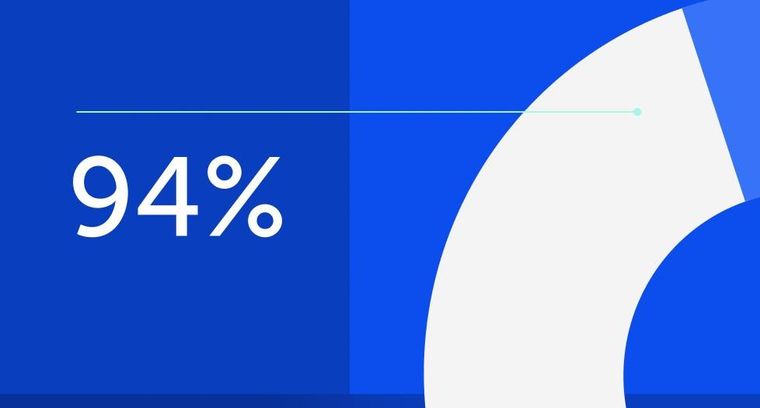
94% of researchers rate our articles as excellent or good
Learn more about the work of our research integrity team to safeguard the quality of each article we publish.
Find out more
ORIGINAL RESEARCH article
Front. Neural Circuits, 16 May 2018
Volume 12 - 2018 | https://doi.org/10.3389/fncir.2018.00038
This article is part of the Research TopicComputational Methodologies in Brain Imaging and Connectivity: EEG and MEG ApplicationsView all 11 articles
Saccadic eye movements are an inherent component of natural reading, yet their contribution to information processing at subsequent fixation remains elusive. Here we use anatomically-constrained magnetoencephalography (MEG) to examine cortical activity following saccades as healthy human subjects engaged in a one-back word recognition task. This activity was compared with activity following external visual stimulation that mimicked saccades. A combination of procedures was employed to eliminate saccadic ocular artifacts from the MEG signal. Both saccades and saccade-like external visual stimulation produced early-latency responses beginning ~70 ms after onset in occipital cortex and spreading through the ventral and dorsal visual streams to temporal, parietal and frontal cortices. Robust differential activity following the onset of saccades vs. similar external visual stimulation emerged during 150–350 ms in a left-lateralized cortical network. This network included: (i) left lateral occipitotemporal (LOT) and nearby inferotemporal (IT) cortex; (ii) left posterior Sylvian fissure (PSF) and nearby multimodal cortex; and (iii) medial parietooccipital (PO), posterior cingulate and retrosplenial cortices. Moreover, this left-lateralized network colocalized with word repetition priming effects. Together, results suggest that central saccadic mechanisms influence a left-lateralized language network in occipitotemporal and temporal cortex above and beyond saccadic influences at preceding stages of information processing during visual word recognition.
Active reading is a complex skill thought to require coordination between eye movements, attention, and written language processing (Engbert et al., 2005; Pollatsek et al., 2006; Rayner, 2009), yet the neural basis of this coupling remains elusive. In particular, the extent to which saccades impact reading processes at fixation is little understood, since current neurobiological models of visual word processing typically account for neuroimaging and neurophysiological data collected during stable fixation when the natural temporal proximity of saccades and target words is disrupted (Sereno and Rayner, 2003). Recent studies using fMRI and EEG combined with eye tracking have begun to evaluate the ecological validity of these models in reading tasks that include eye movements, albeit focusing on mechanisms of eye movement control and contributions of spatial attention rather than the impact of the saccade itself on information processing at fixation (e.g., Dimigen et al., 2011; Henderson et al., 2015, 2016; Schuster et al., 2015, 2016; but see Kornrumpf et al., 2016). However, active vision studies in humans and monkey indicate that central saccadic signals gated by brain regions that control eye movements and attention modify visual perception and cognition around the time of saccades, acting via distinct mechanisms before, during and after an eye movement (Wurtz, 2008; Berman and Colby, 2009; Ibbotson and Krekelberg, 2011). Recent evidence using magnetoencephalography (MEG) combined with saccade detection in real time supports the view that saccades also impact brain responses to words presented at fixation, revealing various degrees of saccadic modulation in early visual and higher cortical areas (Temereanca et al., 2012). An unexplored question is whether central saccadic signals impact occipitotemporal and temporal cortical areas that are implicated in visual word processing above and beyond preceding saccadic influences in occipital cortex.
During saccades, visual stability emerges in the brain despite abrupt self-induced changes in visual input associated with the eye movement. In contrast to salient external visual motion, self-induced saccadic visual motion is not consciously perceived, although it continues to be processed in the visual system and thus can impact information processing at subsequent fixation (Ibbotson and Cloherty, 2009). Consistent with interactions between visual signals during and after saccades, our previous results suggest that saccadic image motion modulates responses to words presented at fixation (Temereanca et al., 2012). In addition to such visual effects, central saccadic signals mediated by brain regions that control eye movements and attention are known to impact information processing in visual areas, producing effects that include transsaccadic suppression followed by postsaccadic enhancement. Transaccadic suppression from ~100 ms before onset to ~50 ms after the end of saccades is thought to decrease visual sensitivity to saccadic image motion, contributing to visual stability (Ross et al., 2001). Postsaccadic enhancement lasting ~200–400 ms is thought to promote visual perception at fixation (Ibbotson and Cloherty, 2009; Schroeder et al., 2010). Saccadic effects have been reported throughout the visual system, including early cortical and thalamic visual areas (Reppas et al., 2002; Sylvester and Rees, 2006; MacEvoy et al., 2008) as well as in areas of the temporal cortex implicated in visual recognition and memory (Sobotka et al., 1997, 2002; Purpura et al., 2003; Bartlett et al., 2011; Monosov et al., 2011; Jutras et al., 2013). Using anatomically-constrained MEG source estimates to measure patterns of cortical activity with high temporal and spatial resolution, previous evidence suggests that visual and central effects of saccades also alter the dynamics of word-evoked cortical activation (amplitude, time-course) at multiple stages of visual word processing (Temereanca et al., 2012). It is not known, however, if central saccadic signals impact higher stages of visual word representation in occipitotemporal and/or temporal cortex above and beyond preceding effects in occipital cortex.
Here we use anatomically-constrained MEG to examine cortical activity following the onset of saccades as healthy human subjects engaged in a one-back word recognition task. In a parallel experiment in the same participants, this activity was compared with activity following the onset of external visual stimulation that mimicked saccades. With retinal stimulation similar between these conditions, response differences provide insights into the contributions of central saccadic mechanisms to cortical activity after the onset of saccades, when central saccadic signals are present, vs. after external image motion, when such saccadic signals are absent. We introduced a new approach that combines several procedures to eliminate saccadic ocular artifacts from the MEG signal, an inherent difficulty in active reading research with electrophysiological measurements (Berg and Scherg, 1991; Dimigen et al., 2011; Carl et al., 2012). Cortical regions impacted by central saccadic influences were established using cluster-based analysis across space and time, and results were tested on an independent subset of data using parametric statistics. Employing this approach, we compared cortical activity following the onset of saccades and spanning fixation before word appearance vs. similar external visual stimulation. We then examined whether differential responses colocalized with word repetition priming effects, as well as with known saccadic effects on responses to words reported previously in these participants (Temereanca et al., 2012). Together, our results provide the first evidence for central saccadic influences in a left-lateralized language network in occipitotemporal and temporal cortex above and beyond saccadic influences at preceding stages of information processing during visual word recognition.
Participants were healthy, right-handed adults and native English speakers. Seven subjects (mean age of 29 years, range 23–42 years, 5 males) underwent two MEG sessions for Experiments 1–2, and also a structural MRI scan. Participants overlapped in full with those studied in our prior publication on this paradigm (Temereanca et al., 2012). This study was carried out in accordance with the recommendations of the approved guidelines of the Massachusetts General Hospital Institutional Review Board with written informed consent from all subjects. All subjects gave written informed consent in accordance with the Declaration of Helsinki. The experimental protocol was approved by the Massachusetts General Hospital Institutional Review Board.
As described in detail in Temereanca et al. (2012), during a one-back word recognition task, subjects waited for an auditory go-cue at the beginning of each trial to make a saccade between two fixed strings of five crosshairs, 10 degrees apart (Figure 1A). Saccades were detected in real time using the horizontal electrooculogram (EOG) signal and triggered the subsequent word appearance at the new fixation either 76 ms (early word presentation condition) or 643 ms (late condition) later. These latencies include a fixed delay of 33 ms between the stimulus trigger pulses sent by the Presentation program and the stimulus appearance on the projection screen. These latencies ensured in individual subjects that words appeared at re-fixation only after the end of saccades (see online saccade detection and offline computations below), allowing control of stimulus timing (onset and duration) across conditions. The word stimuli were five-letter novel words (50%) and one repeated word presented for 250 ms. No-word trials were included wherein a string of five Xs presented 1243 ms after the saccade detection marked the end of trial. No-word and late word presentation trials were examined here to evaluate the brain activity evoked by saccades in the absence of words. Early, late and no-word trials appeared in pseudo-randomized order, with 1300–1500 ms interstimulus interval. Subjects were instructed to read the stimulus silently and respond as accurately and quickly as possible by pressing a button with their right index finger if the stimulus was the same as that in the previous trial (10%, match trials), and another with their left finger otherwise (90%, non-match trials). We collected 110 trials per condition for each of 10 conditions (early vs. late word presentation, novel vs. repeated words and no-word trials, for right as well as left saccades) in 20 blocks, with short 1–3 min. breaks between blocks and a total recoding time of 90 min. Two additional blocks were used to familiarize the subject with the task before recordings. During recordings, subjects rested their upper jaw on a custom-made bite-bar while comfortably leaning their head against the back of the dewar’s helmet; this approach maintained a steady position of the head relative to the MEG sensors within as well as across recording sessions.
Figure 1. Experimental design and magnetoencephalography (MEG) waveforms from an individual subject. (A) Experiment 1 (natural saccades, left). Subjects performed a one-back word recognition task while reading words presented foveally after saccades detected in real time. Cued by a brief tone, subjects made saccades between two strings of five crosshairs separated by 10° and subsequently maintained fixation before words appeared early or late after saccade detection, or until the end of trial (no-word trials). No-word and late word presentation trials were used to examine cortical activity following the onset of saccades in the absence of words, during a time-window of −200 to 500 ms relative to the onset of saccades. Experiment 2 (external image movement, right). In parallel experiments, the same subjects performed the one-back word recognition task while reading words presented foveally after external image movement that mimicked saccades. The experimental design, including image movement and word presentation timing, matched those in the saccade task. No-word and late word presentation trials were used to examine cortical activity after the onset of saccade-like external image movement. AC, Auditory cue; BP, button press. (B) Mean horizontal electrooculogram (EOG) and MEG waveforms from a representative subject generated by right (red) and left (blue) saccades (a) before and (b) after saccadic artifact reduction with Spatial Signal Space Projection (SSP) method. Right and left saccades produced ocular artifacts with opposite polarity, measured as significant correlations between the horizontal EOG and MEG signals (see “Results” section). SSP filtering eliminated or greatly attenuated artifacts across individual MEG channels. (c) Mean horizontal EOG and MEG waveforms generated by left (black) and right (gray) image movement that mimicked right and left saccades, respectively.
Word stimuli were five-letter words balanced across conditions with respect to lexical frequency (Kucera and Nelson Francis, 1967; range, 1–192/million), concreteness index (range, 220–648), and stress. Both novel words (50% of trials) and one repeated word were presented. For the repeated word condition, we repeatedly presented a single word either early or late after right and left saccades in order to reliably assess postsaccadic effects on responses in early visual areas that are sensitive to the visual attributes of the stimulus (Temereanca et al., 2012). Analysis of novel vs. repeated word contrast was reported and discussed extensively in Temereanca et al. (2012), revealing left-lateralized word repetition effects consistent with well-established results in language research.
Stimuli were presented on a computer-driven projection and subtended 5 degrees visual angle; there was about one letter per degree of visual angle. The whole projection screen subtended 47 degrees.
Occasionally, the electronic circuit did not detect a saccade and as a result failed to trigger the word appearance. For these trials (<10% of all trials), feedback was provided immediately by the appearance of the word “error” at the missed saccade target location, which cued the subject to correct gaze by fixating the missed location and await a new trial. This small failure rate indicates that subjects were able to perform the saccades consistently and stereotypically.
Cortical responses related to words were reported in Temereanca et al. (2012). In the present study, we examined cortical activity following the onset of saccades in the absence of words, estimated from no-word trials and late word presentation trials. We then compared this pattern with the cortical activity following the onset of external visual stimulation that mimicked saccades estimated in Experiment 2.
In parallel experiments words were presented after external image movement that mimicked an eye movement (Figure 1A). Following the auditory cue (AC), subjects were instructed to maintain their gaze stationary in the center of the screen while the two strings of five crosshairs 10 degrees apart were moved to mimic the retinal image motion during an eye movement. Words were presented at fixation either early (59 ms) or late (626 ms) after the external image movement offset. No-word trials were also included wherein a string of five Xs presented 1243 ms after movement offset marked the end of trial. These no-word trials as well as the late word presentation trials were examined here to evaluate the brain activity following the onset of saccade-like image motion in the absence of words. Word and no-word trials appeared in pseudo-randomized order, with 1300–1500 ms interstimulus interval. Based on data in Experiment 1, for each subject we computed the mean and variance of the saccade onset latencies with respect to the AC (see offline computations of saccade times below). Random numbers following this distribution were generated and used here to set the onset time of the image movement relative to the AC. Motion velocity and duration matched the average values obtained for saccades in preliminary experiments. Specifically, motion stimuli consisted of a sequence of five frames presented at 60 Hz, which changed the location of the two crosshairs on the screen by a total of 10 deg to mimic the motion stimulus on the retina during 10 deg horizontal saccades (see Figure 1A). The duration of the motion stimulus was 83 ms (5 frames × 1/60 Hz = 83 ms) and matched the average saccade duration of ~80 ms obtained in preliminary experiments; the shift for each individual frame was chosen to match the velocity profile of saccades (as inferred from the EOG signal): 1.5 deg for first frame, 2.6 deg, 2.9 deg, 2.2 deg and 0.8 deg for subsequent frames, respectively. Experiment 2 paralleled Experiment 1 in every other aspect regarding word stimuli, inclusion of no-word trials, number of trials per condition and number of blocks, collection of behavioral data as well as task instructions.
Our experimental design differs from normal reading in several ways, discussed also in Temereanca et al. (2012). Specifically, natural reading involves sequences of self-paced, small (1–2°) eye movements separated by short fixations in text. Here, an AC marks the beginning of each trial, and the subject waits for the cue to either make a large (10°) voluntary saccade between two stable crosshairs in Experiment 1, or to keep their gaze stationary as the image moves in Experiment 2. These differences help control word-stimulus timing (onset and duration), and maintain similar cognitive processes between word-stimulus presentation and behavioral decision across experimental conditions in Experiment 1 and Experiment 2. The present experimental design also eliminates residual visual activity from a previous fixation by introducing ~1.5 s of stationary fixation between trials.
Whole-head MEG was recorded in a magnetically and electrically shielded room (Imedco AG, Hägendorf, Switzerland) using a Neuromag Vectorview system (Elekta Neuromag Oy., Helsinki, Finland) with 306-channels arranged in triplets of two orthogonal planar gradiometers and a magnetometer. The acquisition band-pass was 0.01–200 Hz and the data were digitized at 600 samples/s. The horizontal and vertical components of eye-movements were recorded concurrently with MEG using two pairs of bipolar EOG electrodes. For subsequent coregistration with the structural MRI and to record the position of the head relative to the sensor array, the locations of four head-position indicator (HPI) coils attached to the scalp, three fiducial landmarks (nasion and auricular points), and additional scalp surface points were digitized using a 3Space Fastrak system (Polhemus, Colchester, VT, USA) integrated with the Vectorview system.
Saccades were detected in real time using the EOG signal for horizontal eye movements, which was sent online to a saccade-detection circuit employing filters and a threshold comparator (Temereanca et al., 2012). Saccade detection triggered the word presentation at two delays adjusted in preliminary experiments so that the display changed at refixation only after the end of the saccade, allowing control of word stimulus timing (onset and duration) across conditions.
The times of saccades and word presentation relative to saccades were confirmed offline. The beginning and endpoint of saccades were computed for each subject and experimental condition (e.g., separately for early, late and no-word conditions corresponding to right saccades and left saccades) based on the EOG signal averaged across trials aligned to the time of online saccade detection, using in-house software in MATLAB (Mathworks, Natick, MA, USA). The algorithm started at peak velocity computed as the first temporal derivative of the EOG trace and searched backward and forward to fixation. Saccade onset and offset were defined as the first point in time preceding peak velocity and the last point following peak velocity, respectively, for which velocity was larger than 3.3 SDs from the mean baseline value (p < 0.001).
These offline computations revealed saccade latencies of 297.1 ± 21.2 ms (mean ± SD) following the AC. They also showed that the saccade-detection circuit detected saccades in the EOG signal 47 ms on average following saccade onset; detection times varied across subjects and for right vs. left saccades, accounting for words appearing on average 21 ms earlier after the end of right than left saccades (range, 14–30 ms; Temereanca et al., 2012). These offline computations confirmed that words appeared in the early condition 119.9 ± 2.9 ms (mean ± SE) and in the late condition 686.7 ± 3.0 ms after the onset of saccades; these times include a fixed delay of 33 ms between the stimulus trigger pulses sent by the Presentation program and the stimulus appearance on the projection screen, which is attributed in part to the projector’s refresh rate of 60 Hz. These computations revealed that right and left saccades had similar durations of 82.8 ms and 81.3 ms on average, respectively (paired t-test, p > 0.5; range, 75–91 ms).
Noisy MEG channels were identified by inspection of raw data and offline averages and were excluded from subsequent analysis. MEG data were low-pass filtered at 40 Hz. In Experiment 1, trials including right or left saccades detected in real-time were rejected based on large vertical EOG (>150 μV) indicative of blinks. The raw EOG signals aligned to the saccade onsets revealed similar amplitudes and shapes across trials, consistent with subjects performing stereotypical saccades between the two strings of crosshairs. In Experiment 2, trials during steady eye fixation were rejected based on large vertical and horizontal EOG signals (>150 μV) indicative of blinks and horizontal eye movements, respectively. The raw EOG signals from individual trials included in further analysis were visually inspected and no evidence was found for systematic eye movements beyond the horizontal saccades detected in real-time in Experiment 1.
Average waveforms relative to the onset of saccades and saccade-like external image motion were obtained separately from no-word trials and from late word presentation trials, as follows. In each subject, averages were computed separately for right and left saccades aligned to saccade onsets (N ~ 65–100 trials), as well as for right and left saccades together. Similarly, averages were computed separately for right and left external image motion aligned to the image motion onset as well as for right and left movement together.
To map the MEG signal onto the cortex and estimate the spatiotemporal cortical activation following the onset of saccades in the absence of horizontal saccadic artifacts, we introduced an approach that combines two complementary analyses. First, we averaged an equal number of right and left saccade trials (after randomly eliminating trials from the more numerous condition). In our experimental design, right and left saccades gave rise to ocular artifacts of opposite sign but similar amplitudes (Figure 1B) that canceled when averaged, thus effectively annulling horizontal saccadic artifacts. Although visual inspection of the EOG signal revealed no systematic eye movements beyond the horizontal saccades included in the experimental design, it is still conceivable that there are differences in eye movement behavior across right and left saccade conditions, producing asymmetric eye movements which could lead to artifacts not canceled by the averaging procedure. To control for an impact of any remaining artifacts, including those introduced by any asymmetric eye movements, in a second analysis we employed spatial and temporal signal-space projection (SSP) methods separately for right saccades and left saccades, respectively, and only afterwards we averaged across right and left saccade trials. SSP projections computed separately for right and left saccades are expected to eliminate or greatly attenuate artifacts across individual MEG channels before averaging, including any artifacts introduced by asymmetric eye movements. Consequently, similar results in these complementary analyses would provide evidence that saccadic artifacts do not impact results in the present study.
Specifically, to suppress saccadic artifacts, we used the SSP method implemented in the MNE software (Hämäläinen, 1995; Uusitalo and Ilmoniemi, 1997) as well as a temporal filtering method similar to that described in Taulu and Simola (2006). For SSP, the spatial subspace containing the ocular artifact was estimated from the MEG data. A covariance matrix was computed from concatenated epochs spanning 5 ms before and 90 ms after right and left saccade onsets. A singular value decomposition (SVD) was performed on the covariance matrix separately for magnetometers and gradiometers. One singular vector in each set of sensors, corresponding to the highest singular value, was used to construct a linear projection operator corresponding to the ocular artifact and applied to the MEG data. The noise subspace corresponding to the ocular artifact was thus eliminated.
In the temporal filtering method, a temporal SSP corresponding to the ocular (non-brain) sources was estimated from the EOG data of each non-overlapping time-window of 16 s. SVD was performed on the normalized EOG in each time window, resulting in a orthonormal set of eigenvectors used to compute the temporal SSP operator, which was then applied to the MEG data, eliminating temporal components corresponding to the ocular artifact.
Both the spatial and temporal SSP approaches have the potential drawback of attenuating brain signals of interest. For the spatial SSP, this dampening can be rigorously assessed by computing the amount by which the signals from any given cortical region are attenuated by the application of the spatial SSP operator. Specifically, to assess the dampening of the cortical signals from SSP vectors, we computed the subspace correlation between each of the columns of the gain matrix and the space spanned by the SSP vectors. This index equals zero if the gain matrix column is orthogonal to the SSP space, and one if it is parallel to it. The SSP spatial filter was computed by concatenating data from all epochs within a time window from 5 ms before to 90 ms after right and left saccade onsets. The filter was applied to the data at all times. The value representing the amount of dampening is data and time independent, depending only on the time window chosen to compute the SSP vectors. We found that the average of this dampening was below 0.1, suggesting only a minor effect on the MEG signals of interest. Furthermore, in the source analysis we took this dampening correctly into account by applying the spatial SSP operator to the forward solution and noise covariance matrices. Analogously, the temporal SSP procedure dampens any brain signals which are correlated with the EOG data. Since the space of potential brain signal waveforms is unknown, it is impossible to make a conclusive quantitative assessment of this effect. However, the EOG electrodes are relatively far away from most brain regions, with possibly the most frontal parts of the cortex being an exception, and, therefore, we do not expect that the application of temporal SSP seriously distorts the estimated source waveforms. In addition, it is unlikely that the brain signals themselves have waveforms highly correlated with EOG which relates to the ocular activity. This conclusion is further supported by the fact that the source estimates computed from original data with left and right saccades averaged were similar to those resulting from data which had either spatial or temporal SSP applied to them before the averaging procedure.
In addition to canceling saccadic artifacts of opposite polarity, trial averaging across saccades in opposite directions might dampen saccadic signals of interest, including preparatory activity. That in turn could decrease observed differences between experimental conditions to different degrees across cortical regions. We note, however, that the varying sizes of effects across cortical regions reported here were found to be in agreement with those produced by saccades and external image-motion on subsequent word-evoked responses reported in Temereanca et al. (2012) and detailed in the “Discussion” section, with the latter having been established in the absence of averaging, separately for right saccades and left saccades.
Saccadic artifacts were measured by assessing the correlation between the horizontal EOG, which mirrors ocular artifacts, and the MEG signal in both original and filtered data (Figure 1B). In each subject, EOG and MEG data segments from −10 to 70 ms around saccade onset were concatenated across trials, and a correlation coefficient was computed between concatenated data from the horizontal EOG and each MEG channel. Correlation coefficients were mapped to a normal distribution using a Fisher z-transformation and then tested for significance from 0 using a paired t-test across subjects. Focusing on a period around the saccade, this correlation approach detects the presence of the saccadic artifact with high sensitivity regardless of the artifact amplitude.
MRI recordings (1.5 T Sonata scanner, Siemens Medical Solutions, Erlangen, Germany) consisted of two structural 3D magnetization prepared rapid gradient echo (MPRAGE) scans (TR/TE/TI = 2.73 s/3.31 ms/1 s, flip angle = 7°, 128 × 1.3 mm-thick sagittal slices at an in-plane resolution of 1 mm2) and two multi-echo multi flip angle (5° and 30°) fast low-angle shot (FLASH) scans (TR/TE = 20 ms/(1.8 + 1.82 × n) ms, n = 0–7). The standard MPRAGEs were used for individual cortical surface reconstructions with FreeSurfer1 and for registering MEG data to the individual subject’s anatomy (Dale et al., 1999; Fischl et al., 1999a,b). The FLASH sequences were used to compute the inner skull surface for the boundary element model (BEM). This information was then employed in computing the MEG forward solution. Cortical surfaces were inflated to visualize both gyri and sulci and to morph the hemispheres into a sphere for inter-subject registration based on the sulcal-gyral pattern (Fischl et al., 1999a,b).
MEG average signals were further analyzed to estimate the corresponding patterns of brain activity (current sources) across cortical locations and time. MEG measures the magnetic fields generated by post-synaptic currents in the brain. These current sources were estimated using the linear minimum-norm estimate (MNE) approach (Dale and Sereno, 1993; Hämäläinen and Ilmoniemi, 1994) and information of the head anatomy obtained from anatomical MRI data using the MNE software2 (Gramfort et al., 2014). The solution space for the estimated currents was constrained to the gray/white matter boundary reconstructed for each individual from the structural MRI, which was subsampled to 4098 dipole elements per hemisphere with ~5-mm spacing (Dale et al., 1999; Fischl et al., 1999a). A forward solution for the source space was computed using a one-layer BEM model (Hämäläinen and Sarvas, 1989). A noise covariance matrix was calculated from 200-ms baseline periods prior to the AC that preceded saccades (Experiment 1) or external image motion (Experiment 2) in individual trials. The noise covariance matrix and the forward solution were used to create a linear inverse operator (Dale et al., 2000) that was applied to the data at each time point, producing time-courses of activity at each cortical location. Current orientations were approximately constrained to be perpendicular to the cortical surface by setting source variances for the transverse components to be 0.6 times the variance of the normal components (Lin et al., 2006). For group analysis, the inverse solutions were registered to the average cortical surface computed across all subjects using an algorithm matching the cortical folding patterns (Fischl et al., 1999b). The current estimate at each cortical location was divided by the estimated baseline variance, resulting in an F-like statistic (Dale et al., 2000). The square root of the F statistic, which is a signal-to-noise ratio estimate, is analogous to a z-score and allows the visualization of results as dynamic statistical parametric maps (dSPM). The dSPM identifies locations where current strength estimates are most reliable based on their signal-to-noise ratio.
Differences in estimated cortical activity following the onset of saccades vs. saccade-like external image movement in space and time were established using the MEG signals from no-word trials and cluster-based statistics, a nonparametric permutation-based method that inherently corrects for multiple comparisons (Maris and Oostenveld, 2007). Specifically, a paired t-test across subjects was used to compare saccade vs. external image movement conditions at each time point and for each vertex on the cortex. Spatiotemporal clusters were then computed to include spatially and temporally contiguous sources on the cortex and within the 100–500 ms response window that exhibited significant differences at uncorrected p < 0.05. For cluster calculations on the cortex, we computed the adjacency matrix across vertices using Brainstorm (Tadel et al., 2011). For each cluster, the sum of t-score values was calculated across every spatiotemporal point within the cluster. Permutation testing was performed by randomly permuting conditions within subjects. For each permutation, we repeated previous steps of the analysis and then selected the cluster with the maximum sum of t-score values. We used 1000 permutations to obtain a distribution of maximum sums, which constitutes our null distribution. A p-value was then computed for each original (non-permute) cluster by finding the number of times the values in the null distribution were higher than the original cluster t-score; this p-value is corrected for multiple-comparisons across space and time. We selected original clusters that exhibited corrected p < 0.05.
For clusters of significant differential responses following the onset of saccades vs. external image movement (corrected p < 0.05), we computed the spatial overlap with anatomical labels generated in FreeSurfer as well as with manually-drawn labels from our previous investigation of word-evoked responses in this subject group (Temereanca et al., 2012). As explained in detail in Temereanca et al. (2012), the latter regions of interest (ROIs) have been selected a priori based on their implication in previous studies of visual word recognition, and because they met the study criteria for significant activation in response to visual words. ROIs were represented on the average brain of all subjects. The same ROIs were used for all subjects by automatic spherical morphing of original labels to individual subjects (Fischl et al., 1999b). Regional time-courses of estimated cortical responses for individual subjects and conditions were computed by averaging the absolute current values within an ROI across voxels at each time point.
Results emerging from the cluster-based statistical analysis were confirmed using independent data from late word presentation trials and parametric statistics. Specifically, for an individual cortical region and time interval corresponding to a spatiotemporal cluster and fixed across subjects, we compared activity following saccades vs. saccade-like external image movement using a two-tailed paired t-test.
The approach employed here to compare cortical activity following the onset of saccades vs. saccade-like external visual stimulation was not feasible for the early word presentation trials because of variation in word-onset times relative to the saccade onset/offset for right vs. left saccades and across subjects (see “Saccade Detection” section above), which generate differences in visual stimulation across conditions.
Behavioral performance in the one-back word recognition task, previously reported in Temereanca et al. (2012), was similar in Experiment 1 and Experiment 2, consistent with similar task engagement. Subjects determined whether the word presented at fixation was the same as that in the previous trial (10% match trials) or different (90% nonmatch trials). High overall accuracy scores were observed in both experiments, for both one-back match (average percentage correct, 80.06% and 80.16% in Experiments 1 and 2, respectively) and nonmatch trials (99.79% and 99.61%, respectively). Further, behavioral influences of saccades and saccade-like external image movement, measured as changes in reaction times for early minus late word presentation conditions, were found similar (i.e., no main effect of experiment type, p = 0.49; Temereanca et al., 2012).
Cortical activity following the onset of saccades was examined in seven healthy volunteers engaged in a one-back word recognition task, using anatomically-constrained MEG (see “Materials and Methods” section; Figure 1A). Subjects made horizontal saccades between two strings of five crosshairs 10° apart and subsequently maintained fixation before words (novel or repeats) appeared early (76 ms) or late (643 ms) after saccade detection in real time, or until the end of trial (no-word trials). No-word and late word presentation trials were examined here to evaluate the cortical activity evoked by saccades in the absence of words, during a time-window of −200 to 500 ms relative to the onset of saccades. This activity reflects a combination of the retinal activity during the saccade, the retinal activity at the onset of fixation period, as well as central saccadic signals gated by oculomotor brain regions.
The average MEG responses during −200 to 500 ms were similar for no-word and late word presentation trials, respectively, as expected because of identical visual stimulation and task conditions. Figure 1B illustrates averages of the horizontal EOG and MEG responses for right and left no-word trials (N ≈ 90) in an individual subject. Right and left saccades produced ocular artifacts with opposite polarity and similar magnitudes, measured as significant correlations between the horizontal EOG which mirrors ocular artifacts and MEG signals (Figure 1Ba). Artifacts were larger over frontal and most anterior temporal sensors and gradually decreased in magnitude over temporal, parietal and occipital sensors.
To remove saccadic artifacts from the MEG data, we averaged a balanced number of right and left saccades, canceling associated artifacts of similar magnitude but opposite polarity. To control for an impact of potential remaining artifacts attributed to any asymmetric eye movements across right and left trials, in a complementary analysis ocular artifacts were removed from MEG data before the averaging procedure using spatial and temporal SSP filtering separately for right and left saccade trials (see “Materials and Methods” section). Each of these filtering methods eliminated or greatly attenuated artifacts across individual MEG channels (Figure 1Bb). Remaining artifacts were evaluated by computing the correlation between the horizontal EOG and MEG waveforms around the time of saccades (see “Materials and Methods” section). Using this highly sensitive detection method that ignores the amplitude of the remaining artifact, significant correlations were found in 214 out of 306 MEG channels before filtering, but only in 95 frontal channels after spatial SSP. Most importantly, the application of the SSP revealed strong MEG responses with a stable baseline and onset latencies not correlating with the EOG saccade waveform. Averages of right and left saccades were computed for the original signal as well as after artifact removal with spatial and temporal SSP, and produced similar results for all analyses described below, providing evidence that saccadic artifacts do not impact the results reported here.
Estimates of cortical activity were calculated across locations and time using a distributed source modeling approach that constrained current sources to the cortical surface of each participant reconstructed from structural MRI (Dale and Sereno, 1993). Noise-normalized dSPMs (Dale et al., 2000) were computed to evaluate the statistical significance of estimated responses relative to baseline activity measured prior to the AC in each trial. Figure 2 illustrates snapshots of average dSPMs across subjects for two-time windows after saccade onset, computed separately for right saccades and for the average of right and left saccades. The first-time window (40–80 ms after onset) occurs during the saccades and exhibits large saccadic artifact, whereas the second window (80–500 ms after onset) begins around the end of saccades and spans fixation. For right saccades, activity at 60 ms was dominated by saccadic ocular artifacts which exhibited a maximum in the orbitofrontal cortex and extended throughout the frontal and anterior temporal cortex and to a lesser degree to more posterior temporal, parietal and occipital cortex. Spatial and temporal SSP filters greatly reduced ocular artifacts in frontal cortex and temporal poles, and eliminated artifacts in more posterior temporal, parietal and occipital regions. Further, estimates of the average of right and left saccades reliably showed the largest signal-to-noise ratio and minimum ocular artifacts in the most affected regions (orbitofrontal cortex and temporal pole) as well as no contamination across the remaining of the cortical surface. These estimates based on averages of right and left saccades were further analyzed here to examine the cortical activity following the onset of saccades.
Figure 2. Average dynamic statistical parametric maps (dSPMs) across subjects and selected response windows following saccade onset, computed separately for right saccades and for the average of right and left saccades. For right saccades, activity at 60 ms was dominated by saccadic ocular artifacts. Ocular artifacts were greatly reduced for MEG data filtered with SSP. Estimates of the average of right and left saccades showed the largest signal-to-noise ratio and no contamination with ocular artifacts across most of cortical surface and were further analyzed to examine cortical activity following the onset of saccades. Average dSPMs are displayed on the inflated hemispheres of the average brain of all subjects (N = 7). Significance is indicated with color bars.
Figure 3 illustrates the progression of estimated cortical activity following the saccade onset. Activity was prominent in occipital pole and calcarine sulcus starting at ~70 ms and spread in parallel through the dorsal and ventral visual streams to parietal, temporal and frontal cortices. Specifically, activity engaged posterior temporal cortex including putative motion-sensitive area MT+, and spread to posterior Sylvian fissure (PSF), planum temporale (PT) and superior insula (SI); and also recruited regions of frontoparietal networks including areas of superior parietal cortex and intraparietal sulcus, as well as the frontal eye field (FEF) and ventral precentral sulcus. Activity also spread to medial regions including parietooccipital (PO) cortex, precuneus and posterior cingulate; and recruited medial temporal and retrosplenial cortex (RSC). Within the ventral stream, activity recruited occipitotemporal and inferotemporal (IT) regions. Activation in cortical regions exhibited early peaks (range, 80–130 ms) that were followed by subsequent response components (Figures 3, 4) likely reflecting ongoing local processing and long-range network interactions.
Figure 3. Average dSPMs following the onset of saccades in Experiment 1 and external image movement that mimicked saccades in Experiment 2. (A) Experiment 1. Snapshots of average dSPMs at selected latencies after saccade onset. (B) Experiment 2. Snapshots of average dSPMs at selected latencies after saccade-like external image motion. Saccades and saccade-like external visual stimulation produced early-latency responses beginning ~70 ms after onset in occipital cortex and spreading through the ventral and dorsal visual streams to temporal, parietal and frontal cortices. Average dSPMs are displayed on the inflated hemispheres of the average brain of all subjects (N = 7). Significance is indicated with color bars.
Figure 4. Average time-courses of estimated currents in selected cortical regions following the onset of saccades and external image movement that mimicked saccades. Averages were computed across all subjects. Lines are mean responses; shaded areas are mean ± SEM. *Mark regions and time-courses corresponding to spatiotemporal clusters that exhibited significant differential responses after the onset of saccades vs. saccade-like background movement. These clusters are described in Figure 5 and Table 1.
The time-course of estimated cortical activity produced by saccades was visualized in regions of interest (ROIs; see “Materials and Methods” section). Regional waveforms, illustrated in Figure 4, were computed in each ROI and individual subject by averaging values across all voxels. Waveforms showed stable baselines and short-latency responses (range, ~70–110 ms) with multiple peaks and a time-course of ~400 ms, which were not correlated with the EOG saccade waveform. Further, regional waveforms estimated based on right and left saccade averages computed from the original data vs. after filtering with spatial and temporal SSP were similar (data not shown), indicating that activation estimates reflect neural sources rather than ocular artifacts (See “Materials and Methods” section).
Similar to the activity produced following the onset of saccades, cortical activity after the onset of saccade-like external visual stimulation produced early-latency responses in occipital pole and calcarine sulcus at ~70 ms (Figure 1Bc) and spread to temporal, parietal and frontal regions (Figure 3). Figure 4 illustrates regional waveforms in selected cortical areas. The early phase of the response in occipital pole was remarkably similar for saccades and saccade-like external visual stimulation, reflecting similar retinal stimulation across conditions. Differences in cortical activity following the onset of saccades vs. similar external visual stimulation were established using cluster-based analysis and then confirmed using parametric statistics as described below.
Differences in estimated cortical activity following the onset of saccades and saccade-like external image movement were statistically evaluated using the MEG signal from no-word trials and cluster-based statistical analysis across the whole cortex and response time (see “Materials and Methods” section). Specifically, paired t-tests were performed at each time point within 80–500 ms response-window and for each vertex on the cortex. Significant clusters (corrected p < 0.05) with time-courses between 150–350 ms after onset of image motion emerged in several cortical regions illustrated in Figure 5 and summarized in Table 1. Computation of the spatial overlap with anatomical regions segmented in FreeSurfer revealed that significant clusters overlapped with: (i) left lateral occipitotemporal (LOT) and nearby IT cortex; (ii) left PSF including PT; and (iii) medial PO, posterior cingulate and retrosplenial cortices. Regional responses in these ROIs are illustrated in Figure 4 to visualize the time-courses of differential activity across conditions. Activity baselines as well as latencies and earliest phase of responses were similar following saccades and saccade-like external image motion, providing further evidence that current source estimates reflect neural sources rather than saccadic artifacts.
Figure 5. Differences in estimated cortical activity following the onset of saccades vs. saccade-like external image movement. Differences were evaluated using cluster-based analysis across whole cortex and response time. Significant spatiotemporal clusters (corrected p < 0.05) with time-courses between 150–350 ms emerged in a left-lateralized cortical network. This network overlapped with anatomically defined: (i) left lateral occipitotemporal (LOT) and nearby inferotemporal (IT) cortex; (ii) left posterior Sylvian fissure (PSF), superior insula (SI) and nearby planum temporale (PT); and (iii) medial parietooccipital (PO), posterior cingulate (PCC) and retrosplenial cortex (RSC). Average t-score values over 150–350 ms response-window are illustrated for significant clusters only. Average t-score values are indicated with color bars.
Table 1. Results from spatiotemporal cluster-based analysis indentifying cortical regions and time-courses corresponding to significant differences in activity following the onset of saccades vs. background movement that mimicked saccades.
Computation of the spatial overlap between the left-lateralized occipitotemporal and temporal clusters and the word repetition priming effects (novel words vs. repeats) reported previously in this population (Temereanca et al., 2012) revealed that they are colocalized. In addition, this left-lateralized network colocalized with saccadic effects on responses to words at fixation, assessed using the early vs. late word presentation contrast (Temereanca et al., 2012).
For the cortical regions corresponding to significant clusters, the presence of differential activity during 155–225 ms was also tested using independent data from late word presentation trials and two-tailed t-tests, and was found significant (paired t-test, all three p’s < 0.03). Further, while significant differential activity within this time window was found in left LOT cortex (p < 0.03), differences within this time window were not significant in lower visual regions such as occipital pole and left occipitotemporal junction (p’s > 0.05) which provide the incoming afferent information. Also, no differential activity was found in the right LOT cortex (p > 0.05).
A repeated-measure one-way ANOVA with within-subjects factor of region of interest was conducted to compare the degrees of response change from 155–225 ms following the onset of saccades vs. saccade-like external visual stimulation in three selected ROIs, including occipital pole, left ventral occipitotemporal junction, and left LOT and nearby IT cortical cluster. The degrees of response change were significantly different between ROIs (29.7% vs. 42.6% vs. 70.0%, F(2,6) = 5.7154, p = 0.018). Pairwise tests revealed that compared with occipital pole, the degree of response change was significantly larger in left LOT and nearby IT cortex (29.7% vs. 70.0%, p = 0.006), but similar, albeit slightly increased, in left ventral occipitotemporal junction (29.7% vs. 42.6%, p = 0.32), a neighboring visual region processing afferent visual signals from occipital cortex.
Using a new approach to eliminate saccadic ocular artifacts from the MEG signal, the present study examined the spatiotemporal pattern of cortical activity following the onset of saccades as participants engaged in a one-back word recognition task. To evaluate extraretinal, central saccadic influences, the activity produced after the onset of saccades and spanning postsaccadic fixation before word appearance was compared with cortical activity following the onset of external visual stimulation that mimicked saccades. Results revealed robust differential activity in a left-lateralized cortical network during 150–350 ms response-window spanning fixation. Specifically, in line with previous research, differential activity overlapped with anatomically-defined medial PO, retrosplenial and posterior cingulate cortex, brain regions known to be implicated in visuospatial orientation, gaze self-monitoring and working memory (Haarmeier et al., 1997; Tikhonov et al., 2004; Binder et al., 2009; Galletti and Fattori, 2017). In addition, differential activity overlapped with left LOT and nearby IT cortex implicated previously in visual recognition, including visual word-form access (Tarkiainen et al., 1999; Solomyak and Marantz, 2009; Dehaene and Cohen, 2011); and left PSF and nearby multimodal cortex implicated previously in self-induced visual motion perception and language function (Thier et al., 2001; Wise et al., 2001). This left-lateralized network colocalized with word repetition priming effects as well as with known saccadic effects on responses to words presented at fixation (Temereanca et al., 2012), suggesting that central saccadic signals influence visual word processing in these regions. As discussed below, the present results could account for the pattern of various degrees of modulation across cortical regions produced by saccades on subsequent responses to words reported in Temereanca et al. (2012). Together, results provide the first evidence for central saccadic influences in a left-lateralized language network in occipitotemporal and temporal cortex above and beyond saccadic influences at preceding stages of information processing during visual word recognition.
Examining MEG waveforms around saccades is challenging due to ocular artifacts that obscure brain signals (Figure 1; Carl et al., 2012). In our previous report of cortical responses to words presented after saccades, we eliminated saccade-related ocular artifacts by subtracting the MEG signals generated by saccades alone, which include the eye-movement artifact and brain activity associated with saccades (Temereanca et al., 2012). Here we used a new approach to estimate the cortical activity following the onset of saccades in the absence of words at fixation, employing two complementary analyses. First, we averaged an equal number of right and left saccades, which effectively canceled artifacts with similar waveforms but of opposite polarity. In a second approach, using spatial and temporal SSP methods, we eliminated or significantly dampened ocular artifacts across MEG channels and only then averaged across right and left saccades. We conducted our analyses both for averages of right and left trials computed from the original data in the absence of SSP and with the SSP methods followed by this averaging procedure, and obtained similar results, which provides evidence that estimates reflect neural activity rather than contamination from ocular artifacts. Using these complementary analyses, we present here a first report of the spatiotemporal pattern of MEG estimated cortical activity following the onset of saccades.
The temporal and spatial signal projection methods employed here to dampen the saccadic artifacts have both strengths and weaknesses. Temporal signal projection relies on the EOG measurements to regress the artifact. The effectiveness of this method depends on the quality of the EOG recordings, and noise can have a deteriorating effect. While the spatial projection method does not depend on the quality of EOG directly, it assumes spatial stationarity of the MEG signal. If the subject moves, the effectiveness of SSP can be reduced. In summary, if good quality EOG data are available, the temporal signal projection is the preferred option.
Consistent with previous electrophysiological recordings of eye movement potentials in monkey (Purpura et al., 2003), saccades evoked a short-latency visual response ~70 ms after onset in occipital cortex. In addition, we found that the earliest occipital response components were virtually the same for saccades and saccade-like external visual stimulation, suggesting similar early processing of closely matching self-induced (saccadic) vs. external image motion. The visual response evoked by saccadic image motion, albeit not consciously perceived, may impact information processing at fixation via interactions between visual signals during and after saccades (Ibbotson and Cloherty, 2009; Temereanca et al., 2012). Later components of occipital responses exhibited differences across conditions that were not statistically significant using our analysis, although likely they reflect central saccadic signals known to modulate occipital activity during and after saccades (e.g., Sylvester and Rees, 2006; Temereanca et al., 2012).
Cortical activity following the onset of saccades spread in parallel through the ventral and dorsal visual pathways and encompassed temporal, parietal and frontal cortices, in agreement with previous human functional neuroimaging and monkey electrophysiological data. For example, consistent with studies of sensory-motor pathways, activity propagated from early visual cortex to motion-selective area MT+ (Tootell et al., 1995) as well as to superior parietal lobule, lateral intraparietal sulcus (LIP), FEF and ventral precentral sulcus, which are component regions of frontoparietal networks implicated in visual target selection, attention and saccade planning and execution (Colby and Goldberg, 1999; Galletti and Fattori, 2003, 2017; Yeo et al., 2011). Recent fMRI studies reported activation in these frontoparietal regions during natural text reading, consistent with a similar role in controlling eye movements in reading (Choi et al., 2014; Choi and Henderson, 2015). In addition, activity encompassed distributed cortical regions associated with visual, memory, and language functions, including midline precuneus and posterior cingulate cortex subserving visuospatial orientation and gaze self-monitoring; RSC and medial temporal regions supporting working memory (e.g., Mesulam, 1990; Yeo et al., 2011); and occipitotemporal and IT cortices implicated in visual recognition, including visual word recognition in reading. Overall, our new approach that employs anatomically-constrained MEG and cluster-based statistics opens up possibilities for future studies of the temporal relationship and putative interactions between visual, language, working memory and motor processes during complex behaviors such as natural reading.
Comparison of cortical activity following the onset of saccades vs. saccade-like external visual stimulation during one-back word recognition uncovered differential responses with time-courses between 155–225 ms in several cortical regions previously associated with distinct functions. Specifically, robust differential activity was found in PO cortex and PSF, consistent with previous monkey electrophysiology and human lesion studies that implicate these regions in the perception of self-induced image motion (Haarmeier et al., 1997; Thier et al., 2001; Tikhonov et al., 2004). PO cortex contains real motion cells that are activated by an object moving in the visual field, but not by the self-induced movement of its retinal image during a saccade; this cortical area is part of interconnected functional networks in the dorsal visual stream that are involved in different aspects of visual motion encoding, including the recognition of object motion and self motion (Galletti and Fattori, 2003, 2017). Interestingly, a network of interconnected regions at and near PSF that includes SI, ventral somatomotor cortex, and auditory cortex has been implicated in other functions critical for self-monitoring and language such as processing speech movements and hearing one’s own voice (Wise et al., 2001; Yeo et al., 2011).
In addition, we found differential responses in left LOT and nearby IT cortex. Since differences in occipital regions were significantly smaller, the emergence of disproportionately large, robust differences in left LOT cortex, the recipient of afferent visual signals from occipital regions, suggests central saccadic influences in this cortical area above and beyond saccadic influences in occipital cortex. Alternatively, it is possible that small response differences in occipital cortex are amplified by the local circuitry in left LOT cortex, without additional input from a saccade-related mechanism. A related potential factor is the difference in receptive field sizes across regions of the visual stream: visual stimuli would tend to activate more neurons in areas with larger receptive fields such as the LOT compared to the occipital cortex. However, this possibility alone is less likely since evidence from our previous study (Temereanca et al., 2012), discussed below, indicates different degrees of response changes in occipital and LOT cortex following saccades compared to saccade-like external visual stimulation.
Convergent evidence in our study supports a role of this latter differential activity in visual word processing. Firstly, we found that this differential activity is left-lateralized and colocalized with left-lateralized word repetition priming effects that are typically employed to study visual word processing. In addition, this differential activity also colocalized with saccadic modulation of word-evoked responses reported in Temereanca et al. (2012). The left LOT and nearby IT cortices have been implicated previously in word-form access and lexico-semantic processing, respectively (Halgren et al., 1994; Dehaene et al., 2002; Vinckier et al., 2007; Chan et al., 2011). We also found differential responses with a similar time-course overlapping left PT in Sylvian superior temporal cortex, an area previously implicated in grapheme-to-phoneme coding and known to be functionally coupled to the ventral word-form system (Dehaene et al., 2010; van der Mark et al., 2011).
The present results may explain the varying sizes of effects across cortical regions produced by saccades and external image-motion on subsequent word-evoked responses reported in Temereanca et al. (2012). This previous article, summarized in Figure 6A, revealed reduced behavioral performance and responses to words presented early vs. late after saccades as well as early vs. late after external image movement in occipital pole, left ventral occipitotemporal junction and LOT cortex, suggesting that visual effects attributed to image movement during saccades suppress subsequent word responses at re-fixation. Importantly, comparison of saccadic and external movement effects revealed more pronounced word-response modulations after saccades in occipital pole and left ventral occipitotemporal junction, suggesting influences of central saccadic signals in addition to visual influences of image movement. Further, these effects changed in left LOT cortex, reflecting a boost in the word response immediately after saccades but not after external image-motion. The present results suggest that this change reflects further modulation of word-evoked responses in left LOT cortex attributed to central saccadic influences (Figure 6B). Together with previous results, our new findings suggest that central saccadic signals modulate visual word processing in left LOT and nearby IT cortex above and beyond saccadic influences at preceding stages of information processing in lower visual areas.
Figure 6. Summary of saccadic contributions to cortical activity during a one-back visual word recognition task. (A) Differential effects of saccades and external image movement on word-evoked responses (modified from Figure 6, Temereanca et al., 2012) Cortical responses were reduced to words presented early vs. late after saccades, as well as to words presented early vs. late after external image movement that mimicked saccades, suggesting that retinal motion contributes to postsaccadic inhibition. Further, response attenuation was significantly larger in the saccade than external image movement condition in occipital pole (80–120 ms) and ventral occipital junction (120–160 ms), consistent with central postsaccadic influences on word processing in these regions. In contrast, the degrees of response modulations in left occipitotemporal cortex (165–215 ms) were similar across conditions, suggesting that central postsaccadic effects very across cortical regions. In particular, these effects on word-evoked responses changed in left LOT cortex, reflecting a boost in the word response immediately after saccades but not after external image-motion. (B) Cortical activity following the onset of saccades and saccade-like external image movement in the absence of words, in the same cortical regions as above. Robust differential activity following the onset of saccades vs. similar external visual stimulation emerged during 150–350 ms in left LOT cortex. Since differences in lower occipital regions were smaller, below statistical significance in our cluster analysis, the emergence of disproportionately large differences in left LOT cortex, the recipient of afferent visual signals from lower occipital regions, suggests central saccadic influences in this cortical area above and beyond saccadic influences in occipital cortex. Together with previous results, our new findings suggest that central saccadic signals modulate visual word processing in left LOT cortex above and beyond saccadic influences at preceding stages of information processing in occipital cortex.
Natural reading typically involves sequences of frequent, small (1–2°) saccades on target words embedded in text, and therefore differs from our experimental conditions in a number of ways, including the size of saccades, the presence of parafoveal information, and perceptual and cognitive processing difficulty. Human EEG measurements during reading (Kornrumpf et al., 2016) and monkey electrophysiology in occipital cortex during active vision (MacEvoy et al., 2008) suggest that saccadic signals interact with these factors and therefore may impact information processing in reading to a different degree and with a different time-course than in our experimental paradigm. The present results isolate effects of the saccade itself in occipitotemporal extending to nearby inferior temporal cortex, where they predict interactions between saccadic signals, attention and reading processes, including these factors.
Our findings complement active vision research that has revealed central saccadic signals at various levels of visual representation in the ventral stream. Electrophysiological recordings in primates have revealed central saccadic effects in thalamus and a number of visual cortical areas, including a biphasic modulation of visual responses consisting of transsaccadic suppression followed by postsaccadic enhancement (Reppas et al., 2002; Ibbotson and Krekelberg, 2011) as well as changes in oscillatory activity (Purpura et al., 2003; Rajkai et al., 2008). Central saccadic signals also modulate single-unit and local field potential (LFP) visual responses, functional connectivity, and oscillatory phase in regions of the temporal lobe in monkey, suggesting a role in high level visual recognition, memory processes and their coupling (Sobotka et al., 1997, 2002; Bartlett et al., 2011; Jutras et al., 2013). The timing and frequency properties of saccade-triggered LFP signals recorded simultaneously in occipital and temporal regions suggest that the nature of saccadic modulation varies across the cortex (Purpura et al., 2003). The present results are consistent with these previous findings, supporting the presence of distinct saccadic contributions to visual word processing in occipital and temporal cortex, respectively. These saccadic contributions are likely mediated by distributed occulomotor regions that control eye movements in both scene viewing and text reading (Choi et al., 2014; Choi and Henderson, 2015).
Because the retinal stimuli analyzed here consisted of two strings of five-crosses lacking orthographic-lexical attributes, our results suggest a task-dependent (rather than stimulus-driven) mechanism impacting the left occipitotemporal cortex during visual word recognition. In the present experiment, the task goals were identical across conditions and likely involved similar task strategies. It is possible, however, that central saccadic signals interact with attentional mechanisms, impacting processing at refixation. Eye movements and attention are controlled by overlapping neural circuits and have been proposed to interact during active vision (Ross et al., 2001; Purpura et al., 2003). Selective attention is known to enhance processing at various levels of visual word representation depending on task goals (Ruz and Nobre, 2008), and could contribute to activity in left occipitotemporal cortex during our one-back word recognition task. An interaction between central saccadic signals and attention could produce differential modulation of information processing in left occipitotemporal cortex following saccades, when central saccadic signals are present, vs. after similar external image motion, when such saccadic signals are absent. Future studies using complementary task designs and non-word stimuli can bring additional evidence in support of this hypothesis. For example, our results predict similar left-lateralized saccadic contributions when participants are engaged in reading tasks after memory-guided saccades. Further, these left-lateralized contributions are expected to lessen when subjects perform non-reading tasks, processing visual stimuli other than text.
Shifts in visuospatial attention prior to each saccade enhance visual processing at the saccade target (Chelazzi et al., 1993; Steinmetz and Moore, 2014), and have long been proposed to impact reading processes. A recent study combing EEG with eye tracking revealed larger parafoveal preview effects on the occipitotemporal N1 component in active reading with eye movements vs. passive rapid serial visual presentation with flankers (Kornrumpf et al., 2016). These results have been proposed to reflect enhanced parafoveal preprocessing of the upcoming word due to shifts in visuospatial attention prior to the saccade. Whether this mechanism contributes in part to the effects reported here for saccades to peripheral stimuli remain an interesting question for future research.
Another possibility explaining our results is that saccadic contributions to visual word processing vary with reading experience known to alter cortical organization and function. Indeed, literacy is known to enhance left occipitotemporal and PT activations evoked by written and spoken language, and their coupling (Carreiras et al., 2009; Dehaene et al., 2010). Further, literacy appears to mobilize dorsal stream mechanisms for visual motion perception as evidenced by enhanced visual motion function (V5/MT activity and coherent motion sensitivity) in good vs. poor readers and in typical readers vs. dyslexic individuals, whereas visual motion is similar for individuals matched on reading ability (Boets et al., 2011; Olulade et al., 2013). Thus, literacy may shape brain activations evoked by saccadic and external visual motion, and the strength of central saccadic influences in left occipitotemporal cortex and PT, regions undergoing critical changes during reading acquisition. Understanding saccadic contributions during reading and how they change with reading experience may provide important insights into the complex interplay between eye movements and visual, language, attention and memory processes underlying reading and its disorders.
Y-CC, SK, and STemereanca conceived and designed the current study. Y-CC, SK, STaulu and STemereanca performed the analyses for the study. Y-CC, SK, STaulu, GK, EB, MH and STemereanca wrote, edited and revised the manuscript.
This work was supported by National Institutes of Health (NIH) grants R03HD050627 (STemereanca), DP1OD003646 (EB), R01EB006385 (EB and MH), R01EB009048 (MH), National Institute of Biomedical Imaging and Bioengineering 5P41EB015896 (MH), NIH R01HD082527 (GK and MH) I-LABS Ready Mind Project and a grant from the Washington State Life Sciences Discovery Fund (LSDF) (STaulu) NIGMS R01 GM104948 (EB)and a Claflin Distinguished Scholars Award (STemereanca) and Executive Committee on Research (ECOR) Award (STemereanca) from Massachusetts General Hospital.
The authors declare that the research was conducted in the absence of any commercial or financial relationships that could be construed as a potential conflict of interest.
We thank Drs. Seppo Ahlfors and Annika Hulten for thoughtful comments on the manuscript.
Bartlett, A. M., Ovaysikia, S., Logothetis, N. K., and Hoffman, K. L. (2011). Saccades during object viewing modulate oscillatory phase in the superior temporal sulcus. J. Neurosci. 31, 18423–18432. doi: 10.1523/jneurosci.4102-11.2011
Berg, P., and Scherg, M. (1991). Dipole modelling of eye activity and its application to the removal of eye artefacts from the EEG and MEG. Clin. Phys. Physiol. Meas. 12, 49–54. doi: 10.1088/0143-0815/12/a/010
Berman, R., and Colby, C. (2009). Attention and active vision. Vision Res. 49, 1233–1248. doi: 10.1016/j.visres.2008.06.017
Binder, J. R., Desai, R. H., Graves, W. W., and Conant, L. L. (2009). Where is the semantic system? A critical review and meta-analysis of 120 functional neuroimaging studies. Cereb. Cortex 19, 2767–2796. doi: 10.1093/cercor/bhp055
Boets, B., Vandermosten, M., Cornelissen, P., Wouters, J., and Ghesquiere, P. (2011). Coherent motion sensitivity and reading development in the transition from prereading to reading stage. Child Dev. 82, 854–869. doi: 10.1111/j.1467-8624.2010.01527.x
Carl, C., Açik, A., König, P., Engel, A. K., and Hipp, J. F. (2012). The saccadic spike artifact in MEG. Neuroimage 59, 1657–1667. doi: 10.1016/j.neuroimage.2011.09.020
Carreiras, M., Seghier, M. L., Baquero, S., Estévez, A., Lozano, A., Devlin, J. T., et al. (2009). An anatomical signature for literacy. Nature 461, 983–986. doi: 10.1038/nature08461
Chan, A. M., Baker, J. M., Eskandar, E., Schomer, D., Ulbert, I., Marinkovic, K., et al. (2011). First-pass selectivity for semantic categories in human anteroventral temporal lobe. J. Neurosci. 31, 18119–18129. doi: 10.1523/jneurosci.3122-11.2011
Chelazzi, L., Miller, E. K., Duncan, J., and Desimone, R. (1993). A neural basis for visual search in inferior temporal cortex. Nature 363, 345–347. doi: 10.1038/363345a0
Choi, W., Desai, R. H., and Henderson, J. M. (2014). The neural substrates of natural reading: a comparison of normal and nonword text using eyetracking and fMRI. Front. Hum. Neurosci. 8:1024. doi: 10.3389/fnhum.2014.01024
Choi, W., and Henderson, J. M. (2015). Neural correlates of active vision: an fMRI comparison of natural reading and scene viewing. Neuropsychologia 75, 109–118. doi: 10.1016/j.neuropsychologia.2015.05.027
Colby, C. L., and Goldberg, M. E. (1999). Space and attention in parietal cortex. Annu. Rev. Neurosci. 22, 319–349. doi: 10.1146/annurev.neuro.22.1.319
Dale, A. M., Fischl, B., and Sereno, M. I. (1999). Cortical surface-based analysis: I. Segmentation and surface reconstruction. Neuroimage 9, 179–194. doi: 10.1006/nimg.1998.0395
Dale, A. M., Liu, A. K., Fischl, B. R., Buckner, R. L., Belliveau, J. W., Lewine, J. D., et al. (2000). Dynamic statistical parametric mapping: combining fMRI and MEG for high-resolution imaging of cortical activity. Neuron 26, 55–67. doi: 10.1016/S0896-6273(00)81138-1
Dale, A. M., and Sereno, M. I. (1993). Improved localizadon of cortical activity by combining EEG and MEG with MRI cortical surface reconstruction: a linear approach. J. Cogn. Neurosci. 5, 162–176. doi: 10.1162/jocn.1993.5.2.162
Dehaene, S., and Cohen, L. (2011). The unique role of the visual word form area in reading. Trends Cogn. Sci. 15, 254–262. doi: 10.1016/j.tics.2011.04.003
Dehaene, S., Le Clec’H, G., Poline, J. B., Le Bihan, D., and Cohen, L. (2002). The visual word form area: a prelexical representation of visual words in the fusiform gyrus. Neuroreport 15, 321–325. doi: 10.1097/00001756-200203040-00015
Dehaene, S., Pegado, F., Braga, L. W., Ventura, P., Nunes Filho, G., Jobert, A., et al. (2010). How learning to read changes the cortical networks for vision and language. Science 330, 1359–1364. doi: 10.1126/science.1194140
Dimigen, O., Sommer, W., Hohlfeld, A., Jacobs, A. M., and Kliegl, R. (2011). Coregistration of eye movements and EEG in natural reading: analyses and review. J. Exp. Psychol. Gen. 140, 552–572. doi: 10.1037/a0023885
Engbert, R., Nuthmann, A., Richter, E. M., and Kliegl, R. (2005). SWIFT: a dynamical model of saccade generation during reading. Psychol. Rev. 112, 777–813. doi: 10.1037/0033-295X.112.4.777
Fischl, B., Sereno, M. I., and Dale, A. M. (1999a). Cortical surface-based analysis: II: inflation, flattening, and a surface-based coordinate system. Neuroimage 9, 195–207. doi: 10.1006/nimg.1998.0396
Fischl, B., Sereno, M. I., Tootell, R. B., and Dale, A. M. (1999b). High-resolution intersubject averaging and a coordinate system for the cortical surface. Hum. Brain Mapp. 8, 272–284. doi: 10.1002/(sici)1097-0193(1999)8:4<272::aid-hbm10>3.0.co;2-4
Galletti, C., and Fattori, P. (2003). Neuronal mechanisms for detection of motion in the field of view. Neuropsychologia 41, 1717–1727. doi: 10.1016/s0028-3932(03)00174-x
Galletti, C., and Fattori, P. (2017). The dorsal visual stream revisited: stable circuits or dynamic pathways? Cortex 98, 203–217. doi: 10.1016/j.cortex.2017.01.009
Gramfort, A., Luessi, M., Larson, E., Engemann, D. A., Strohmeier, D., Brodbeck, C., et al. (2014). MNE software for processing MEG and EEG data. Neuroimage 86, 446–460. doi: 10.1016/j.neuroimage.2013.10.027
Haarmeier, T., Thier, P., Repnow, M., and Petersen, D. (1997). False perception of motion in a patient who cannot compensate for eye movements. Nature 389, 849–852. doi: 10.1038/39872
Halgren, E., Baudena, P., Heit, G., Clarke, J. M., Marinkovic, K., and Clarke, M. (1994). Spatio-temporal stages in face and word processing. I. Depth-recorded potentials in the human occipital, temporal and parietal lobes. J. Physiol. Paris 88, 1–50. doi: 10.1016/0928-4257(94)90092-2
Hämäläinen, M. S. (1995). Functional localization based on measurements with a whole-head magnetometer system. Brain Topogr. 7, 283–289. doi: 10.1007/bf01195254
Hämäläinen, M. S., and Ilmoniemi, R. J. (1994). Interpreting magnetic fields of the brain: minimum norm estimates. Med. Biol. Eng. Comput. 32, 35–42. doi: 10.1007/bf02512476
Hämäläinen, M. S., and Sarvas, J. (1989). Realistic conductivity geometry model of the human head for interpretation of neuromagnetic data. IEEE Trans. Biomed. Eng. 36, 165–171. doi: 10.1109/10.16463
Henderson, J. M., Choi, W., Lowder, M. W., and Ferreira, F. (2016). Language structure in the brain: a fixation-related fMRI study of syntactic surprisal in reading. Neuroimage 132, 293–300. doi: 10.1016/j.neuroimage.2016.02.050
Henderson, J. M., Choi, W., Luke, S. G., and Desai, R. H. (2015). Neural correlates of fixation duration in natural reading: evidence from fixation-related fMRI. Neuroimage 119, 390–397. doi: 10.1016/j.neuroimage.2015.06.072
Ibbotson, M. R., and Cloherty, S. L. (2009). Visual perception: saccadic omission—suppression or temporal masking? Hear. Res. 19, R493–496. doi: 10.1016/j.cub.2009.05.010
Ibbotson, M. R., and Krekelberg, B. (2011). Visual perception and saccadic eye movements. Curr. Opin. Neurobiol. 21, 553–558. doi: 10.1016/j.conb.2011.05.012
Jutras, M. J., Fries, P., and Buffalo, E. A. (2013). Oscillatory activity in the monkey hippocampus during visual exploration and memory formation. Proc. Natl. Acad. Sci. U S A 110, 13144–13149. doi: 10.1073/pnas.1302351110
Kornrumpf, B., Niefind, F., Sommer, W., and Dimigen, O. (2016). Neural correlates of word recognition: a systematic comparison of natural reading and rapid serial visual presentation. J. Cogn. Neurosci. 28, 1374–1391. doi: 10.1162/jocn_a_00977
Kucera, H., and Nelson Francis, W. (1967). Computational Analysis of Present-Day American English. Providence, RI: Brown University Press.
Lin, F. H., Belliveau, J. W., Dale, A. M., and Hämäläinen, M. S. (2006). Distributed current estimates using cortical orientation constraints. Hum. Brain Mapp. 27, 1–13. doi: 10.1002/hbm.20155
MacEvoy, S. P., Hanks, T. D., and Paradiso, M. A. (2008). Macaque V1 activity during natural vision: effects of natural scenes and saccades. J. Neurophysiol. 99, 460–472. doi: 10.1152/jn.00612.2007
Maris, E., and Oostenveld, R. (2007). Nonparametric statistical testing of EEG- and MEG-data. J. Neurosci. Methods 164, 177–190. doi: 10.1016/j.jneumeth.2007.03.024
Mesulam, M. (1990). Large-scale neurocognitive networks and distributed processing for attention, language, and memory. Ann. Neurol. 28, 597–613. doi: 10.1002/ana.410280502
Monosov, I. E., Sheinberg, D. L., and Thompson, K. G. (2011). The effects of prefrontal cortex inactivation on object responses of single neurons in the inferotemporal cortex during visual search. J. Neurosci. 31, 15956–15961. doi: 10.1523/jneurosci.2995-11.2011
Olulade, O. A., Napoliello, E. M., and Eden, G. F. (2013). Abnormal visual motion processing is not a cause of dyslexia. Neuron 79, 180–190. doi: 10.1016/j.neuron.2013.05.002
Pollatsek, A., Reichle, E. D., and Rayner, K. (2006). Tests of the E-Z Reader model: exploring the interface between cognition and eye-movement control. Cogn. Psychol. 52, 1–56. doi: 10.1016/j.cogpsych.2005.06.001
Purpura, K. P., Kalik, S. F., and Schiff, N. D. (2003). Analysis of perisaccadic field potentials in the occipitotemporal pathway during active vision. J. Neurophysiol. 90, 3455–3478. doi: 10.1152/jn.00011.2003
Rajkai, C., Lakatos, P., Chen, C. M., Pincze, Z., Karmos, G., and Schroeder, C. E. (2008). Transient cortical excitation at the onset of visual fixation. Cereb. Cortex 18, 200–209. doi: 10.1093/cercor/bhm046
Rayner, K. (2009). Eye movements and attention in reading, scene perception, and visual search. Q. J. Exp. Psychol. 62, 1457–1506. doi: 10.1080/17470210902816461
Reppas, J. B., Usrey, W. M., and Reid, R. C. (2002). Saccadic eye movements modulate visual responses in the lateral geniculate nucleus. Neuron 35, 961–974. doi: 10.1016/s0896-6273(02)00823-1
Ross, J., Morrone, M. C., Goldberg, M. E., and Burr, D. C. (2001). Changes in visual perception at the time of saccades. Trends Neurosci. 24, 113–121. doi: 10.1016/s0166-2236(00)01685-4
Ruz, M., and Nobre, A. C. (2008). Attention modulates initial stages of visual word processing. J. Cogn. Neurosci. 20, 1727–1736. doi: 10.1162/jocn.2008.20119
Schroeder, C. E., Wilson, D. A., Radman, T., Scharfman, H., and Lakatos, P. (2010). Dynamics of active sensing and perceptual selection. Curr. Opin. Neurobiol. 20, 172–176. doi: 10.1016/j.conb.2010.02.010
Schuster, S., Hawelka, S., Hutzler, F., Kronbichler, M., and Richlan, F. (2016). Words in context: the effects of length, frequency, and predictability on brain responses during natural reading. Cereb. Cortex 26, 3889–3904. doi: 10.1093/cercor/bhw184
Schuster, S., Hawelka, S., Richlan, F., Ludersdorfer, P., and Hutzler, F. (2015). Eyes on words: a fixation-related fMRI study of the left occipito-temporal cortex during self-paced silent reading of words and pseudowords. Sci. Rep. 5:12686. doi: 10.1038/srep12686
Sereno, S. C., and Rayner, K. (2003). Measuring word recognition in reading: eye movements and event-related potentials. Trends Cogn. Sci. 7, 489–493. doi: 10.1016/j.tics.2003.09.010
Sobotka, S., Nowicka, A., and Ringo, J. L. (1997). Activity linked to externally cued saccades in single units recorded from hippocampal, parahippocampal, and inferotemporal areas of macaques. J. Neurophysiol. 78, 2156–2163. doi: 10.1152/jn.1997.78.4.2156
Sobotka, S., Zuo, W., and Ringo, J. L. (2002). Is the functional connectivity within temporal lobe influenced by saccadic eye movements?. J. Neurophysiol. 88, 1675–1684. doi: 10.1152/jn.2002.88.4.1675
Solomyak, O., and Marantz, A. (2009). Lexical access in early stages of visual word processing: a single-trial correlational MEG study of heteronym recognition. Brain Lang. 108, 191–196. doi: 10.1016/j.bandl.2008.09.004
Steinmetz, N. A., and Moore, T. (2014). Eye movement preparation modulates neuronal responses in area V4 when dissociated from attentional demands. Neuron 83, 496–506. doi: 10.1016/j.neuron.2014.06.014
Sylvester, R., and Rees, G. (2006). Extraretinal saccadic signals in human LGN and early retinotopic cortex. Neuroimage 30, 214–219. doi: 10.1016/j.neuroimage.2005.09.014
Tadel, F., Baillet, S., Mosher, J. C., Pantazis, D., and Leahy, R. M. (2011). Brainstorm: a user-friendly application for MEG/EEG analysis. Comput. Intell. Neurosci. 2011:879716. doi: 10.1155/2011/879716
Tarkiainen, A., Helenius, P., Hansen, P. C., Cornelissen, P. L., and Salmelin, R. (1999). Dynamics of letter string perception in the human occipitotemporal cortex. Brain 122, 2119–2132. doi: 10.1093/brain/122.11.2119
Taulu, S., and Simola, J. (2006). Spatiotemporal signal space separation method for rejecting nearby interference in MEG measurements. Phys. Med. Biol. 51, 1759–1768. doi: 10.1088/0031-9155/51/7/008
Temereanca, S., Hamalainen, M. S., Kuperberg, G. R., Stufflebeam, S. M., Halgren, E., and Brown, E. N. (2012). Eye movements modulate the spatiotemporal dynamics of word processing. J. Neurosci. 32, 4482–4494. doi: 10.1523/JNEUROSCI.5571-11.2012
Thier, P., Haarmeier, T., Chakraborty, S., Lindner, A., and Tikhonov, A. (2001). Cortical substrates of perceptual stability during eye movements. Neuroimage 14, S33–S39. doi: 10.1006/nimg.2001.0840
Tikhonov, A., Haarmeier, T., Thier, P., Braun, C., and Lutzenberger, W. (2004). Neuromagnetic activity in medial parietooccipital cortex reflects the perception of visual motion during eye movements. Neuroimage 21, 593–600. doi: 10.1016/j.neuroimage.2003.09.045
Tootell, R. B., Reppas, J. B., Kwong, K. K., Malach, R., Born, R. T., Brady, T. J., et al. (1995). Functional analysis of human MT and related visual cortical areas using magnetic resonance imaging. J. Neurosci. 15, 3215–3230. doi: 10.1523/JNEUROSCI.15-04-03215.1995
Uusitalo, M. A., and Ilmoniemi, R. J. (1997). Signal-space projection method for separating MEG or EEG into components. Med. Biol. Eng. Comput. 35, 135–140. doi: 10.1007/bf02534144
van der Mark, S., Klaver, P., Bucher, K., Maurer, U., Schulz, E., Brem, S., et al. (2011). The left occipitotemporal system in reading: disruption of focal fMRI connectivity to left inferior frontal and inferior parietal language areas in children with dyslexia. Neuroimage 54, 2426–2436. doi: 10.1016/j.neuroimage.2010.10.002
Vinckier, F., Dehaene, S., Jobert, A., Dubus, J. P., Sigman, M., and Cohen, L. (2007). Hierarchical coding of letter strings in the ventral stream: dissecting the inner organization of the visual word-form system. Neuron 55, 143–156. doi: 10.1016/j.neuron.2007.05.031
Wise, R. J., Scott, S. K., Blank, S. C., Mummery, C. J., Murphy, K., and Warburton, E. A. (2001). Separate neural subsystems within ‘Wernicke’s area’. Brain 124, 83–95. doi: 10.1093/brain/124.1.83
Wurtz, R. H. (2008). Neuronal mechanisms of visual stability. Vision Res. 48, 2070–2089. doi: 10.1016/j.visres.2008.03.021
Keywords: saccades, visual motion, visual word recognition, reading, magnetoencephalography
Citation: Chang Y-CC, Khan S, Taulu S, Kuperberg G, Brown EN, Hämäläinen MS and Temereanca S (2018) Left-Lateralized Contributions of Saccades to Cortical Activity During a One-Back Word Recognition Task. Front. Neural Circuits 12:38. doi: 10.3389/fncir.2018.00038
Received: 18 October 2017; Accepted: 20 April 2018;
Published: 16 May 2018.
Edited by:
Michael M. Halassa, Massachusetts Institute of Technology (MIT), United StatesReviewed by:
Rossella Breveglieri, Università degli Studi di Bologna, ItalyCopyright © 2018 Chang, Khan, Taulu, Kuperberg, Brown, Hämäläinen and Temereanca. This is an open-access article distributed under the terms of the Creative Commons Attribution License (CC BY). The use, distribution or reproduction in other forums is permitted, provided the original author(s) and the copyright owner are credited and that the original publication in this journal is cited, in accordance with accepted academic practice. No use, distribution or reproduction is permitted which does not comply with these terms.
*Correspondence: Simona Temereanca, c2ltb25hX3RlbWVyZWFuY2FfaWJhbmVzY3VAYnJvd24uZWR1
† These authors have contributed equally to this work.
Disclaimer: All claims expressed in this article are solely those of the authors and do not necessarily represent those of their affiliated organizations, or those of the publisher, the editors and the reviewers. Any product that may be evaluated in this article or claim that may be made by its manufacturer is not guaranteed or endorsed by the publisher.
Research integrity at Frontiers
Learn more about the work of our research integrity team to safeguard the quality of each article we publish.