sensory experience in mouse visual cortex. J. Comp. Neurol. 528, 419–432. doi: 10.1002/cne.24762
Gu, Y., Huang, S., Chang, M. C., Worley, P., Kirkwood, A., and Quinlan, E. M. (2013). Obligatory role for the immediate early gene NARP in critical period plasticity. Neuron 79, 335–346. doi: 10.1016/j.neuron.2013.05.016
Gu, Y., Tran, T., Murase, S., Borrell, A., Kirkwood, A., and Quinlan, E. M. (2016). Neuregulin-dependent regulation of fast-spiking interneuron excitability controls the timing of the critical period. J. Neurosci. 36, 10285–10295. doi: 10.1523/JNEUROSCI.4242-15.2016
Harkness, J. H., Gonzalez, A. E., Bushana, P. N., Jorgensen, E. T., Hegarty, D. M., Di Nardo, A. A., et al. (2021). Diurnal changes in perineuronal nets and parvalbumin neurons in the rat medial prefrontal cortex. Brain Struct. Funct. 226, 1135–1153. doi: 10.1007/s00429-021-02229-4
Härtig, W., Derouiche, A., Welt, K., Brauer, K., Grosche, J., Mäder, M., et al. (1999). Cortical neurons immunoreactive for the potassium channel Kv3.1b subunit are predominantly surrounded by perineuronal nets presumed as a buffering system for cations. Brain Res. 842, 15–29. doi: 10.1016/s0006-8993(99)01784-9
Hayani, H., Song, I., and Dityatev, A. (2018). Increased excitability and reduced excitatory synaptic input into fast-spiking CA2 interneurons after enzymatic attenuation of extracellular matrix. Front. Cell. Neurosci. 12:149. doi: 10.3389/fncel.2018.00149
Hensch, T. K. (2005). Critical period plasticity in local cortical circuits. Nat. Rev. Neurosci. 6, 877–888. doi: 10.1038/nrn1787
Hensch, T. K., and Quinlan, E. M. (2018). Critical periods in amblyopia. Vis. Neurosci. 35:E014. doi: 10.1017/S0952523817000219
Hou, X., Yoshioka, N., Tsukano, H., Sakai, A., Miyata, S., Watanabe, Y., et al. (2017). Chondroitin sulfate is required for onset and offset of critical period plasticity in visual cortex. Sci. Rep. 7:12646. doi: 10.1038/s41598-017-04007-x
Hu, H., Gan, J., and Jonas, P. (2014). Fast-spiking, parvalbumin+ GABAergic interneurons: from cellular design to microcircuit function. Science 345:1255263. doi: 10.1126/science.1255263
Huang, Z. J., Kirkwood, A., Pizzorusso, T., Porciatti, V., Morales, B., Bear, M. F., et al. (1999). BDNF regulates the maturation of inhibition and the critical period of plasticity in mouse visual cortex. Cell 98, 739–755. doi: 10.1016/s0092-8674(00)81509-3
Huntley, G. W. (2012). Synaptic circuit remodelling by matrix metalloproteinases in health and disease. Nat. Rev. Neurosci. 13, 743–757. doi: 10.1038/nrn3320
Ikezu, S., Yeh, H., Delpech, J.-C., Woodbury, M. E., Van Enoo, A. A., Ruan, Z., et al. (2021). Inhibition of colony stimulating factor 1 receptor corrects maternal inflammation-induced microglial and synaptic dysfunction and behavioral abnormalities. Mol. Psychiatry 26, 1808–1831. doi: 10.1038/s41380-020-0671-2
Jang, M., Gould, E., Xu, J., Kim, E. J., and Kim, J. H. (2019). Oligodendrocytes regulate presynaptic properties and neurotransmission through BDNF signaling in the mouse brainstem. eLife 8:e42156. doi: 10.7554/eLife.42156
Kalish, B. T., Barkat, T. R., Diel, E. E., Zhang, E. J., Greenberg, M. E., and Hensch, T. K. (2020). Single-nucleus RNA sequencing of mouse auditory cortex reveals critical period triggers and brakes. Proc. Natl. Acad. Sci. U S A 117, 11744–11752. doi: 10.1073/pnas.1920433117
Kelly, E. A., Russo, A. S., Jackson, C. D., Lamantia, C. E. L., and Majewska, A. K. (2015). Proteolytic regulation of synaptic plasticity in the mouse primary visual cortex: Analysis of matrix metalloproteinase 9 deficient mice. Front. Cell. Neurosci. 9:369. doi: 10.3389/fncel.2015.00369
Kelwick, R., Desanlis, I., Wheeler, G. N., and Edwards, D. R. (2015). The ADAMTS (A Disintegrin and Metalloproteinase with Thrombospondin motifs) family. Genome Biol. 16:113. doi: 10.1186/s13059-015-0676-3
Kind, P. C., Sengpiel, F., Beaver, C. J., Crocker-Buque, A., Kelly, G. M., Matthews, R. T., et al. (2013). The development and activity-dependent expression of aggrecan in the cat visual cortex. Cereb. Cortex 23, 349–360. doi: 10.1093/cercor/bhs015
Klune, C. B., Jin, B., and DeNardo, L. A. (2021). Linking mPFC circuit maturation to the developmental regulation of emotional memory and cognitive flexibility. eLife 10:e64567. doi: 10.7554/eLife.64567
Kobayashi, Y., Ye, Z., and Hensch, T. K. (2015). Clock genes control cortical critical period timing. Neuron 86, 264–275. doi: 10.1016/j.neuron.2015.02.036
Koizumi, H., Kurabayashi, N., Watanabe, Y., and Sanada, K. (2013). Increased anxiety in offspring reared by circadian clock mutant mice. PLoS One 8:e66021. doi: 10.1371/journal.pone.0066021
Krishnan, K., Wang, B.-S., Lu, J., Wang, L., Maffei, A., Cang, J., et al. (2015). MeCP2 regulates the timing of critical period plasticity that shapes functional connectivity in primary visual cortex. Proc. Natl. Acad. Sci. U S A 112, E4782–E4791. doi: 10.1073/pnas.1506499112
Lee, H. H. C., Bernard, C., Ye, Z., Acampora, D., Simeone, A., Prochiantz, A., et al. (2017). Genetic Otx2 mis-localization delays critical period plasticity across brain regions. Mol. Psychiatry 22, 680–688. doi: 10.1038/mp.2017.1
Lemarchant, S., Pruvost, M., Montaner, J., Emery, E., Vivien, D., Kanninen, K., et al. (2013). ADAMTS proteoglycanases in the physiological and pathological central nervous system. J. Neuroinflammation 10:899. doi: 10.1186/1742-2094-10-133
Lennartsson, A., Arner, E., Fagiolini, M., Saxena, A., Andersson, R., Takahashi, H., et al. (2015). Remodeling of retrotransposon elements during epigenetic induction of adult visual cortical plasticity by HDAC inhibitors. Epigenetics Chromatin 8:55. doi: 10.1186/s13072-015-0043-3
Lenoir, S., Varangot, A., Lebouvier, L., Galli, T., Hommet, Y., and Vivien, D. (2019). Post-synaptic release of the neuronal tissue-type plasminogen activator (tPA). Front. Cell. Neurosci. 13:164. doi: 10.3389/fncel.2019.00164
Lensjø, K. K., Lepperød, M. E., Dick, G., Hafting, T., and Fyhn, M. (2017). Removal of perineuronal nets unlocks juvenile plasticity through network mechanisms of decreased inhibition and increased gamma activity. J. Neurosci. 37, 1269–1283. doi: 10.1523/JNEUROSCI.2504-16.2016
Lesnikova, A., Casarotto, P. C., Fred, S. M., Voipio, M., Winkel, F., Steinzeig, A., et al. (2021). Chondroitinase and antidepressants promote plasticity by releasing TRKB from dephosphorylating control of ptpσ in parvalbumin neurons. J. Neurosci. 41, 972–980. doi: 10.1523/JNEUROSCI.2228-20.2020
Levy, C., Brooks, J. M., Chen, J., Su, J., and Fox, M. A. (2015). Cell-specific and developmental expression of lectican-cleaving proteases in mouse hippocampus and neocortex. J. Comp. Neurol. 523, 629–648. doi: 10.1002/cne.23701
Liu, X., Bates, R., Yin, D. M., Shen, C., Wang, F., Su, N., et al. (2011). Specific regulation of NRG1 isoform expression by neuronal activity. J. Neurosci. 31, 8491–8501. doi: 10.1523/JNEUROSCI.5317-10.2011
Louessard, M., Lacroix, A., Martineau, M., Mondielli, G., Montagne, A., Lesept, F., et al. (2016). Tissue plasminogen activator expression is restricted to subsets of excitatory pyramidal glutamatergic neurons. Mol. Neurobiol. 53, 5000–5012. doi: 10.1007/s12035-015-9432-7
Lowrey, P. L., and Takahashi, J. S. (2011). Genetics of circadian rhythms in mammalian model organisms. Adv. Genet. 74, 175–230. doi: 10.1016/B978-0-12-387690-4.00006-4
Lu, B., Pang, P. T., and Woo, N. H. (2005). The yin and yang of neurotrophin action. Nat. Rev. Neurosci. 6, 603–614. doi: 10.1038/nrn1726
Lu, D., Shang, G., He, X., Bai, X.-C., and Zhang, X. (2021). Architecture of the sema3A/plexinA4/neuropilin tripartite complex. Nat. Commun. 12:3172. doi: 10.1038/s41467-021-23541-x
Lugert, S., Kremer, T., Jagasia, R., Herrmann, A., Aigner, S., Giachino, C., et al. (2017). Glypican-2 levels in cerebrospinal fluid predict the status of adult hippocampal neurogenesis. Sci. Rep. 7:46543. doi: 10.1038/srep46543
Lupori, L., Cornuti, S., Mazziotti, R., Borghi, E., Ottaviano, E., Cas, M. D., et al. (2022). The gut microbiota of environmentally enriched mice regulates visual cortical plasticity. Cell Rep. 38:110212. doi: 10.1016/j.celrep.2021.110212
Makinodan, M., Rosen, K. M., Ito, S., and Corfas, G. (2012). A critical period for social experience-dependent oligodendrocyte maturation and myelination. Science 337, 1357–1360. doi: 10.1126/science.1220845
Marco, E. M., Velarde, E., Llorente, R., and Laviola, G. (2016). Disrupted circadian rhythm as a common player in developmental models of neuropsychiatric disorders. Curr. Top. Behav. Neurosci. 29, 155–181. doi: 10.1007/7854_2015_419
Marín, O. (2012). Interneuron dysfunction in psychiatric disorders. Nat. Rev. Neurosci. 13, 107–120. doi: 10.1038/nrn3155
Masri, S., and Sassone-Corsi, P. (2010). Plasticity and specificity of the circadian epigenome. Nat. Neurosci. 13, 1324–1329. doi: 10.1038/nn.2668
Mataga, N., Nagai, N., and Hensch, T. K. (2002). Permissive proteolytic activity for visual cortical plasticity. Proc. Natl. Acad. Sci. U S A 99, 7717–7721. doi: 10.1073/pnas.102088899
McRae, P. A., Rocco, M. M., Kelly, G., Brumberg, J. C., and Matthews, R. T. (2007). Sensory deprivation alters aggrecan and perineuronal net expression in the mouse barrel cortex. J. Neurosci. 27, 5405–5413. doi: 10.1523/JNEUROSCI.5425-06.2007
Méndez, P., and Bacci, A. (2011). Assortment of GABAergic plasticity in the cortical interneuron melting pot. Neural Plast. 2011:976856. doi: 10.1016/j.saa.2022.121163
Millán-Zambrano, G., Burton, A., Bannister, A. J., and Schneider, R. (2022). Histone post-translational modifications—cause and consequence of genome function. Nat. Rev. Genet. . [Online ahead of print]. doi: 10.1038/s41576-022-00468-7
Miyata, S., Nishimura, Y., and Nakashima, T. (2007). Perineuronal nets protect against amyloid β-protein neurotoxicity in cultured cortical neurons. Brain Res. 1150, 200–206. doi: 10.1016/j.brainres.2007.02.066
Morello, N., Schina, R., Pilotto, F., Phillips, M., Melani, R., Plicato, O., et al. (2018). Loss of Mecp2 causes atypical synaptic and molecular plasticity of parvalbumin-expressing interneurons reflecting rett syndrome-like sensorimotor defects. eNeuro 5:ENEURO.0086-18.2018. doi: 10.1523/ENEURO.0086-18.2018
Morishita, H., Kundakovic, M., Bicks, L., Mitchell, A., and Akbarian, S. (2015). Interneuron epigenomes during the critical period of cortical plasticity: implications for schizophrenia. Neurobiol. Learn. Mem. 124, 104–110. doi: 10.1016/j.nlm.2015.03.005
Myung, J., Schmal, C., Hong, S., Tsukizawa, Y., Rose, P., Zhang, Y., et al. (2018). The choroid plexus is an important circadian clock component. Nat. Commun. 9:1062. doi: 10.1038/s41467-018-03507-2
Nadanaka, S., Miyata, S., Yaqiang, B., Tamura, J. I., Habuchi, O., and Kitagawa, H. (2020). Reconsideration of the semaphorin-3a binding motif found in chondroitin sulfate using galnac4s-6st-knockout mice. Biomolecules 10:1499. doi: 10.3390/biom10111499
Nakamura, M., Valerio, P., Bhumika, S., and Barkat, T. R. (2020). Sequential organization of critical periods in the mouse auditory system. Cell Rep. 32:108070. doi: 10.1016/j.celrep.2020.108070
Napoli, D., Lupori, L., Mazziotti, R., Sagona, G., Bagnoli, S., Samad, M., et al. (2020). MiR-29 coordinates age-dependent plasticity brakes in the adult visual cortex. EMBO Rep. 21:e50431. doi: 10.15252/embr.202050431
Nawreen, N., Cotella, E. M., Morano, R., Mahbod, P., Dalal, K., Fitzgerald, M., et al. (2020). Chemogenetic inhibition of infralimbic prefrontal cortex GABAergic parvalbumin interneurons attenuates the impact of chronic stress in male mice. eNeuro 7:ENEURO.0423-19.2020. doi: 10.1523/ENEURO.0423-19.2020
Nelson, C. A., and Gabard-Durnam, L. J. (2020). Early adversity and critical periods: neurodevelopmental consequences of violating the expectable environment. Trends Neurosci. 43, 133–143. doi: 10.1016/j.tins.2020.01.002
Nott, A., Cho, S., Seo, J., and Tsai, L. H. (2015). HDAC2 expression in parvalbumin interneurons regulates synaptic plasticity in the mouse visual cortex. Neuroepigenetics 1, 34–40. doi: 10.1016/j.nepig.2014.10.005
Page, C. E., Shepard, R., Heslin, K., and Coutellier, L. (2019). Prefrontal parvalbumin cells are sensitive to stress and mediate anxiety-related behaviors in female mice. Sci. Rep. 9:19772. doi: 10.1038/s41598-019-56424-9
Pantazopoulos, H., Gisabella, B., Rexrode, L., Benefield, D., Yildiz, E., Seltzer, P., et al. (2020). Circadian rhythms of perineuronal net composition. eNeuro 7:ENEURO.0034-19.2020. doi: 10.1523/ENEURO.0034-19.2020
Parkhurst, C. N., Yang, G., Ninan, I., Savas, J. N., Yates, J. R., Lafaille, J. J., et al. (2013). Microglia promote learning-dependent synapse formation through brain-derived neurotrophic factor. Cell 155, 1596–1609. doi: 10.1016/j.cell.2013.11.030
Patrizi, A., Awad, P. N., Chattopadhyaya, B., Li, C., Di Cristo, G., and Fagiolini, M. (2020). Accelerated hyper-maturation of parvalbumin circuits in the absence of MeCP2. Cereb. Cortex 30, 256–268. doi: 10.1093/cercor/bhz085
Piekarski, D. J., Boivin, J. R., and Wilbrecht, L. (2017). Ovarian hormones organize the maturation of inhibitory neurotransmission in the frontal cortex at puberty onset in female mice. Curr. Biol. 27, 1735–1745.e3. doi: 10.1016/j.cub.2017.05.027
Pizzorusso, T., Medini, P., Berardi, N., Chierzi, S., Fawcett, J. W., and Maffei, L. (2002). Reactivation of ocular dominance plasticity in the adult visual cortex. Science 298, 1248–1251. doi: 10.1126/science.1072699
Praetorius, J., and Damkier, H. H. (2017). Transport across the choroid plexus epithelium. Am. J. Physiol. Cell Physiol. 312, C673–C686. doi: 10.1152/ajpcell.00041.2017
Putignano, E., Lonetti, G., Cancedda, L., Ratto, G., Costa, M., Maffei, L., et al. (2007). Developmental downregulation of histone posttranslational modifications regulates visual cortical plasticity. Neuron 53, 747–759. doi: 10.1016/j.neuron.2007.02.007
Redzic, Z. (2011). Molecular biology of the blood-brain and the blood-cerebrospinal fluid barriers: similarities and differences. Fluids Barriers CNS 8:3. doi: 10.1186/2045-8118-8-3
Reh, R. K., Dias, B. G., Nelson, C. A., Kaufer, D., Werker, J. F., Kolb, B., et al. (2020). Critical period regulation across multiple timescales. Proc. Natl. Acad. Sci. U S A 117, 23242–23251. doi: 10.1073/pnas.1820836117
Reinhard, S. M., Razak, K., and Ethell, I. M. (2015). A delicate balance: role of MMP-9 in brain development and pathophysiology of neurodevelopmental disorders. Front. Cell. Neurosci. 9:280. doi: 10.3389/fncel.2015.00280
Ribic, A., Biederer, T., and Morishita, H. (2019). Emerging roles of synapse organizers in the regulation of critical periods. Neural Plast. 2019:1538137. doi: 10.1155/2019/1538137
Ribot, J., Breton, R., Calvo, C.-F., Moulard, J., Ezan, P., Zapata, J., et al. (2021). Astrocytes close the mouse critical period for visual plasticity. Science 373, 77–81. doi: 10.1126/science.abf5273
Rossier, J., Bernard, A., Cabungcal, J.-H., Perrenoud, Q., Savoye, A., Gallopin, T., et al. (2015). Cortical fast-spiking parvalbumin interneurons enwrapped in the perineuronal net express the metallopeptidases Adamts8, Adamts15 and Neprilysin. Mol. Psychiatry 20, 154–161. doi: 10.1038/mp.2014.162
Rutishauser, U. (2008). Polysialic acid in the plasticity of the developing and adult vertebrate nervous system. Nat. Rev. Neurosci. 9, 26–35. doi: 10.1038/nrn2285
Sakai, A., Nakato, R., Ling, Y., Hou, X., Hara, N., Iijima, T., et al. (2017). Genome-wide target analyses of Otx2 homeoprotein in postnatal cortex. Front. Neurosci. 11:307. doi: 10.3389/fnins.2017.00307
Salter, M. W., and Beggs, S. (2014). Sublime microglia: expanding roles for the guardians of the CNS. Cell 158, 15–24. doi: 10.1016/j.cell.2014.06.008
Santello, M., Toni, N., and Volterra, A. (2019). Astrocyte function from information processing to cognition and cognitive impairment. Nat. Neurosci. 22, 154–166. doi: 10.1038/s41593-018-0325-8
Shi, L., and Bergson, C. M. (2020). Neuregulin 1: an intriguing therapeutic target for neurodevelopmental disorders. Transl. Psychiatry 10:190. doi: 10.1038/s41398-020-00868-5
Silingardi, D., Scali, M., Belluomini, G., and Pizzorusso, T. (2010). Epigenetic treatments of adult rats promote recovery from visual acuity deficits induced by long-term monocular deprivation. Eur. J. Neurosci. 31, 2185–2192. doi: 10.1111/j.1460-9568.2010.07261.x
Singh, S. K., Stogsdill, J. A., Pulimood, N. S., Dingsdale, H., Kim, Y. H., Pilaz, L.-J., et al. (2016). Astrocytes assemble thalamocortical synapses by bridging NRX1α and NL1 via hevin. Cell 164, 183–196. doi: 10.1016/j.cell.2015.11.034
Sipe, G. O., Lowery, R. L., Tremblay, M.-È., Kelly, E. A., Lamantia, C. E., and Majewska, A. K. (2016). Microglial P2Y12 is necessary for synaptic plasticity in mouse visual cortex. Nat. Commun. 7:10905. doi: 10.1038/ncomms10905
Smith, M. R., Burman, P., Sadahiro, M., Kidd, B. A., Dudley, J. T., and Morishita, H. (2016). Integrative analysis of disease signatures shows inflammation disrupts juvenile experience-dependent cortical plasticity. eNeuro 3:ENEURO.0240-16.2016. doi: 10.1523/ENEURO.0240-16.2016
Sohal, V. S., Zhang, F., Yizhar, O., and Deisseroth, K. (2009). Parvalbumin neurons and gamma rhythms enhance cortical circuit performance. Nature 459, 698–702. doi: 10.1038/nature07991
Spatazza, J., Lee, H. H. C., DiNardo, A. A., Tibaldi, L., Joliot, A., Hensch, T. K., et al. (2013). Choroid-plexus-derived Otx2 homeoprotein constrains adult cortical plasticity. Cell Rep. 3, 1815–1823. doi: 10.1016/j.celrep.2013.05.014
Sullivan, C. S., Gotthard, I., Wyatt, E. V., Bongu, S., Mohan, V., Weinberg, R. J., et al. (2018). Perineuronal net protein neurocan inhibits NCAM/EphA3 repellent signaling in GABAergic interneurons. Sci. Rep. 8:6143. doi: 10.1038/s41598-018-24272-8
Sun, Y., Ikrar, T., Davis, M. F., Gong, N., Zheng, X., Luo, Z. D., et al. (2016). Neuregulin-1/ErbB4 signaling regulates visual cortical plasticity. Neuron 92, 160–173. doi: 10.1016/j.neuron.2016.08.033
Sun, Q., Li, X., Ren, M., Zhao, M., Zhong, Q., Ren, Y., et al. (2019). A whole-brain map of long-range inputs to GABAergic interneurons in the mouse medial prefrontal cortex. Nat. Neurosci. 22, 1357–1370. doi: 10.1038/s41593-019-0429-9
Suttkus, A., Rohn, S., Weigel, S., Glöckner, P., Arendt, T., and Morawski, M. (2014). Aggrecan, link protein and tenascin-R are essential components of the perineuronal net to protect neurons against iron-induced oxidative stress. Cell Death Dis. 5:e1119. doi: 10.1038/cddis.2014.25
Sweeney, M. D., Zhao, Z., Montagne, A., Nelson, A. R., and Zlokovic, B. V. (2019). Blood-brain barrier: from physiology to disease and back. Physiol. Rev. 99, 21–78. doi: 10.1152/physrev.00050.2017
Testa, D., Prochiantz, A., and Di Nardo, A. A. (2019). Perineuronal nets in brain physiology and disease. Semin. Cell Dev. Biol. 89, 125–135. doi: 10.1016/j.semcdb.2018.09.011
Testa-Silva, G., Loebel, A., Giugliano, M., de Kock, C. P. J., Mansvelder, H. D., and Meredith, R. M. (2012). Hyperconnectivity and slow synapses during early development of medial prefrontal cortex in a mouse model for mental retardation and autism. Cereb. Cortex 22, 1333–1342. doi: 10.1093/cercor/bhr224
Tognini, P., Napoli, D., Tola, J., Silingardi, D., Della Ragione, F., D’esposito, M., et al. (2015). Experience-dependent DNA methylation regulates plasticity in the developing visual cortex. Nat. Neurosci. 18, 956–958. doi: 10.1038/nn.4026
Uchida, K., Hasuoka, K., Fuse, T., Kobayashi, K., Moriya, T., Suzuki, M., et al. (2021). Thyroid hormone insufficiency alters the expression of psychiatric disorder-related molecules in the hypothyroid mouse brain during the early postnatal period. Sci. Rep. 11:6723. doi: 10.1038/s41598-021-86237-8
Vahaba, D. M., and Remage-Healey, L. (2018). Neuroestrogens rapidly shape auditory circuits to support communication learning and perception: evidence from songbirds. Horm. Behav. 104, 77–87. doi: 10.1016/j.yhbeh.2018.03.007
Van Bodegom, M., Homberg, J. R., and Henckens, M. J. A. G. (2017). Modulation of the hypothalamic-pituitary-adrenal axis by early life stress exposure. Front. Cell. Neurosci. 11:87. doi: 10.3389/fncel.2017.00087
Van’t Spijker, H. M., Rowlands, D., Rossier, J., Haenzi, B., Fawcett, J. W., and Kwok, J. C. F. (2019). Neuronal pentraxin 2 binds PNNs and enhances PNN formation. Neural Plast. 2019:6804575. doi: 10.1155/2019/6804575
Vasistha, N. A., Pardo-Navarro, M., Gasthaus, J., Weijers, D., Müller, M. K., García-González, D., et al. (2020). Maternal inflammation has a profound effect on cortical interneuron development in a stage and subtype-specific manner. Mol. Psychiatry 25, 2313–2329. doi: 10.1038/s41380-019-0539-5
Venturino, A., Schulz, R., Jesús-Cortés, H. D., Maes, M. E., Nagy, B., Reilly-Andújar, F., et al. (2021). Microglia enable mature perineuronal nets disassembly upon anesthetic ketamine exposure or 60-Hz light entrainment in the healthy brain. Cell Rep. 36:109313. doi: 10.1016/j.celrep.2021.109313
Vincent, C., Gilabert-Juan, J., Gibel-Russo, R., Alvarez-Fischer, D., Krebs, M.-O., Le Pen, G., et al. (2021). Non-cell-autonomous OTX2 transcription factor regulates anxiety-related behavior in the mouse. Mol. Psychiatry 26, 6469–6480. doi: 10.1038/s41380-021-01132-y
Vo, T., Carulli, D., Ehlert, E. M. E., Kwok, J. C. F., Dick, G., Mecollari, V., et al. (2013). The chemorepulsive axon guidance protein semaphorin3A is a constituent of perineuronal nets in the adult rodent brain. Mol. Cell. Neurosci. 56, 186–200. doi: 10.1016/j.mcn.2013.04.009
Wiese, S., Karus, M., and Faissner, A. (2012). Astrocytes as a source for extracellular matrix molecules and cytokines. Front. Pharmacol. 3:120. doi: 10.3389/fphar.2012.00120
Willing, J., and Wagner, C. K. (2016). Progesterone receptor expression in the developing mesocortical dopamine pathway: importance for complex cognitive behavior in adulthood. Neuroendocrinology 103, 207–222. doi: 10.1159/000434725
Xenos, D., Kamceva, M., Tomasi, S., Cardin, J. A., Schwartz, M. L., and Vaccarino, F. M. (2018). Loss of TrkB signaling in parvalbumin-expressing basket cells results in network activity disruption and abnormal behavior. Cereb. Cortex 28, 3399–3413. doi: 10.1093/cercor/bhx173
Yang, S.-S., Mack, N. R., Shu, Y., and Gao, W.-J. (2021). Prefrontal GABAergic interneurons gate long-range afferents to regulate prefrontal cortex-associated complex behaviors. Front. Neural Circuits 15:716408. doi: 10.3389/fncir.2021.716408
Keywords: parvalbumin (PV), homeoprotein, astrocyctes, oligodedrocytes, microglia, epigenetics, perineuronal net (PNN)
Citation: Gibel-Russo R, Benacom D and Di Nardo AA (2022) Non-Cell-Autonomous Factors Implicated in Parvalbumin Interneuron Maturation and Critical Periods. Front. Neural Circuits 16:875873. doi: 10.3389/fncir.2022.875873
Received: 14 February 2022; Accepted: 04 April 2022;
Published: 26 April 2022.
Edited by:
Paola Tognini, University of Pisa, ItalyReviewed by:
Gabriele Chelini, University of Trento, ItalyRamon Guirado, University of Valencia, Spain
Annarita Patrizi, German Cancer Research Center (DKFZ), Germany
Copyright © 2022 Gibel-Russo, Benacom and Di Nardo. This is an open-access article distributed under the terms of the Creative Commons Attribution License (CC BY). The use, distribution or reproduction in other forums is permitted, provided the original author(s) and the copyright owner(s) are credited and that the original publication in this journal is cited, in accordance with accepted academic practice. No use, distribution or reproduction is permitted which does not comply with these terms.
*Correspondence: Ariel A. Di Nardo, YXJpZWwuZGluYXJkb0Bjb2xsZWdlLWRlLWZyYW5jZS5mcg==
†These authors have contributed equally to this work