- 1Department of Psychiatry, Columbia University, New York, NY, United States
- 2Department of Molecular Therapeutics, New York State Psychiatric Institute, New York, NY, United States
- 3Neuroscience Initiative, Advanced Science Research Center, Graduate Center of The City University of New York, New York, NY, United States
- 4Department of Psychology, College of Staten Island, City University of New York, Staten Island, NY, United States
- 5CUNY Neuroscience Collaborative, The Graduate Center, City University of New York, New York, NY, United States
Discovered just over 20 years ago, dopamine neurons have the ability to cotransmit both dopamine and glutamate. Yet, the functional roles of dopamine neuron glutamate cotransmission and their implications for therapeutic use are just emerging. This review article encompasses the current body of evidence investigating the functions of dopamine neurons of the ventral midbrain that cotransmit glutamate. Since its discovery in dopamine neuron cultures, further work in vivo confirmed dopamine neuron glutamate cotransmission across species. From there, growing interest has led to research related to neural functioning including roles in synaptic signaling, development, and behavior. Functional connectome mapping reveals robust connections in multiple forebrain regions to various cell types, most notably to cholinergic interneurons in both the medial shell of the nucleus accumbens and the lateral dorsal striatum. Glutamate markers in dopamine neurons reach peak levels during embryonic development and increase in response to various toxins, suggesting dopamine neuron glutamate cotransmission may serve neuroprotective roles. Findings from behavioral analyses reveal prominent roles for dopamine neuron glutamate cotransmission in responses to psychostimulants, in positive valence and cognitive systems and for subtle roles in negative valence systems. Insight into dopamine neuron glutamate cotransmission informs the pathophysiology of neuropsychiatric disorders such as addiction, schizophrenia and Parkinson Disease, with therapeutic implications.
Introduction
Dopamine (DA) neurons were first identified by their monoamine content, and then by the expression of the DA synthetic enzyme tyrosine hydroxylase (TH) (for review see Iversen and Iversen, 2007). Heterogeneity of DA neurons was first recognized as mediolateral differences between ventral tegmental area (VTA) and substantia nigra (SN) DA neurons (for reviews on this topic see Grace et al., 2007; Liss and Roeper, 2008). DA neurons, like most central nervous system neurons, use multiple neurotransmitters (Kupfermann, 1991), adding a further dimension of heterogeneity. Peptide cotransmission was recognized first, with evidence that DA neurons use cholecystokinin and neurotensin as cotransmitters (Hökfelt et al., 1980; Gonzalez-Reyes et al., 2012).
Cotransmission involving two small molecule neurotransmitters — especially with competing synaptic actions — was recognized more recently (for review see Hnasko and Edwards, 2012). DA neuron glutamate (GLU) cotransmission was first shown in single-cell microcultures of identified rat DA neurons (Sulzer et al., 1998). Electrical stimulation of genetically tagged DA neurons in quasi-horizontal mouse brain slices revealed DA neuron GLU cotransmission in the ventral striatum (Chuhma et al., 2004) and its frequency dependent modulation by concomitantly released DA (Chuhma et al., 2009). Optogenetic stimulation of DA neuron terminals showed that DA neurons make monosynaptic GLU connections to spiny projection neurons (SPNs) in the nucleus accumbens (NAc) (Stuber et al., 2010; Tecuapetla et al., 2010). DA neurons cotransmitting GLU (DA-GLU neurons) require both vesicular monoamine transporter 2 (VMAT2) for DA release (Fon et al., 1997) and vesicular glutamate transporter 2 (VGLUT2 for protein, VGluT2 for gene and mRNA) for GLU release (Dal Bo et al., 2004; Hnasko et al., 2010; Stuber et al., 2010). DA neurons also use GABA as a small molecule cotransmitter (for reviews see Tritsch et al., 2012; Granger et al., 2017). DA neuron GLU cotransmission extends from fruit flies to humans (Figure 1), arguing for important physiological roles.
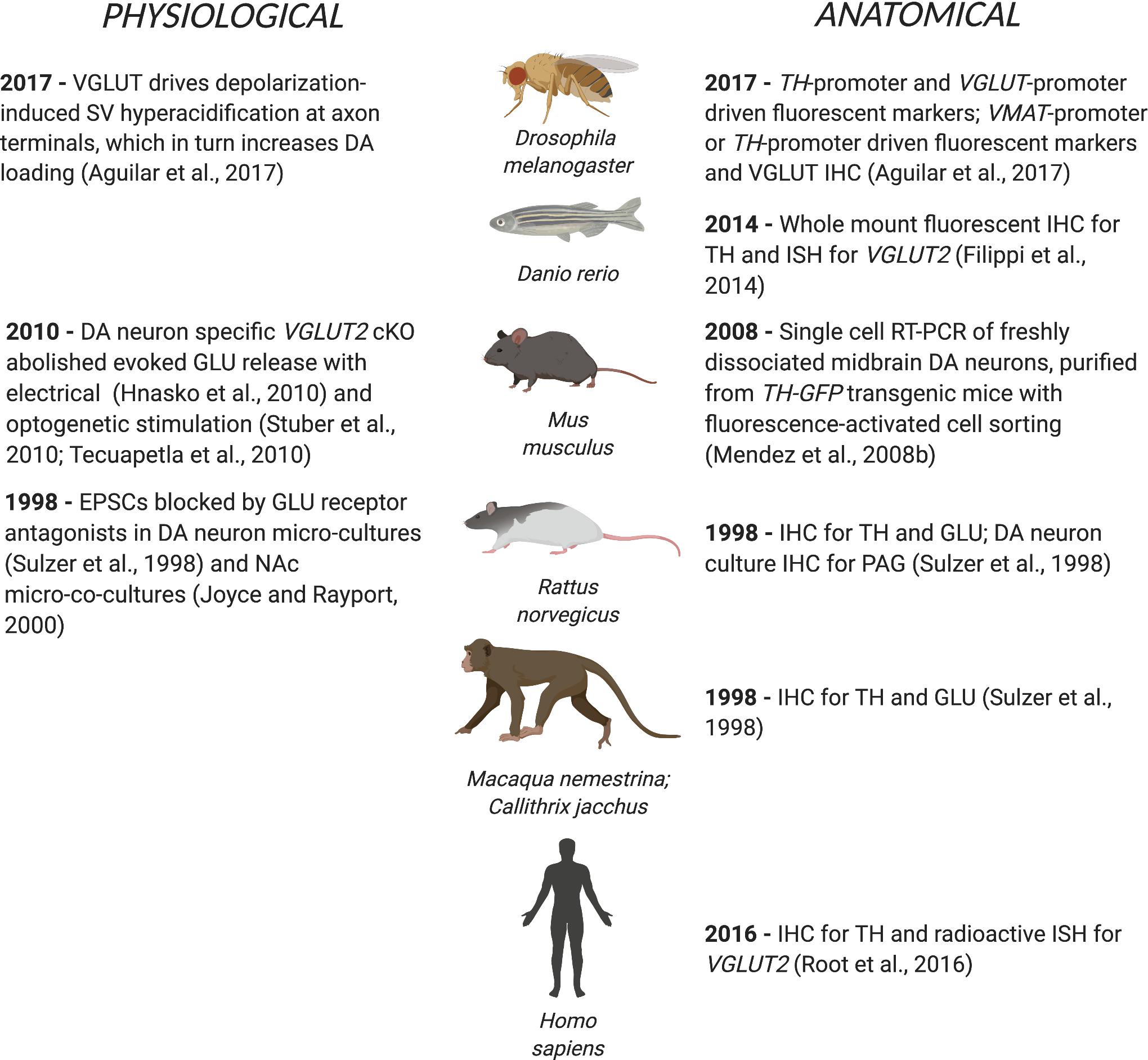
Figure 1. DA neuron GLU cotransmission spans phylogeny from flies to humans. The first physiological or anatomical evidence for DA neuron GLU cotransmission is cited by species.
This review focuses on DA neuron GLU cotransmission and addresses the key questions: (1) Where do DA-GLU neurons project? (2) Are DA and GLU released together or separately? (3) What are the synaptic functions of DA neuron GLU cotransmission? (4) What are the developmental roles of DA neuron GLU cotransmission? (5) How are DA-GLU neurons affected by DA neuron toxins? (6) What are the behavioral roles of DA neuron GLU cotransmission? (7) Does DA neuron GLU cotransmission have a role in human disorders?
Where Do DA-GLU Neurons Project?
DA-GLU Neurons in the Ventral Midbrain
Dopamine neurons in the ventral midbrain are divided between the VTA and SN. DA-GLU neurons show a medial preponderance, are mainly in the VTA, and project predominantly to the ventral striatum/NAc (Li et al., 2013; Morales and Root, 2014; Yamaguchi et al., 2015; Zhang et al., 2015; Root et al., 2016; Chuhma et al., 2018; Poulin et al., 2018; Mingote et al., 2019). DA-GLU neurons are identified by TH and VGluT2 expression. Expression of VGluT2 in DA neurons is necessary and sufficient to enable GLU cotransmission (Takamori et al., 2000). Indeed, DA-neuron-specific VGluT2 cKO eliminated GLU-cotransmission synaptic responses (Stuber et al., 2010). Visualizing VGluT2 expression in cell bodies requires in situ hybridization (ISH) or ectopic reporter expression driven by the VGluT2 promoter, as VGLUT2 is rapidly exported to axon terminals. The number of DA-GLU (i.e., TH+/VGLUT2+) neurons varies across the lifespan, species, brain region and study (Table 1). In the VTA, DA-GLU neurons account for 10-30% of DA neurons, and are most abundant in the interfascicular nucleus (IF), the central linear nucleus (CLi), the rostral linear nucleus (RLi), and the parabrachial pigmented nucleus (PBP) (Kawano et al., 2006; Li et al., 2013). In the SN, DA-GLU neurons account for about 5–10%, and are most abundant in the dorsal SN pars compacta (SNc) and the pars lateralis in rodents, as well as primates including humans (Yamaguchi et al., 2013; Root et al., 2016; Steinkellner et al., 2018).
DA-GLU Projections
Combinatorial intersectional genetic strategies (Fenno et al., 2014, 2020) have enabled visualization of DA-GLU neurons and their projections (Poulin et al., 2018). This has confirmed that DA-GLU neurons comprise about 30% of VTA neurons (Poulin et al., 2018; Mingote et al., 2019) and send dense projections to the NAc medial shell (m-shell), discrete, dense, column-like projections to the olfactory tubercle (OT), and sparse projections to the prefrontal cortex (PFC), mostly to deeper layers of the infralimbic and prelimbic cortices (Poulin et al., 2018). Particularly in the dorsal portion of the m-shell, all TH+ fibers are VGLUT2+, indicating that DA neuron projections in this region are predominantly from DA-GLU neurons, consistent with recent retrograde tracer studies (Mongia et al., 2019). DA-GLU neurons in the lateral SNc project to the lateral dorsal striatum with denser projections to the caudal striatum, or tail (Poulin et al., 2018). SNc DA-GLU neurons also project to the central nucleus of the amygdala (CeA), the lateral part of the capsular division, and sparsely to the ventral-most lateral nucleus and the posterior nucleus, as well as to DA islands in the entorhinal cortex (EntC) (Poulin et al., 2018; Mingote et al., 2019). Thus, DA-GLU neurons have discrete, but widely distributed forebrain projections.
Physiological Connectivity of DA-GLU Neurons
Functional connectome mapping has addressed how the projections of DA-GLU neurons translate to their synaptic actions (Mingote et al., 2015a). Functional connectome mapping is the systematic recording of the strength and incidence of monosynaptic connections to identified postsynaptic neurons by optogenetic stimulation of genetically defined presynaptic neuron populations (Chuhma et al., 2011; Chuhma, 2015, 2021; Eskenazi et al., 2019). DA neurons make the most robust GLU connections in the ventral striatum, in the NAc core and shell, and the OT (Wieland et al., 2014), in accordance with the densest DA-GLU neuron projections (Poulin et al., 2018; Mingote et al., 2019; Figure 2). In the NAc m-shell, DA-GLU neurons elicit fast glutamatergic EPSCs mediated by inotropic GLU receptors (iGluR) in all SPNs, fast-spiking interneurons (FSIs) and cholinergic interneurons (ChIs), with the strongest in ChIs (Chuhma et al., 2014). In the lateral dorsal striatum, the strongest iGluR EPSCs are seen in striatonigral SPNs (Cai and Ford, 2018; Chuhma et al., 2018), and weaker EPSCs in ChIs. In addition, DA-GLU neurons elicit slower EPSCs mediated by metabotropic GLU receptors (mGluRs) in lateral dorsal striatum ChIs (Straub et al., 2014; Cai and Ford, 2018; Chuhma et al., 2018). Outside the striatum, EPSCs are seen occasionally in pyramidal neurons of layers II-III in cingulate cortex (CingC) (Mingote et al., 2015a), and in GABA interneurons in the PFC, contributing to disynaptic inhibition of pyramidal neurons (Kabanova et al., 2015; Pérez-López et al., 2018). DA-GLU neurons projecting to the cortex are mainly located in the RLi, PBP, and rostral VTA (Gorelova et al., 2012). In the EntC, DA-GLU neurons elicit EPSCs in pyramidal neurons in DA islands, while they make no connections in the hippocampus (Mingote et al., 2015a). In line with higher levels of VGluT2 expression in DA neurons projecting to the amygdala (Taylor et al., 2014; Poulin et al., 2018), DA-GLU neurons target the CeA but not the basolateral amygdala (BLA) (Mingote et al., 2015a). Of note, most of these studies have been performed on brain slices from juvenile mice; thus, future studies on mice in early life or late adulthood may differ since the proportion of DA neurons expressing VGluT2 may change with age (see below). In summary, DA-GLU neurons connect to different cell types in different target regions, with the highest incidence of connectivity in the NAc m-shell and lateral dorsal striatum and the largest EPSCs in the EntC.
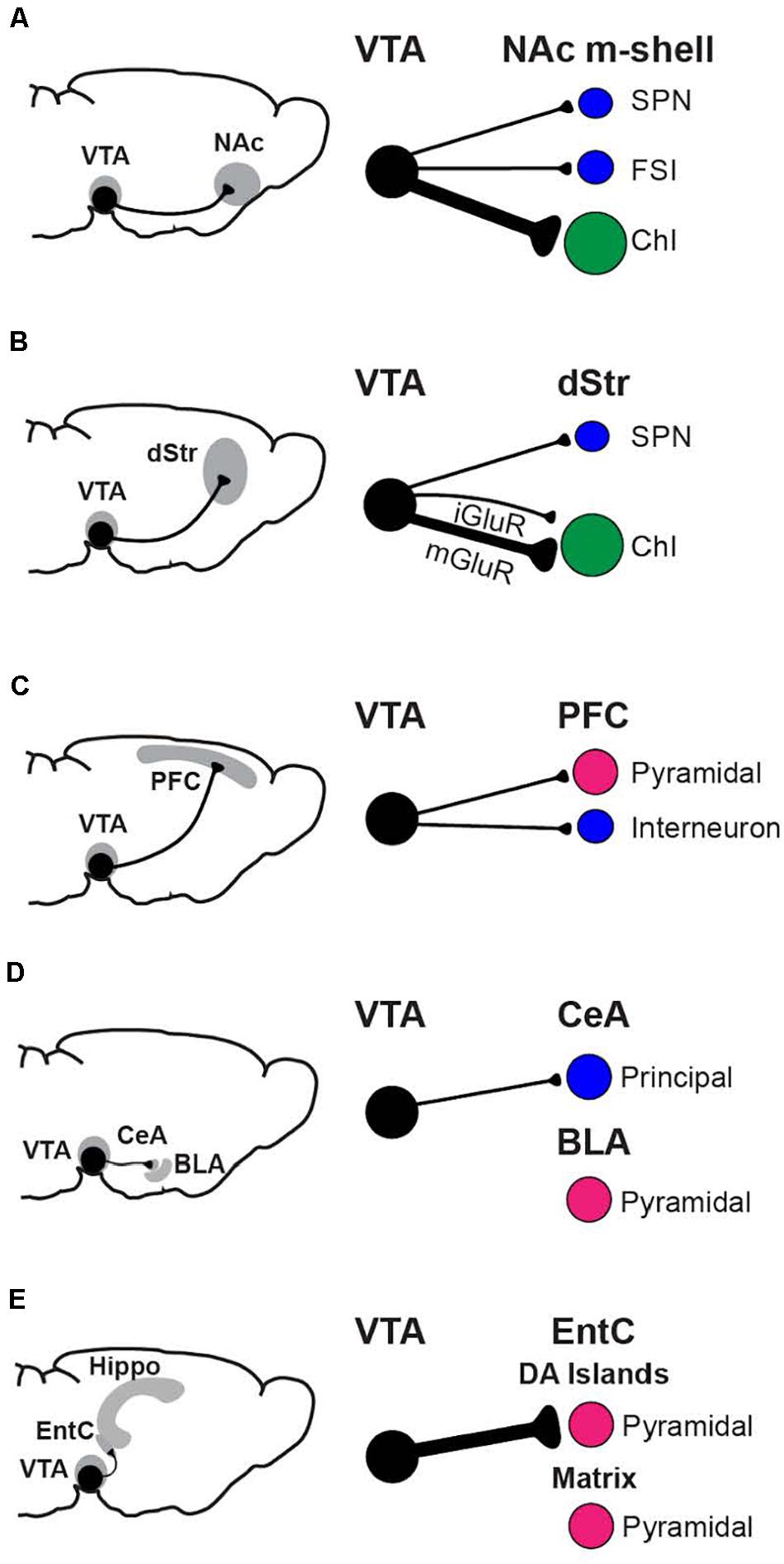
Figure 2. Functional connectome analysis of VTA DA neuron GLU cotransmission. Regions with prominent connections, the NAc m-shell (A), dorsal striatum (B), prefrontal cortex (C), amygdala (D), and hippocampal formation (E) are shown, with the neurons principally targeted by DA-GLU neurons in each region. The strength of connections is indicated by the thickness of the axons (black lines). Postsynaptic neurons are GABAergic (blue), GLUergic (magenta), or cholinergic (green).
Are DA and GLU Released Together or Separately?
Cotransmission can be viewed as a physiological/functional property that may arise from several anatomical/structural arrangements (Figure 3). Here we use the definitions of cotransmission as the release of multiple different neurotransmitters from the same neuron, and corelease as the release of different neurotransmitters from the same synaptic vesicle (SV) (Vaaga et al., 2014; Svensson et al., 2018). Furthermore, SVs with different neurotransmitters may colocalize within the same varicosity, or segregate to different varicosities of the same neuron (e.g., some at symmetric synapses, others at asymmetric synapses).
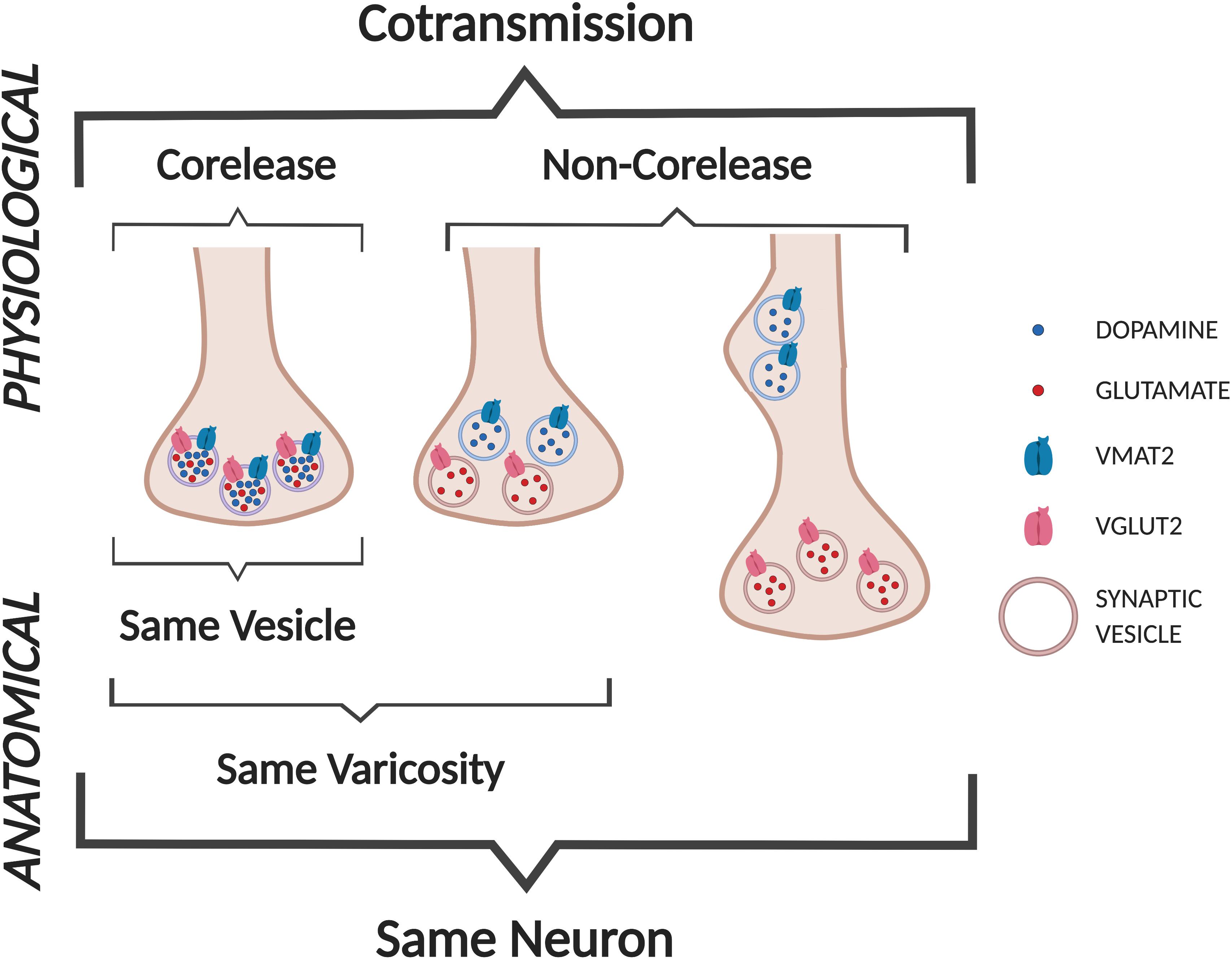
Figure 3. Cotransmission configurations. We define DA neuron GLU cotransmission as the release of DA and GLU from the same neuron. Anatomically, DA and GLU could be released from the same vesicles (labeled as corelease), or from separate sites in the same varicosity, or more distant sites within the same axon (not shown).
For corelease of DA and GLU, individual SVs must have both VMAT2 and VGLUT2. Co-immunoprecipitation with anti-VMAT2 and anti-VGLUT2 antibodies identified a population of striatal SVs consistent with corelease (Hnasko et al., 2010), although not in a subsequent study (Zhang et al., 2015). Uptake of GLU into a SV may potentiate the uptake and subsequent release of DA (Hnasko and Edwards, 2012; Aguilar et al., 2017), via vesicular synergy (Gras et al., 2008; Amilhon et al., 2010; El Mestikawy et al., 2011). Vesicular synergy refers to corelease where one neurotransmitter potentiates the uptake of another neurotransmitter in the same SV (El Mestikawy et al., 2011). VGLUT2 cotransports GLU with a single Cl– into SVs in exchange for a single H+, thereby increasing negative charge inside SVs (Maycox et al., 1988; Cidon and Sihra, 1989) (Figure 4). This negative charge drives vacuolar-type H+-ATPase to increase inward flux of protons, causing SV acidification (Blakely and Edwards, 2012). In turn, DA enters SVs via VMAT2 in exchange for two H+ (Johnson, 1988), resulting in increased intravesicular DA concentration, and increased vesicular DA upon release. Vesicular synergy in DA neuron SVs has been shown by changes in intravesicular pH in response to both DA and GLU gradients (Hnasko et al., 2010; Aguilar et al., 2017). In mouse striatal slices, VGLUT2-dependent SV acidification is associated with increased DA release (Aguilar et al., 2017). DATCre;VGluT2flox/flox cKO mice show less striatal DA release (Stuber et al., 2010; Alsiö et al., 2011) and injections of an AAV-Cre viral vector into the VTA of VGluT2flox/flox mice showed diminished SV acidification (Aguilar et al., 2017). These observations argue for corelease, as they require both VGLUT2 and VMAT2 in the same SV.
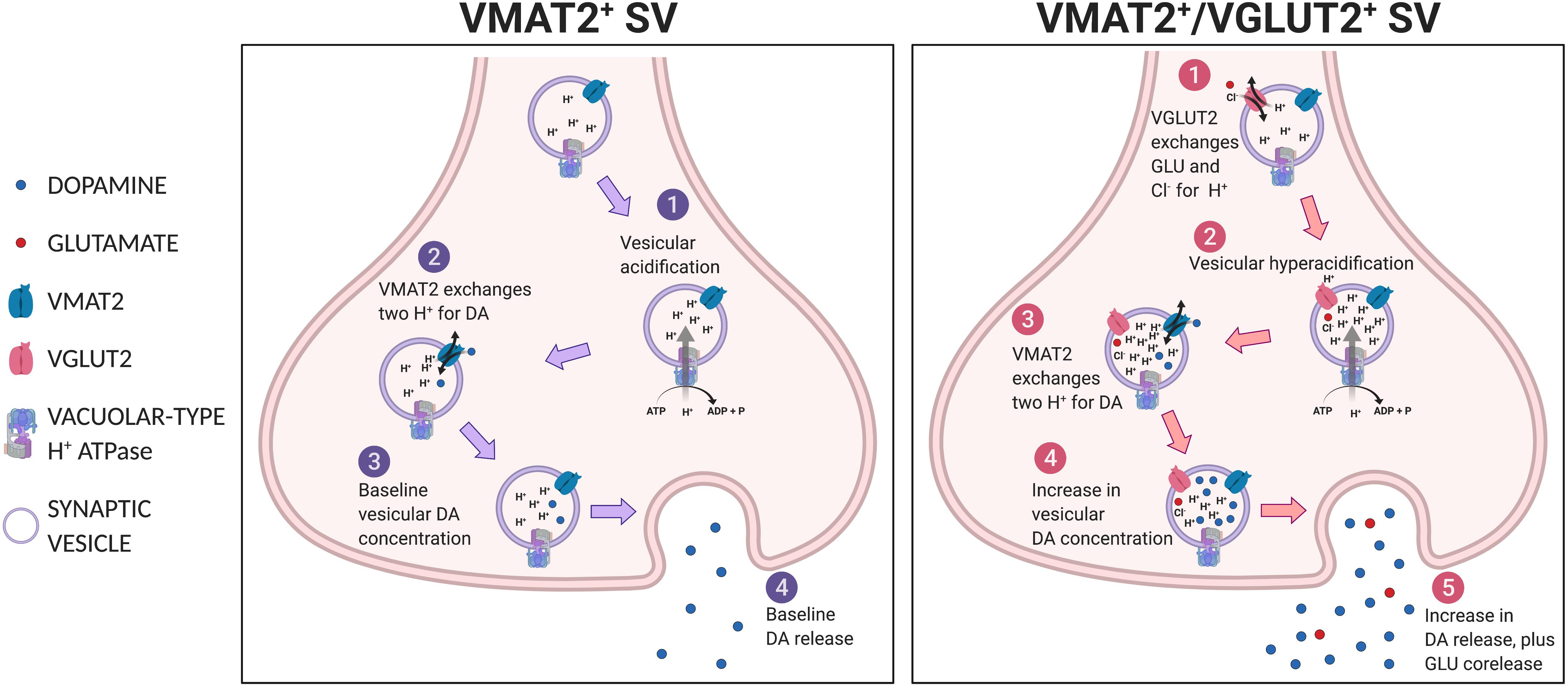
Figure 4. Vesicular synergy. Shown in the left panel, a VMAT2+ SV undergoes (1) vesicular acidification, then (2) VMAT2 exchanges two H+s for DA to achieve (3) baseline vesicular DA concentration and subsequent (4) baseline DA release. Shown in the right panel, a VGLUT2+/VMAT2+ co-expressing SV, (1) VGLUT2 transports GLU and Cl– into SV, which potentiates (2) vacuolar-type H+ATPase to hyperacidify the SV, thus (3) more DA is drawn in via VMAT2 in exchange for protons, resulting in (4) greater intravesicular DA concentration and subsequent release (5).
Anatomically, DA and GLU release sites appear to be segregated. In rats, anterograde tracing from the SN revealed two types of DA neuron synapses in the striatum (Hattori et al., 1991). Symmetric synapses were seen in TH+ varicosities in en passant configuration, consistent with sites of DA release; asymmetric synapses were located in TH– axon terminals, consistent with the release of a non-DA excitatory neurotransmitter. Immunostaining of microcultures of single DA neurons showed that DA neurons have partially overlapping populations of TH+ and GLU+ varicosities (Sulzer et al., 1998). Several subsequent ultrastructural studies have found sparse TH+/VGLUT2+ varicosities in rat (Bérubé-Carrière et al., 2009; Moss et al., 2011) and mouse striata (Bérubé-Carrière et al., 2012; Fortin et al., 2019). VMAT2 and VGLUT2 appear to be actively trafficked to different processes; VMAT2 overexpression does not reduce segregation, consistent with an active process that mediates spatial segregation (Zhang et al., 2015). DA neurons co-cultured with ventral striatal neurons demonstrated enhanced segregation of TH+ and VGLUT2+ varicosities, suggesting that target-dependent factors may influence VGluT2 expression and/or VGLUT2 localization (Fortin et al., 2019).
Although DA transients and cotransmitted GLU EPSCs elicited by optogenetic stimulation share similar release properties (Adrover et al., 2014), more recent functional studies support segregation of DA and GLU release. DA and GLU release by optogenetic stimulation deplete with different kinetics, are coupled to different types of presynaptic Ca2+ channels, and are differentially coupled to active zone proteins (adaptor protein 3, synaptic vesicle protein 2 and piccolo) (Silm et al., 2019). These findings are consistent with spatial segregation of DA and GLU SVs. However, studies in Drosophila demonstrate that a single VGLUT protein is sufficient to fill a SV with GLU (Daniels et al., 2006); thus, VGluT2 expression levels with a physiological impact may be below the detection threshold of some methods under certain conditions, e.g., immunohistochemistry (IHC) under electron microscopy. Ultimately, while low levels of VGLUT2 in VMAT2-containing SVs may mediate corelease, spatial segregation of DA and GLU release sites appears to be the predominant configuration in DA-GLU neurons.
What Are the Synaptic Functions of DA Neuron GLU Cotransmission?
Excitatory Synaptic Transmission
DA volume transmission — where DA is released at non-synaptic sites and diffuses to extra-synaptic receptors — signals on a slower time frame than direct synaptic connections (Sulzer et al., 2016). In contrast, GLU cotransmission via direct synaptic connections operates on a faster time frame and conveys a discrete signal (though GLU can also act on a slower time scale at extrasynaptic sites via mGluRs). In NAc m-shell ChIs, optogenetic stimulation of DA neuron axons elicits a burst mediated by iGluRs, followed by a post-burst hyperpolarization mediated mainly by small conductance Ca2+-dependent K+ channels and partially by D2 receptors (Chuhma et al., 2014). In lateral dorsal striatum ChIs, the response is a pause mediated by D2 receptors followed by excitation mediated by mGluR1 and D1/5 receptors coupling to transient receptor potential channels 3 and 7 (Cai and Ford, 2018; Chuhma et al., 2018).
Dopamine neuron GLU EPSCs are subject to frequency-dependent DA modulation. In the NAc m-shell, DA causes counteracting D2-mediated presynaptic inhibition and D1-mediated postsynaptic facilitation through closure of K+ channels on GLU cotransmission. At tonic-firing frequencies D2-mediated presynaptic inhibition dominates and GLU responses are attenuated, while at burst-firing frequencies postsynaptic facilitation dominates and the GLU responses are enhanced (Chuhma et al., 2009). DA neuron GLU EPSPs are attenuated subsequent to low-dose amphetamine, whereas high-dose amphetamine attenuates fast DA transmission as well (Chuhma et al., 2014).
Circuit-Level Effects
In the striatum, DA neurons make GLU connections preferentially to ChIs in the NAc m-shell and lateral dorsal striatum (Chuhma et al., 2014, 2018; Cai and Ford, 2018). ChIs are distributed throughout the striatum with widespread axonal arborizations. Most striatal neurons express acetylcholine receptors, particularly on their presynaptic terminals (Lim et al., 2014; Ztaou and Amalric, 2019). This points to widespread effects of DA neuron GLU cotransmission on striatal circuits via modulation of ChI activity (Stocco, 2012; Zhang and Cragg, 2017; Assous and Tepper, 2019). DA neuron GLU cotransmission can also exert positive feedback on DA neuron transmission via presynaptic nicotinic acetylcholine receptors (nAChRs) (Figure 5). In the m-shell, DA neuron GLU cotransmission activates ChIs directly with short latency (Chuhma et al., 2014; Mingote et al., 2017), potentially inducing synchronized activation of ChIs (Mingote et al., 2019). Increased ChI activity may then activate nAChRs on DA neuron terminals resulting in an increase in DA release (Cachope et al., 2012; Threlfell et al., 2012), forming a positive feedback loop. Lack of DA neuron GLU cotransmission in DATCre;VGluT2flox/flox cKO mice disrupts this loop; it also reduces DA release in the striatum, in line with disrupted vesicular synergy (Stuber et al., 2010; Alsiö et al., 2011).
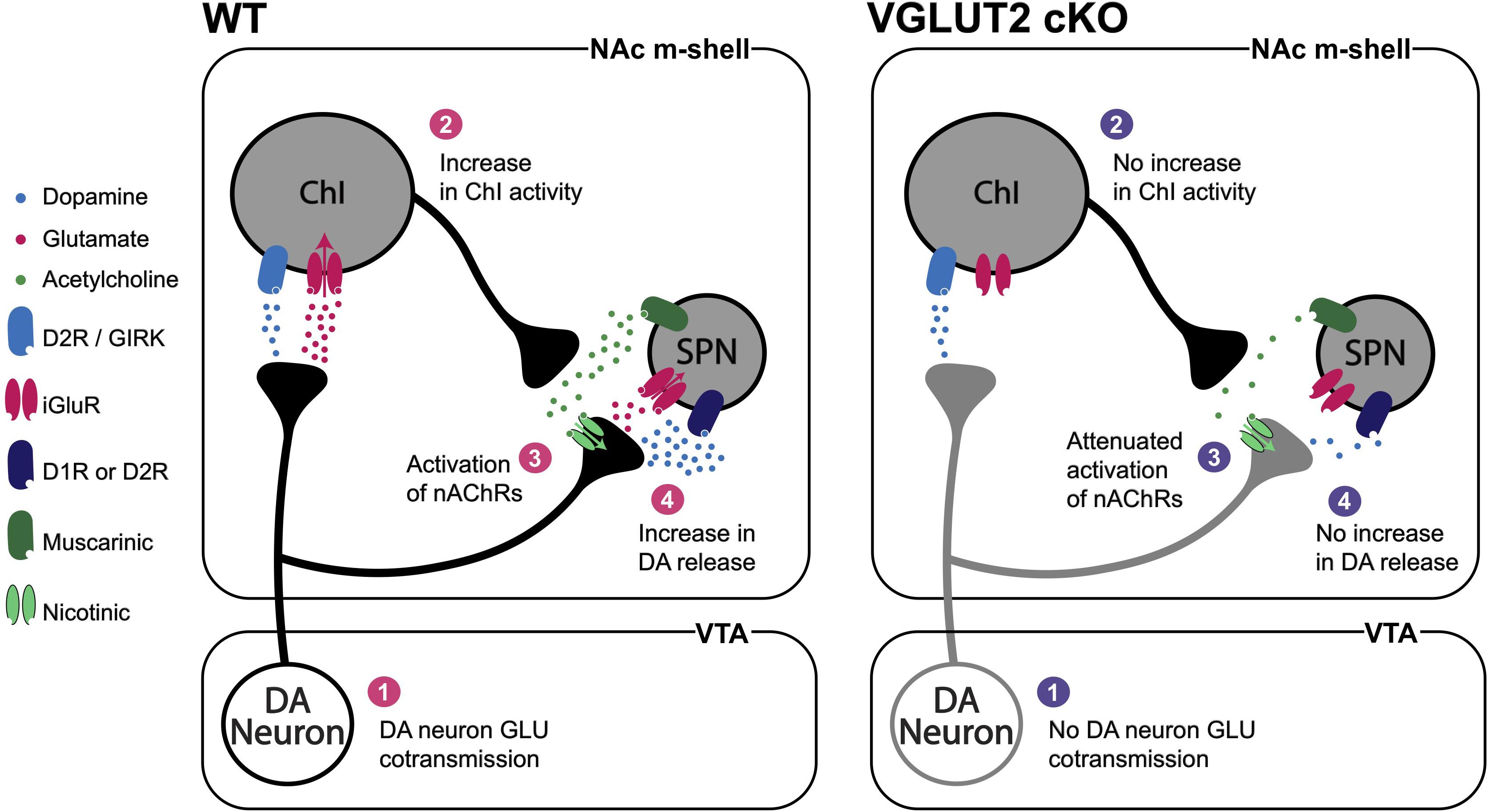
Figure 5. DA neuron GLU cotransmission circuit effects. DA neuron GLU cotransmission (1) increases ChI activity (2) and acetylcholine release that activates nAChRs on DA neuron terminals (3) to increase DA release (4). In DA-neuron-specific VGluT2 cKO mice (1) there would be no increase in ChI activity, (2) attenuated activation of nAChRs (3) and reduced DA release (4). Vesicular synergy is not shown in this figure.
Dopamine neuron GLU cotransmission appears to regulate activity in multiple brain regions. DATCre;VGluT2flox/flox cKO mice have widespread alterations in immediate early genes c-fos and Nur77 in striatal subregions (Alsiö et al., 2011). Circuit-level alterations are also shown by an increase in AMPA/NMDA ratio in D1-receptor expressing SPNs in the NAc in tamoxifen-inducible DA-neuron-specific VGluT2 cKO (DATCre–ERT2;VGluT2flox/flox) mice, in which VGluT2 is conditionally excised from DA neurons in adulthood (Papathanou et al., 2018). In acute hippocampal slices, local field potential recordings revealed THIRES–Cre;VGluT2flox/flox cKO mice had fewer kainate-induced gamma oscillations and more epileptic activity than controls (Nordenankar et al., 2015); suggesting network-wide effects that may alter excitation/inhibition balance involving multiple brain regions.
What Are the Developmental Roles of DA Neuron GLU Cotransmission?
Embryonic Differentiation of DA Neurons and Development of VGluT2 Expression
During development most, if not all, DA neurons in the ventral midbrain express VGluT2, and a substantial portion continue to do so in adulthood (Wallén-Mackenzie et al., 2006; Dal Bo et al., 2008; Birgner et al., 2010; Fortin et al., 2012; Trudeau et al., 2014; Steinkellner et al., 2018; Bimpisidis and Wallén-Mackenzie, 2019; Dumas and Wallén-Mackenzie, 2019; Kouwenhoven et al., 2020; Table 1). Embryonic cell-fate labeling shows that >90% of DA neurons in the VTA and SN in adult mice expressed VGluT2 during development (Steinkellner et al., 2018; Kouwenhoven et al., 2020; Fougère et al., 2021).
In the medial VTA, where most DA-GLU neurons are located, DA neuron differentiation is directed by zinc finger transcription factor Gli2 (Kabanova et al., 2015). Gli2 mediates sonic hedgehog (Shh)-induced formation of DA neuron progenitor cells around embryonic day (E) 9. Conditional knockout (cKO) of Gli2 during this period in En1Cre/+;Gli2zfd/flox (termed Gli2Δ Mb>E9.0) cKO mice reduced the number of TH+ neurons by about 50% and TH+/VGLUT2+ neurons by about 70%, while the number of VGLUT2-only (i.e., TH–/VGLUT2+) neurons is unaffected (Kabanova et al., 2015). The decrease in TH+/VGLUT2+ DA neurons leads to a significant reduction of DA neuron GLU cotransmission to inhibitory interneurons in the PFC (Kabanova et al., 2015). Remarkably, Shh continues to provide trophic support to DA neurons in adulthood, as DA-neuron-specific Shh cKO (ShhnLZC/C;DATCre) accelerates DA neuron degeneration via failure of reciprocal trophic support (Gonzalez-Reyes et al., 2012).
In addition to being the vesicular glutamate transporter subtype preferentially expressed in DA neurons, VGluT2 is also the predominant subtype expressed in the embryonic brain (Boulland et al., 2004). VGluT2 null mice (VGluT2flox/flox;PCre) die shortly after birth due to the role of VGLUT2 in brainstem respiratory central pattern generators (Moechars et al., 2006; Wallén-Mackenzie et al., 2006). DA-neuron-specific VGluT2 cKO, driven by either DATCre or THCre transgenes in VGluT2flox/flox mice, is not lethal. However, the VGluT2 cKO affects DA neuron survival, maturation (including projections and formation of connections), and response to injury (Dal Bo et al., 2008; Bérubé-Carrière et al., 2009; Fortin et al., 2012; Shen et al., 2018; Steinkellner et al., 2018; Kouwenhoven et al., 2020). Since VGluT2 expression in nascent DA neurons is detected around E10, prior to expression of DA neuron markers (Dumas and Wallén-Mackenzie, 2019), even DATCre;VGluT2flox/flox and THCre;VGluT2flox/flox cKO mice likely express VGluT2 in DA neurons transiently. DAT expression starts at E14 and Cre-dependent recombination in DATCre mice is clearly observed at E17 (Bäckman et al., 2006), indicating that Cre-dependent VGluT2 excision occurs in late embryonic life. TH expression begins before this, as shown by TH+/VGLUT2+ neurons detected during E11.5–12.5 (Birgner et al., 2010; Nordenankar et al., 2015). Thus, it is important to note that findings from studies using THCre;VGluT2flox/flox cKO mice represent an earlier loss of VGLUT2 in DA neurons during embryonic development whereas DATCre;VGluT2flox/flox cKO mice reflect the loss of VGLUT2 function in DA neurons in the early postnatal period.
Regulation of Maturation and Growth
Dopamine neurons in DATCre;VGluT2flox/flox cKO mice have smaller soma size, shorter axonal lengths and reduced neurite complexity (Fortin et al., 2012). Although there were no apparent changes in the configuration of the medial forebrain bundle, the total number of TH+ neurons are reduced by ∼25% in the VTA and ∼20% in the SNc (Fortin et al., 2012). There are significant reductions in TH+ axon density and DA release, measured with cyclic voltammetry, in the NAc shell, but not in the NAc core (Fortin et al., 2012), consistent with the more prominent GLU cotransmission in the NAc shell. Expression of DA receptors was increased in both the dorsal and ventral striatum in DATCre;VGluT2flox/flox cKO mice, further suggesting a role for DA neuron GLU cotransmission in the establishment of meso-striatal projections (Alsiö et al., 2011).
In co-cultures of DA and GABA neurons, only ∼20% of TH+ neurons coexpress VGluT2, whereas in pure DA neuron cultures ∼50% of TH+ neurons coexpress VGluT2 (Mendez et al., 2008b). GABA did not reduce TH+/VGLUT2+ co-labeling in DA neuron culture, suggesting that a contact-dependent mechanism is required for downregulation of VGluT2 expression (Mendez et al., 2008b). Quinolinic acid lesions of the medial dorsal striatum led to increased VGluT2 expression in midbrain DA neurons (Mendez et al., 2008b). This could be a consequence of lost neurotrophic support from postsynaptic targets, or lack of afferent inputs to midbrain DA neurons. A more recent study showed that co-culture of DA neurons with dorsal striatal neurons reduced VGluT2 mRNA expression, whereas co-culture of DA neurons with ventral striatal neurons increased VGluT2 expression (Fortin et al., 2019). These findings suggest further that striatal neurons exert trophic effects on VGluT2 expression in midbrain DA neurons. Overall, both pre and postsynaptic mechanisms appear to be important for growth and survival of DA-GLU neurons.
How Are DA-GLU Neurons Affected by DA Neuron Toxins?
DA-GLU neurons appear to be less vulnerable to the DA neuron toxins 6-hydroxydopamine (6-OHDA) and 1-methyl-4-phenyl-1,2,3,6-tetrahydropyridine (MPTP) (Table 2). Intraventricular 6-OHDA injections in juvenile and adult rats increase the proportion of TH+/VGLUT2+ neurons among TH+ neurons in the VTA (Dal Bo et al., 2008; Bérubé-Carrière et al., 2009). 6-OHDA injections in the dorsal striatum increase the proportion of TH+/VGLUT2+ neurons in the SN (Steinkellner et al., 2018), and TH+/VGLUT2+ axon terminals in the NAc (Bérubé-Carrière et al., 2009). This increased ratio of TH+/VGLUT2+ neurons in ventral midbrain DA neurons after toxin exposure could be due to re-expression of VGluT2 in the surviving TH+/VGLUT2– neurons (i.e., ‘neurotransmitter switching,’ see Spitzer, 2015 for review), or reduced susceptibility of TH+/VGLUT2+ neurons. Thus, an increase of TH+/VGLUT2+ projections in the striatum could be due to new projections of VTA TH+/VGLUT2+ neurons compensating for the loss of SN TH+/VGLUT2+ neurons, or SN TH+/VGLUT2– neurons switching to TH+/VGLUT2+, resulting in an increase in the number of DA-GLU neurons. In mouse SN DA neuron culture, 1-methyl-4-phenylpyridinium (MPP+) exposure increases VGluT2 copy number per cell, while TH copy number per cell is reduced (Kouwenhoven et al., 2020). This suggests that cellular stress drives neurotransmitter switching and similar mechanisms may be activated in surviving DA neurons after toxin exposure.
SN DA neurons in DATIRES–Cre/+;VGluT2flox/flox cKO mice are more vulnerable to 6-OHDA and MPTP than those in DATIRES–Cre/+;VGluT2flox/+ conditional heterozygous (cHET) control mice (Shen et al., 2018; Steinkellner et al., 2018; Kouwenhoven et al., 2020). DATIRES–Cre/+;VGluT2flox/flox cKO mice, compared to cHET mice, have reduced levels of brain derived neurotrophic factor and its receptor TrkB in VTA and SN DA neurons, and are more vulnerable to MPTP (Shen et al., 2018). Viral rescue of VGluT2 expression with an AAV-DIO-VGluT2 vector in DA neurons of DATIRES–Cre;VGluT2flox/flox cKO mice recovered brain derived neurotrophic factor/TrkB expression and thereby attenuated MPTP-induced DA neuron toxicity. MPTP-induced expression of proapoptotic marker BAX in the midbrain is not different between DATIRES–Cre/+;VGluT2flox/flox cKO mice and cHET control mice, suggesting that a neuroprotective function of VGLUT2 is not related to production of proapoptotic/antiapoptotic factors (Shen et al., 2018). Thus, VGluT2 expression appears to be neuroprotective via neurotrophic signaling rather than an anti-apoptotic mechanism. However, VGLUT2 appears not to have a purely protective effect as overexpression of VGluT2 is neurotoxic in both flies and mice, leading to upregulation of markers of apoptosis and inflammatory gliosis (Steinkellner et al., 2018).
What Are the Behavioral Roles of DA Neuron GLU Cotransmission?
In this section we have parsed pre-clinical behavioral findings from studies of DA neuron GLU cotransmission along the Research Domain Criteria delineated by the National Institute of Mental Health (Table 3–7). The Research Domain Criteria were constructed to provide a research framework for mental disorders based on multiple levels, from genomics to behaviors, organized around major divisions called domains and subdivisions called constructs, meant to encapsulate different aspects that model human functioning in areas related to emotion, cognition and behavior (Insel, 2014). Using this format facilitates comparisons across studies and species.
Positive Valence Systems
Within the Positive Valence Systems domain, DA neuron GLU cotransmission affects two constructs: reward-responsiveness (Table 3) and reward learning (Table 4). Disruption of DA neuron GLU cotransmission in DATCre;VGluT2flox/flox cKO mice blunts acute responses to psychostimulants (Birgner et al., 2010; Hnasko et al., 2010; Fortin et al., 2012; Steinkellner et al., 2018). Although DATCre;VGluT2flox/flox cKO mice were initially hyporesponsive to doses of cocaine, they still showed sensitization (Hnasko et al., 2010) — a measure of increasing reward-responsiveness to repeated exposures to the same dose, which models pathologic incentive motivation in addiction (Robinson and Berridge, 2008). Conversely, cHET of GLU recycling enzyme glutaminase (GLS1) in DA neurons did not affect acute responses to amphetamine, but did diminish sensitization and blunted responses to subsequent challenge doses (Mingote et al., 2017). Even when initial responses are intact, impaired DA neuron GLU cotransmission still disrupts reward responsiveness. Since reduced GLU cotransmission does not affect motor control or negative valence systems (see below), the blunted reward responsiveness is not secondary to motor or emotional impairment.
Cocaine-seeking induced by drug-paired cues and cocaine intravenous self-administration are enhanced in DATCre;VGluT2flox/flox in cKO mice (Alsiö et al., 2011). Operant conditioning for high-sucrose food is also enhanced in DATCre;VGluT2flox/flox cKO mice, showing that DA neuron GLU cotransmission modulates intensity of responses not only to psychostimulants, but also to natural rewards (Alsiö et al., 2011). DATIRES–Cre;VGluT2flox/flox cKO mice showed reduced progressive intracranial optogenetic self-stimulation of VTA TH+ neurons, supporting the hypothesis that DA neuron GLU cotransmission regulates the magnitude of operant behaviors (Wang et al., 2017). Although GLU released from DA neurons may not be critical for the acquisition of conditioned reinforcement, its loss nonetheless affects positive valence systems. For example, VGluT2Cre;THflox/flox cKO mice, which have TH excised from VGLUT2+ DA neurons (i.e., DA neurons with blunted DA transmission but intact GLU cotransmission), optogenetic stimulation of VGluT2Cre/AAV–DIO–ChR2 VTA neurons was sufficient to reinforce behavior (Zell et al., 2020). Although this study did not discriminate contributions of GLU-only (non-DAergic) neurons and GLU cotransmission from DA-GLU neurons, GLU cotransmission from DA-GLU neurons presumably contributes to DA-independent positive reinforcement.
The only DA-neuron-specific VGluT2 cKO study without an impaired response to acute psychostimulants used a THIRES–Cre transgene instead of a DATCre or DATIRES–Cre transgene to establish the DA-neuron-specific VGluT2 cKO (Nordenankar et al., 2015). Subsequent reviews have cautioned about comparisons between THCre and DATCre induced conditional gene expression (Pupe and Wallén-Mackenzie, 2015; Stuber et al., 2015; Lammel et al., 2015; Buck et al., 2020; Fischer et al., 2020). Briefly, THCre mice cause more developmental effects than DATCre mice, because TH expression begins earlier in development than DAT (see above), and is more widespread and ectopic (i.e., neurons that are positive for TH mRNA but not TH protein) (Di Porzio et al., 1990). Although, DATCre mice also show off-target recombination in a subset of DAT-negative neurons in particular limbic areas (Papathanou et al., 2019). Also, because TH is part of the synthetic pathway of norepinephrine, norepinephrine neurons will be affected in THCre mice as well. It should be noted that intensity of responses to psychostimulants can also be affected by background strain, e.g., C57BL/6J mice show greater responses than 129S2/SvHsd mice (Chen et al., 2007). Although the background strain issue is partly mitigated by use of littermate controls, difference in background strains must be considered when comparing studies (Crawley et al., 1997; Bailey et al., 2006; Linder, 2006, 2001; Yoshiki and Moriwaki, 2006).
Behavioral studies using cKO mice with DAT or TH promoters to drive Cre recombinase to excise floxed VGluT2 from DA neurons must be interpreted with caution, because effects seen in adulthood can be caused by developmental derangements and/or effects of diminished GLU cotransmission in adulthood. Both DAT and TH are expressed during embryogenesis (Di Porzio et al., 1990; Bäckman et al., 2006), thus, DATCre;VGluT2flox/flox and THCre;VGluT2flox/flox cKO mice lose VGLUT2 function in DA neurons in early life (see above). For example, DATCre;VGluT2flox/flox cKO mice show impaired responses to psychostimulants, and have reduced TH+ neuron numbers, thus the impaired responses to psychostimulants could be due to lack of DA neuron GLU cotransmission in adulthood and/or reduced TH+ neurons (Birgner et al., 2010; Fortin et al., 2012). Of note, DATIRES–Cre;Gls1flox/+ cHET mice also have impaired responses to psychostimulants, despite unaffected DA neuron number or DA release (Mingote et al., 2017). To further circumvent issues related to developmental alterations, Papathanou and colleagues knocked out VGluT2 from DA neurons in adulthood using tamoxifen-inducible DA-neuron-specific VGluT2 cKO (DATCre–ERT2;VGluT2flox/flox) mice (Papathanou et al., 2018). Control DATCre;VGluT2flox/flox cKO mice showed blunted sensitization to cocaine and amphetamine, in agreement with previous studies (Hnasko et al., 2010; Fortin et al., 2012; Mingote et al., 2017), whereas DATCre–ERT2;VGluT2flox/flox cKO mice given tamoxifen at 8–9 weeks of age did not show psychostimulant-induced hyperlocomotion (Papathanou et al., 2018), thus demonstrating that DA-neuron-specific VGluT2 expression in adulthood is necessary for full psychostimulant responsivity. A potential confound is that all mice receiving tamoxifen showed blunted responses to psychostimulants – regardless of genotype (i.e., both DATCre–ERT2;VGluT2flox/flox cKO and DATCre–ERT2;VGluT2flox/+ cHET). These blunted responses to psychostimulants could be due to tamoxifen itself, which impairs locomotor responses to amphetamine, even if tamoxifen is not given on the day of locomotor testing (Mikelman et al., 2018). Nonetheless, this suggests that DA neuron GLU cotransmission later in life still mediates psychostimulant responses, but perhaps less so than estimated from observations in DATCre;VGluT2flox/flox and THCre;VGluT2flox/flox cKO mice.
Cognitive Control
Roles for DA neuron GLU cotransmission in the cognitive control domain have been studied with latent inhibition and tests of spatial working memory (Table 5). Latent inhibition is a testable cognitive behavior with clinical relevance to schizophrenia, observed in both rodent models and in clinical studies (Gaisler-Salomon et al., 2009; Weiner and Arad, 2009). Latent inhibition assesses how pre-exposure to a conditioned stimulus (CS; typically, a tone) prevents formation of an association between that CS and an unconditioned stimulus (US; typically, a shock). In mice, testing for latent inhibition has three phases. First, the CS-only pre-exposure phase, all mice are placed in a chamber but only the experimental group is exposed several times to a tone, whereas the control group is not. Second, the CS-US pairing phase, both groups of mice are placed in the testing chamber and receive a footshock paired with the tone. Last, the CS-only test phase, all mice are exposed to the tone and freezing behaviors are measured. Sufficient pre-exposure to the tone reduces freezing during the CS-only test phase, despite the temporal delay between pre-exposure and test (latent inhibition). DATIRES–Cre;Gls1flox/+ cHET mice showed an enhanced latent inhibition, i.e., an enhanced ability to discriminate cue saliency (Mingote et al., 2017), suggesting that abrogated GLU release from DA neurons facilitates cognitive function.
THIRES–Cre;VGluT2flox/flox cKO mice have impaired learning a radial arm maze, a task used to assess spatial working memory (Nordenankar et al., 2015). Although THIRES–Cre;VGluT2flox/flox cKO mice were still able to learn the task, they took significantly longer and made more reference memory errors, but not working memory errors, than THIRES–Cre;VGluT2flox/+ cHET controls (Nordenankar et al., 2015). Reference memory errors are thought to reflect hippocampal deficits, whereas working memory errors reflect impairments in frontal cortical networks (Yoon et al., 2008). Lack of DA neuron GLU cotransmission appears to impair hippocampal reference memory, suggesting that intact cotransmission may facilitate spatial reasoning beyond simply improving attention. Gli2Δ Mb>E9.0 cKO also results in a substantial reduction in medial VTA TH+/VGLUT2+ neurons and increases perseverative behavior on the five-choice serial reaction time task, suggesting impaired visuospatial attention and motor impulsivity (Kabanova et al., 2015). However, the contribution of mesocortical GLU-only neurons, which are also reduced by Gli2 cKO in DA neurons, cannot be excluded. Again, since reduced GLU cotransmission does not appear to affect motor control or negative valence systems (see below), the effects on cognitive control are not secondary to motor or emotional impairment.
Negative Valence Systems
Behaviors related to acute and sustained threats are largely unaffected by impaired DA neuron GLU cotransmission (Birgner et al., 2010; Fortin et al., 2012; Nordenankar et al., 2015; Mingote et al., 2017; Table 6). Standard tests of anxiety, such as the elevated-plus maze and open field test, are mostly unaffected in DATCre;VGluT2flox/flox cKO mice and THIRES–Cre;VGluT2flox/flox cKO mice (Birgner et al., 2010; Nordenankar et al., 2015); however, after MPTP administration, DATIRES–Cre;VGluT2flox/flox showed increased anxiety on the elevated-plus maze (Shen et al., 2018). Similarly, freezing in a fear-conditioning paradigm did not differ in DATIRES–Cre;Gls1flox/+ cHET mice (Mingote et al., 2017). Performance on the forced-swim test, a measure of a depressive-like phenotype, is largely unchanged in DATCre;VGluT2flox/flox cKO mice and THIRES–Cre;VGluT2flox/flox cKO mice (Birgner et al., 2010; Nordenankar et al., 2015), though one study showed a decreased latency to immobilization on day one but not on day two in DATCre;VGluT2flox/flox cKO mice (Fortin et al., 2012).
Motor Control
Loss or decrease of DA neuron GLU cotransmission, whether in DATCre;VGluT2flox/flox cKO mice, THIRES–Cre;VGluT2flox/flox cKO mice or DATIRES–Cre;Gls1flox/+ cHET mice, does not alter basic motor and arousal function (Birgner et al., 2010; Hnasko et al., 2010; Fortin et al., 2012; Nordenankar et al., 2015; Mingote et al., 2017), with few exceptions in one study using DATIRES–Cre/+;VGluT2flox/flox cKO mice (Steinkellner et al., 2018; Table 7). Gross locomotor function is normal in DATCre;VGluT2flox/flox cKO mice and THIRES–Cre;VGluT2flox/flox cKO mice (Hnasko et al., 2010; Fortin et al., 2012; Nordenankar et al., 2015). Motor coordination tested with rotarod is normal in studies using both sexes of DATCre;VGluT2flox/flox cKO mice (Birgner et al., 2010; Hnasko et al., 2010), although one study using only DATCre;VGluT2flox/flox cKO male mice showed impairment (Fortin et al., 2012). It remains unresolved whether this reflects variation between studies or differential effects between males and females, as no female-only study has been performed. MPTP-induced motor impairments were more pronounced in DATIRES–Cre;VGluT2flox/flox cKO mice, but restored by VGluT2 viral rescue (Shen et al., 2018). The lack of change in motor control could be related to lesser DA neuron GLU cotransmission in the dorsal striatum, which is more associated with motor learning.
Does DA Neuron GLU Cotransmission Have a Role in Human Disorders?
Understanding behavioral roles of DA-GLU neurons offers potential insight into human neuropsychiatric disorders. Interactions between DA and GLU figure prominently in neuropsychiatric disorders, and DA neuron GLU cotransmission is one of the points where DA and GLU interact.
Substance Use Disorders/Addiction
In humans, post-mortem studies of cigarette smokers have demonstrated increased VTA VGLUT2 (human gene) expression compared to healthy controls (Flatscher-Bader et al., 2008). Given that microarrays were performed specifically in the VTA, even though TH-VGLUT2 double-staining was not performed, it is likely some of the VGLUT2 expressing neurons were DA neurons, suggesting that either increased cotransmission may be a risk factor for smoking or that smoking may alter VGLUT2 expression in DA neurons. In mice, neonatal nicotine exposure increases numbers of DA-GLU neurons and nicotine preference in adulthood (Romoli et al., 2019). Selectively targeting DA neuron GLU cotransmission may thus serve as a potential treatment for addiction (Bimpisidis and Wallén-Mackenzie, 2019), especially psychostimulant use disorders perhaps by facilitating behavioral switching (Mingote et al., 2019). Further discussion about DA-GLU neurons and addiction is found in recent reviews (Trudeau et al., 2014; Steinkellner et al., 2018; Bimpisidis and Wallén-Mackenzie, 2019; Buck et al., 2020; Fischer et al., 2020).
Psychotic Disorders
Both DA and GLU are implicated in the patho-etiology of schizophrenia by findings ranging from psychopharmacology, post-mortem analyses and in vivo brain imaging (for review see Howes et al., 2015). DA neuron GLU cotransmission serves as one potential point of confluence of DA and GLU actions (Chuhma et al., 2017).
One specific role of DA-GLU cotransmission is perhaps best demonstrated in studies of latent inhibition, which models cognitive impairments in schizophrenia, as well as in animal models (Weiner and Arad, 2009). Humans at high-risk for developing psychosis demonstrate deficits in latent inhibition, suggesting it is a cognitive marker of psychotic propensity, rather than a secondary effect of medication or a consequence of chronic schizophrenia (Kraus et al., 2016). As mentioned above, potentiation of latent inhibition in DA neuron DATIRES–Cre;Gls1flox/+ cHET mice (Mingote et al., 2017) emphasizes the therapeutic potential of reducing DA neuron GLU cotransmission.
Parkinson Disease
The main motor symptoms of Parkinson Disease (PD) are primarily due to the loss of nigrostriatal DA neurons. A recent study found that following partial loss of DA inputs, DA-driven inhibition of cholinergic activity in the dorsomedial striatum is preserved due to reduced DA reuptake, while GLU co-release evoked excitation in the dorsolateral striatum is lost due to a downregulation of mGluR1 (Cai et al., 2021). Altered DA-acetylcholine interactions have been hypothesized to underpin some of the symptoms of PD (Ztaou and Amalric, 2019). Since DA neuron GLU cotransmission regulates ChI activity, elucidating mechanisms of this regulation may help delineate PD pathophysiology and therapeutics.
One of the most promising treatments for PD is stem cell implantation (Widner et al., 1992; Mendez et al., 2002, 2008a; Wijeyekoon and Barker, 2009). For successful implantation, it is crucial to choose DA neurons in the appropriate developmental stage to survive and form connections (Lindvall, 2012), which may benefit from appropriate VGluT2 expression levels. For example, wildtype VGluT2 expression appears to be neuroprotective to DA neurons in PD mouse models (Dal Bo et al., 2008; Bérubé-Carrière et al., 2009; Shen et al., 2018; Steinkellner et al., 2018; Kouwenhoven et al., 2020), though VGluT2 overexpression appears to be neurotoxic to DA neurons (Steinkellner et al., 2018). Thus, determining a specific range of appropriate VGluT2 expression levels to optimize survival may be an important consideration in transplantation protocols to treat PD.
Potential Directions for Circuit-Based Pharmacotherapy
Given its involvement in circuitry underlying various neuropsychiatric disorders — ranging from schizophrenia, addiction, to PD — DA neuron GLU cotransmission is a considerable target of treatment for neuropsychiatric disorders. Refined molecular genetic manipulations can target discrete DA neuron subtypes, opening up new avenues for investigation and serving as proof-of-principle for future treatment of neuropsychiatric disorders.
One such approach is Genetic Pharmacotherapy, which is defined as the use of genetic interventions in mouse models to elucidate potential drug targets prior to the development of specific ligands (Gellman et al., 2011). This strategy enables the evaluation of therapeutic potential for target gene modification without costly and time-consuming development of specific ligands that may lack regional specificity and face issues regarding blood-brain barrier permeability. Genetic Pharmacotherapy achieves region-specific functional modulation by using molecular genetic techniques, such as conditional gene knockouts, to target neurons that express specific markers. This approach has already shown DA neuron GLU cotransmission as a viable target in schizophrenia treatment; DA neuron specific reduction of the GLU recycling enzyme GLS1 affects behaviors relevant to schizophrenia (Mingote et al., 2015b, 2017).
Furthermore, preclinical findings of neural function are applied to clinical trials using gene therapy with non-replicative, non-toxic viral vectors (for review see Lykken et al., 2018). Gene therapy requires characterization of specific circuits impacting a neuropsychiatric disorder, rather than pharmacologic targeting of specific, but widely distributed, cell-signaling receptors (Gordon, 2016). Additionally, because gene therapy can be brain-region specific, and even cell-type specific, it would presumably have less off-target effects compared to oral medications. DA neuron GLU cotransmission is an example of how a genetically distinct neuronal subpopulation affects phenotypes relevant to neuropsychiatric disorders, thus serving as a target for treatment development.
Conclusion
Dopamine neurons capable of GLU cotransmission serve as an example of how a specific subset of neurons within a diverse neuronal population can have distinct functions. As the gap between bench and bedside narrows and therapeutic options widen, e.g., non-pharmacological interventions such as gene therapy with intersectional control, DA neuron GLU cotransmission may be targeted for treatment of neuropsychiatric disorders.
Author Contributions
DE, SR, and NC: concept, design, and writing. SR and NC: supervision. DE: literature research. DE, LM, SM, LY, SZ, VV, SR, and NC: analysis, interpretation, and critical review. All authors agreed to be accountable for the content of the work.
Funding
This work was supported by the National Institute on Drug Abuse R01 DA038966 and National Institute of Mental Health R01 MH117128 and T32 MH018870.
Conflict of Interest
The authors declare that the research was conducted in the absence of any commercial or financial relationships that could be construed as a potential conflict of interest.
Acknowledgments
Figures 1, 3, 4 were created with BioRender.com.
Abbreviations
6-OHDA, 6-hydroxydopamine; BLA, basolateral amygdala; CeA, central nucleus of the amygdala; ChI, cholinergic interneuron; cHET, conditional heterozygous; CingC, cingulate cortex; cKO, conditional knockout; CLi, central linear nucleus; CS, conditioned stimulus; DA, dopamine; DAT, dopamine transporter; E#, embryonic day; EGFP, enhanced green fluorescent protein; EntC, entorhinal cortex; EPSC, excitatory postsynaptic current; flox, floxed allele; FSI, fast-spiking interneuron; GLU, glutamate; Gls1, glutaminase 1; Hippo, hippocampus; IF, interfascicular nucleus; iGluR, ionotropic glutamate receptor; IHC, immunohistochemistry; IRES, internal ribosome entry site; ISH, in situ hybridization; mGluR, metabotropic glutamate receptor; MPP+, 1-methyl-4-phenyl pyridinium; MPTP, N-methyl -4-phenyl-1,2,3,6-tetrahydropyridine; m-shell, medial shell; nAChR, nicotinic acetylcholine receptor; NAc, nucleus accumbens; OT, olfactory tubercle; P#, postnatal day; PD, Parkinson disease; PBP, parabrachial pigmented nucleus; PFC, prefrontal cortex; PIF, parainterfascicular nucleus; PN, paranigral nucleus; RLi, rostral linear nucleus; sc RT-PCR, single cell reverse transcriptase polymerase chain reaction; SPN, spiny projection neuron; Shh, sonic hedgehog; SN, substantia nigra; SNc, substantia nigra pars compacta; SV, synaptic vesicle; TH, tyrosine hydroxylase; TH+/VGLUT+, TH and VGLUT2 double-labeling; US, unconditioned stimulus; VTA, ventral tegmental area; VGluT2, vesicular glutamate transporter 2 (rodent gene); VGLUT2, vesicular glutamate transporter 2 (human gene); VGLUT2, vesicular glutamate transporter 2 (protein); VMAT2, vesicular monoamine transporter 2; WT, wildtype.
References
Adrover, M. F., Shin, J. H., and Alvarez, V. A. (2014). Glutamate and dopamine transmission from midbrain dopamine neurons share similar release properties but are differentially affected by cocaine. J. Neurosci. 34, 3183–3192. doi: 10.1523/JNEUROSCI.4958-13.2014
Aguilar, J. I., Dunn, M., Mingote, S., Karam, C. S., Farino, Z. J., Sonders, M. S., et al. (2017). Neuronal depolarization drives increased dopamine synaptic vesicle loading via VGLUT. Neuron 95, 1074–1088. doi: 10.1016/j.neuron.2017.07.038
Alsiö, J., Nordenankar, K., Arvidsson, E., Birgner, C., Mahmoudi, S., Halbout, B., et al. (2011). Enhanced sucrose and cocaine self-administration and cue-induced drug seeking after loss of VGLUT2 in midbrain dopamine neurons in mice. J. Neurosci. 31, 12593–12603. doi: 10.1523/JNEUROSCI.2397-11.2011
Amilhon, B., Lepicard, E., Renoir, T., Mongeau, R., Popa, D., Poirel, O., et al. (2010). VGLUT3 (vesicular glutamate transporter type 3) contribution to the regulation of serotonergic transmission and anxiety. J. Neurosci. 30, 2198–2210. doi: 10.1523/JNEUROSCI.5196-09.2010
Assous, M., and Tepper, J. M. (2019). Excitatory extrinsic afferents to striatal interneurons and interactions with striatal microcircuitry. Eur. J. Neurosci. 49, 593–603. doi: 10.1111/ejn.13881
Bäckman, C. M., Malik, N., Zhang, Y., Shan, L., Grinberg, A., Hoffer, B. J., et al. (2006). Characterization of a mouse strain expressing Cre recombinase from the 3’ untranslated region of the dopamine transporter locus. Genesis 44, 383–390. doi: 10.1002/dvg.20228
Bailey, K. R., Rustay, N. R., and Crawley, J. N. (2006). Behavioral phenotyping of transgenic and knockout mice: practical concerns and potential pitfalls. ILAR J. 47, 124–131. doi: 10.1093/ilar.47.2.124
Bérubé-Carrière, N., Guay, G., Fortin, G. M., Kullander, K., Olson, L., and Wallén-Mackenzie, Å, et al. (2012). Ultrastructural characterization of the mesostriatal dopamine innervation in mice, including two mouse lines of conditional VGLUT2 knockout in dopamine neurons. Eur. J. Neurosci. 35, 527–538. doi: 10.1111/j.1460-9568.2012.07992.x
Bérubé-Carrière, N., Riad, M., Dal Bo, G., Lévesque, D., Trudeau, L.-E., and Descarries, L. (2009). The dual dopamine-glutamate phenotype of growing mesencephalic neurons regresses in mature rat brain. J. Comp. Neurol. 517, 873–891. doi: 10.1002/cne.22194
Bimpisidis, Z., and Wallén-Mackenzie, Å (2019). Neurocircuitry of reward and addiction: potential impact of dopamine-glutamate co-release as future target in substance use disorder. J. Clin. Med. 8:1887. doi: 10.3390/jcm8111887
Birgner, C., Nordenankar, K., Lundblad, M., Mendez, J. A., Smith, C., le Grevès, M., et al. (2010). VGLUT2 in dopamine neurons is required for psychostimulant-induced behavioral activation. Proc Natl Acad Sci USA 107, 389–394. doi: 10.1073/pnas.0910986107
Blakely, R. D., and Edwards, R. H. (2012). Vesicular and plasma membrane transporters for neurotransmitters. Cold Spring Harb. Perspect. Biol. 4:a005595. doi: 10.1101/cshperspect.a005595
Boulland, J.-L., Qureshi, T., Seal, R. P., Rafiki, A., Gundersen, V., Bergersen, L. H., et al. (2004). Expression of the vesicular glutamate transporters during development indicates the widespread corelease of multiple neurotransmitters. J. Comp. Neurol. 480, 264–280. doi: 10.1002/cne.20354
Buck, S. A., Torregrossa, M. M., Logan, R. W., and Freyberg, Z. (2020). Roles of dopamine and glutamate co-release in the nucleus accumbens in mediating the actions of drugs of abuse. FEBS J. 288, 1462–1474. doi: 10.1111/febs.15496
Cachope, R., Mateo, Y., Mathur, B. N., Irving, J., Wang, H.-L., Morales, M., et al. (2012). Selective activation of cholinergic interneurons enhances accumbal phasic dopamine release: setting the tone for reward processing. Cell Rep. 2, 33–41. doi: 10.1016/j.celrep.2012.05.011
Cai, Y., and Ford, C. P. (2018). Dopamine cells differentially regulate striatal cholinergic transmission across regions through corelease of dopamine and glutamate. Cell Rep. 25, 3148–3157. doi: 10.1016/j.celrep.2018.11.053
Cai, Y., Nielsen, B. E., Boxer, E. E., Aoto, J., and Ford, C. P. (2021). Loss of nigral excitation of cholinergic interneurons contributes to parkinsonian motor impairments. Neuron 109, 1137–1149. doi: 10.1016/j.neuron.2021.01.028
Chen, R., Zhang, M., Park, S., and Gnegy, M. E. (2007). C57BL/6J mice show greater amphetamine-induced locomotor activation and dopamine efflux in the striatum than 129S2/SvHsd mice. Pharmacol. Biochem. Behav. 87, 158–163. doi: 10.1016/j.pbb.2007.04.012
Chuhma, N. (2015). “Optogenetic Analysis of Striatal Connections to Determine Functional Connectomes,” in Optogenetics, eds H. Yawo, H. Kandori, and A. Koizumi (Tokyo: Springer), 265–277.
Chuhma, N. (2021). Functional connectome analysis of the striatum with optogenetics. Adv. Exp. Med. Biol. 1293, 417–428. doi: 10.1007/978-981-15-8763-4_27
Chuhma, N., Choi, W. Y., Mingote, S., and Rayport, S. (2009). Dopamine neuron glutamate cotransmission: frequency-dependent modulation in the mesoventromedial projection. Neuroscience 164, 1068–1083. doi: 10.1016/j.neuroscience.2009.08.057
Chuhma, N., Mingote, S., Kalmbach, A., Yetnikoff, L., and Rayport, S. (2017). Heterogeneity in dopamine neuron synaptic actions across the striatum and its relevance for schizophrenia. Biol. Psychiatry 81, 43–51. doi: 10.1016/j.biopsych.2016.07.002
Chuhma, N., Mingote, S., Moore, H., and Rayport, S. (2014). Dopamine neurons control striatal cholinergic neurons via regionally heterogeneous dopamine and glutamate signaling. Neuron 81, 901–912. doi: 10.1016/j.neuron.2013.12.027
Chuhma, N., Mingote, S., Yetnikoff, L., Kalmbach, A., Ma, T., Ztaou, S., et al. (2018). Dopamine neuron glutamate cotransmission evokes a delayed excitation in lateral dorsal striatal cholinergic interneurons. elife 7:e39786. doi: 10.7554/eLife.39786
Chuhma, N., Tanaka, K. F., Hen, R., and Rayport, S. (2011). Functional connectome of the striatal medium spiny neuron. J. Neurosci. 31, 1183–1192. doi: 10.1523/JNEUROSCI.3833-10.2011
Chuhma, N., Zhang, H., Masson, J., Zhuang, X., Sulzer, D., Hen, R., et al. (2004). Dopamine neurons mediate a fast excitatory signal via their glutamatergic synapses. J. Neurosci. 24, 972–981. doi: 10.1523/JNEUROSCI.4317-03.2004
Cidon, S., and Sihra, T. S. (1989). Characterization of a H+-ATPase in rat brain synaptic vesicles. Coupling to L-glutamate transport. J. Biol. Chem. 264, 8281–8288.
Crawley, J. N., Belknap, J. K., Collins, A., Crabbe, J. C., Frankel, W., Henderson, N., et al. (1997). Behavioral phenotypes of inbred mouse strains: implications and recommendations for molecular studies. Psychopharmacology 132, 107–124. doi: 10.1007/s002130050327
Dal Bo, G., Bérubé-Carrière, N., Mendez, J. A., Leo, D., Riad, M., Descarries, L., et al. (2008). Enhanced glutamatergic phenotype of mesencephalic dopamine neurons after neonatal 6-hydroxydopamine lesion. Neuroscience 156, 59–70. doi: 10.1016/j.neuroscience.2008.07.032
Dal Bo, G., St-Gelais, F., Danik, M., Williams, S., Cotton, M., and Trudeau, L.-E. (2004). Dopamine neurons in culture express VGLUT2 explaining their capacity to release glutamate at synapses in addition to dopamine. J. Neurochem. 88, 1398–1405. doi: 10.1046/j.1471-4159.2003.02277.x
Daniels, R. W., Collins, C. A., Chen, K., Gelfand, M. V., Featherstone, D. E., and DiAntonio, A. (2006). A single vesicular glutamate transporter is sufficient to fill a synaptic vesicle. Neuron 49, 11–16. doi: 10.1016/j.neuron.2005.11.032
Di Porzio, U., Zuddas, A., Cosenza-Murphy, D. B., and Barker, J. L. (1990). Early appearance of tyrosine hydroxylase immunoreactive cells in the mesencephalon of mouse embryos. Int. J. Dev. Neurosci. 8, 523–532. doi: 10.1016/0736-5748(90)90044-3
Dumas, S., and Wallén-Mackenzie, Å (2019). Developmental co-expression of Vglut2 and Nurr1 in a mes-di-encephalic continuum preceeds dopamine and glutamate neuron specification. Front. Cell Dev. Biol. 7:307. doi: 10.3389/fcell.2019.00307
El Mestikawy, S., Wallén-Mackenzie, A., Fortin, G. M., Descarries, L., and Trudeau, L.-E. (2011). From glutamate co-release to vesicular synergy: vesicular glutamate transporters. Nat. Rev. Neurosci. 12, 204–216. doi: 10.1038/nrn2969
Eskenazi, D., Chuhma, N., Mingote, S., Ztaou, S., and Rayport, S. (2019). “Functional Connectome Mapping,” in Compendium of In Vivo Monitoring in Real-Time Molecular Neuroscience. Volume 3: Probing Brain Function, Disease and Injury with Enhanced Optical and Electrochemical Sensors, eds G. S. Wilson and A. C. Michael (Singapore: World Scientific Conpany), 49–71.
Fenno, L. E., Mattis, J., Ramakrishnan, C., Hyun, M., Lee, S. Y., He, M., et al. (2014). Targeting cells with single vectors using multiple-feature Boolean logic. Nat. Methods 11, 763–772. doi: 10.1038/nmeth.2996
Fenno, L. E., Ramakrishnan, C., Kim, Y. S., Evans, K. E., Lo, M., Vesuna, S., et al. (2020). Comprehensive dual- and triple-feature intersectional single-vector delivery of diverse functional payloads to cells of behaving mammals. Neuron 107, 836–853. doi: 10.1016/j.neuron.2020.06.003
Fischer, K. D., Knackstedt, L. A., and Rosenberg, P. A. (2020). Glutamate homeostasis and dopamine signaling: implications for psychostimulant addiction behavior. Neurochem. Int. 144:104896. doi: 10.1016/j.neuint.2020.104896
Flatscher-Bader, T., Zuvela, N., Landis, N., and Wilce, P. A. (2008). Smoking and alcoholism target genes associated with plasticity and glutamate transmission in the human ventral tegmental area. Hum. Mol. Genet. 17, 38–51. doi: 10.1093/hmg/ddm283
Fon, E. A., Pothos, E. N., Sun, B. C., Killeen, N., Sulzer, D., and Edwards, R. H. (1997). Vesicular transport regulates monoamine storage and release but is not essential for amphetamine action. Neuron 19, 1271–1283. doi: 10.1016/s0896-6273(00)80418-3
Fortin, G. M., Bourque, M.-J., Mendez, J. A., Leo, D., Nordenankar, K., Birgner, C., et al. (2012). Glutamate corelease promotes growth and survival of midbrain dopamine neurons. J. Neurosci. 32, 17477–17491. doi: 10.1523/JNEUROSCI.1939-12.2012
Fortin, G. M., Ducrot, C., Giguère, N., Kouwenhoven, W. M., Bourque, M.-J., Pacelli, C., et al. (2019). Segregation of dopamine and glutamate release sites in dopamine neuron axons: regulation by striatal target cells. FASEB J. 33, 400–417. doi: 10.1096/fj.201800713RR
Fougère, M., van der Zouwen, C. I., Boutin, J., and Ryczko, D. (2021). Heterogeneous expression of dopaminergic markers and Vglut2 in mouse mesodiencephalic dopaminergic nuclei A8-A13. J. Comp. Neurol. 529, 1273–1292. doi: 10.1002/cne.25020
Gaisler-Salomon, I., Miller, G. M., Chuhma, N., Lee, S., Zhang, H., Ghoddoussi, F., et al. (2009). Glutaminase-deficient mice display hippocampal hypoactivity, insensitivity to pro-psychotic drugs and potentiated latent inhibition: relevance to schizophrenia. Neuropsychopharmacology 34, 2305–2322. doi: 10.1038/npp.2009.58
Gellman, C., Mingote, S., Wang, Y., Gaisler-Salomon, I., and Rayport, S. (2011). “Genetic Pharmacotherapy,” in Drug Discovery and Development - Present and Future, ed. I. M. Kapetanovic (Croatia: InTech), 125–150.
Gonzalez-Reyes, L. E., Verbitsky, M., Blesa, J., Jackson-Lewis, V., Paredes, D., Tillack, K., et al. (2012). Sonic hedgehog maintains cellular and neurochemical homeostasis in the adult nigrostriatal circuit. Neuron 75, 306–319. doi: 10.1016/j.neuron.2012.05.018
Gordon, J. A. (2016). On being a circuit psychiatrist. Nat. Neurosci. 19, 1385–1386. doi: 10.1038/nn.4419
Gorelova, N., Mulholland, P. J., Chandler, L. J., and Seamans, J. K. (2012). The glutamatergic component of the mesocortical pathway emanating from different subregions of the ventral midbrain. Cereb. Cortex 22, 327–336. doi: 10.1093/cercor/bhr107
Grace, A. A., Floresco, S. B., Goto, Y., and Lodge, D. J. (2007). Regulation of firing of dopaminergic neurons and control of goal-directed behaviors. Trends Neurosci. 30, 220–227. doi: 10.1016/j.tins.2007.03.003
Granger, A. J., Wallace, M. L., and Sabatini, B. L. (2017). Multi-transmitter neurons in the mammalian central nervous system. Curr. Opin. Neurobiol. 45, 85–91. doi: 10.1016/j.conb.2017.04.007
Gras, C., Amilhon, B., Lepicard, E. M., Poirel, O., Vinatier, J., Herbin, M., et al. (2008). The vesicular glutamate transporter VGLUT3 synergizes striatal acetylcholine tone. Nat. Neurosci. 11, 292–300. doi: 10.1038/nn2052
Hattori, T., Takada, M., Moriizumi, T., and Van der Kooy, D. (1991). Single dopaminergic nigrostriatal neurons form two chemically distinct synaptic types: possible transmitter segregation within neurons. J. Comp. Neurol. 309, 391–401. doi: 10.1002/cne.903090308
Hnasko, T. S., Chuhma, N., Zhang, H., Goh, G. Y., Sulzer, D., Palmiter, R. D., et al. (2010). Vesicular glutamate transport promotes dopamine storage and glutamate corelease in vivo. Neuron 65, 643–656. doi: 10.1016/j.neuron.2010.02.012
Hnasko, T. S., and Edwards, R. H. (2012). Neurotransmitter corelease: mechanism and physiological role. Annu. Rev. Physiol. 74, 225–243. doi: 10.1146/annurev-physiol-020911-153315
Hökfelt, T., Johansson, O., Ljungdahl, A., Lundberg, J. M., and Schultzberg, M. (1980). Peptidergic neurones. Nature 284, 515–521. doi: 10.1038/284515a0
Howes, O., McCutcheon, R., and Stone, J. (2015). Glutamate and dopamine in schizophrenia: an update for the 21st century. J Psychopharmacol. 29, 97–115. doi: 10.1177/0269881114563634
Insel, T. (2014). The NIMH research domain criteria (RDoC) project: precision medicine for psychiatry. Am. J. Psychiatry 171, 395–397. doi: 10.1176/appi.ajp.2014.14020138
Iversen, S. D., and Iversen, L. L. (2007). Dopamine: 50 years in perspective. Trends Neurosci. 30, 188–193. doi: 10.1016/j.tins.2007.03.002
Johnson, R. G. (1988). Accumulation of biological amines into chromaffin granules: a model for hormone and neurotransmitter transport. Physiol. Rev. 68, 232–307. doi: 10.1152/physrev.1988.68.1.232
Kabanova, A., Pabst, M., Lorkowski, M., Braganza, O., Boehlen, A., Nikbakht, N., et al. (2015). Function and developmental origin of a mesocortical inhibitory circuit. Nat. Neurosci. 18, 872–882. doi: 10.1038/nn.4020
Kawano, M., Kawasaki, A., Sakata-Haga, H., Fukui, Y., Kawano, H., Nogami, H., et al. (2006). Particular subpopulations of midbrain and hypothalamic dopamine neurons express vesicular glutamate transporter 2 in the rat brain. J. Comp. Neurol. 498, 581–592. doi: 10.1002/cne.21054
Kouwenhoven, W. M., Fortin, G., Penttinen, A.-M., Florence, C., Delignat-Lavaud, B., Bourque, M.-J., et al. (2020). Vglut2 expression in dopamine neurons contributes to postlesional striatal reinnervation. J. Neurosci. 40, 8262–8275. doi: 10.1523/JNEUROSCI.0823-20.2020
Kraus, M., Rapisarda, A., Lam, M., Thong, J. Y. J., Lee, J., Subramaniam, M., et al. (2016). Disrupted latent inhibition in individuals at ultra high-risk for developing psychosis. Schizophr. Res. Cogn. 6, 1–8. doi: 10.1016/j.scog.2016.07.003
Kupfermann, I. (1991). Functional studies of cotransmission. Physiol. Rev. 71, 683–732. doi: 10.1152/physrev.1991.71.3.683
Lammel, S., Steinberg, E. E., Földy, C., Wall, N. R., Beier, K., Luo, L., et al. (2015). Diversity of transgenic mouse models for selective targeting of midbrain dopamine neurons. Neuron 85, 429–438. doi: 10.1016/j.neuron.2014.12.036
Li, X., Qi, J., Yamaguchi, T., Wang, H.-L., and Morales, M. (2013). Heterogeneous composition of dopamine neurons of the rat A10 region: molecular evidence for diverse signaling properties. Brain Struct. Funct. 218, 1159–1176. doi: 10.1007/s00429-012-0452-z
Lim, S. A. O., Kang, U. J., and McGehee, D. S. (2014). Striatal cholinergic interneuron regulation and circuit effects. Front. Synaptic Neurosci. 6:22. doi: 10.3389/fnsyn.2014.00022
Linder, C. C. (2001). The influence of genetic background on spontaneous and genetically engineered mouse models of complex diseases. Lab Anim. 30, 34–39.
Linder, C. C. (2006). Genetic variables that influence phenotype. ILAR J. 47, 132–140. doi: 10.1093/ilar.47.2.132
Lindvall, O. (2012). Dopaminergic neurons for Parkinson’s therapy. Nat. Biotechnol. 30, 56–58. doi: 10.1038/nbt.2077
Liss, B., and Roeper, J. (2008). Individual dopamine midbrain neurons: functional diversity and flexibility in health and disease. Brain Res. Rev. 58, 314–321. doi: 10.1016/j.brainresrev.2007.10.004
Lykken, E. A., Shyng, C., Edwards, R. J., Rozenberg, A., and Gray, S. J. (2018). Recent progress and considerations for AAV gene therapies targeting the central nervous system. J. Neurodev. Disord. 10:16. doi: 10.1186/s11689-018-9234-0
Maycox, P. R., Deckwerth, T., Hell, J. W., and Jahn, R. (1988). Glutamate uptake by brain synaptic vesicles. Energy dependence of transport and functional reconstitution in proteoliposomes. J. Biol. Chem. 263, 15423–15428. doi: 10.1016/S0021-9258(19)37605-7
Mendez, I., Dagher, A., Hong, M., Gaudet, P., Weerasinghe, S., McAlister, V., et al. (2002). Simultaneous intrastriatal and intranigral fetal dopaminergic grafts in patients with Parkinson disease: a pilot study. Report of three cases. J. Neurosurg. 96, 589–596. doi: 10.3171/jns.2002.96.3.0589
Mendez, I., Viñuela, A., Astradsson, A., Mukhida, K., Hallett, P., Robertson, H., et al. (2008a). Dopamine neurons implanted into people with Parkinson’s disease survive without pathology for 14 years. Nat. Med. 14, 507–509. doi: 10.1038/nm1752
Mendez, J. A., Bourque, M.-J., Dal Bo, G., Bourdeau, M. L., Danik, M., Williams, S., et al. (2008b). Developmental and target-dependent regulation of vesicular glutamate transporter expression by dopamine neurons. J. Neurosci. 28, 6309–6318. doi: 10.1523/JNEUROSCI.1331-08.2008
Mikelman, S. R., Guptaroy, B., Schmitt, K. C., Jones, K. T., Zhen, J., Reith, M. E. A., et al. (2018). Tamoxifen directly interacts with the dopamine transporter. J. Pharmacol. Exp. Ther. 367, 119–128. doi: 10.1124/jpet.118.248179
Mingote, S., Amsellem, A., Kempf, A., Rayport, S., and Chuhma, N. (2019). Dopamine-glutamate neuron projections to the nucleus accumbens medial shell and behavioral switching. Neurochem. Int. 129:104482. doi: 10.1016/j.neuint.2019.104482
Mingote, S., Chuhma, N., Kalmbach, A., Thomsen, G. M., Wang, Y., Mihali, A., et al. (2017). Dopamine neuron dependent behaviors mediated by glutamate cotransmission. elife 6:e27566. doi: 10.7554/eLife.27566
Mingote, S., Chuhma, N., Kusnoor, S. V., Field, B., Deutch, A. Y., and Rayport, S. (2015a). Functional connectome analysis of dopamine neuron glutamatergic connections in forebrain regions. J. Neurosci. 35, 16259–16271. doi: 10.1523/JNEUROSCI.1674-15.2015
Mingote, S., Masson, J., Gellman, C., Thomsen, G. M., Lin, C.-S., Merker, R. J., et al. (2015b). Genetic pharmacotherapy as an early CNS drug development strategy: testing glutaminase inhibition for schizophrenia treatment in adult mice. Front. Syst. Neurosci. 9:165. doi: 10.3389/fnsys.2015.00165
Moechars, D., Weston, M. C., Leo, S., Callaerts-Vegh, Z., Goris, I., Daneels, G., et al. (2006). Vesicular glutamate transporter VGLUT2 expression levels control quantal size and neuropathic pain. J. Neurosci. 26, 12055–12066. doi: 10.1523/JNEUROSCI.2556-06.2006
Mongia, S., Yamaguchi, T., Liu, B., Zhang, S., Wang, H., and Morales, M. (2019). The ventral tegmental area has calbindin neurons with the capability to co-release glutamate and dopamine into the nucleus accumbens. Eur. J. Neurosci. 50, 3968–3984. doi: 10.1111/ejn.14493
Morales, M., and Root, D. H. (2014). Glutamate neurons within the midbrain dopamine regions. Neuroscience 282, 60–68. doi: 10.1016/j.neuroscience.2014.05.032
Moss, J., Ungless, M. A., and Bolam, J. P. (2011). Dopaminergic axons in different divisions of the adult rat striatal complex do not express vesicular glutamate transporters. Eur. J. Neurosci. 33, 1205–1211. doi: 10.1111/j.1460-9568.2011.07594.x
Nordenankar, K., Smith-Anttila, C. J. A., Schweizer, N., Viereckel, T., Birgner, C., Mejia-Toiber, J., et al. (2015). Increased hippocampal excitability and impaired spatial memory function in mice lacking VGLUT2 selectively in neurons defined by tyrosine hydroxylase promoter activity. Brain Struct. Funct. 220, 2171–2190. doi: 10.1007/s00429-014-0778-9
Papathanou, M., Creed, M., Dorst, M. C., Bimpisidis, Z., Dumas, S., Pettersson, H., et al. (2018). Targeting VGLUT2 in mature dopamine neurons decreases Mesoaccumbal glutamatergic transmission and identifies a role for glutamate co-release in synaptic plasticity by increasing baseline AMPA/NMDA Ratio. Front. Neural. Circuits 12:64. doi: 10.3389/fncir.2018.00064
Papathanou, M., Dumas, S., Pettersson, H., Olson, L., and Wallén-Mackenzie, Å (2019). Off-target effects in transgenic mice: characterization of dopamine transporter (DAT)-cre transgenic mouse lines exposes multiple non-dopaminergic neuronal clusters available for selective targeting within limbic neurocircuitry. Eneuro 6:ENEURO.198–ENEURO.119. doi: 10.1523/ENEURO.0198-19.2019
Pérez-López, J. L., Contreras-López, R., Ramírez-Jarquín, J. O., and Tecuapetla, F. (2018). Direct glutamatergic signaling from midbrain dopaminergic neurons onto pyramidal prefrontal cortex neurons. Front. Neural Circuits 12:70. doi: 10.3389/fncir.2018.00070
Poulin, J.-F., Caronia, G., Hofer, C., Cui, Q., Helm, B., Ramakrishnan, C., et al. (2018). Mapping projections of molecularly defined dopamine neuron subtypes using intersectional genetic approaches. Nat. Neurosci. 21, 1260–1271. doi: 10.1038/s41593-018-0203-4
Pupe, S., and Wallén-Mackenzie, Å (2015). Cre-driven optogenetics in the heterogeneous genetic panorama of the VTA. Trends Neurosci. 38, 375–386. doi: 10.1016/j.tins.2015.04.005
Robinson, T. E., and Berridge, K. C. (2008). Review. The incentive sensitization theory of addiction: some current issues. Philos. Trans. R. Soc. Lond. B. Biol. Sci. 363, 3137–3146. doi: 10.1098/rstb.2008.0093
Romoli, B., Lozada, A. F., Sandoval, I. M., Manfredsson, F. P., Hnasko, T. S., Berg, D. K., et al. (2019). Neonatal nicotine exposure primes midbrain neurons to a dopaminergic phenotype and increases adult drug consumption. Biol. Psychiatry 86, 344–355. doi: 10.1016/j.biopsych.2019.04.019
Root, D. H., Wang, H.-L., Liu, B., Barker, D. J., Mód, L., Szocsics, P., et al. (2016). Glutamate neurons are intermixed with midbrain dopamine neurons in nonhuman primates and humans. Sci. Rep. 6:30615. doi: 10.1038/srep30615
Shen, H., Marino, R. A. M., McDevitt, R. A., Bi, G.-H., Chen, K., Madeo, G., et al. (2018). Genetic deletion of vesicular glutamate transporter in dopamine neurons increases vulnerability to MPTP-induced neurotoxicity in mice. Proc Natl Acad Sci USA 115, E11532–E11541. doi: 10.1073/pnas.1800886115
Silm, K., Yang, J., Marcott, P. F., Asensio, C. S., Eriksen, J., Guthrie, D. A., et al. (2019). Synaptic vesicle recycling pathway determines neurotransmitter content and release properties. Neuron 102, 786–800. doi: 10.1016/j.neuron.2019.03.031
Spitzer, N. C. (2015). Neurotransmitter switching? no surprise. Neuron 86, 1131–1144. doi: 10.1016/j.neuron.2015.05.028
Steinkellner, T., Zell, V., Farino, Z. J., Sonders, M. S., Villeneuve, M., Freyberg, R. J., et al. (2018). Role for VGLUT2 in selective vulnerability of midbrain dopamine neurons. J. Clin. Invest. 128, 774–788. doi: 10.1172/JCI95795
Stocco, A. (2012). Acetylcholine-based entropy in response selection: a model of how striatal interneurons modulate exploration, exploitation, and response variability in decision-making. Front. Neurosci. 6:18. doi: 10.3389/fnins.2012.00018
Straub, C., Tritsch, N. X., Hagan, N. A., Gu, C., and Sabatini, B. L. (2014). Multiphasic modulation of cholinergic interneurons by nigrostriatal afferents. J. Neurosci. 34, 8557–8569. doi: 10.1523/JNEUROSCI.0589-14.2014
Stuber, G. D., Hnasko, T. S., Britt, J. P., Edwards, R. H., and Bonci, A. (2010). Dopaminergic terminals in the nucleus accumbens but not the dorsal striatum corelease glutamate. J. Neurosci. 30, 8229–8233. doi: 10.1523/JNEUROSCI.1754-10.2010
Stuber, G. D., Stamatakis, A. M., and Kantak, P. A. (2015). Considerations when using cre-driver rodent lines for studying ventral tegmental area circuitry. Neuron 85, 439–445. doi: 10.1016/j.neuron.2014.12.034
Sulzer, D., Cragg, S. J., and Rice, M. E. (2016). Striatal dopamine neurotransmission: regulation of release and uptake. Basal Ganglia 6, 123–148. doi: 10.1016/j.baga.2016.02.001
Sulzer, D., Joyce, M. P., Lin, L., Geldwert, D., Haber, S. N., Hattori, T., et al. (1998). Dopamine neurons make glutamatergic synapses in vitro. J. Neurosci. 18, 4588–4602.
Svensson, E., Apergis-Schoute, J., Burnstock, G., Nusbaum, M. P., Parker, D., and Schiöth, H. B. (2018). General principles of neuronal co-transmission: insights from multiple model systems. Front. Neural Circuits 12:117. doi: 10.3389/fncir.2018.00117
Takamori, S., Rhee, J. S., Rosenmund, C., and Jahn, R. (2000). Identification of a vesicular glutamate transporter that defines a glutamatergic phenotype in neurons. Nature 407, 189–194. doi: 10.1038/35025070
Taylor, S. R., Badurek, S., Dileone, R. J., Nashmi, R., Minichiello, L., and Picciotto, M. R. (2014). GABAergic and glutamatergic efferents of the mouse ventral tegmental area. J. Comp. Neurol. 522, 3308–3334. doi: 10.1002/cne.23603
Tecuapetla, F., Patel, J. C., Xenias, H., English, D., Tadros, I., Shah, F., et al. (2010). Glutamatergic signaling by mesolimbic dopamine neurons in the nucleus accumbens. J. Neurosci. 30, 7105–7110. doi: 10.1523/JNEUROSCI.0265-10.2010
Threlfell, S., Lalic, T., Platt, N. J., Jennings, K. A., Deisseroth, K., and Cragg, S. J. (2012). Striatal dopamine release is triggered by synchronized activity in cholinergic interneurons. Neuron 75, 58–64. doi: 10.1016/j.neuron.2012.04.038
Tritsch, N. X., Ding, J. B., and Sabatini, B. L. (2012). Dopaminergic neurons inhibit striatal output through non-canonical release of GABA. Nature 490, 262–266. doi: 10.1038/nature11466
Trudeau, L.-E., Hnasko, T. S., Wallén-Mackenzie, A., Morales, M., Rayport, S., and Sulzer, D. (2014). The multilingual nature of dopamine neurons. Prog. Brain Res. 211, 141–164. doi: 10.1016/B978-0-444-63425-2.00006-4
Vaaga, C. E., Borisovska, M., and Westbrook, G. L. (2014). Dual-transmitter neurons: functional implications of co-release and co-transmission. Curr. Opin. Neurobiol. 29, 25–32. doi: 10.1016/j.conb.2014.04.010
Wallén-Mackenzie, A., Gezelius, H., Thoby-Brisson, M., Nygård, A., Enjin, A., Fujiyama, F., et al. (2006). Vesicular glutamate transporter 2 is required for central respiratory rhythm generation but not for locomotor central pattern generation. J. Neurosci. 26, 12294–12307. doi: 10.1523/JNEUROSCI.3855-06.2006
Wang, D. V., Viereckel, T., Zell, V., Konradsson-Geuken, Å, Broker, C. J., Talishinsky, A., et al. (2017). Disrupting glutamate co-transmission does not affect acquisition of conditioned behavior reinforced by dopamine neuron activation. Cell. Rep. 18, 2584–2591. doi: 10.1016/j.celrep.2017.02.062
Weiner, I., and Arad, M. (2009). Using the pharmacology of latent inhibition to model domains of pathology in schizophrenia and their treatment. Behav. Brain Res. 204, 369–386. doi: 10.1016/j.bbr.2009.05.004
Widner, H., Tetrud, J., Rehncrona, S., Snow, B., Brundin, P., Gustavii, B., et al. (1992). Bilateral fetal mesencephalic grafting in two patients with parkinsonism induced by 1-methyl-4-phenyl-1,2,3,6-tetrahydropyridine (MPTP). N. Engl. J. Med. 327, 1556–1563. doi: 10.1056/NEJM199211263272203
Wieland, S., Du, D., Oswald, M. J., Parlato, R., Köhr, G., and Kelsch, W. (2014). Phasic dopaminergic activity exerts fast control of cholinergic interneuron firing via sequential NMDA, D2, and D1 receptor activation. J. Neurosci. 34, 11549–11559. doi: 10.1523/JNEUROSCI.1175-14.2014
Wijeyekoon, R., and Barker, R. A. (2009). Cell replacement therapy for Parkinson’s disease. Biochim. Biophys. Acta 1792, 688–702. doi: 10.1016/j.bbadis.2008.10.007
Yamaguchi, T., Qi, J., Wang, H.-L., Zhang, S., and Morales, M. (2015). Glutamatergic and dopaminergic neurons in the mouse ventral tegmental area. Eur. J. Neurosci. 41, 760–772. doi: 10.1111/ejn.12818
Yamaguchi, T., Sheen, W., and Morales, M. (2007). Glutamatergic neurons are present in the rat ventral tegmental area. Eur. J. Neurosci. 25, 106–118. doi: 10.1111/j.1460-9568.2006.05263.x
Yamaguchi, T., Wang, H.-L., and Morales, M. (2013). Glutamate neurons in the substantia nigra compacta and retrorubral field. Eur. J. Neurosci. 38, 3602–3610. doi: 10.1111/ejn.12359
Yan, Y., Peng, C., Arvin, M. C., Jin, X.-T., Kim, V. J., Ramsey, M. D., et al. (2018). Nicotinic cholinergic receptors in VTA glutamate neurons modulate excitatory transmission. Cell Rep. 23, 2236–2244. doi: 10.1016/j.celrep.2018.04.062
Yoon, T., Okada, J., Jung, M. W., and Kim, J. J. (2008). Prefrontal cortex and hippocampus subserve different components of working memory in rats. Learn. Mem. 15, 97–105. doi: 10.1101/lm.850808
Yoshiki, A., and Moriwaki, K. (2006). Mouse phenome research: implications of genetic background. ILAR J. 47, 94–102. doi: 10.1093/ilar.47.2.94
Zell, V., Steinkellner, T., Hollon, N. G., Warlow, S. M., Souter, E., Faget, L., et al. (2020). VTA glutamate neuron activity drives positive reinforcement absent dopamine co-release. Neuron 107, 864–873. doi: 10.1016/j.neuron.2020.06.011
Zhang, S., Qi, J., Li, X., Wang, H.-L., Britt, J. P., Hoffman, A. F., et al. (2015). Dopaminergic and glutamatergic microdomains in a subset of rodent mesoaccumbens axons. Nat. Neurosci. 18, 386–392. doi: 10.1038/nn.3945
Zhang, Y.-F., and Cragg, S. J. (2017). Pauses in striatal cholinergic interneurons: what is revealed by their common themes and variations? Front. Syst. Neurosci. 11:80. doi: 10.3389/fnsys.2017.00080
Keywords: VGLUT2, VMAT2, glutaminase, schizophrenia, addiction, psychostimulant, Parkinson disease
Citation: Eskenazi D, Malave L, Mingote S, Yetnikoff L, Ztaou S, Velicu V, Rayport S and Chuhma N (2021) Dopamine Neurons That Cotransmit Glutamate, From Synapses to Circuits to Behavior. Front. Neural Circuits 15:665386. doi: 10.3389/fncir.2021.665386
Received: 08 February 2021; Accepted: 16 April 2021;
Published: 19 May 2021.
Edited by:
Jean-Francois Poulin, McGill University, CanadaReviewed by:
Åsa Wallén-Mackenzie, Uppsala University, SwedenLouis-Eric Trudeau, Université de Montréal, Canada
Copyright © 2021 Eskenazi, Malave, Mingote, Yetnikoff, Ztaou, Velicu, Rayport and Chuhma. This is an open-access article distributed under the terms of the Creative Commons Attribution License (CC BY). The use, distribution or reproduction in other forums is permitted, provided the original author(s) and the copyright owner(s) are credited and that the original publication in this journal is cited, in accordance with accepted academic practice. No use, distribution or reproduction is permitted which does not comply with these terms.
*Correspondence: Stephen Rayport, U3RlcGhlbi5SYXlwb3J0QG55c3BpLmNvbHVtYmlhLmVkdQ==; Nao Chuhma, TmFvLkNodW1hQG55c3BpLmNvbHVtYmlhLmVkdQ==