- 1Division of Neurobiology, Faculty of Biology, Ludwig-Maximilians-University Munich, Munich, Germany
- 2Research Group Molecular Neurogenetics, Max Planck Institute of Psychiatry, Munich, Germany
Sensory systems have to be malleable to context-dependent modulations occurring over different time scales, in order to serve their evolutionary function of informing about the external world while also eliciting survival-promoting behaviors. Stress is a major context-dependent signal that can have fast and delayed effects on sensory systems, especially on the auditory system. Urocortin 3 (UCN3) is a member of the corticotropin-releasing factor family. As a neuropeptide, UCN3 regulates synaptic activity much faster than the classic steroid hormones of the hypothalamic-pituitary-adrenal axis. Moreover, due to the lack of synaptic re-uptake mechanisms, UCN3 can have more long-lasting and far-reaching effects. To date, a modest number of studies have reported the presence of UCN3 or its receptor CRFR2 in the auditory system, particularly in the cochlea and the superior olivary complex, and have highlighted the importance of this stress neuropeptide for protecting auditory function. However, a comprehensive map of all neurons synthesizing UCN3 or CRFR2 within the auditory pathway is lacking. Here, we utilize two reporter mouse lines to elucidate the expression patterns of UCN3 and CRFR2 in the auditory system. Additional immunolabelling enables further characterization of the neurons that synthesize UCN3 or CRFR2. Surprisingly, our results indicate that within the auditory system, UCN3 is expressed predominantly in principal cells, whereas CRFR2 expression is strongest in non-principal, presumably multisensory, cell types. Based on the presence or absence of overlap between UCN3 and CRFR2 labeling, our data suggest unusual modes of neuromodulation by UCN3, involving volume transmission and autocrine signaling.
Introduction
Temporary changes in hearing during stressful situations or episodes of anxiety or sadness are commonly experienced by humans and animals (Neuser and Knoop, 1986; Schmitt et al., 2000; Horner, 2003; Kadner et al., 2006; Mazurek et al., 2010; Pacheco-Unguetti and Parmentier, 2014; Lin et al., 2016; Szczepek et al., 2018). Such changes can range from decreased attention to sincere auditory hallucinations (Hoskin et al., 2014). More profound and chronic stress system dysregulation such as with post-traumatic stress disorder (PTSD) can come with a plethora of associated auditory abnormalities (Shalev et al., 2000; Guthrie and Bryant, 2005; Karl et al., 2006; Clifford et al., 2018). Conversely, specific acoustic qualities of sound can trigger stress-like sensations or even fear in both humans and animals, with the acoustic startle response being the most prominent example (Davis, 1984; Trost et al., 2012; Koelsch et al., 2013; Koelsch, 2014; Behler and Uppenkamp, 2020; Hegewald et al., 2020).
There is a clear reciprocity between stress and sensory systems. On one hand, the stress response relies on incoming information from the external environment provided by sensory systems to execute its function of maintaining allostasis, while on the other hand, sensory systems have to adapt constantly to changes in external conditions that are potentially threatening to the animal’s current state. Since ears, unlike eyes, are open and sensing for 24 h a day, the auditory system plays a pivotal role in survival and has to be protected not only from noise trauma, but also from degenerative damage resulting from non-auditory stressors such as infections and head trauma. Hence, strong two-way interactions between stress signaling and the auditory system are warranted.
In the auditory system, the medial subdivision of the medial geniculate body (MGBm), the external cortex of the inferior colliculus (ICe) and the auditory cortex (Au) are well-known areas of connection to stress pathways. In particular, the MGBm-amygdala-pathway is known to be involved in auditory fear conditioning and in attributing emotional salience to sounds (LeDoux et al., 1984). The ICe receives hypothalamic input (Sakanaka et al., 1987) which might be involved in circadian regulation of stress, since both areas contain independent clocks (Park et al., 2016). The primary auditory cortex (Au1) sends direct projections to the lateral amygdala, which in turn projects to the auditory association cortex (Romanski and LeDoux, 1993; McDonald, 1998; Phelps and LeDoux, 2005). On top of these discrete connections, a wide network of mostly serotoninergic and cholinergic projections coming from the reticular formation innervate the auditory system throughout its extent from cochlea to cortex (Klepper and Herbert, 1991; Hurley and Hall, 2011; Schofield et al., 2011). Noradrenergic inputs, which mostly target the dorsal (DCN) and the granule cell domain (GCD) of the cochlear nucleus (CN), the ICe, the superior olivary complex (SOC), and the Au are even less understood (Levitt and Moore, 1978; Klepper and Herbert, 1991; Mulders and Robertson, 2001).
Non-auditory areas are crucial in shaping auditory responses by stressors coming from other sensory modalities. For example, olfactory stimulation with predator odor has been shown to trigger changes in neuronal firing rates of the locus coeruleus, a brainstem nucleus that receives auditory input and releases norepinephrine (Curtis et al., 2012). There, presentation of a stressor such as corticotropin-releasing factor (CRF) or a predator odor increases spontaneous tonic firing and decreases sound-evoked phasic firing of the neurons. Such a shift from tonic to phasic firing in locus coeruleus neurons is suggested to facilitate different behavioral reactions (Aston-Jones and Cohen, 2005).
The activation of the hypothalamic-pituitary-adrenal axis (HPA) is commonly regarded as the cornerstone of the stress response. Briefly, this entails release of CRF from hypothalamic paraventricular nucleus’ neurons into the anterior pituitary gland, which in turn secretes adrenocorticotropic hormone (ACTH) that, upon reaching the adrenal glands, stimulates cortisol synthesis and release. Cortisol targets specific cell groups throughout the body via blood circulation (Charmandari et al., 2005). In its target cells, cortisol binds to its nuclear receptor and elicits the transcriptional modifications to adapt cellular function. It takes about 30 min to reach sufficient ACTH concentrations in the blood for cortisol to be synthetized (Sapolsky et al., 2000), a delay that could impede the timeliness of the response.
Thanks to the pioneering work of the Vetter-lab, a much faster, HPA axis-independent stress axis has been discovered in the cochlea, that not only involves all the constituents for cortisol production, but also a local CRF system (Basappa et al., 2012). The CRF system consists of two receptors CRFR1 and CRFR2, four ligands and the non-membrane-bound CRF-binding-protein. The four ligands, CRF, urocortin 1 (UCN1), 2 (UCN2), and 3 (UCN3) display different affinities for the two receptor types. CRF and UCN1 are most affine to CRFR1 but may also at bind to CRFR2 at very high concentrations (Chang et al., 1993; Chen et al., 1993; Vita et al., 1993; Perrin et al., 1995; Deussing and Chen, 2018). In contrast, UCN2 and UCN3 bind exclusively to CRFR2 (Lewis et al., 2001; Reyes et al., 2001; Deussing et al., 2010).
The most abundant stress peptide in the auditory system is CRF, which is expressed in the cochlea (Basappa et al., 2012), the principal neurons of the lateral superior olive (LSO), the ventral nucleus of the lateral lemniscus (VNLL), the inferior colliculus (IC), and the medial geniculate body (MGB) (including the peripeduncular and posterior intralaminar nuclei), the Brodman areas 20, 39, 40, and 41 and, although much weaker, in the deep layers of the DCN and lateral part of the medial nucleus of the trapezoid body (MNTB) (Imaki et al., 1991). UCN1 expression in the auditory system is much more distinct and has been reported only in a small subset of lateral olivocochlear bundle cells (LOC) with high characteristic frequencies (CF) as well as in the neuropil of the DCN deep layers and IC (Kozicz et al., 1998; Kaiser et al., 2011).
To date, no data are available that suggest UCN2 expression in the auditory system (Lewis et al., 2001). That makes UCN3 the primary ligand for CRFR2. So far, UCN3 expression in the auditory system was reported in the cochlea and SOC (Lewis et al., 2001; Li et al., 2002; Fischl et al., 2019). However, the physiological importance of UCN3 and its receptor CRFR2 for auditory function has been emphasized by knockout models of UCN3 and CRFR2, both showing enhanced vulnerability to noise trauma (Graham et al., 2010; Fischl et al., 2019). Although noise trauma and systemic stress are two major causes of hearing loss in humans (Masuda et al., 2012), our knowledge of the UCN3–CRFR2 contribution to auditory signal processing is rather limited.
In the present study, we take advantage of two reporter mouse lines, one for UCN3 and the other for its receptor CRFR2 to provide an extensive description of UCN3 and CRFR2 expression in the central auditory system. Immunocytochemistry was used for co-labeling the neurons highlighted by the reporter to allow the best possible identification of specific areas and cell types.
Characteristic expression patterns throughout this study showed UCN3 expression in principal auditory neurons and CRFR2 expression in non-principal/multimodal neurons of the same nucleus. Fewer areas revealed neurons that express both the ligand as well as the receptor. Together, both types of expression suggest that volume transmission as well as autocrine regulation are possible signaling mechanisms for UCN3.
Materials and Methods
All experimental procedures were reviewed and approved by the Bavarian district government (ROB-55.2-2532.Vet_02-18-1183) and were done according to the European Communities Council Directive (2010/63/EU). Mice were housed in a vivarium with a normal light–dark cycle (12 h light/12 h dark) and food and water ad libitum.
Mouse Models
Experiments were conducted on four UCN3 reporter mice (UCN3 tdTom) and three CRFR2 reporter mice (CRFR2 tdTom) of both sexes (five males and two females). After weaning, mice were separated by sex and group housed with same sex littermates until used in the experiment. In this absence of deliberate stressors, no differences in expression patterns were observed between males and females.
Reporter mice were generated by breeding UCN3-Cre mice [Tg(UCN3-Cre)KF31Gsat; The Gene Expression Nervous System Atlas (GENSAT) Project; Mutant Mouse Resource & Research Centers (MMRC) stock no: 033033-UCD] or CRFR2-Cre mice (Henckens et al., 2017) with R26CAG–LSL–tdTomato mice (Ai9, The Jackson Laboratory, Bar Harbor, ME, United States; stock no: 007905) as previously described (Shemesh et al., 2016).
Immunohistochemistry
Mice received an overdose of pentobarbital (400 mg kg–1 body weight; I.P.) and were perfusion-fixed with 4% paraformaldehyde (PFA) intracardially. Following overnight postfixation in 4% PFA, brainstems were sectioned coronally at 50 μm using a vibrating blade microtome (V1200S, Leica, Wetzlar, Germany). After rinsing in phosphate-buffered saline (PBS), sections were transferred to a blocking solution containing 1% bovine serum albumin, 0.5% Triton X-100, and 0.1% saponin in PBS. The floating sections were then incubated for 48 h at 4°C in blocking solution containing primary antibodies, which were used in different combinations as specified in the figures. Sections were washed three times in PBS for 15 min and were then incubated with secondary antibodies overnight at 4°C. Antibodies against calbindin D28K (SWANT, #07F Burgdorf, Switzerland, 1:300) were combined with secondary antibodies Alexa 488 (Dianova anti-rabbit, #115-545-206, Hamburg, Germany, 1:200). Antibodies against parvalbumin PV-28 (SWANT, Burgdorf, Switzerland, 1:500) were combined with secondary antibodies AMCA (Dianova, anti-mouse, Hamburg, Germany, #715-156-150, 1:100). Antibodies against VGluT1 (Synaptic Systems #135304, Göttingen, Germany, 1:500) were combined with secondary antibodies Alexa 647 (Dianova, anti-guinea pig, Hamburg, Germany, #706-605-148). Antibodies against vesicular glutamate transporter type 2 (VGluT2) (Synaptic Systems, Göttingen, Germany, #135402, 1:500) were combined with secondary antibodies Alexa 488 (Dianova anti-rabbit, #115-545-206, Hamburg, Germany, 1:200). Antibodies against glycine transporter type 2 (GlyT2; Millipore #1773, 1:1000) were combined with secondary antibodies Alexa 647 (Dianova, anti-guinea pig, Hamburg, Germany, #706-605-148). Antibodies against VChat (Synaptic System #139105, Göttingen, Germany, 1:200) were combined with secondary antibodies Alexa 647 (Dianova, anti-guinea pig, Hamburg, Germany, #706-605-148). Sections were washed in PBS, mounted on slides and coverslipped with vectashield mounting medium (Vector Laboratories, Burlingame, CA, United States).
Confocal Microscopy and Image Analysis
Confocal optical sections were acquired with a confocal laser-scanning microscope equipped with HCX PL APO CS 20×/NA0.7 and HCX PL APO Lambda Blue 63×/NA1.4 immersion oil objectives (Leica). Fluorochromes were visualized with excitation wavelengths of 405 nm (emission filter 410–430 nm) for amino-methylcoumarin (AMCA), 488 nm (emission filter 510–540 nm) for Alexa 488, 561 nm (emission filter 565–585 nm) for Cy3, and 647 nm (emission filter 663–738 nm) for Alexa 647. For each optical section, the images were collected sequentially for the different fluorochromes. Stacks of 8-bit grayscale images were obtained with axial distances of 290 nm between optical sections and pixel sizes of 120–1520 nm depending on the selected zoom factor and objective. To improve the signal-to-noise ratio, images were averaged from three successive scans. RGB stacks, montages of RGB optical sections and maximum-intensity projections were assembled using the ImageJ StackGroom plugin. Color schemes were adjusted to C-M-Y-W.
Results
Expression patterns of UCN3 and CRFR2 in the cochlea and spiral ganglion neurons were reported in detail before (Graham et al., 2010; Fischl et al., 2019), so that here, we start with the description of UCN3 and CRFR2 expression in the CN. From there we proceed to the SOC, the LL, the IC, and the MGB. The auditory cortex exhibited hardly any UCN3 or CRFR2 expression so that we focused on subcortical auditory areas.
Cochlear Nucleus
The mouse CN (Figure 1A) is divided into four main areas: anteroventral (AVCN), posteroventral (PVCN), and dorsal (DCN) CN as well as the granule cell domain (GCD) (Harrison and Irving, 1965; Mugnaini et al., 1980; Willard and Ryugo, 1983). AVCN and PVCN contain similar neuronal cell types such as globular (GBCs) and spherical bushy cells (SBCs), stellate cells (T-stellate and D-stellate), and little cells (LCs), all of which are regarded as principal auditory neurons (Cant, 1993; Oertel et al., 2011; Ngodup et al., 2020). In addition, the PVCN also contains octopus cells (OCs) which inhabit their own domain near the auditory nerve root region (Oertel et al., 2000). Location, morphology and co-labeling with recognized markers were used to identify these neurons.
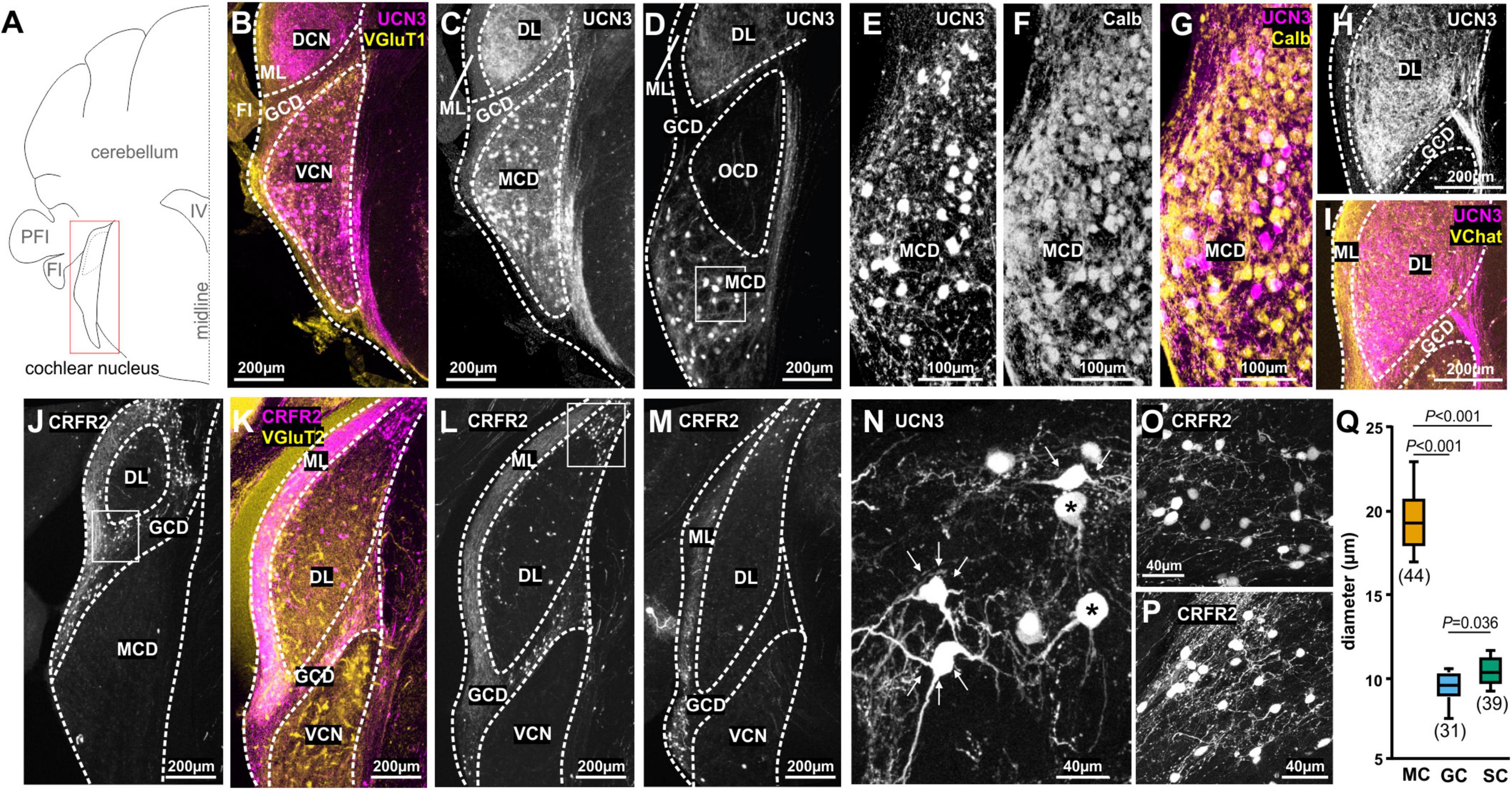
Figure 1. Spatial segregation of UCN3 and CRFR2 expression in the cochlear nucleus. (A) Schematic coronal section showing the cochlear nucleus as the region of interest. PFI, paraflocculus; FI, flocculus; IV, fourth ventricle. (B) UCN3 expression (magenta) in the VCN and in the DCN VGluT1 (yellow) labeling dominates in the molecular layer (ML) of the DCN and the granule cell domain (GDC). (C) Same image as in (B). UCN-expression in neuronal cell bodies of the VCN magnocellular domain (MCD) and in dense fiber networks in the deep layer (DL) of DCN. (D) More caudal coronal section highlighting the lack of UCN3 expression in the octopus cell domain (OCD). Square in the MCD indicates UCN3-positive cell bodies in the MCD, shown in higher magnification in (M). (E–H) Co-staining of UCN3-positive neurons in the MCD (E) with calbindin (F). Neurons co-labeled for UCN3 (magenta) and calbindin (yellow) appear white. (H,I) UCN3-expressing fibers in the DCN deep layer (DL) do not enter the molecular layer (ML), which is characterized by yellow VChat labeling in (I). (J–L) Coronal sections in (J–L) show CRFR2 expression in different rostro-caudal positions along the cochlear nucleus. (J) CRFR2 expression in the parallel fibers of the DCN molecular layer (ML), in granule cells of the granule cell domain (GCD), but not in the MCD of the VCN. Square in the GCD indicates UCN3-positive cell bodies shown in higher magnification in (O). (K) Co-staining of CRFR2 (magenta) and VGluT2 (yellow) in a more caudal image compared to (J). White color in the GCD and the ML suggest co-labeling. (L) Same image as in (K). CRFR2 expression in ML parallel fibers, in GCD granule cells and in neurons of the small cell cap (white square), which are shown in higher magnification in (P). (M) CRFR2 expression in a more caudal section compared to (J,K), depicting again parallel fibers, granule cells, and small cells. (N) Higher magnification of UCN3-positive MCD neurons reveal a globular (asterisk) or multipolar shape (white arrows). (O) Higher magnification of CRFR2-expressing granule cells. (P) Higher magnification of CRFR2-expressing small cap cells. (Q) Quantification of cell diameters of magnocellular cells of the VCN (MC), granule cells (GC), and small cells (SC). ANOVA was used to test for statistical differences.
The UCN3 tdTom reporter mouse showed an abundance of UCN3-positive neuronal cell bodies in the magnocellular domain (MCD) of AVCN and PVCN (Figures 1B–G). Auditory nerve terminals innervate the VCN tonotopically, with ventro-lateral neurons receiving inputs originating from the apex of the cochlea and therefore being responsive to low-frequency sounds. Neurons located more dorso-medially receive input from the base of the cochlea, which is sensitive to higher sound frequencies (Liberman, 1991, 1993). UCN3-positive neurons are found predominantly in the ventro-lateral, low-frequency part of the AVCN and progress to occupy more intermediate areas in the PVCN. No UCN3 expression was observed in the octopus cell domain (OCD), which is visualized by the empty space visible in Figure 1D. Only some of the UCN3-positive neurons in the MCD of the VCN co-localize with the Ca2+ binding protein calbindin (Figures 1E–G). Therefore we used size measures to assess if these UCN3-positive neurons qualify as canonical principal auditory neurons, which in the MCD are quite large in diameter (Oertel et al., 2000; Bazwinsky et al., 2008; Lauer et al., 2013). In addition to the large soma size (mean ± SD: 19.59 ± 2.17 μm; n = 44 neurons; 3 mice; Figures 1N,Q), morphological characteristics such as a round soma, typical bush-like dendrites, and projections to the contralateral MNTB and ipsilateral LSO allowed us to identify some UCN3-positive neurons as bushy cells (Figure 1N; Webster and Trune, 1982). However, a large number of UCN3-positive neurons in the VCN seem to be stellate cells based on their distinct multipolar (stellate) shape (Figure 1N) and by prominent ascending fibers originating from these cells and profusely innervating the ipsilateral DCN (Figures 1H,I). The observation of many UCN3-positive axons leaving the VCN, crossing in the trapezoid body, but not terminating in a calyx of Held suggest a T-stellate cell origin, as the “T” in T-stellate was, indeed, given to underline the fact that very often these neurons send axons across to the other side through the trapezoid (tz) body (Oertel et al., 1990). Other UCN3-positive stellate cell axons connect the VCN to the DCN. These fibers could be of D- or T-stellate cell origin and the neuronal somata of these cell types are found rostrocaudally in the VCN. The innervation pattern of this bundle is similar to that of metabotropic acetylcholine receptor type 2 (AChR M2) recently described (Malfatti et al., 2021). Using an AChR M2 reporter mouse, this bundle was interpreted as originating from VCN T-stellate cells. After entering the DCN, the UCN3-positive fibers span the whole of the deep layers, but without entering the molecular domain, which is characterized by an abundance of cholinergic inputs (Figures 1G,H; Fujino and Oertel, 2001). In conclusion, the UCN3 tdTom reporter shows that in the VCN, a small number of globular bushy cells and a larger number of stellate cells express UCN3. Both, bushy cells and T-stellate cells are found in the MCD of the nucleus and are involved in the faithful transmission of sound information from the cochlear nerve. In contrast to the MCD, the granular cell domain (GCD) does not exhibit any UCN3-positive cells or fibers (Figures 1C,D). Instead, UCN3-positive projections originating from the VCN innervate large parts of the DCN, spanning the deep (polymorphic) and the fusiform layers up to the molecular layer but not trespassing into the GCD nor the molecular layer. The GCD forms a more or less defined area between the magnocellular core of the VCN and the DCN (Mugnaini et al., 1980). The GCD consists mainly of granule cells as well as some other less frequent cell types (unipolar brush cells, chestnut cells, and Golgi cells) (Floris et al., 1994; Weedman et al., 1996; Yaeger and Trussell, 2015). Cells in the GCD are considered non-principal neurons. Although they respond to sound stimulation, especially at high intensities, they mainly integrate sound information with multisensory inputs, rather than to encode straightforward sound properties (Yang et al., 2005; Flores et al., 2015). Much of this multisensory, integrative processing takes place in the molecular layer. The axons of the granule cells populate the molecular layer of the DCN as parallel fibers where they interact with the dendrites of fusiform cells and cartwheel cells. Inputs from non-auditory areas such as the pontine nuclei, the nucleus cuneatus, the vestibular nucleus or the spinal trigeminal nucleus (Zhao et al., 1995; Wright and Ryugo, 1996; Ohlrogge et al., 2001; Ryugo et al., 2003; Zhou and Shore, 2004; Zhan et al., 2006; Zhan and Ryugo, 2007) tend to be positive for the VGluT2, whereas primary auditory inputs are predominantly VGluT1 positive (Zhan and Ryugo, 2007; Zeng et al., 2011).
The expression pattern of CRFR2, differs considerably from the UCN3 expression patterns. In the CN, CRFR2 is expressed almost exclusively in neurons and axonal tracts of the GCD (Figures 1J–M). Cell bodies and axons span the entire rostrocaudal extent of the GCD, including the lamina between VCN and DCN (Figures 1J–M). The morphology of these cells suggests them to be granule cells (Figure 1O). They exhibit the characteristic small size with a mean (±SD) diameter of 9.51 ± 1.02 μm (n = 31 neurons; 3 mice; Figure 1Q). This size is significantly smaller then neurons of the MCD (ANOVA: p ≤ 0.001; Figures 1O,Q). Another prominent feature of granule cells are their axons, which take the characteristic parallel course with respect to the nucleus borders. The CRFR2-positive fibers inhabit the DCN molecular layer, which is also VGluT2-positive and predestines them as granule cells’ parallel fibers (Figures 1J–M; Rubio et al., 2008). In addition to CRFR2 labeling of granule cells and parallel fibers, another population of slightly bigger CRFR2-positive cells were observed at the dorsolateral edge of the DCN (Figures 1J–L,P). These neurons are slightly larger in diameter (mean ± SD: 10.45 ± 0.88 μm; n = 39 neurons; 3 mice) than granule cells (ANOVA: p = 0.036; Figures 1O–Q), but clearly smaller than magnocellular cells (ANOVA: p ≤ 0.001; Figures 1N,Q). Their size and the fact that they are not entirely embedded in the parallel fiber mesh, but rather somewhat underneath suggests them to be small cap cells (Osen, 1969; Cant, 1993; Ryugo, 2008). Small cap cells receive input from medial olivocochlear complex (MOC) neurons in the ventral nucleus of the trapezoid body (VNTB) and project back to the VNTB and also to the MGB (Benson and Brown, 1990; Ryan et al., 1990; Brown et al., 1991; Thompson and Thompson, 1991; Ye et al., 2000; Malmierca et al., 2002; de Venecia et al., 2005; Darrow et al., 2012; Hockley et al., 2021). Both areas, the GCD and the small cap cell location contain CRFR2 positive fibers. In contrast to the UCN3 expression, only a few cells in the magnocellular cores of DCN and VCN were CRFR2 positive. CRFR2-positive cells in in the AVCN are most likely GBCs that form calyces of Held in the lateral part of the MNTB as we will describe in the next paragraph.
Superior Olivary Complex
The mouse SOC is a cluster of interacting nuclei serving essential functions of auditory processing which require both temporal precision and binaural integration. Roughly, the SOC nuclei can be sorted into those involved in sound source localization in the horizontal plane like the medial and lateral nucleus of the trapezoid body (LNTB and MNTB) and the medial superior olive (MSO) and LSO and those that are not involved in sound localization (Grothe and Pecka, 2014). The latter include the periolivary nuclei like the VNTB, the superior paraolivary nucleus (SPN), and the dorsal periolivary nucleus (DPO), whose function seems to vary between species and ranges from efferent feedback to encoding communication sounds (Frank and Goodrich, 2018; Kopp-Scheinpflug et al., 2018).
We previously reported the expression of UCN3 in the auditory brainstem nuclei with respect to the protection of the auditory system from sound over-exposure (Fischl et al., 2019). Surprisingly, this protective function was not accompanied by high numbers of UCN3-positive neuronal cell bodies in the SOC, but rather with an abundance of UCN3 expressing axons and synaptic terminals in this area (Figures 2A,B). A few UCN3-positive somata are found at the dorsomedial edge of the SPN, the VNTB and in a poorly defined area around the dorsolateral edge of LSO possibly corresponding to the DPO (Figures 2B,C). Cells in the VNTB and the DPO areas are part of the MOC system. Distinct UCN3 expression was observed at the calyces of Held (Figures 2B,D). This calyceal UCN3 expression was confined to the calyces contacting MNTB neurons in the lateral subdivision of the MNTB (Figure 2B). According to the tonotopic organization of the MNTB, the neurons that receive the UCN3-positive input are low-CF cells. The UCN3 expression in the calyces of Held corroborate the UCN3-positive AVCN neurons to be identified and GBCs (Felmy and Schneggenburger, 2004). Other, contralaterally originating, ascending fibers terminate in the MSO and VNTB area. In addition, we observed ipsilateral ascending fibers, which contact lateral, low-frequency LSO neurons. The origin of these UCN3-positive axons is most likely in the AVCN bushy cells. Another distinctive bundle of UCN3-expressing fibers terminates at the level of the DPO (Figures 2B,C). Overall, compared to the CN, UCN3 expression in the SOC neurons is scarce. This is reflected by equally sparse CRFR2 expression in the SOC. However, the expression of CRFR2 in lateral calyces of the MNTB (Figure 2F) as well as in fibers in the lateral limb of the LSO (Figure 2E) should be highlighted, because they mirror the UCN3 expression in these nuclei and suggest a possible autocrine regulation of CRFR2 (Figures 2B,E).
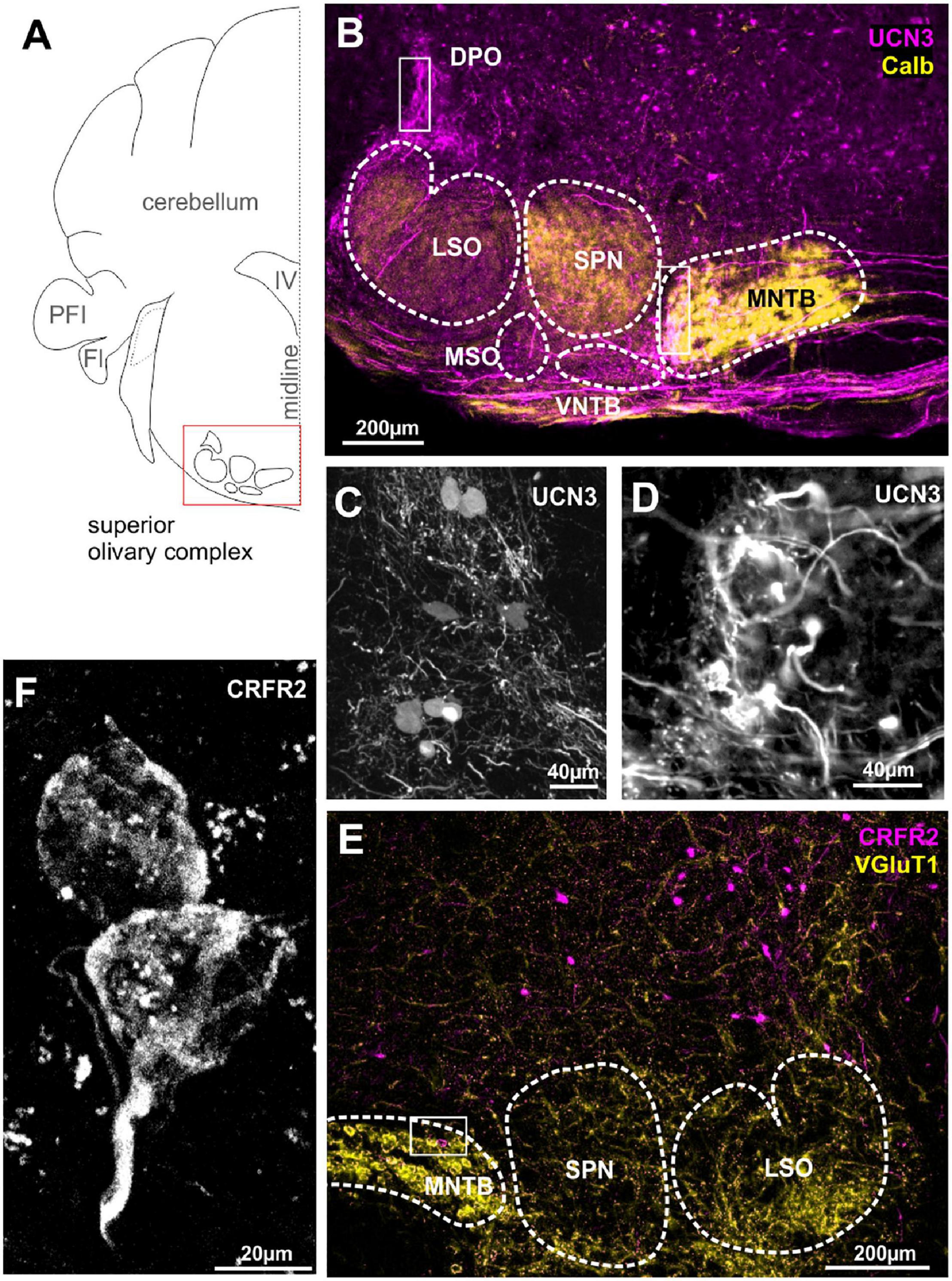
Figure 2. Urocortin 3-positive neurons and fibers dominate over sparse CRFR2 expression in the SOC. (A) Schematic coronal section showing the superior olivary complex (SOC) as the region of interest. PFI, paraflocculus; FI, flocculus; IV, fourth ventricle. (B) Dense UCN3-positive fiber bundles (magenta) enter the SOC from the contralateral side of the brain and innervate the MNTB, SPN, and LSO. Calbindin (yellow) is used as counter stain for SOC nuclei. UCN3-positive neuronal cell bodies are present in the VNTB and in the DPO (white square). Calyces of Held in the lateral, low-frequency MNTB are UCN3-positive (white square in MNTB). The data shown in (A–C) corroborate previously published findings (Fischl et al., 2019), but are shown here for direct comparison with the data on the UCN3 receptor CRFR2. (C) Higher magnification of UCN3-positive DPO neurons and fibers. (D) Higher magnification of UCN3-positive calyx synapses in the lateral MNTB. (E) Sparse CRFR2 expression (magenta) in the SOC. Instead, CRFR2-positive neurons are found in the reticular formation dorsal to the SOC. VGluT1 (yellow) is used a counterstain. (F) CRFR2 expression in the calyces of Held.
Lateral Lemnisci
The cochlear nuclei and the SOC connect to the IC via a fiber bundle that passes along the lateral edge of the brainstem and is termed lateral lemniscus (Figure 3A). Within these fibers, there are three distinct neuronal populations, the dorsal, the intermediate and the ventral nucleus of the lateral lemniscus, DNLL, INLL, and VNLL, respectively (Merchán et al., 1997; Oertel and Wickesberg, 2002; Ito et al., 2011). The nuclei of the LL receive their input predominantly from the contralateral VCN and the ipsilateral SOC (Glendenning et al., 1981). Additionally, the DNLL exchanges reciprocal projections with the contralateral DNLL (Oliver and Shneiderman, 1989). Although there is still much to learn about the LL’s role in auditory processing, a main function seems to be to send a fast feed-forward inhibition as well as a long-lasting inhibition into the IC (Ammer et al., 2015). The INLL receives additional input from the contralateral paralemniscal nucleus (Kelly et al., 2009). The nuclei of the lateral lemniscus (NLLs) seem to provide a major link between the auditory and the stress system. UCN3 is strongly expressed in both, neuronal cell bodies as well as fibers in all three nuclei, the VNLL, the INLL, and the DNLL (Figure 3B). UCN3 expression in VNLL seems to colabel with VGluT1, but less so in the INLL and DNLL (Figure 3B). A group of UCN3-positive neurons medial from the DNLL most likely belong to the brachium (Figures 3A,B).
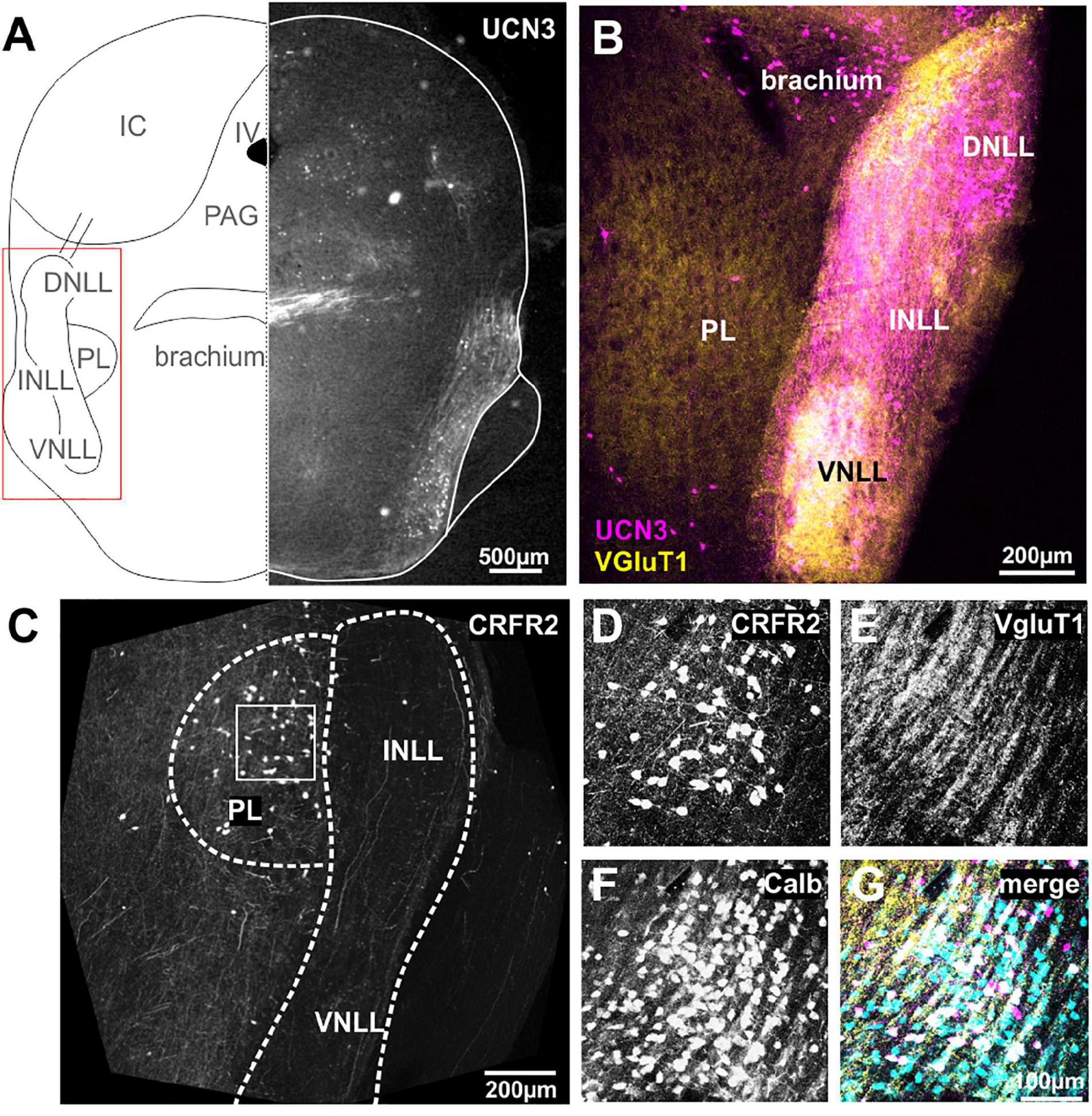
Figure 3. Neurons and fibers of the lateral lemniscus express UCN3 but not CRFR2. (A) Schematic coronal section showing the nuclei of the lateral lemniscus (NLL) as the region of interest. IV, fourth ventricle; PL, paralemniscal nucleus; PAG, periaqueductal gray; IC, inferior colliculus. Left side shows UCN3 expression in the brachium, the intermediate (INLL), and the ventral (VNLL) nuclei of the lateral lemniscus. (B) Higher magnification of the NLL shows UCN3 expression (magenta) in the brachium and all three NLLs. White color in the VNLL suggests co-labeling of UCN3 (magenta) and VGluT1 terminals. The paralemniscal (PL) areas are medial to the INLL are positive for VGluT1, but show no UCN3 expression. (C) Instead the PL shows strong CRFR2 labeling. (D) CRFR2-positive neural cell bodies in the PL. (E) Dense network of VGluT1-positive fibers surrounding PL neurons. (F) PL neurons are calbindin-positive. (G) Co-labeling of CRFR2 (magenta), VGluT1 (yellow), and calbindin (turquoise) suggests that a subpopulation of calbindin-positive neurons also express CRFR2 (white color).
Despite the strong UCN3 expression, the NLLs seem to be devoid of CRFR2 expression. Only very few CRFR2-positive fibers pass through the NLLs (Figure 3C). Instead, CRFR2 is strongly expressed in the paraleminscal nucleus (PL) located medial to the INLL (Figure 3C). The PL is not a principal auditory nucleus, but it receives auditory input and is potentially involved in audio-vocal feedback (Covey, 1993; Metzner, 1993; Feliciano et al., 1995; Hage et al., 2006; Varga et al., 2008). CRFR2-positive PL neurons are embedded in a dense network of VGluT1-positive fibers (Figures 3D,E). Almost all of the UCN3-positive PL neurons are also expressing calbindin (Figures 3F,G).
Inferior Colliculus
The IC is an auditory midbrain structure that receives ascending input from nearly all auditory brainstem nuclei, processes this information into new coding strategies and passes it on to the auditory thalamus. The IC is divided into the central core region (ICc) which harbors principal auditory neurons and into the external shell or cortex region (ICe) which receives multimodal inputs (Winer and Schreiner, 2005). Here, we report an extensive innervation of the ICc by UCN3-positive fibers (Figures 4A,B,D). More specifically, a long-range projection originating from the ipsilateral lateral lemniscus innervates the most lateral and ventral locations in the ICc. These again, as in case of the lateral calyces in the MNTB and the inputs to the lateral limb of the LSO, are areas containing low sound frequency-tuned cells. Strong GlyT2 labeling in the UCN3-positive areas of the ICc (Figure 4C), suggest that the UCN3-positive fibers may originate from the glycinergic VNLL neurons, rather than from the GABAergic DNLL neurons. Because GlyT2 antibodies only label the vesicular transporters in the glycinergic terminals but not along the axons, the UCN3-positive fibers appear purely magenta before entering the IC, but look more whitish upon entering the ICc, suggesting co-labeling with GlyT2 (Figures 4C,D). In contrast to the abundance of UCN3-positive fibers, neuronal cells bodies expressing UCN3 were only rarely observed in the ICc and in the dorsal IC (ICd; Figure 4B).
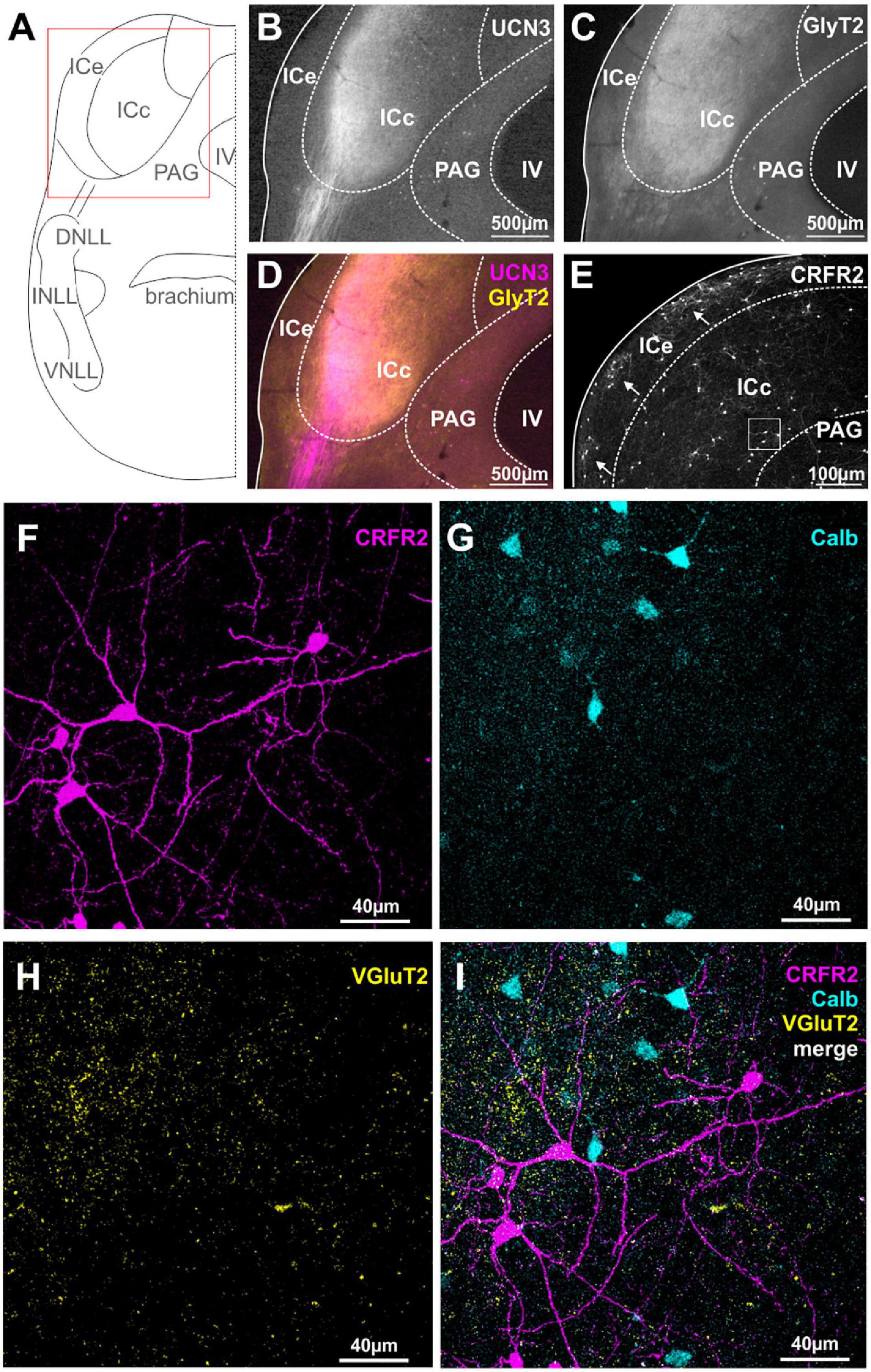
Figure 4. Spatial segregation of UCN3 and CRFR2 expression in the IC. (A) Schematic coronal section showing the inferior colliculus (IC) as the region of interest. IV, fourth ventricle; PAG, periaqueductal gray; NLLs, nuclei of the lateral lemniscus. (B) UCN3-positive fibers innervate the lateral part of the central nucleus of the IC (ICc). (C) GlyT2 expression in the IC. (D) Co-staining of UCN3 (magenta) and GlyT2 (yellow) shows a change in color of UCN3-positive fibers being magenta before entering the IC and appearing more whitish within the IC. UCN3 expression was not observed in the external (ICe) and dorsal (ICd) cortex of the IC. (E) Clusters of CRFR2-positive neuronal cell bodies in the ICe (white arrows). CRFR2-positive cells in ICc (white square) will be shown in higher magnification in (F–I). (F) Higher magnification of CRFR2-positive cell bodies in ICc. (G) Calbindin-positive neurons in ICc. (H) VGluT2 labeling in ICc. (I) Merged image reveals no overlap between CRFR2 (magenta) and calbindin (turquoise) expression.
Similar to our observations on the DCN, the expression patterns of CRFR2 seems to be spatially segregated from the UCN3 expression. CRFR2 is predominantly expressed in cell bodies of ICe and to a much lesser extent in neuronal cell bodies of the ICd and ICc. Interestingly, within the ICe, CRFR2 expression is clustered in circular patches of tissue (Figure 4E) that have been previously described as expressing GAD67 and being the targets of somatosensory and other multisensory inputs to this area (Lesicko et al., 2016). The ICe is known to be an integrative-modulatory area, and the sources of this modulation also include descending projections from principal neurons in higher auditory centers (Adams, 1980). These descending projections, however, tend to segregate neatly outside of the aforementioned patches (Lesicko et al., 2016).
In the ICc and the ICd only few neurons are CRFR2-positive. These are scattered throughout the two subdivisions do not follow a clear pattern of distribution. The CRFR2-positive ICc neurons are characterized by a stellate morphology without a strong cellular orientation axis (Figure 4F). This suggest that these neurons are of the non-flat/disc-shape type that span multiple isofrequency laminae (Meininger et al., 1986). These CRFR2-positive ICc neurons are embedded in a dense network of VGluT2-positive fibers (Figures 4F,H,I). The CRFR2-positive neurons are also distinct from those which express calbindin (Figures 4F,G,I). Both of these observations indicate that CRFR2-positive ICc neurons are different from those ICc neurons that receive the main auditory input mediating sound localization information from the lower brainstem (Takahashi et al., 1987).
The expression patterns of UCN3 and CRFR2 in the IC lack obvious synaptic contacts between the ligand- and the receptor-expressing neurons, strengthening the hypothesis of non-synaptic means of volume transmission in this system.
Medial Geniculate Body
The MGB is the auditory part of the thalamus and is composed of three main subdivisions, dorsal (MGBd), ventral (MGBv), and MGBm (Anderson et al., 2009). These subdivisions give rise to two major information streams to the cortex: the lemniscal stream (through MGBv) conveying ascending auditory information from the IC to Au1 (Winer, 1992; Anderson and Linden, 2011), and the non-lemniscal (through MGBd and MGBm) stream, conveying multimodal, more context-dependent information to secondary auditory cortex areas (Winer and Schreiner, 2005; Anderson et al., 2009). In addition, there are other parageniculate nuclei: the suprageniculate thalamic nucleus (SG), the posterior intralaminar thalamic nucleus (PIN), and the posterior limitans thalamic nucleus, which is also known as pretectothalamic lamina (PTL) (Anderson and Linden, 2011; Marquez-Legorreta et al., 2016).
Many UCN3-positive neuronal cell bodies were observed in the non-principal auditory areas like the MGBm, PIN, and PTL (Figures 5A,B). In addition, there are UCN3-positive fibers in the MGBm as well as a thin layer, which might be the marginal zone of the medial geniculate (Figures 5A,B). Counterstaining with parvalbumin, which in the brainstem is generally considered as an indicator for principal auditory neurons, showed only little overlap with UCN3-positive neurons in the MGBm (Figures 5B,D,E). However, in the MGB, many of the neurons in the MGBd and PIN are calbindin positive (Figures 5B,C,E), even though these areas are not considered to contain auditory principal neurons (Cruikshank et al., 2001; Lu et al., 2009; Marquez-Legorreta et al., 2016). This suggests that calbindin and parvalbumin might characterize different neurons in the thalamus compared to auditory brainstem and midbrain.
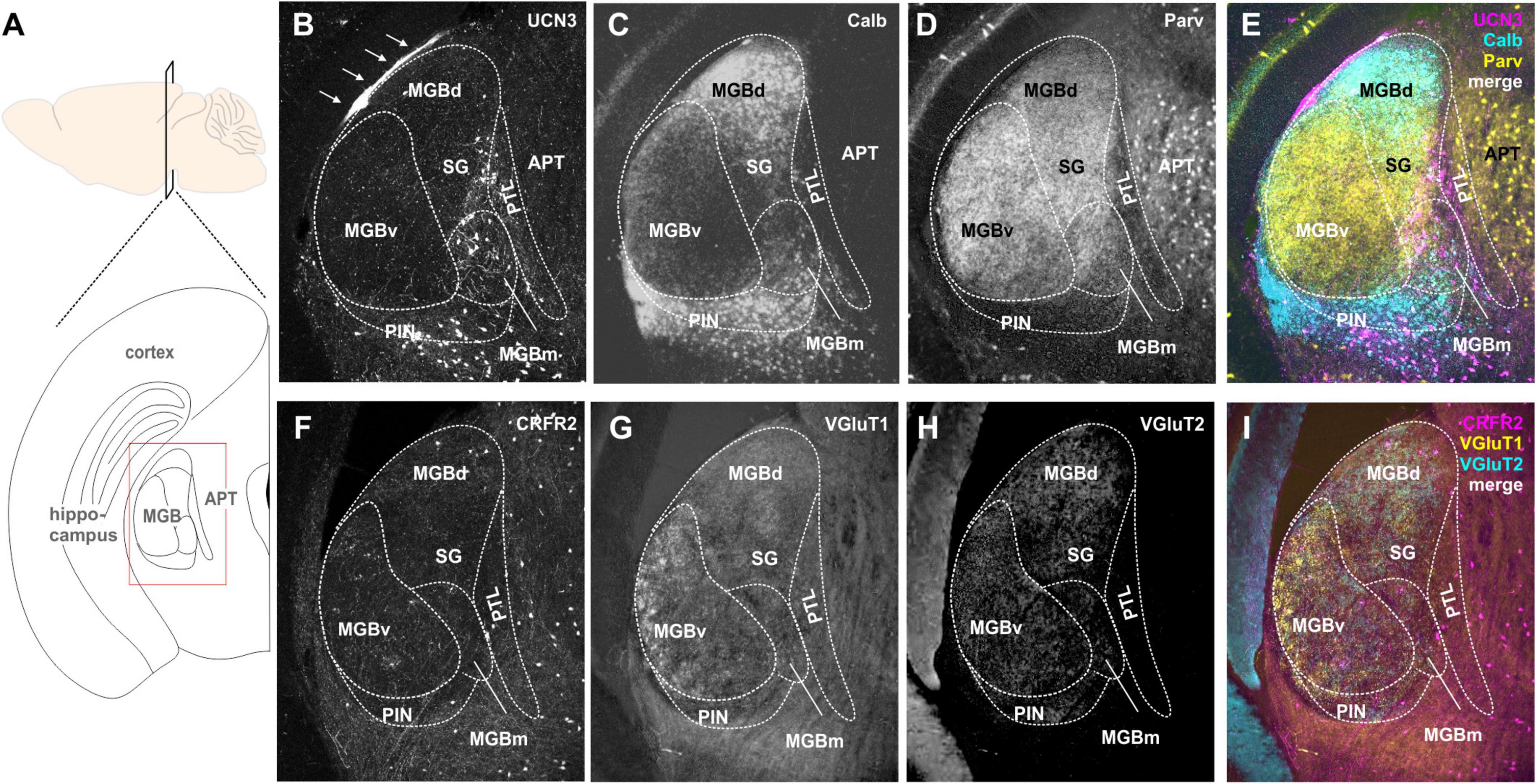
Figure 5. Expression of UCN3 and CRFR2 in the medial geniculate body (MGB) and the pretectal thalamic transition zones. (A) Sagital view of the brain indicating the level of the schematic coronal section (below) showing the medial geniculate body (MGB) as the region of interest. APT. anterior pretectal nucleus. (B) UCN3-expression in the medial subdivision of the MGB (MGBm), in the posterior intralaminar thalamic nucleus (PIN), and in the pretectal thalamic lamina (PTL). The thin layer of UCN3-positive fibers might occur in the marginal zone of the medial geniculate (white arrows). Calbindin (C) and parvalbumin (D) expression in the MGB were used as counterstain to identify substructures of the MGB. (E) Merge shows parvalbumin (yellow) expression in ventral MGB (MGBv), the suprageniculate thalamic nucleus (SG), and APT. Calbindin is mainly expressed in dorsal MGB (MGBd) and PIN. Neither parvalbumin nor calbindin show strong expression in the UCN3-positive neurons in MGBm and PIN. (F) Few CRFR2-positive neuronal cell bodies are present in the MGBm and the PTL. A network of CRFR2-positive fibers seems to surround the MGB without entering its core. (G–I) VGlutT1 (G) and VGluT2 (H) were used as counterstain.
CRFR2-expression in the MGB was observed mostly in fibers and terminals (Figures 5F–I). These seemed to extent over several areas, but were especially prominent in the MGBm, PIN, and PTL. The origins of these CRFR2-positive fibers are most likely the multimodal domains of the IC, the ICe, and ICd. Additionally, a few CRFR2-positive neuronal cell bodies were present in the area of the PTL. However, even though the PTL also contained UCN3-positive neurons, at this point it is unclear if UCN3 and CRFR2 are expressed in the same cells. The lack of strong CRFR2 expression suggests that the receptor is expressed at a distant location like the amygdala where the UCN3-positive MGBm neurons project to. This would complete the pathway for auditory fear conditioning, hence providing an interesting link between auditory and stress system (Linke et al., 2000).
Discussion
In this study, we characterized the expression patterns of the stress peptide UCN3 and its receptor CRFR2 in the mouse auditory pathway and found a strong presence in most subcortical structures. The combination of ligand and receptor expression allowed forming hypotheses about possible signaling mechanisms, which can be tested in future physiological experiments. In most auditory areas, a spatial segregation between UCN3-expression in auditory structures containing auditory principal neurons and CRFR2-expression in multisensory areas was observed (Table 1). This study introduces stress peptides as potential modulators of central auditory function.
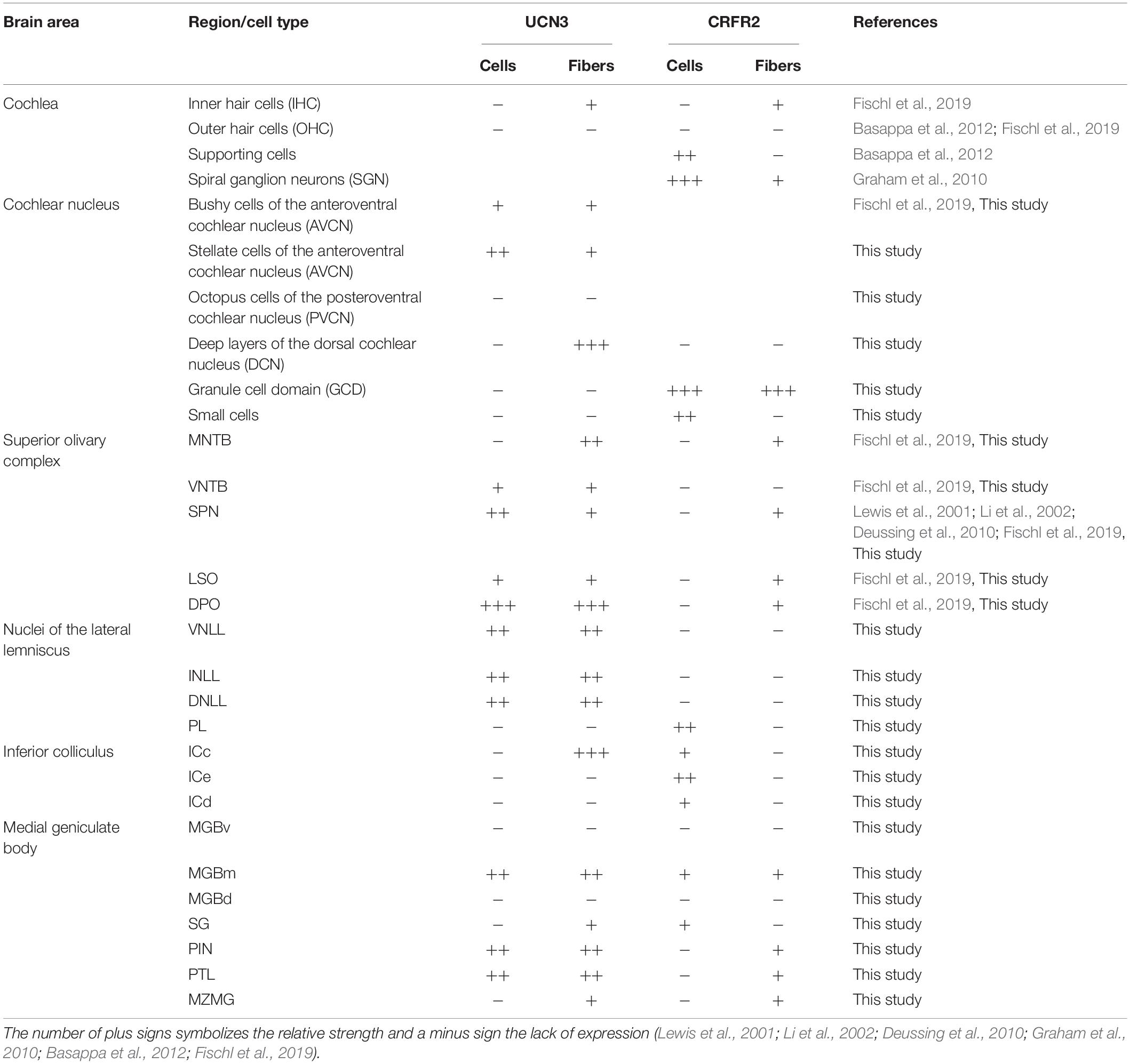
Table 1. Distribution of UCN3- and CRFR2-positive neuronal cell bodies, fibers, and terminals in the auditory pathway.
Benefits and Shortcomings of Using Reporter Mouse Lines to Study Protein Expression
The expression of the fluorescent protein starts whenever the gene of interest turns on. It then produces the fluorescent protein, which will stay in the neurons. Therefore, the most common criticism of reporter mouse lines is the question of when in the lifetime of the animal the protein of interest is expressed. Consequently, UCN3 might not be expressed constitutively in all the auditory nuclei that we described in this study and it is possible that some of these expression patterns are developmentally regulated or are subject to specific stressful events. Nevertheless, utilizing these reporter models allowed us to observe the entire expression patterns that could occur for example in different behaviorally relevant situations or at different time points in an animal’s life. Since the influence of systemic stressors on auditory processing is not yet understood, knowing the auditory areas or cell types that can potentially be modulated by stress signaling is an important first step.
A major advantage of using reporter mouse lines for stress peptide signaling is that the expression can be visualized even when the target protein is very small and is expressed at very low concentrations. This aspect is crucial because neuropeptides are notoriously produced in very small quantities, mainly because they bind to G-protein coupled receptors (GPCRs), which have nanomolar sensitivities. This is in stark contrast to most ionotropic receptors, which have micromolar sensitivities (van den Pol, 2012). The small quantity and the small size of the peptide itself (38 amino acids for UCN3) makes detection with conventional immunohistochemistry particularly challenging. In addition, CRFR2 has some quite unusual characteristics that make it also difficult to detect via antibody binding. Specifically, CRFR2 contains a non-cleavable pseudosignaling peptide (PSP) attached to its N-terminal (Schulz et al., 2010; Teichmann et al., 2012). The PSP is absent from the other receptor type, CRFR1, and it confers some unique physiological traits of CRFR2 expression. In fact, PSP has been shown to anchor CRFR2 to the endoplasmatic reticulum and prevent its expression at the level of the membrane. This could potentially also impede the detection through receptor-mediated autoradiography, because the receptor might be inaccessible for radio ligand binding if the proper physiological triggers for membrane expression have not taken place. Tethering of the receptor intracellularly might also mask antigens for immunohistochemistry and hence much information could be lost by this technique. Besides, binding of the radiolabeled ligand has to compete with the endogenous ligand binding which might already be occupying the site.
Fluorescent in situ hybridization (FISH) and other genetic-based methods are also inferior to the use of reporter mice, because they can provide only a snapshot in time of the target’s expression that portrays only the genetic material actively being translated and therefore is highly dependent on any stress-related conditions of the animal prior to the sacrifice. Initial reports utilized FISH to study the general expression patterns of UCN3 and CRFR2 in the mammalian brain included information on the auditory system (Lewis et al., 2001; Li et al., 2002).
Receptor-Ligand Mismatch
For decades, neuroscientists have reported a rather unexpected phenomenon regarding neurotransmitters and, even more so, neuropeptides: an apparent lack of direct synaptic contact between neurons expressing a receptor and those expressing its high-affinity ligand in certain brain areas and cell types (Herkenham, 1987). An observation that seems to contradict the dogma of synaptic transmission. Even if acknowledged, this phenomenon has not spurred extensive investigation, with a few notable exceptions (Agnati et al., 1995; Ludwig and Leng, 2006). Very often, this intriguing finding has been attributed to technical limitations as explained in the previous paragraph. However, other possible solutions to this mystery have been explored and even proved for some neuropeptides (Liu et al., 1994; Liu and Sandkuhler, 1995; Brown et al., 2008; Ha et al., 2013). First of all, one has to consider that neuropeptides, in contrast to classical neurotransmitters, could reach their target receptor over very long distances through blood circulation. That is the case for example for leptin, ghrelin, and insulin, which are released at the level of the gastrointestinal system, but also affect the central nervous system (Rhea et al., 2018; Stengel and Tache, 2018). Not all neuropeptides can cross the blood–brain barrier; however, experimental evidence shows generalized effects of UCN3 after central injections, supporting a neuroendocrine option for UCN3 (Sharpe and Phillips, 2009; Yeh et al., 2016).
Besides the long-range endocrine signaling, some neuropeptides such as galanin (Freund-Mercier and Stoeckel, 1995) and oxytocin can also be released by dendrites and neuronal somata (Vila-Porcile et al., 2009). In invertebrates, where mechanisms of neuropeptide release have been studied more intensely, it has been shown that neuropeptides are shuffled anterogradely and retrogradely within the same neuron using different molecular motors (Barkus et al., 2008; Wong et al., 2012).
Finally, the lack of synaptic re-uptake mechanisms and the presence of extracellular peptidases are strong indicators that volume transmission plays a major role in many instances. However, due to the presence of the extracellular peptidases, most of the effects remain local and limited in time (Defea et al., 2000). This is emphasized by the fact that neuropeptides and their receptors have an overall discrete expression in certain areas. A more widespread diffusion would defeat the purpose of this specialization.
Possible Binding of CRFR2 by Other Ligands
The possibility, that in some areas UCN3 might not be the only ligand for CRFR2 has to be taken into account when making functional considerations. UCN2 can also bind to CRFR2 with high affinity, UCN1 can bind with moderate and CRF with low affinity. To date, there is no report about the presence of UCN2 in the auditory system. However, CRF positive neuronal cell bodies are present in the DCN, lateral MTNB, ICe, DNLL, VNLL, and MGB (Imaki et al., 1991). Although CRF has a very low affinity for CRFR2 and would have to be released at extremely high concentrations to sufficiently occupy the receptor, it cannot be excluded that CRF might also act on CRFR2 receptors in the auditory pathway. Despite possible binding of CRFR2 by UCN2 or CRF, UCN3 is still the most likely ligand (Li et al., 2002). In contrast to the other ligands, UCN3 binds exclusively to CRFR2, and so its sole purpose is to be released and to bind this receptor. Hence, the spatial segregation between presynaptic UCN3 and postsynaptic CRFR2 expression that we observed in multiple auditory areas, could not simply be explained by postulating that in these areas CRF rather than UCN3 might be the main ligand. Moreover, it suggests that if UCN3 is expressed it has to bind to its exclusive receptor CRFR2 to have a functional effect even if it involves volume transmission.
Volume transmission might be corroborated by the fact that another ligand-receptor pairs of stress peptides also shows a segregation of ligand and receptor. In the IC, the ligand CRF is expressed in the integrative cells of the external cortex (Imaki et al., 1991), whereas its receptor, CRFR1 is expressed in principal auditory neurons of the central nucleus (Justice et al., 2008). A note of caution has to be given though, that the latter publication was not specifically focused on the auditory system and the identity of the cell types expressing CRFR1 was not clearly established.
Nevertheless, the general presence of other ligands gives an interesting perspective on how this system could be extremely well refined for balanced modulation. For example, certain stressors might be too mild to release a large enough quantity of CRF to compete with UCN3 for CRFR2 binding, which would make the modulation modality- and/or intensity-dependent. Alternatively, CRF might be released by a different population of neurons than UCN3, which is mostly released by neurons tuned to low sound frequencies.
Functional Implications of Urocortin 3 and CRFR2 Expression in the Auditory System
The most intriguing finding of our study is that the neuromodulatory ligand UCN3 tends to be expressed in auditory principal neurons, whereas CRFR2 labeling is mostly found in non-principal, multimodal neurons. Such a distribution is unusual, because typically the auditory principal neurons are the target of neuromodulatory inputs rather than being their source (Schofield et al., 2011; Sizemore et al., 2020). An obvious example is the CN where UCN3 is expressed in the magnocellular core of both VCN and DCN while CRFR2 is mostly found in the granular cell domain and the parallel fibers. Similarly, in the IC, UCN3 positive fibers are found within the central nucleus, while CRFR2 is expressed in the external cortex of the IC.
Normally, we would consider modulation as coming from other systems, that are either cross-modal or are devoted to modulation itself like the reticular formation, both of which would then alter the incoming auditory information. Indeed, the presence and function of modulatory inputs to auditory structures have been described in many studies (Ryugo et al., 2003). For example, a large body of work on the DCN revealed how somatosensory inputs from head and neck can suppress self-generated sound perception (Shore, 2005; Koehler and Shore, 2013; Singla et al., 2017). A lot of clarification came from identifying the anatomical origin of these modulatory fibers and in-depth mechanistic explanations (Trussell and Oertel, 2018). More so, clinical evidence from the treatment of certain types of tinnitus utilizing cranio-cervical manipulations provided additional strength to these data (Levine, 1999).
However, the occurrence of reverse patterns of modulation as we describe here for the UCN3–CRFR2 system is novel and its functional significance is up for discovery. Although a few cross-modal feedback projections from auditory structures might form part of the UCN3–CRFR2 system, to our knowledge, the finding of an on-site modulation of stressful situations has not yet been explored in the central auditory pathway.
The interesting scenario here is that sound-driven release of the ligand might affect the cells responsible for receiving external modulation and set them up for specific types of firing. For instance, release of UCN3 from fibers reaching the central nucleus of the IC might bypass the principal auditory cells in ICc and instead directly modulate the activity of the multimodal cells in the ICe via volume transmission. The timescale of volume transmission is certainly an interesting aspect with regard to the fast signal processing of primary ascending auditory information. Non-gaseous neuromodulators including UCN3 are long-lived and their lifetime is typically determined by the tissue-specific degradation processes (for review see, Russo, 2017). Although, to our knowledge, the lifetime of UCN3 in the brain is not yet known, the lifetime of other neuropeptides such as oxytocin and vasopressin in the cerebrospinal fluid is reported to be up to 20 min. These 20 min together with the downstream G-protein coupled signaling cascade of UCN3 and most other neuropeptides suggest the action of UCN3 to be slow compared to the primary auditory signal processing. However, since processing of auditory information takes place at different time scales from microseconds to many seconds or even hours, UCN3 signaling is just in time to modulate processes involved in temporal abstraction rather than temporal resolution or temporal integration (Kopp-Scheinpflug and Linden, 2021). Such a setting could maintain faithfulness of direct ascending auditory transmission on one hand, while at the same time interfering with the modulatory effects of the ICe cells and their inputs to non-auditory areas.
Another interesting finding is that CRFR2 expression in the SOC seems less abundant than that of UCN3. Instead, CRFR2 expression in surrounding non-auditory areas of the cranial nerve and nuclei of the reticular formation is very strong, suggesting that one purpose of UCN3-release by SOC neurons might be to affect surrounding non-auditory structures rather than afferent auditory principal neurons. With respect to the SOC as an evolutionary highly conserved collection of nuclei that are essential for the execution of precise and survival-promoting encoding of sound information, too much modulatory impact could even be detrimental in the SOC. An exception of the UCN3 positive structures in the SOC are the calyces of Held innervating lateral, low-frequency tuned MNTB neurons. Here, we found an area in which an autocrine modulation could take place, since these lateral calyces of Held express both the receptor and the ligand. It is still unknown, whether the same calyces express both ligand and receptor; a question that will have to be answered by single cell physiology or through the generation of a double reporter mouse line. In this case, the autocrine route seems to be the prevalent one, even if it does not exclude that volume transmission is also happening. In fact, it is known that neuropeptides can travel up to hundreds of micrometers to reach their receptor (Fuxe et al., 2010); yet the concentration that actually reaches the furthest targets declines linearly with the amount to extracellular peptidases being expressed along the way. To date, information on the presence and localization of extracellular peptidases is still lacking in these auditory areas. Hence, it seems most reasonable that the CRFR2 expressing calyces in the lateral MNTB would receive the majority of UCN3 released within the same area compared to other CRFR2 expressing cells more at a distance. Similar possible combinations of autocrine and paracrine signaling has been suggested for CRF-CRFR1 signaling between cochlear supporting cells (Graham et al., 2011).
The strong expression of UCN3 in VNTB neurons is most likely due to its contribution in the efferent feedback system of the medial olivocochlear complex (MOC). MOC neurons project to the outer hair cells of the cochlea and protect these during damaging sound intensities. However, prior research on the contribution of UCN3 during sound over exposure did not show an effect on cochlear outer hair cells (Fischl et al., 2019). Efferent projections of MOC neurons also send collaterals into the CN targeting the small cell cap. Here, we showed that the small cells express CRFR2 and therefore qualify as the MOC-UCN3-target. Indeed, a very recent study investigating the physiology of CN small cells suggest a special role for these cells in processing communication sounds, a function that is most certainly subject to stress and emotional modulation (Hockley et al., 2021).
Conclusion
With this work, we aim to highlight the presence of the UCN3–CRFR2 system as so far unexplored neuromodulators in the central auditory pathway. First, the expression of both factors is widespread in subcortical auditory nuclei. Second, the ligand-receptor expression patterns suggest unusual forms of neurotransmission such as local or long distance volume transmission and possibly even autocrine regulation. Third, an interesting pattern of segregation between the ligand being expressed in auditory principal cells and the receptor being expressed in non-principal neurons implies a stress-dependent modulation of the canonical modulators. These results open up a new field of research, investigating which stressors could be activated under what circumstances and how these stressors influence central auditory processing.
Data Availability Statement
The raw data supporting the conclusions of this article will be made available by the authors, without undue reservation.
Ethics Statement
The animal study was reviewed and approved by the Bavarian district government.
Author Contributions
All authors contributed to the conception and design of the study and data collection. SP performed experiments and wrote the first draft of the manuscript. All authors contributed to manuscript revision, and read and approved the submitted version.
Conflict of Interest
The authors declare that the research was conducted in the absence of any commercial or financial relationships that could be construed as a potential conflict of interest.
Publisher’s Note
All claims expressed in this article are solely those of the authors and do not necessarily represent those of their affiliated organizations, or those of the publisher, the editors and the reviewers. Any product that may be evaluated in this article, or claim that may be made by its manufacturer, is not guaranteed or endorsed by the publisher.
Funding
This research was funded by the DFG (SFB870 A-10 and GS-82 Graduate School of Systemic Neurosciences GSNLMU), by the German Ministry of Science and Education (IMADAPT, FKZ: 01KU1901), and by the Marie Skłodowska-Curie innovative training network PurinesDX.
Acknowledgments
The authors gratefully acknowledge Benedikt Grothe for constant support, Lucy Anderson for helpful comments on the manuscript, and Olga Alexandrova and Hilde Wohlfrom for expert technical support throughout the process.
References
Adams, J. C. (1980). Crossed and descending projections to the inferior colliculus. Neurosci. Lett. 19, 1–5. doi: 10.1016/0304-3940(80)90246-3
Agnati, L. F., Zoli, M., Stromberg, I., and Fuxe, K. (1995). Intercellular communication in the brain: wiring versus volume transmission. Neuroscience 69, 711–726. doi: 10.1016/0306-4522(95)00308-6
Ammer, J. J., Siveke, I., and Felmy, F. (2015). Activity-dependent transmission and integration control the timescales of auditory processing at an inhibitory synapse. Curr. Biol. 25, 1562–1572. doi: 10.1016/j.cub.2015.04.026
Anderson, L. A., Christianson, G. B., and Linden, J. F. (2009). Stimulus-specific adaptation occurs in the auditory thalamus. J. Neurosci. 29, 7359–7363. doi: 10.1523/JNEUROSCI.0793-09.2009
Anderson, L. A., and Linden, J. F. (2011). Physiological differences between histologically defined subdivisions in the mouse auditory thalamus. Hear. Res. 274, 48–60. doi: 10.1016/j.heares.2010.12.016
Aston-Jones, G., and Cohen, J. D. (2005). An integrative theory of locus coeruleus-norepinephrine function: adaptive gain and optimal performance. Annu. Rev. Neurosci. 28, 403–450. doi: 10.1146/annurev.neuro.28.061604.135709
Barkus, R. V., Klyachko, O., Horiuchi, D., Dickson, B. J., and Saxton, W. M. (2008). Identification of an axonal kinesin-3 motor for fast anterograde vesicle transport that facilitates retrograde transport of neuropeptides. Mol. Biol. Cell 19, 274–283. doi: 10.1091/mbc.e07-03-0261
Basappa, J., Graham, C. E., Turcan, S., and Vetter, D. E. (2012). The cochlea as an independent neuroendocrine organ: expression and possible roles of a local hypothalamic-pituitary-adrenal axis-equivalent signaling system. Hear. Res. 288, 3–18. doi: 10.1016/j.heares.2012.03.007
Bazwinsky, I., Hartig, W., and Rubsamen, R. (2008). Characterization of cochlear nucleus principal cells of Meriones unguiculatus and Monodelphis domestica by use of calcium-binding protein immunolabeling. J. Chem. Neuroanat. 35, 158–174. doi: 10.1016/j.jchemneu.2007.10.003
Behler, O., and Uppenkamp, S. (2020). Activation in human auditory cortex in relation to the loudness and unpleasantness of low-frequency and infrasound stimuli. PLoS One 15:e0229088. doi: 10.1371/journal.pone.0229088
Benson, T. E., and Brown, M. C. (1990). Synapses formed by olivocochlear axon branches in the mouse cochlear nucleus. J. Comp. Neurol. 295, 52–70. doi: 10.1002/cne.902950106
Brown, C. H., Ruan, M., Scott, V., Tobin, V. A., and Ludwig, M. (2008). Multi-factorial somato-dendritic regulation of phasic spike discharge in vasopressin neurons. Prog. Brain Res. 170, 219–228. doi: 10.1016/S0079-6123(08)00419-6
Brown, M. C., Pierce, S., and Berglund, A. M. (1991). Cochlear-nucleus branches of thick (medial) olivocochlear fibers in the mouse: a cochleotopic projection. J. Comp. Neurol. 303, 300–315. doi: 10.1002/cne.903030211
Cant, N. (1993). “The synaptic organization of the ventral cochlear nucleus of the cat: the peripheral cap of small cells,” in The Mammalian Cochlear Nuclei, eds M. A. Merchàn, J. M. Juiz, D. A. Godfrey, and E. Mugnaini (Boston: Springer), 91–105.
Chang, C. P., Pearse, R. V. II, O’Connell, S., and Rosenfeld, M. G. (1993). Identification of a seven transmembrane helix receptor for corticotropin-releasing factor and sauvagine in mammalian brain. Neuron 11, 1187–1195. doi: 10.1016/0896-6273(93)90230-o
Charmandari, E., Tsigos, C., and Chrousos, G. (2005). Endocrinology of the stress response. Annu. Rev. Physiol. 67, 259–284. doi: 10.1146/annurev.physiol.67.040403.120816
Chen, R., Lewis, K. A., Perrin, M. H., and Vale, W. W. (1993). Expression cloning of a human corticotropin-releasing-factor receptor. Proc. Natl. Acad. Sci. USA 90, 8967–8971. doi: 10.1073/pnas.90.19.8967
Clifford, G., Dalgleish, T., and Hitchcock, C. (2018). Prevalence of auditory pseudohallucinations in adult survivors of physical and sexual trauma with chronic post-traumatic stress disorder (PTSD). Behav. Res. Ther. 111, 113–118. doi: 10.1016/j.brat.2018.10.015
Covey, E. (1993). Response properties of single units in the dorsal nucleus of the lateral lemniscus and paralemniscal zone of an echolocating bat. J. Neurophysiol. 69, 842–859. doi: 10.1152/jn.1993.69.3.842
Cruikshank, S. J., Killackey, H. P., and Metherate, R. (2001). Parvalbumin and calbindin are differentially distributed within primary and secondary subregions of the mouse auditory forebrain. Neuroscience 105, 553–569. doi: 10.1016/s0306-4522(01)00226-3
Curtis, A. L., Leiser, S. C., Snyder, K., and Valentino, R. J. (2012). Predator stress engages corticotropin-releasing factor and opioid systems to alter the operating mode of locus coeruleus norepinephrine neurons. Neuropharmacology 62, 1737–1745. doi: 10.1016/j.neuropharm.2011.11.020
Darrow, K. N., Benson, T. E., and Brown, M. C. (2012). Planar multipolar cells in the cochlear nucleus project to medial olivocochlear neurons in mouse. J. Comp. Neurol. 520, 1365–1375. doi: 10.1002/cne.22797
Davis, M. (1984). “The mammalian startle response,” in Neural mechanisms of startle behavior, ed. R. C. Eaton (New York, NY: Plenum Press), 287–351.
de Venecia, R. K., Liberman, M. C., Guinan, J. J. Jr., and Brown, M. C. (2005). Medial olivocochlear reflex interneurons are located in the posteroventral cochlear nucleus: a kainic acid lesion study in guinea pigs. J. Comp. Neurol. 487, 345–360. doi: 10.1002/cne.20550
Defea, K., Schmidlin, F., Dery, O., Grady, E. F., and Bunnett, N. W. (2000). Mechanisms of initiation and termination of signalling by neuropeptide receptors: a comparison with the proteinase-activated receptors. Biochem. Soc. Trans. 28, 419–426.
Deussing, J. M., Breu, J., Kuhne, C., Kallnik, M., Bunck, M., Glasl, L., et al. (2010). Urocortin 3 modulates social discrimination abilities via corticotropin-releasing hormone receptor type 2. J. Neurosci. 30, 9103–9116. doi: 10.1523/JNEUROSCI.1049-10.2010
Deussing, J. M., and Chen, A. (2018). The Corticotropin-Releasing Factor Family: Physiology of the Stress Response. Physiol. Rev. 98, 2225–2286. doi: 10.1152/physrev.00042.2017
Feliciano, M., Saldana, E., and Mugnaini, D. (1995). Direct projections from the rat primary auditory neocortex to nucleus sagulum, paralemniscal regions, superior olivary complex and cochlear nuclei. Aud. Neurosci. 1995, 287–308.
Felmy, F., and Schneggenburger, R. (2004). Developmental expression of the Ca2+-binding proteins calretinin and parvalbumin at the calyx of Held of rats and mice. Eur. J. Neurosci. 20, 1473–1482. doi: 10.1111/j.1460-9568.2004.03604.x
Fischl, M. J., Ueberfuhr, M. A., Drexl, M., Pagella, S., Sinclair, J. L., Alexandrova, O., et al. (2019). Urocortin 3 signalling in the auditory brainstem aids recovery of hearing after reversible noise-induced threshold shift. J. Physiol. 597, 4341–4355. doi: 10.1113/JP278132
Flores, E. N., Duggan, A., Madathany, T., Hogan, A. K., Marquez, F. G., Kumar, G., et al. (2015). A non-canonical pathway from cochlea to brain signals tissue-damaging noise. Curr. Biol. 25, 606–612. doi: 10.1016/j.cub.2015.01.009
Floris, A., Dino, M., Jacobowitz, D. M., and Mugnaini, E. (1994). The unipolar brush cells of the rat cerebellar cortex and cochlear nucleus are calretinin-positive: a study by light and electron microscopic immunocytochemistry. Anat. Embryol. 189, 495–520. doi: 10.1007/BF00186824
Frank, M. M., and Goodrich, L. V. (2018). Talking back: Development of the olivocochlear efferent system. Wiley Interdiscip. Rev. Dev. Biol. 7:e324. doi: 10.1002/wdev.324
Freund-Mercier, M. J., and Stoeckel, M. E. (1995). Somatodendritic autoreceptors on oxytocin neurones. Adv. Exp. Med. Biol. 395, 185–194.
Fujino, K., and Oertel, D. (2001). Cholinergic modulation of stellate cells in the mammalian ventral cochlear nucleus. J. Neurosci. 21, 7372–7383.
Fuxe, K., Dahlstrom, A. B., Jonsson, G., Marcellino, D., Guescini, M., Dam, M., et al. (2010). The discovery of central monoamine neurons gave volume transmission to the wired brain. Prog. Neurobiol. 90, 82–100. doi: 10.1016/j.pneurobio.2009.10.012
Glendenning, K. K., Brunso-Bechtold, J. K., Thompson, G. C., and Masterton, R. B. (1981). Ascending auditory afferents to the nuclei of the lateral lemniscus. J. Comp. Neurol. 197, 673–703. doi: 10.1002/cne.901970409
Graham, C. E., Basappa, J., Turcan, S., and Vetter, D. E. (2011). The cochlear CRF signaling systems and their mechanisms of action in modulating cochlear sensitivity and protection against trauma. Mol. Neurobiol. 44, 383–406. doi: 10.1007/s12035-011-8203-3
Graham, C. E., Basappa, J., and Vetter, D. E. (2010). A corticotropin-releasing factor system expressed in the cochlea modulates hearing sensitivity and protects against noise-induced hearing loss. Neurobiol. Dis. 38, 246–258. doi: 10.1016/j.nbd.2010.01.014
Grothe, B., and Pecka, M. (2014). The natural history of sound localization in mammals–a story of neuronal inhibition. Front. Neural. Circuits 8:116. doi: 10.3389/fncir.2014.00116
Guthrie, R. M., and Bryant, R. A. (2005). Auditory startle response in firefighters before and after trauma exposure. Am. J. Psychiatry 162, 283–290. doi: 10.1176/appi.ajp.162.2.283
Ha, S., Baver, S., Huo, L., Gata, A., Hairston, J., Huntoon, N., et al. (2013). Somato-dendritic localization and signaling by leptin receptors in hypothalamic POMC and AgRP neurons. PLoS One 8:e77622. doi: 10.1371/journal.pone.0077622
Hage, S. R., Jurgens, U., and Ehret, G. (2006). Audio-vocal interaction in the pontine brainstem during self-initiated vocalization in the squirrel monkey. Eur. J. Neurosci. 23, 3297–3308. doi: 10.1111/j.1460-9568.2006.04835.x
Harrison, J. M., and Irving, R. (1965). The Anterior Ventral Cochlear Nucleus. J. Comp. Neurol. 124, 15–41. doi: 10.1002/cne.901240103
Hegewald, J., Schubert, M., Freiberg, A., Romero Starke, K., Augustin, F., Riedel-Heller, S. G., et al. (2020). Traffic noise and mental health: a systematic review and meta-analysis. Int. J. Environ. Res. Public Health 17, 17176175. doi: 10.3390/ijerph17176175
Henckens, M., Printz, Y., Shamgar, U., Dine, J., Lebow, M., Drori, Y., et al. (2017). CRF receptor type 2 neurons in the posterior bed nucleus of the stria terminalis critically contribute to stress recovery. Mol. Psychiatry 22, 1691–1700. doi: 10.1038/mp.2016.133
Herkenham, M. (1987). Mismatches between neurotransmitter and receptor localizations in brain: observations and implications. Neuroscience 23, 1–38. doi: 10.1016/0306-4522(87)90268-5
Hockley, A., Wu, C., and Shore, S. (2021). Cochlear nucleus small cells use olivocochlear collaterals to encode sounds in noise. bioRxiv 2021:444983.
Horner, K. C. (2003). The emotional ear in stress. Neurosci. Biobehav. Rev. 27, 437–446. doi: 10.1016/s0149-7634(03)00071-x
Hoskin, R., Hunter, M. D., and Woodruff, P. W. (2014). The effect of psychological stress and expectation on auditory perception: A signal detection analysis. Br. J. Psychol. 105, 524–546. doi: 10.1111/bjop.12048
Hurley, L. M., and Hall, I. C. (2011). Context-dependent modulation of auditory processing by serotonin. Hear. Res. 279, 74–84. doi: 10.1016/j.heares.2010.12.015
Imaki, J., Imaki, T., Vale, W., and Sawchenko, P. E. (1991). Distribution of corticotropin-releasing factor mRNA and immunoreactivity in the central auditory system of the rat. Brain Res. 547, 28–36. doi: 10.1016/0006-8993(91)90571-c
Ito, T., Bishop, D. C., and Oliver, D. L. (2011). Expression of glutamate and inhibitory amino acid vesicular transporters in the rodent auditory brainstem. J. Comp. Neurol. 519, 316–340. doi: 10.1002/cne.22521
Justice, N. J., Yuan, Z. F., Sawchenko, P. E., and Vale, W. (2008). Type 1 corticotropin-releasing factor receptor expression reported in BAC transgenic mice: implications for reconciling ligand-receptor mismatch in the central corticotropin-releasing factor system. J. Comp. Neurol. 511, 479–496. doi: 10.1002/cne.21848
Kadner, A., Pressimone, V. J., Lally, B. E., Salm, A. K., and Berrebi, A. S. (2006). Low-frequency hearing loss in prenatally stressed rats. Neuroreport 17, 635–638. doi: 10.1097/00001756-200604240-00015
Kaiser, A., Alexandrova, O., and Grothe, B. (2011). Urocortin-expressing olivocochlear neurons exhibit tonotopic and developmental changes in the auditory brainstem and in the innervation of the cochlea. J. Comp. Neurol. 519, 2758–2778. doi: 10.1002/cne.22650
Karl, A., Malta, L. S., and Maercker, A. (2006). Meta-analytic review of event-related potential studies in post-traumatic stress disorder. Biol. Psychol. 71, 123–147. doi: 10.1016/j.biopsycho.2005.03.004
Kelly, J. B., van Adel, B. A., and Ito, M. (2009). Anatomical projections of the nuclei of the lateral lemniscus in the albino rat (Rattus norvegicus). J. Comp. Neurol. 512, 573–593. doi: 10.1002/cne.21929
Klepper, A., and Herbert, H. (1991). Distribution and origin of noradrenergic and serotonergic fibers in the cochlear nucleus and inferior colliculus of the rat. Brain Res. 557, 190–201. doi: 10.1016/0006-8993(91)90134-h
Koehler, S. D., and Shore, S. E. (2013). Stimulus-timing dependent multisensory plasticity in the guinea pig dorsal cochlear nucleus. PLoS One 8:e59828. doi: 10.1371/journal.pone.0059828
Koelsch, S. (2014). Brain correlates of music-evoked emotions. Nat. Rev. Neurosci. 15, 170–180. doi: 10.1038/nrn3666
Koelsch, S., Skouras, S., Fritz, T., Herrera, P., Bonhage, C., Kussner, M. B., et al. (2013). The roles of superficial amygdala and auditory cortex in music-evoked fear and joy. Neuroimage 81, 49–60. doi: 10.1016/j.neuroimage.2013.05.008
Kopp-Scheinpflug, C., and Linden, J. F. (2021). “Coding of Temporal Information,” in The Senses: A Comprehensive Reference, ed. B. Fritzsch (Cambridge: Academic Press), 691–712.
Kopp-Scheinpflug, C., Sinclair, J. L., and Linden, J. F. (2018). When Sound Stops: Offset Responses in the Auditory System. Trends Neurosci. 41, 712–728. doi: 10.1016/j.tins.2018.08.009
Kozicz, T., Yanaihara, H., and Arimura, A. (1998). Distribution of urocortin-like immunoreactivity in the central nervous system of the rat. J. Comp. Neurol. 391, 1–10. doi: 10.1002/(sici)1096-9861(19980202)391
Lauer, A. M., Connelly, C. J., Graham, H., and Ryugo, D. K. (2013). Morphological characterization of bushy cells and their inputs in the laboratory mouse (Mus musculus) anteroventral cochlear nucleus. PLoS One 8:e73308. doi: 10.1371/journal.pone.0073308
LeDoux, J. E., Sakaguchi, A., and Reis, D. J. (1984). Subcortical efferent projections of the medial geniculate nucleus mediate emotional responses conditioned to acoustic stimuli. J. Neurosci. 4, 683–698.
Lesicko, A. M., Hristova, T. S., Maigler, K. C., and Llano, D. A. (2016). Connectional Modularity of Top-Down and Bottom-Up Multimodal Inputs to the Lateral Cortex of the Mouse Inferior Colliculus. J. Neurosci. 36, 11037–11050. doi: 10.1523/JNEUROSCI.4134-15.2016
Levine, R. A. (1999). Somatic (craniocervical) tinnitus and the dorsal cochlear nucleus hypothesis. Am. J. Otolaryngol. 20, 351–362. doi: 10.1016/s0196-0709(99)90074-1
Levitt, P., and Moore, R. Y. (1978). Noradrenaline neuron innervation of the neocortex in the rat. Brain Res. 139, 219–231. doi: 10.1016/0006-8993(78)90925-3
Lewis, K., Li, C., Perrin, M. H., Blount, A., Kunitake, K., Donaldson, C., et al. (2001). Identification of urocortin III, an additional member of the corticotropin-releasing factor (CRF) family with high affinity for the CRF2 receptor. Proc. Natl. Acad. Sci. USA 98, 7570–7575. doi: 10.1073/pnas.121165198
Li, C., Vaughan, J., Sawchenko, P. E., and Vale, W. W. (2002). Urocortin III-immunoreactive projections in rat brain: partial overlap with sites of type 2 corticotrophin-releasing factor receptor expression. J. Neurosci. 22, 991–1001.
Liberman, M. C. (1991). Central projections of auditory-nerve fibers of differing spontaneous rate. I. Anteroventral cochlear nucleus. J. Comp. Neurol. 313, 240–258. doi: 10.1002/cne.903130205
Liberman, M. C. (1993). Central projections of auditory nerve fibers of differing spontaneous rate, II: Posteroventral and dorsal cochlear nuclei. J. Comp. Neurol. 327, 17–36. doi: 10.1002/cne.903270103
Lin, C. S., Lin, Y. S., Liu, C. F., Weng, S. F., Lin, C., and Lin, B. S. (2016). Increased risk of sudden sensorineural hearing loss in patients with depressive disorders: population-based cohort study. J. Laryngol. Otol. 130, 42–49. doi: 10.1017/S0022215115002960
Linke, R., Braune, G., and Schwegler, H. (2000). Differential projection of the posterior paralaminar thalamic nuclei to the amygdaloid complex in the rat. Exp. Brain Res. 134, 520–532. doi: 10.1007/s002210000475
Liu, H., Brown, J. L., Jasmin, L., Maggio, J. E., Vigna, S. R., Mantyh, P. W., et al. (1994). Synaptic relationship between substance P and the substance P receptor: light and electron microscopic characterization of the mismatch between neuropeptides and their receptors. Proc. Natl. Acad. Sci. USA 91, 1009–1013. doi: 10.1073/pnas.91.3.1009
Liu, X. G., and Sandkuhler, J. (1995). The effects of extrasynaptic substance P on nociceptive neurons in laminae I and II in rat lumbar spinal dorsal horn. Neuroscience 68, 1207–1218. doi: 10.1016/0306-4522(95)00187-n
Lu, E., Llano, D. A., and Sherman, S. M. (2009). Different distributions of calbindin and calretinin immunostaining across the medial and dorsal divisions of the mouse medial geniculate body. Hear. Res. 257, 16–23. doi: 10.1016/j.heares.2009.07.009
Ludwig, M., and Leng, G. (2006). Dendritic peptide release and peptide-dependent behaviours. Nat. Rev. Neurosci. 7, 126–136. doi: 10.1038/nrn1845
Malfatti, T., Ciralli, B., Hilscher, M. M., Edwards, S. J., Kullander, K., Leao, R. N., et al. (2021). Using Cortical neuron markers to target cells in the dorsal cochlear nucleus. eNeuro 8:2020. doi: 10.1523/ENEURO.0413-20.2020
Malmierca, M. S., Merchan, M. A., Henkel, C. K., and Oliver, D. L. (2002). Direct projections from cochlear nuclear complex to auditory thalamus in the rat. J. Neurosci. 22, 10891–10897.
Marquez-Legorreta, E., Horta-Junior Jde, A., Berrebi, A. S., and Saldana, E. (2016). Organization of the Zone of Transition between the Pretectum and the Thalamus, with Emphasis on the Pretectothalamic Lamina. Front. Neuroanat. 10:82. doi: 10.3389/fnana.2016.00082
Masuda, M., Kanzaki, S., Minami, S., Kikuchi, J., Kanzaki, J., Sato, H., et al. (2012). Correlations of inflammatory biomarkers with the onset and prognosis of idiopathic sudden sensorineural hearing loss. Otol. Neurotol. 33, 1142–1150. doi: 10.1097/MAO.0b013e3182635417
Mazurek, B., Haupt, H., Joachim, R., Klapp, B. F., Stover, T., and Szczepek, A. J. (2010). Stress induces transient auditory hypersensitivity in rats. Hear. Res. 259, 55–63. doi: 10.1016/j.heares.2009.10.006
McDonald, A. J. (1998). Cortical pathways to the mammalian amygdala. Prog. Neurobiol. 55, 257–332. doi: 10.1016/s0301-0082(98)00003-3
Meininger, V., Pol, D., and Derer, P. (1986). The inferior colliculus of the mouse. A Nissl and Golgi study. Neuroscience 17, 1159–1179. doi: 10.1016/0306-4522(86)90085-0
Merchán, M. A., Malmierca, M. S., Bajo, V. M., and Bjaalie, J. G. (1997). “The nuclei of the lateral lemniscus,” in Acoustical signal processing in the central auditory system, ed. J. Syka. Berlin: Springer. 211–226.
Metzner, W. (1993). An audio-vocal interface in echolocating horseshoe bats. J. Neurosci. 13, 1899–1915.
Mugnaini, E., Warr, W. B., and Osen, K. K. (1980). Distribution and light microscopic features of granule cells in the cochlear nuclei of cat, rat, and mouse. J. Comp. Neurol. 191, 581–606. doi: 10.1002/cne.901910406
Mulders, W. H., and Robertson, D. (2001). Origin of the noradrenergic innervation of the superior olivary complex in the rat. J. Chem. Neuroanat. 21, 313–322. doi: 10.1016/s0891-0618(01)00118-1
Neuser, J., and Knoop, T. (1986). Sudden idiopathic hearing loss: psychopathology and antecedent stressful life-events. Br. J. Med. Psychol. 59, 245–251. doi: 10.1111/j.2044-8341.1986.tb02690.x
Ngodup, T., Romero, G. E., and Trussell, L. O. (2020). Identification of an inhibitory neuron subtype, the L-stellate cell of the cochlear nucleus. Elife 9:54350. doi: 10.7554/eLife.54350
Oertel, D., Bal, R., Gardner, S. M., Smith, P. H., and Joris, P. X. (2000). Detection of synchrony in the activity of auditory nerve fibers by octopus cells of the mammalian cochlear nucleus. Proc. Natl. Acad. Sci. USA 97, 11773–11779. doi: 10.1073/pnas.97.22.11773
Oertel, D., and Wickesberg, R. E. (2002). “Ascending pathways through ventral nuclei of the lateral lemniscus and their possible role in pattern recognition in natural sounds,” in Integrative functions in the mammalian auditory pathway, eds D. Oertel and R. Fay (Berlin: Springer), 207–237.
Oertel, D., Wright, S., Cao, X. J., Ferragamo, M., and Bal, R. (2011). The multiple functions of T stellate/multipolar/chopper cells in the ventral cochlear nucleus. Hear. Res. 276, 61–69. doi: 10.1016/j.heares.2010.10.018
Oertel, D., Wu, S. H., Garb, M. W., and Dizack, C. (1990). Morphology and physiology of cells in slice preparations of the posteroventral cochlear nucleus of mice. J. Comp. Neurol. 295, 136–154. doi: 10.1002/cne.902950112
Ohlrogge, M., Doucet, J. R., and Ryugo, D. K. (2001). Projections of the pontine nuclei to the cochlear nucleus in rats. J. Comp. Neurol. 436, 290–303.
Oliver, D. L., and Shneiderman, A. (1989). An EM study of the dorsal nucleus of the lateral lemniscus: inhibitory, commissural, synaptic connections between ascending auditory pathways. J. Neurosci. 9, 967–982.
Osen, K. K. (1969). Cytoarchitecture of the cochlear nuclei in the cat. J. Comp. Neurol. 136, 453–484. doi: 10.1002/cne.901360407
Pacheco-Unguetti, A. P., and Parmentier, F. B. (2014). Sadness increases distraction by auditory deviant stimuli. Emotion 14, 203–213. doi: 10.1037/a0034289
Park, J. S., Cederroth, C. R., Basinou, V., Meltser, I., Lundkvist, G., and Canlon, B. (2016). Identification of a circadian clock in the inferior colliculus and its dysregulation by noise exposure. J. Neurosci. 36, 5509–5519. doi: 10.1523/JNEUROSCI.3616-15.2016
Perrin, M., Donaldson, C., Chen, R., Blount, A., Berggren, T., Bilezikjian, L., et al. (1995). Identification of a second corticotropin-releasing factor receptor gene and characterization of a cDNA expressed in heart. Proc. Natl. Acad. Sci. USA 92, 2969–2973. doi: 10.1073/pnas.92.7.2969
Phelps, E. A., and LeDoux, J. E. (2005). Contributions of the amygdala to emotion processing: from animal models to human behavior. Neuron 48, 175–187. doi: 10.1016/j.neuron.2005.09.025
Reyes, T. M., Lewis, K., Perrin, M. H., Kunitake, K. S., Vaughan, J., Arias, C. A., et al. (2001). Urocortin II: a member of the corticotropin-releasing factor (CRF) neuropeptide family that is selectively bound by type 2 CRF receptors. Proc. Natl. Acad. Sci. USA 98, 2843–2848. doi: 10.1073/pnas.051626398
Rhea, E. M., Rask-Madsen, C., and Banks, W. A. (2018). Insulin transport across the blood-brain barrier can occur independently of the insulin receptor. J. Physiol. 596, 4753–4765. doi: 10.1113/JP276149
Romanski, L. M., and LeDoux, J. E. (1993). Information cascade from primary auditory cortex to the amygdala: corticocortical and corticoamygdaloid projections of temporal cortex in the rat. Cereb. Cortex 3, 515–532. doi: 10.1093/cercor/3.6.515
Rubio, M. E., Gudsnuk, K. A., Smith, Y., and Ryugo, D. K. (2008). Revealing the molecular layer of the primate dorsal cochlear nucleus. Neuroscience 154, 99–113. doi: 10.1016/j.neuroscience.2007.12.016
Russo, A. F. (2017). Overview of neuropeptides: awakening the senses? Headache 57, (Suppl. 2), 37–46. doi: 10.1111/head.13084
Ryan, A. F., Keithley, E. M., Wang, Z. X., and Schwartz, I. R. (1990). Collaterals from lateral and medial olivocochlear efferent neurons innervate different regions of the cochlear nucleus and adjacent brainstem. J. Comp. Neurol. 300, 572–582. doi: 10.1002/cne.903000410
Ryugo, D. K. (2008). Projections of low spontaneous rate, high threshold auditory nerve fibers to the small cell cap of the cochlear nucleus in cats. Neuroscience 154, 114–126. doi: 10.1016/j.neuroscience.2007.10.052
Ryugo, D. K., Haenggeli, C. A., and Doucet, J. R. (2003). Multimodal inputs to the granule cell domain of the cochlear nucleus. Exp. Brain Res. 153, 477–485. doi: 10.1007/s00221-003-1605-3
Sakanaka, M., Shibasaki, T., and Lederis, K. (1987). Corticotropin-releasing factor-containing afferents to the inferior colliculus of the rat brain. Brain Res. 414, 68–76. doi: 10.1016/0006-8993(87)91326-6
Sapolsky, R. M., Romero, L. M., and Munck, A. U. (2000). How do glucocorticoids influence stress responses? Integrating permissive, suppressive, stimulatory, and preparative actions. Endocr. Rev. 21, 55–89. doi: 10.1210/edrv.21.1.0389
Schmitt, C., Patak, M., and Kroner-Herwig, B. (2000). Stress and the onset of sudden hearing loss and tinnitus. Int. Tinnitus J. 6, 41–49.
Schofield, B. R., Motts, S. D., and Mellott, J. G. (2011). Cholinergic cells of the pontomesencephalic tegmentum: connections with auditory structures from cochlear nucleus to cortex. Hear. Res. 279, 85–95. doi: 10.1016/j.heares.2010.12.019
Schulz, K., Rutz, C., Westendorf, C., Ridelis, I., Vogelbein, S., Furkert, J., et al. (2010). The pseudo signal peptide of the corticotropin-releasing factor receptor type 2a decreases receptor expression and prevents Gi-mediated inhibition of adenylyl cyclase activity. J. Biol. Chem. 285, 32878–32887. doi: 10.1074/jbc.M110.129627
Shalev, A. Y., Peri, T., Brandes, D., Freedman, S., Orr, S. P., and Pitman, R. K. (2000). Auditory startle response in trauma survivors with posttraumatic stress disorder: a prospective study. Am. J. Psychiatry 157, 255–261. doi: 10.1176/appi.ajp.157.2.255
Sharpe, A. L., and Phillips, T. J. (2009). Central urocortin 3 administration decreases limited-access ethanol intake in nondependent mice. Behav. Pharmacol. 20, 346–351. doi: 10.1097/FBP.0b013e32832f01ba
Shemesh, Y., Forkosh, O., Mahn, M., Anpilov, S., Sztainberg, Y., Manashirov, S., et al. (2016). Ucn3 and CRF-R2 in the medial amygdala regulate complex social dynamics. Nat. Neurosci. 19, 1489–1496. doi: 10.1038/nn.4346
Shore, S. E. (2005). Multisensory integration in the dorsal cochlear nucleus: unit responses to acoustic and trigeminal ganglion stimulation. Eur. J. Neurosci. 21, 3334–3348. doi: 10.1111/j.1460-9568.2005.04142.x
Singla, S., Dempsey, C., Warren, R., Enikolopov, A. G., and Sawtell, N. B. (2017). A cerebellum-like circuit in the auditory system cancels responses to self-generated sounds. Nat. Neurosci. 20, 943–950. doi: 10.1038/nn.4567
Sizemore, T. R., Hurley, L. M., and Dacks, A. M. (2020). Serotonergic modulation across sensory modalities. J. Neurophysiol. 123, 2406–2425. doi: 10.1152/jn.00034.2020
Stengel, A., and Tache, Y. (2018). Gut-Brain neuroendocrine signaling under conditions of stress-focus on food intake-regulatory mediators. Front. Endocrinol. 9:498. doi: 10.3389/fendo.2018.00498
Szczepek, A. J., Dietz, G. P. H., Reich, U., Hegend, O., Olze, H., and Mazurek, B. (2018). Differences in stress-induced modulation of the auditory system between wistar and lewis rats. Front. Neurosci. 12:828. doi: 10.3389/fnins.2018.00828
Takahashi, T. T., Carr, C. E., Brecha, N., and Konishi, M. (1987). Calcium binding protein-like immunoreactivity labels the terminal field of nucleus laminaris of the barn owl. J. Neurosci. 7, 1843–1856.
Teichmann, A., Rutz, C., Kreuchwig, A., Krause, G., Wiesner, B., and Schulein, R. (2012). The Pseudo signal peptide of the corticotropin-releasing factor receptor type 2A prevents receptor oligomerization. J. Biol. Chem. 287, 27265–27274. doi: 10.1074/jbc.M112.360594
Thompson, A. M., and Thompson, G. C. (1991). Posteroventral cochlear nucleus projections to olivocochlear neurons. J. Comp. Neurol. 303, 267–285. doi: 10.1002/cne.903030209
Trost, W., Ethofer, T., Zentner, M., and Vuilleumier, P. (2012). Mapping aesthetic musical emotions in the brain. Cereb. Cortex 22, 2769–2783. doi: 10.1093/cercor/bhr353
Trussell, L. O., and Oertel, D. (2018). “Microcircuits of the dorsal cochlear nucleus,” in The Mammalian Auditory Pathways, eds D. L. Oliver, N. Cant, R. R. Fay, and A. N. Popper (New York: Springer), 73–99.
van den Pol, A. N. (2012). Neuropeptide transmission in brain circuits. Neuron 76, 98–115. doi: 10.1016/j.neuron.2012.09.014
Varga, T., Palkovits, M., Usdin, T. B., and Dobolyi, A. (2008). The medial paralemniscal nucleus and its afferent neuronal connections in rat. J. Comp. Neurol. 511, 221–237. doi: 10.1002/cne.21829
Vila-Porcile, E., Xu, Z. Q., Mailly, P., Nagy, F., Calas, A., Hokfelt, T., et al. (2009). Dendritic synthesis and release of the neuropeptide galanin: morphological evidence from studies on rat locus coeruleus neurons. J. Comp. Neurol. 516, 199–212. doi: 10.1002/cne.22105
Vita, N., Laurent, P., Lefort, S., Chalon, P., Lelias, J. M., Kaghad, M., et al. (1993). Primary structure and functional expression of mouse pituitary and human brain corticotrophin releasing factor receptors. FEBS Lett. 335, 1–5. doi: 10.1016/0014-5793(93)80427-v
Webster, D. B., and Trune, D. R. (1982). Cochlear nuclear complex of mice. Am. J. Anat. 163, 103–130. doi: 10.1002/aja.1001630202
Weedman, D. L., Pongstaporn, T., and Ryugo, D. K. (1996). Ultrastructural study of the granule cell domain of the cochlear nucleus in rats: mossy fiber endings and their targets. J. Comp. Neurol. 369, 345–360.
Willard, F. H., and Ryugo, D. K. (1983). Anatomy of the central auditory system in The auditory psychobiology of the Mouse, (Springfield, IL: Charles C Thomas Publisher), 201–304.
Winer, J. A. (1992). “The functional architecture of the medial geniculate body and the primary auditory cortex,” in The mammalian auditory pathway: Neuroanatomy, eds D. B. Webster and R. R. Fay New York, NY: Springer 222–409.
Winer, J. A., and Schreiner, C. E. (2005). “The central auditory system: a functional analysis,” in The Inferior Colliculus, eds J. A. Winer and C. E. Schreiner (New York, NY: Springer), 1–68.
Wong, M. Y., Zhou, C., Shakiryanova, D., Lloyd, T. E., Deitcher, D. L., and Levitan, E. S. (2012). Neuropeptide delivery to synapses by long-range vesicle circulation and sporadic capture. Cell 148, 1029–1038. doi: 10.1016/j.cell.2011.12.036
Wright, D. D., and Ryugo, D. K. (1996). Mossy fiber projections from the cuneate nucleus to the cochlear nucleus in the rat. J. Comp. Neurol. 365, 159–172.
Yaeger, D. B., and Trussell, L. O. (2015). Single granule cells excite Golgi cells and evoke feedback inhibition in the cochlear nucleus. J. Neurosci. 35, 4741–4750. doi: 10.1523/JNEUROSCI.3665-14.2015
Yang, Y., Saint Marie, R. L., and Oliver, D. L. (2005). Granule cells in the cochlear nucleus sensitive to sound activation detected by Fos protein expression. Neuroscience 136, 865–882. doi: 10.1016/j.neuroscience.2005.02.007
Ye, Y., Machado, D. G., and Kim, D. O. (2000). Projection of the marginal shell of the anteroventral cochlear nucleus to olivocochlear neurons in the cat. J. Comp. Neurol. 420, 127–138.
Yeh, C., Ting, C. H., Doong, M. L., Chi, C. W., Lee, S. D., and Chen, C. Y. (2016). Intracerebroventricular urocortin 3 counteracts central acyl ghrelin-induced hyperphagic and gastroprokinetic effects via CRF receptor 2 in rats. Drug Des. Devel. Ther. 10, 3281–3290. doi: 10.2147/DDDT.S113195
Zeng, C., Shroff, H., and Shore, S. E. (2011). Cuneate and spinal trigeminal nucleus projections to the cochlear nucleus are differentially associated with vesicular glutamate transporter-2. Neuroscience 176, 142–151. doi: 10.1016/j.neuroscience.2010.12.010
Zhan, X., Pongstaporn, T., and Ryugo, D. K. (2006). Projections of the second cervical dorsal root ganglion to the cochlear nucleus in rats. J. Comp. Neurol. 496, 335–348. doi: 10.1002/cne.20917
Zhan, X., and Ryugo, D. K. (2007). Projections of the lateral reticular nucleus to the cochlear nucleus in rats. J. Comp. Neurol. 504, 583–598. doi: 10.1002/cne.21463
Zhao, H. B., Parham, K., Ghoshal, S., and Kim, D. O. (1995). Small neurons in the vestibular nerve root project to the marginal shell of the anteroventral cochlear nucleus in the cat. Brain Res. 700, 295–298. doi: 10.1016/0006-8993(95)01078-a
Keywords: urocortin, CRFR2, auditory, stress signaling, multimodal, volume transmission, calyx of Held synapse
Citation: Pagella S, Deussing JM and Kopp-Scheinpflug C (2021) Expression Patterns of the Neuropeptide Urocortin 3 and Its Receptor CRFR2 in the Mouse Central Auditory System. Front. Neural Circuits 15:747472. doi: 10.3389/fncir.2021.747472
Received: 26 July 2021; Accepted: 18 October 2021;
Published: 12 November 2021.
Edited by:
Catherine Carr, University of Maryland, College Park, United StatesReviewed by:
Douglas E. Vetter, University of Mississippi Medical Center, United StatesMax Anstötz, Northwestern University, United States
Laura M. Hurley, Indiana University Bloomington, United States
Copyright © 2021 Pagella, Deussing and Kopp-Scheinpflug. This is an open-access article distributed under the terms of the Creative Commons Attribution License (CC BY). The use, distribution or reproduction in other forums is permitted, provided the original author(s) and the copyright owner(s) are credited and that the original publication in this journal is cited, in accordance with accepted academic practice. No use, distribution or reproduction is permitted which does not comply with these terms.
*Correspondence: Conny Kopp-Scheinpflug, Y2tzQGJpby5sbXUuZGU=