- Center for Systems Neuroscience, Department of Psychological and Brain Sciences, Boston University, Boston, MA, United States
This article provides a review of the effects of activation of muscarinic and nicotinic receptors on the physiological properties of circuits in the hippocampal formation. Previous articles have described detailed computational hypotheses about the role of cholinergic neuromodulation in enhancing the dynamics for encoding in cortical structures and the role of reduced cholinergic modulation in allowing consolidation of previously encoded information. This article will focus on addressing the broad scope of different modulatory effects observed within hippocampal circuits, highlighting the heterogeneity of cholinergic modulation in terms of the physiological effects of activation of muscarinic and nicotinic receptors and the heterogeneity of effects on different subclasses of neurons.
Introduction
Besides its role as a neurotransmitter in the peripheral nervous system, acetylcholine (ACh) in the central nervous system mainly acts as a neuromodulator by modulation of neuronal excitability, presynaptic release probability, postsynaptic responsiveness and synaptic plasticity. Thereby, ACh plays an important role in modulating cortical circuit activity. Empirical evidence indicates a role for ACh in normal physiological cognitive functions including attention to sensory stimuli (Sarter et al., 2005; Pinto et al., 2013; Bloem et al., 2014), coding of location and movement speed, learning and memory (Haam and Yakel, 2017) as well as a substantial role in regulating transitions between waking and sleep states (Xu et al., 2015). Previous articles have described detailed computational hypotheses about the role of cholinergic neuromodulation in enhancing the dynamics for encoding in cortical structures (Hasselmo, 2006) and the role of reduced cholinergic modulation in allowing consolidation of previously encoded information (Hasselmo, 1999). Rather than focus on these computational hypotheses, we aim to emphasize the broad scope of experimental data concerning the heterogeneity of different circuits and the cellular level modulatory effects of muscarinic and nicotinic receptors that will need to be addressed in future computational hypotheses.
Overall, cortical ACh is believed to enhance the signal-to-noise ratio (Hasselmo et al., 1995; Yu and Dayan, 2005; Hasselmo, 2006; Minces et al., 2017) and thus cortical sensitivity to external stimuli including attention and cue detection (Gritton et al., 2016). It also changes network dynamics in the hippocampal formation to allow more efficient encoding of novel stimuli (Hasselmo, 2006) that might underlie the effects of ACh on learning and memory behavior observed in rodents and human studies.
Evidence for the involvement of ACh in behavioral states associated with arousal comes from microdialysis measurements of ACh concentrations in rat brain lysates. These experiments revealed increases of ACh concentrations in the cortex and hippocampal formation during active waking and high arousal associated with presentation of novel stimuli or fear stimuli in comparison to quiet waking (Marrosu et al., 1995; Acquas et al., 1996). Furthermore, hippocampal ACh levels increase when animals are exposed to a novel spatial environment (Aloisi et al., 1997; Ceccarelli et al., 1999; Giovannini et al., 2001; Bianchi et al., 2003).
Notably, these changes in ACh concentrations in the brain are associated with changes in the overall electrical activity of the brain. In the hippocampal formation, this change in network activity is most easily observable as changes in theta rhythmic oscillatory activity, i.e., local field potential oscillations in the frequency range between 6–12 Hz in rodents peaking around 7–8 Hz in the running animal. Theta oscillations have been also recorded in the medial temporal lobe of human patients before undergoing brain surgery for epilepsy treatment. These recordings show task-dependent increases in lower frequency theta power during navigation in virtual environments (Kahana et al., 1999; Ekstrom et al., 2005). Recent recordings in humans while in motion in a real world arena showed a correlation between theta oscillation frequency and movement speed (Bohbot et al., 2017), very similar to the situation observed in rodents.
We will therefore begin our discussion of cholinergic modulation of neurons in the hippocampus and associated cortical regions with a discussion of the regulation of theta oscillations by ACh and its implication for memory processes.
Cholinergic Modulation of Theta Activity
Numerous experiments using different approaches including electrolytic lesions (Winson, 1978) or pharmacological inactivation (Chrobak et al., 1989) have shown that theta oscillations in the hippocampal formation are diminished when neuronal activity in the medial septum/diagonal band of Broca (MSDB) is inhibited. The MSDB consists of cholinergic, glutamatergic and GABAergic subpopulations with rhythmic activity of the Parvalbumin (PV+) GABAergic neurons leading hippocampal rhythmic activity (Hangya et al., 2009). Nevertheless, there is an association between theta oscillations and cholinergic activity. First, electrical stimulation of the medial septal area can induce theta oscillations in the hippocampal formation (Green and Arduini, 1954) and theta activity is correlated with cholinergic activity (Monmaur et al., 1997). Furthermore, cholinergic agonists can induce theta-rhythmic activity patterns in the in vitro hippocampal slice preparation (Konopacki et al., 1987). Second, inactivation of the MSDB cholinergic subpopulation by focal injections of atropine, a muscarinic acetylcholine receptor (mAChR) antagonist, into the MSDB inhibits a lower frequency component (4–6 Hz) of theta oscillations in rats and rabbits (Kramis et al., 1975). A second higher frequency component (8–12 Hz) of theta oscillations was not affected by focal atropine injections. These experiments revealed the existence of an atropine-sensitive or cholinergic activity-dependent theta component and an atropine-resistant higher frequency theta component, which was most prominent during movement and is believed to be primarily driven by entorhinal cortex (EC) activity. Third, more recent development of amperometric measurements of cholinergic activity, which work on the time-scale of seconds (Burmeister et al., 2008), demonstrate ACh release occurs over many seconds after the appearance of spontaneous or induced theta oscillations in urethane-anesthetized rats (Zhang et al., 2010). Fourth, optogenetic activation of cholinergic medial septal neurons induces hippocampal theta oscillations (Dannenberg et al., 2015) and suppresses sharp wave-ripple events (Vandecasteele et al., 2014), which are the hallmark of a hippocampal network state characterized by the absence of theta oscillations.
The vast majority of cholinergic fibers in the hippocampal formation arise from the innervation by the cholinergic MSDB projection neurons, which are also integrated in the MSDB network which paces hippocampal rhythmic activity. Thus, under physiological conditions, the increase of ACh concentrations in hippocampal (and cortical) structures coincides with changes in hippocampal rhythmic activity paced by the rhythmic activity of the GABAergic MSDB neurons (Stewart and Fox, 1990; Tóth et al., 1997; Varga et al., 2008; Hangya et al., 2009). In addition, in vitro data utilizing a complete septohippocampal preparation showed carbachol application-induced theta-like hippocampal oscillatory field potential activity that was synchronized with rhythmic IPSPs and rebound spiking in Ih expressing GABAergic MSDB neurons, which may generate or maintain theta rhythmic activity in the septohippocampal circuit (Manseau et al., 2008). We hypothesize that these changes act together to promote the processing and encoding of novel information into episodic memory. We will therefore briefly discuss the general function of theta oscillations for memory processing and then continue our discussion on the cellular effects of ACh and behavioral consequences.
Theta rhythmic activity is temporally structured and thus coordinates activity at different levels ranging from between-brain area synchronization to the organization of synaptic activity. The coordination of neuronal activity between brain regions has been shown for prefrontal cortex and hippocampus in spatial working memory tasks in rodents (Hyman et al., 2005; Jones and Wilson, 2005; Benchenane et al., 2010). Furthermore, between area phase synchronization of theta oscillations between visual cortex area V4 and the lateral prefrontal cortex have been observed in humans performing a visual memory task (Liebe et al., 2012). This synchronization on the local field potential level extended to the synchronization of spiking activity between V4 and the lateral prefrontal cortex. Similar spike-theta phase synchronizations have been shown between and within different brain regions in rodents (Skaggs et al., 1996; Lisman and Jensen, 2013) as well as in the medial temporal lobe in humans, where the tight coupling of spiking activity and the underlying theta oscillations predicted successful memory formation (Rutishauser et al., 2010). The temporal structure provided by theta oscillatory activity also provides temporal windows for local circuit computations (Mizuseki et al., 2009). The same theta frequency stimulus can induce both long-term potentiation (LTP) or long-term depression (LTD), depending on which phase of the theta oscillation it is delivered (Huerta and Lisman, 1995; Hyman et al., 2003). Taken together, these data show synaptic activity, spiking activity and local circuit computations differ at the peak and trough of local theta oscillations. These different activity patterns become even more obvious when looking at recordings of gamma activity (25–110 Hz) superimposed on theta oscillations. These data show that the magnitude and frequency of gamma oscillations change between the peak and trough phases of the theta cycle (Bragin et al., 1995; Belluscio et al., 2012). In hippocampal CA1, slow gamma (~25–55 Hz) occurs at the trough of local theta oscillations and is driven by input from the CA3 region, whereas fast gamma (~60–110 Hz) occurs around the peak of the local theta cycle and coincides with inputs from layer III medial EC (Colgin et al., 2009). The temporal coordination of entorhinal and CA3 inputs in concert with recruiting local inhibition controls spike timing in CA1 neurons and consequently phase precession in CA1 place cells, as shown recently by Fernández-Ruiz et al. (2017). Systemic administration of muscarinic receptor blockers weakens the phase relationship of gamma to theta in the EC (Newman et al., 2013). Finally, the different activity states temporally defined and separated by the opposite phases of the theta oscillation might reflect separate computational time windows for encoding and retrieval processes (Hasselmo et al., 2002) as discussed in greater detail below.
Acetylcholine Effects on Memory
In humans, pharmacological disruption of cholinergic function by systemic administration of the muscarinic receptor antagonist scopolamine impairs new word encoding for subsequent free recall (Ghoneim and Mewaldt, 1977) or paired-associate learning (Atri et al., 2004). Systemic administration of scopolamine in humans impairs both object and spatial n-back working memory (Green et al., 2005). In the same study, simultaneous application of scopolamine and the nicotinic receptor antagonist mecamylamine produced even greater impairments, suggesting synergistic actions of muscarinic and nicotinic receptor activation for this kind of working memory. In rodents, pharmacological blockade of either hippocampal nicotinic receptors or M1 muscarinic receptors by local drug injections in rats impairs memory performance in 8-arm radial maze tasks (Ohno et al., 1993, 1994) and direct injection of scopolamine into the dorsal hippocampus impairs encoding of spatial information in the Morris water maze-task (Blokland et al., 1992). Importantly, local injections of scopolamine into the hippocampal CA3 or CA1 subfields in rats performing the modified Hebb-Williams maze-task selectively disrupted encoding of spatial information, while sparing retrieval (Rogers and Kesner, 2003). Conversely, enhancing ACh levels in CA3 or CA1 by local injections of the acetylcholinesterase (AChE) inhibitor physostigmine selectively disrupted retrieval, but spared encoding. In addition, activation of mAChR on apical dendrites of CA1 hippocampal pyramidal neurons leads to cytosolic calcium rises which acts to amplify nuclear calcium rises in response to trains of action potentials, modulating gene transcription (Power and Sah, 2002). Taken together with the positive effects of hippocampal ACh on synaptic plasticity and theta oscillations, these data favor a model in which high levels of ACh promote an encoding state of the entorhinal-hippocampal network.
Cellular Effects of Acetylcholine
One possible mechanism contributing to the maintenance of information during working memory tasks as well as during the encoding of novel information is the intrinsic capacity of individual neurons to exhibit persistent spiking activity. This intrinsic persistent spiking has been demonstrated in vitro in neurons of the medial EC layer II (Klink and Alonso, 1997), medial EC superficial layer III (Yoshida et al., 2008) and deep layer V (Egorov et al., 2002), lateral EC layer III (Tahvildari et al., 2007), dorsal presubiculum (Yoshida and Hasselmo, 2009) as well as hippocampal subregions CA1 (Knauer et al., 2013) and CA3 (Jochems and Yoshida, 2013) in rats. In vitro, these neurons can fire for minutes after an initial depolarizing current injection, if the cholinergic agonist carbachol (Klink and Alonso, 1997; Egorov et al., 2002) or an agonist of the metabotropic glutamate receptor is present in the bath solution (Yoshida et al., 2008). Persistent spiking activity of neurons in layer V of the medial EC has been shown to be graded and can be maintained at different frequencies for many minutes (Egorov et al., 2002). This graded persistent firing could allow these neurons to integrate synaptic input over extended periods. Mechanistically, the induction of persistent spiking has been attributed to the activation of a Ca2+ sensitive cationic current (Jochems and Yoshida, 2013; Knauer et al., 2013).
These intrinsic cellular mechanisms could contribute to persistent spiking that has been observed in vivo, albeit the in vivo activity may depend on network dynamics. Recordings performed by Suzuki et al. (1997) show a sample-specific delay of activity in the EC during the delay intervals of a place memory task in macaques, and Young et al. (1997) observed prolonged odor-selective activity throughout or at the end of the memory delay period of an odor-guided delayed nonmatching-to-sample task in rats. Furthermore, recordings from head-direction cells in the dorsal presubiculum have shown that these neurons continue to spike when the animal’s head remains in the preferred direction of the cell (Taube and Muller, 1998), thus showing a very similar persistence of spiking activity. Graded persistent spiking activity is not limited to areas of the hippocampal formation, but can also be observed in other areas, such as the oculomotor system (Robinson, 1972), the somatosensory system (Romo et al., 1999), or the head direction system (Taube and Bassett, 2003). These cellular effects could contribute to network persistent activity observed during delayed matching tasks in human subjects as well (Schon et al., 2004). This persistent activity is reduced by systemic administration of muscarinic receptor blockers (Schon et al., 2005).
Acetylcholine Receptors in the Hippocampal Formation
In area CA1 of the hippocampus, only approximately 7% of axon terminals from cholinergic neurons form synaptic junctional specializations (Umbriaco et al., 1995), and immunoelectron microscopic studies revealed a low frequency rate of synaptic membrane differentiations on choline acetyltransferase (ChAT)-immunostained cholinergic axon terminals in various regions of the CNS including the hippocampus, suggesting that diffuse transmission by ACh prevails in many regions of the CNS (Descarries et al., 1997). These anatomical data thus indicated that ACh is released in a manner described as volume transmission, i.e., ACh is released from the axonal terminals into the extracellular space. This view, however, was recently challenged by Takacs et al. (2017) demonstrating that all hippocampal cholinergic terminals establish synapses, and vesicles dock only at synapses. Nonetheless, ACh release in the hippocampal formation affects multiple cells and cellular compartments, which further contributes to network complexity. First, ACh acts via different subtypes, namely nicotinic and mAChR. Second, these receptors are differentially expressed on multiple interneuron subtypes and principal cells (Levey et al., 1995; Picciotto et al., 2012), as well as astrocytes (Van Der Zee et al., 1993; Sharma and Vijayaraghavan, 2001; Pabst et al., 2016). Third, these receptors are also found at different cellular compartments. It is therefore a difficult task to decipher the individual contributions of all these factors to the overall network effects of ACh. We will begin the discussion of the different cellular effects of ACh with a description of the nicotinic and muscarinic receptor types in the hippocampal formation and the functional consequences of their activation and will then summarize the cholinergic effects on the various interneuron subtypes in hippocampus and neocortical regions.
Cellular Effects of Nicotinic Receptor Activation in Principal Neurons
The nicotinic acetylcholine receptor (nAChR) is an ionotropic receptor built as a homo- or heteromeric pentamer, which can be activated pharmacologically by the drug nicotine and functions as a non-selective, excitatory cation channel (Changeux et al., 1998; Picciotto et al., 2012). On a behavioral level, local infusion of nicotinic antagonists into the hippocampus results in spatial location memory impairment in rats (Ohno et al., 1993) highlighting the positive effect of nicotinic receptor activation on encoding. The predominant nAChR type in the hippocampus is the (α7)5 homomer (Séguéla et al., 1993; Radcliffe et al., 1999), followed in expression levels by the heteromeric (α4)2(β2)3 and (α3)2(β4)3 channel compositions (Zoli et al., 1998; Radcliffe et al., 1999).
Data from early experiments using intracellular recordings in guinea-pig hippocampal slices (Benardo and Prince, 1982a, b) demonstrated that perfusion of slices with medium containing the muscarinic antagonists atropine or scopolamine blocked the majority of ACh actions on CA1 pyramidal cell membrane potential changes pointing to a dominant role of muscarinic, but not nicotinic, receptors in modulation of cellular excitability. However, functional calcium imaging with Fura-2-acetoxymethyl ester revealed functional α7 nAChRs in CA3 principal cells as well as dentate gyrus (DG) granule cells (Grybko et al., 2010). Likewise, in region CA1 of rat hippocampal slices in vitro, stimulation with choline, which is a selective α7 nAChR agonist, in combination with an allosteric modulator of α7 nAChRs evoked small but reliable membrane depolarizations of about 4 mV (Kalappa et al., 2010). Taken together, these experiments provide evidence for functional somato-dendritic α7 nAChRs on DG granule as well as hippocampal pyramidal cells.
Importantly, there is a strong functional expression of α7 nAChRs on glutamatergic presynaptic terminals inside region CA3 (Gray et al., 1996), which can enhance the release of glutamate via protein kinase A activation (Cheng and Yakel, 2014). Activation of these receptors induced high-frequency bursts of miniature excitatory postsynaptic currents (mEPSCs) in CA3 pyramidal cells in rat hippocampal slices (Gray et al., 1996; Sharma and Vijayaraghavan, 2003). Such mEPSCs were sufficient to drive postsynaptic spiking in the absence of incoming action potentials, which were inhibited by tetrodotoxin application (Sharma and Vijayaraghavan, 2003). Consistent with these observations, nicotine has been demonstrated in vitro to increase intracellular Ca2+ in mossy fiber presynaptic terminals and to enhance the frequency of mEPSCs, as well as miniature inhibitory postsynaptic currents (mIPSCs) recorded from CA3 neurons in rat hippocampal slices (Radcliffe et al., 1999). Likewise, nicotine application caused a short initial reduction followed by a longer period of enhancement of stimulation-induced field excitatory postsynaptic potential (EPSP) amplitudes (Giocomo and Hasselmo, 2005). This effect was selective for stratum lacunosum-moleculare, and was absent in stratum radiatum of CA3. However, this effect was blocked by GABA antagonists indicating it was mediated by effects on GABAergic transmission. These results indicate selective nicotinic receptor-mediated enhancement of afferent inputs to hippocampal CA3, whereas recurrent excitation appears to remain unaffected. The nicotinic enhancement of synaptic transmission in the hippocampal formation is consistent with the proposed role of nicotinic receptors in enhancing thalamic input to neocortical structures (Gil et al., 1997; Disney et al., 2007) and the output of cortical neurons (Poorthuis et al., 2013). These network effects of nicotine in neocortical structures may serve to enhance mechanisms of attention (McGaughy and Sarter, 1998; Bloem et al., 2014).
Cellular Effects of Muscarinic Acetylcholine Receptor Activation in Principal Neurons
In contrast to the ionotropic nature of the nicotinic AChR type, the muscarinic AChR, which is activated by the drug muscarine, is metabotropic, i.e., it acts via functional coupling and activation of heteromeric G proteins. Five subtypes of mAChR have been identified and termed M1–5. The receptor types M1, M3 and M5 are coupled to Gq proteins which activate phospholipase C, which leads to Ca2+ influx and activation of intracellular signaling cascades. In contrast, M2 and M4 receptors are coupled to Gi/o proteins, that inhibit the enzyme adenylyl cyclase and thereby reduce the production of cAMP (Wess, 2003). A quantification of relative proportions of the M1–M5 mAChR subtypes with immunoprecipitation followed by a radioligand binding assay in post-mortem tissue of the human hippocampus found about 60% M1, 20% M2, 15% M4 and roughly 5% M3 receptor expression (Flynn et al., 1995). Similarly, the same method applied to rat hippocampal tissue revealed a proportion of about 36% M1, 33% M2 and 27% M4 receptor expression. M3 receptors were not examined (Levey et al., 1995). Although M5 mRNA can be detected by in situ hybridization in CA1 pyramidal cells of the rat hippocampus (Vilaró et al., 1990), the protein expression is very low (Wall et al., 1992) with unknown functional significance. Hence, M1, M2, and M4 are the most prevalent receptor subtypes in the hippocampus. The expression of the M2 muscarinic subtype is restricted to interneurons, thus is not present on principal cells (Levey et al., 1995). M1 is widely distributed within the hippocampus and preferentially expressed in somata and dendrites of hippocampal pyramidal and DG granule cells (Levey et al., 1995; Yamasaki et al., 2010), with only a small fraction expressed on axons and terminals. From a functional perspective, M1 receptors are mainly responsible for the modulation of pyramidal cell excitability upon transient/phasic ACh application in slices (Gulledge and Kawaguchi, 2007).
Pioneering studies of cholinergic effects on hippocampal pyramidal cells using intracellular recordings in combination with pharmacology utilizing the in vitro guinea pig hippocampal slice preparation by Benardo and Prince (1982a) demonstrated a slow and long-lasting depolarization of CA1 pyramidal neurons and increases in spike frequency upon drop or iontophoretic application of ACh to the dendritic region in stratum oriens and stratum radiatum. The observed muscarinic cholinergic depolarization occurred as a result of blockade of voltage-dependent K conductance (distinct from that of the delayed rectifier; Benardo and Prince, 1982c) resembling the M-current initially discovered in bullfrog sympathetic ganglion cells (Brown and Adams, 1980). Additionally, these findings are consistent with subsequent studies utilizing rat hippocampal slices showing a slow depolarization of pyramidal cells via cholinergic modulation through suppression of a leak potassium current (Cole and Nicoll, 1984).
Interestingly, CA1 and CA3 principal cells respond differently to such phasic local ACh puff applications. ACh application near the somata of CA1 principal cells resulted in a 2.5 mV hyperpolarization of the membrane potential and inhibited action potential generation via calcium dependent activation of small conductance calcium activated potassium (SK) channels, Dasari and Gulledge (2011) while ACh applied focally to CA3 principal cell somata generated a small depolarization of about 0.6 mV. In contrast to phasic application, tonic cholinergic modulation of CA1 principal cells via application of carbachol decreases medium afterhyperpolarizations (AHPs) and the early component of the slow AHPs, generated afterdepolarizations (ADPs) via M1 mAChRs, and depolarized CA1 principal neurons via M1 and M3 mAChRs (Dasari and Gulledge, 2011) consistent with previous studies (Madison and Nicoll, 1984; Madison et al., 1987) showing a reduction of spike frequency accommodation due to a blockade of calcium-activated potassium slow AHPs.
Besides M1, M4 is the other major mAChR subtype responsible for direct cholinergic modulation of the excitatory hippocampal circuit. In contrast to the preferential somato-dendritic localization of M1, M4 is mainly located in glutamatergic terminals and mediates cholinergic presynaptic inhibition of Schaffer collateral EPSPs in vitro (Shirey et al., 2008; Dasari and Gulledge, 2011). As reviewed previously (Hasselmo, 2006), the cholinergic presynaptic inhibition of glutamatergic synaptic transmission in the hippocampus has been shown in a wide range of studies in vitro (Hounsgaard, 1978; Valentino and Dingledine, 1981; Lambert and Teyler, 1991) and in vivo utilizing recordings of CA1 field potential responses evoked by ipsilateral CA3 (Herreras et al., 1988) or commissural stimulation in anesthetized rats (Rovira et al., 1983), consistent with earlier findings by Leung and Vanderwolf (1980) showing that injections of atropine sulfate severely dampen the oscillations in averaged evoked potentials in CA1 of rats during walking or similar movements. ACh mediates a stronger presynaptic inhibition of the midapical than basal and distal apical excitation (Leung and Péloquin, 2010) and presynaptic muscarinic inhibition has been shown to be stronger at excitatory recurrent connections and the Schaffer collaterals compared to the afferent input from EC (Hasselmo and Schnell, 1994; Hasselmo et al., 1995).
Cellular Effects of Nicotinic Receptor Activation in Interneurons
In contrast to pyramidal and granule cells, interneurons can have very large nAChR currents with fast kinetics. However, these effects vary substantially between different neuronal subtypes within the hippocampal formation (McQuiston and Madison, 1999c; McQuiston, 2014). Activation of nicotinic currents, mainly mediated by the α7 subtype, have been observed in recordings of stratum radiatum interneurons in the hippocampus (Jones and Yakel, 1997; Frazier et al., 1998; McQuiston and Madison, 1999c) and in DG molecular layer interneurons, hilar interneurons and the glutamatergic mossy cells of the dentate hilus (Frazier et al., 2003). These currents have been also observed in oriens-lacunosum moleculare (O-LM) interneurons in rat hippocampal slices (Alkondon et al., 1999; McQuiston and Madison, 1999c). In addition, Alkondon et al. (1999) also found slow but long-lasting depolarizations mediated by activation of α4β2 nicotinic receptors in both stratum radiatum and O-LM interneurons. In contrast to these experiments, which used pressure injections of ACh, a more recent study from Bell et al. (2011) used optogenetics to trigger synaptic release of ACh. Similar to the results by Alkondon et al. (1999), they found that optogenetic activation of cholinergic fibers resulted in mostly subthreshold depolarizations with slow kinetics mediated by the activation of α4β2 nicotinic receptors in CA1 O-LM interneurons. However, they did not find α7 nAChR mediated currents in O-LM interneurons, raising the possibility that the type of stimulation (optogenetic vs. pressure injection) might bias activation of nicotinic receptor subtypes. Nevertheless, experimental data indicates that, in general, any of the individual interneuron morphological subtypes can be modulated by all three nAChR subtypes (Alkondon and Albuquerque, 2004). A significant portion of the network effects of ACh likely results from the modulation of specific subtypes of inhibitory interneurons, which are in a powerful position to control rhythmic activity, and synaptic inputs to and spiking output from pyramidal neurons (McQuiston and Madison, 1999a; McQuiston, 2014).
Cellular Effects of Muscarinic Acetylcholine Receptor Activation in Interneurons
In contrast to the mainly slow depolarizing synaptic response mediated by mAChR activation in principal cells, hippocampal interneurons respond with a much greater diversity regarding the waveform of synaptic potentials, as shown for CA1 interneurons in rat hippocampal slices with electrical (McQuiston and Madison, 1999a; Widmer et al., 2006) or optogenetic stimulation of synaptic ACh release (Bell et al., 2013). Benardo and Prince (1982a) provided one of the earliest observations of ACh-mediated excitation of hippocampal inhibitory interneurons and subsequent inhibition of pyramidal neurons in guinea pig slices. Cholinergic excitation of hippocampal interneurons was later confirmed using recordings in rat hippocampal slices, and this excitation was mostly blocked by muscarinic antagonists (Reece and Schwartzkroin, 1991).
Consistently, interneurons that responded to optogenetically released ACh predominantly had muscarinic (80%) vs. nicotinic (17%) mediated changes in membrane potential, and only 3% of interneurons had mixed responses (Bell et al., 2013). In the studies from Widmer et al. (2006) and Bell et al. (2013) the majority of interneurons (64% and 40%, respectively) responded with an atropine-sensitive (i.e., muscarinic) slow depolarization upon synaptic ACh release. In those studies, 13% and 25%, respectively, responded with a biphasic hyperpolarization and depolarization, and 20% and 35%, respectively, showed a pure hyperpolarizing response. Depolarization of hippocampal interneurons by bath application of carbachol, a cholinergic agonist, can induce theta-frequency membrane potential oscillations in vitro, which has been suggested to contribute to intrinsically generated theta-rhythmic firing of pyramidal neurons due to rebound spiking (Chapman and Lacaille, 1999). However, only a minor fraction of cells in the study by Widmer et al. (2006) responded with membrane potential oscillations (2%) without any obvious correlation to a morphological classification of the different interneurons. In the study by Bell et al. (2013), the hyperpolarizing response observed in a subset of interneurons was demonstrated to be mediated via activation of an inwardly rectifying potassium channel by activation of the M4 mAChR subtype, whereas the depolarizations were likely produced by M3 receptor activation. Considering the correlation of ACh levels with different functional network states, it is noteworthy that hyperpolarizing responses required less optogenetic stimulation strength, i.e., less synaptic ACh release, than depolarizing responses. This favors a model proposed by McQuiston (2014), in which low levels of ACh favor disinhibition, whereas higher levels of ACh favor inhibition of hippocampal principal cells. If interneurons are depolarized by M1/M3 mAChR activation, this can lead to consistently enhanced firing frequency and the production of ADPs, as shown for O-LM interneurons (Lawrence et al., 2006b) as well as basket cells (Cea-del Rio et al., 2010) in CA1. Concomitantly, mAChR activation enhances firing reliability and precision to theta frequency input in O-LM (Lawrence et al., 2006a) as well as Cholecystokinin (CCK+) positive Schaffer collateral associated and basket cells (Cea-del Rio et al., 2011). Despite the effect of increasing IPSC frequency in CA1 and CA3 pyramidal neurons, activation by carbachol significantly decreased the amplitude of monosynaptically evoked IPSCs mediated by perisomatic inhibitory interneurons indicating postsynaptic depolarization of interneurons is combined with the presynaptic inhibition of inhibitory transmitter release (Pitler and Alger, 1992; Behrends and ten Bruggencate, 1993; Szabó et al., 2010). Presynaptic M2-type mAChRs were responsible for the reduction in IPSC amplitude in CA3 axo-axonic and PV+ basket cell-pyramidal cell pairs, whereas postsynaptic M1/M3 receptors in pyramidal cells triggered the synthesis of endocannabinoids, which activated type 1 cannabinoid receptors (CB1) at the terminals of CCK+ basket cells, resulting in reduced GABA release (Fukudome et al., 2004; Szabó et al., 2010). Besides their abundant presynaptic location on inhibitory terminals, M2 mAChRs are also expressed postsynaptically in dendrites and somata of hippocampal interneurons located inside or close to stratum oriens as well as hilar interneurons (Hájos et al., 1998; Rouse et al., 1998). Furthermore, M2 mAChRs are located not only on non-cholinergic, but also cholinergic terminals (Rouse et al., 2000), where they can function as presynaptic autoreceptors, the activation of which inhibits ACh release, as shown in synaptosomes from rat hippocampus (Raiteri et al., 1984).
Cholinergic Effects Based on Molecular Markers for Interneuron Subtypes
Interneurons display an immense functional, morphological and genetic diversity, as described in previous reviews of the classification of interneuron subtypes (McBain and Fisahn, 2001; Somogyi and Klausberger, 2005; Rudy et al., 2011; Kepecs and Fishell, 2014). The previous sections focused on different morphological subtypes identified by anatomical features of the neurons, whereas this section will focus on differences in cholinergic effects based on different molecular markers for interneurons. Distinct expression profiles of neuropeptides that relate to points of synaptic contact, morphological structure and intrinsic excitability (Freund and Buzsáki, 1996; Kepecs and Fishell, 2014) distinguish interneurons. These characteristics contribute to shaping the spike timing of downstream neurons (Klausberger and Somogyi, 2008), mediating synchronization and rhythmicity (Cobb et al., 1995) with respect to local ongoing network rhythms, and balancing the excitatory gain through divisive (or arrhythmic) inhibitory control. Their pivotal role in local computation lies in their ability to innervate specific sublaminar populations as well as subcellular compartments. Thus, cholinergic modulation of interneuron activity can have strong influences on local network computations. However, there is still a limited understanding of the functional role of the differential cholinergic responses in the different subtypes of interneurons. Here, we summarize some of the current observations of cholinergic effects on different interneuron subtypes in hippocampus and neocortex.
Interneurons expressing the calcium binding protein parvalbumin (PV) make up approximately 40% of all GABAergic interneurons. However, this is a heterogeneous group of functionally distinct interneuron subtypes. For example, in the hippocampus alone, there are at least three functionally and morphologically distinct populations of PV+ expressing interneurons, namely basket, axo-axonic and bistratified cells. Fast spiking interneurons in the hippocampus and neocortex are often PV+ positive and target the soma of pyramidal cells. In general, these fast spiking PV+ interneurons elicit a range of responses to muscarinic activation in different brain areas. In the frontal cortex fast firing PV+ cells have been shown to be unresponsive to mAchR activation (Kawaguchi, 1997; Gulledge et al., 2007). In the visual cortex, however, PV+ cells have been demonstrated to show mildly hyperpolarizing changes in membrane potential in response to mAchR activation (Xiang et al., 1998). Muscarinic receptors cause presynaptic inhibition of GABA release from fast firing interneurons in thalamocortical slices that contrasts with nicotinic-mediated enhancement of thalamocortical excitatory inputs on pyramidal neurons (Kruglikov and Rudy, 2008). In contrast, a mixture of depolarizing, hyperpolarizing and biphasic responses to muscarinic activation of PV+ basket cells have been observed in slices of the hippocampus (Bell et al., 2013; Yi et al., 2014). In addition to changes in baseline resting membrane potential, hippocampal PV+ basket cells have also been shown to increase their firing frequency during cholinergic modulation. Trains of action potentials elicit slow afterhyperpolarization potentials (sAHPs) in PV+ basket cells, and these sAHPs are reduced by bath application of muscarine (Cea-del Rio et al., 2010). Furthermore, the AHPs following action potentials and the membrane depolarization following cholinergic activation observed in hippocampal PV+ basket cells have been demonstrated to rely on M1 muscarinic receptor expression (Cea-del Rio et al., 2010), and M1 gene knockout produces complex but selective memory deficits in mice (Yi et al., 2014). Taken together, despite many reports of unresponsive or mild effects of ACh on PV+ cells, evidence points to hippocampal and prefrontal PV+ interneurons exhibiting direct depolarization from muscarinic activation (McBain et al., 1994; Chiang et al., 2010).
Muscarinic activation of PV+ interneurons has been observed to increase the frequency of IPSPs in downstream pyramidal cells, but these effects vary greatly depending on brain region and cortical layer. Postsynaptic observations of IPSPs in hippocampus show that muscarinic modulation increases the frequency of spontaneous IPSPs but decreases the amplitude of evoked IPSCs (Pitler and Alger, 1992; Behrends and ten Bruggencate, 1993). This indicates postsynaptic depolarization of interneurons is combined with presynaptic inhibition of the release of GABA. The recruitment of PV+ cells in hippocampus and prefrontal cortex during brain states exhibiting high levels of ACh has been linked to the recognition of novel objects and spatial aspects of working memory (Yi et al., 2014). In the visual cortex, ACh levels have been associated with the regulation of PV+ cells for altering gain control during attentive behavioral states such as locomotion (Fu et al., 2014), although this regulation is likely indirect through a disinhibitory circuit involving vasoactive intestinal peptide (VIP) positive cells.
Another neurochemically defined group of interneurons are somatostatin (SST) positive interneurons (Rudy et al., 2011). This group represents about 30% of GABAergic neurons in the brain including Martinotti cells in the neocortex, and O-LM and bistratified cells in the hippocampus. Martinotti cells in the neocortex and O-LM cells in the hippocampus selectively innervate the dendrites, rather than the perisomatic region of downstream principal cells, but also synapse onto other non-SST+ inhibitory interneurons. Muscarinic agonists have been shown to produce transitions from AHPs to ADPs in O-LM cells which can result in persistent spiking (McQuiston and Madison, 1999b; Lawrence et al., 2006b). Functionally, these ADPs have been linked to the increase in firing response of stratum oriens interneurons to theta frequency inputs (Lawrence et al., 2006a). O-LM cells have been implicated in controlling the inputs to CA1 pyramidal cells, differentially suppressing extrahippocampal (entorhinal cortical) inputs at the distal apical dendrites of pyramidal cells, whilst facilitating inputs from CA3 to proximal dendrites (Leão et al., 2012).
The serotonin receptor 5HT3a expressing group of interneurons, which includes the population of VIP+ expressing interneurons as well as the separate population of neurogliaform cells, make up for the remaining 30% of GABAergic interneurons (Rudy et al., 2011). VIP+ interneurons express both nACHRs and ionotropic serotonergic receptors, suggesting that these neurons mediate rapid changes due to input from neuromodulators. VIP+ interneurons form a particularly interesting population, because they mainly target other interneurons including the PV+ and SST+ interneurons (Pfeffer et al., 2013) and express both nAChRs as well as ionotropic serotonergic receptors suggesting that these neurons are key for mediating rapid changes from neuromodulators. Indeed, the class of VIP+ interneurons has been suggested to mediate disinhibitory control in multiple areas of the neocortex (Pi et al., 2013; Fu et al., 2014), and this effect has been proposed to be due to fast nicotinic activation of VIP+ neurons (Fu et al., 2014). Muscarinic receptors also act to depolarize VIP+ interneurons (Bell et al., 2015). The cholinergic depolarization of VIP+ interneurons has been proposed to cause indirect disinhibition of principal cells via an increase in inhibition onto downstream PV+ and SST+ cells. In vivo experiments show that VIP+ interneuron activity correlates with behavioral recognition of sensory cues (Kuchibhotla et al., 2017) and probably acts to convey information about reinforcement events and behavioral context. Interestingly, in these experiments, optogenetic activation of a very small minority of VIP+ cells (1%–2%) led to the recruitment of nearly 20% of excitatory cells demonstrating the powerful extent of VIP+ activity on the local network. There is still some debate as to whether this disinhibition acts more globally or more locally, since there is more recent evidence that VIP+ cells project within a narrow vertical column and serve to “open holes in the blanket of inhibition” (Karnani et al., 2016).
CCK+ interneurons cells are also within the grouping of 5HT3a expressing inhibitory interneurons, and form a second functional class of perisomatically targeting basket cells. Interestingly, PV+ basket cells express M1 mAChR mRNA, but entirely lack M3 mRNA, whereas CCK+ basket cells show robust expression of both M1 and M3 mRNA. The additional expression of M3 makes the CCK+ basket cells more sensitive than PV+ basket cells for increases in firing rates upon cholinergic input, as shown in CA1 of mouse hippocampal slices (Cea-del Rio et al., 2010). In line with the activation of perisomatic inhibitory interneurons, optogenetically released ACh resulted in an increase of IPSCs onto CA1 pyramidal neurons (Nagode et al., 2011; Bell et al., 2015). Interestingly, the theta-rhythmic IPSCs could be blocked by endocannabinoid release from pyramidal cells, providing further support that the main source of IPSCs are CB1 expressing CCK+ basket cells (Nagode et al., 2011, 2014). Dendritically projecting Schaffer collateral-associated CCK+ cells, which shape dendritic excitability and synaptic integration, showed similar changes in excitability, except that they showed a biphasic change corresponding to an initial M1-mediated hyperpolarization, followed by an M3-mediated depolarization of their membrane potential (Cea-del Rio et al., 2011).
An important line of research has shown that CB1 expressing CCK+ basket cells strongly demonstrate the phenomenon of depolarization induced suppression of inhibition (DSI), a retrograde signaling mechanism, where endocannabinoids are released from depolarized pyramidal cells act on upstream CB1 receptors on CCK+ basket cells to transiently reduce the frequency of GABA vesicle release. Notably, this phenomenon is highly accentuated when muscarinic receptors on CCK+ basket cells cause larger than baseline IPSP frequencies and postsynaptic depolarization amplitudes in CA1 pyramidal cells (Martin and Alger, 1999). Interestingly, activation of M1/M3 receptors also causes an increase in endocannabinoid production (Fukudome et al., 2004). The synergistic interaction between the endocannabinoid and cholinergic systems at presynaptic CCK+ terminals may be modulating the timing and frequency of GABA release onto CA1 pyramids. In turn, the frequency of phasic inhibition may be important for temporal entrainment of CA1 pyramidal cells with respect to ongoing network rhythms, and more generally coordinating downstream pyramidal spike timing (Pouille and Scanziani, 2001; Daw et al., 2009; Alger et al., 2014).
In summary, the subtype-specific cholinergic modulation of interneuron activity can have strong influences on network dynamics in the hippocampus and other cortical structures. Evidence for this was given recently by an in vivo study from Lovett-Barron et al. (2014), in which aversive stimuli were shown to activate CA1 O-LM interneurons via cholinergic input, leading to inhibition of the distal dendrites of CA1 principal cells, which was necessary for successful fear learning.
Cholinergic Control of Network Dynamics
One striking cellular effect of increasing cholinergic activity is the enhancement of the influence of feedforward afferent input while simultaneously suppressing the influence of excitatory feedback connections (Hasselmo, 2006). This has been discussed extensively in previous reviews (Hasselmo, 2006) but is briefly reviewed here. Physiological studies have shown an enhancement of afferent input caused by nicotinic receptor activation, and the presynaptic inhibition of excitatory feedback connections has been shown to be caused by M4 muscarinic presynaptic inhibition (Dasari and Gulledge, 2011). The selective presynaptic inhibition of recurrent excitation might provide a solution to the problem of proactive interference, which occurs when novel information has to be encoded in the same network capable of retrieving previously stored memories (Hasselmo, 2006). For hippocampal CA1, muscarinic presynaptic inhibition suppresses excitatory projections from CA3 to CA1, but spares inputs from medial EC, allowing a dominant influence of afferent input. Consistent with this model of muscarinic presynaptic inhibition in the hippocampus, local infusion of cholinergic antagonists in hippocampus causes an increase in background spiking activity in unit recordings (Brazhnik et al., 2003). Thereby, ACh regulates the spread of excitatory activity within hippocampal and cortical circuits. These findings match with data from in vitro studies showing inhibition of CA1 pyramidal neurons by ACh-mediated excitation of interneurons (Benardo and Prince, 1982a) and inhibition of DG granule cells due to muscarinic amplification of fast excitation in hilar neurons (Brunner and Misgeld, 1994), and more recent data from single unit recordings in the hippocampus and DG showing decreased spiking activity of pyramidal neurons and DG granule cell coinciding with a higher temporal precision of that spiking activity during optogenetic activation of cholinergic MSDB neurons (Dannenberg et al., 2015; Pabst et al., 2016). As outlined above, microdialysis studies show that cholinergic activity is low during quiet waking or slow wave sleep. This would release the presynaptic inhibition of excitatory feedback, allowing increased generation of sharp wave ripple activity (Hasselmo, 1999; Vandecasteele et al., 2014). The activity of medial septal neurons is indeed decreased during sharp wave ripple events (Dragoi et al., 1999) allowing a strong influence of consolidation based on previously modified recurrent connections. Overall, these data support the view that a primary function of septo-hippocampal ACh is to reduce interference in the learning process by adaptively timing and separating encoding from retrieval and consolidation processes (Figure 1).
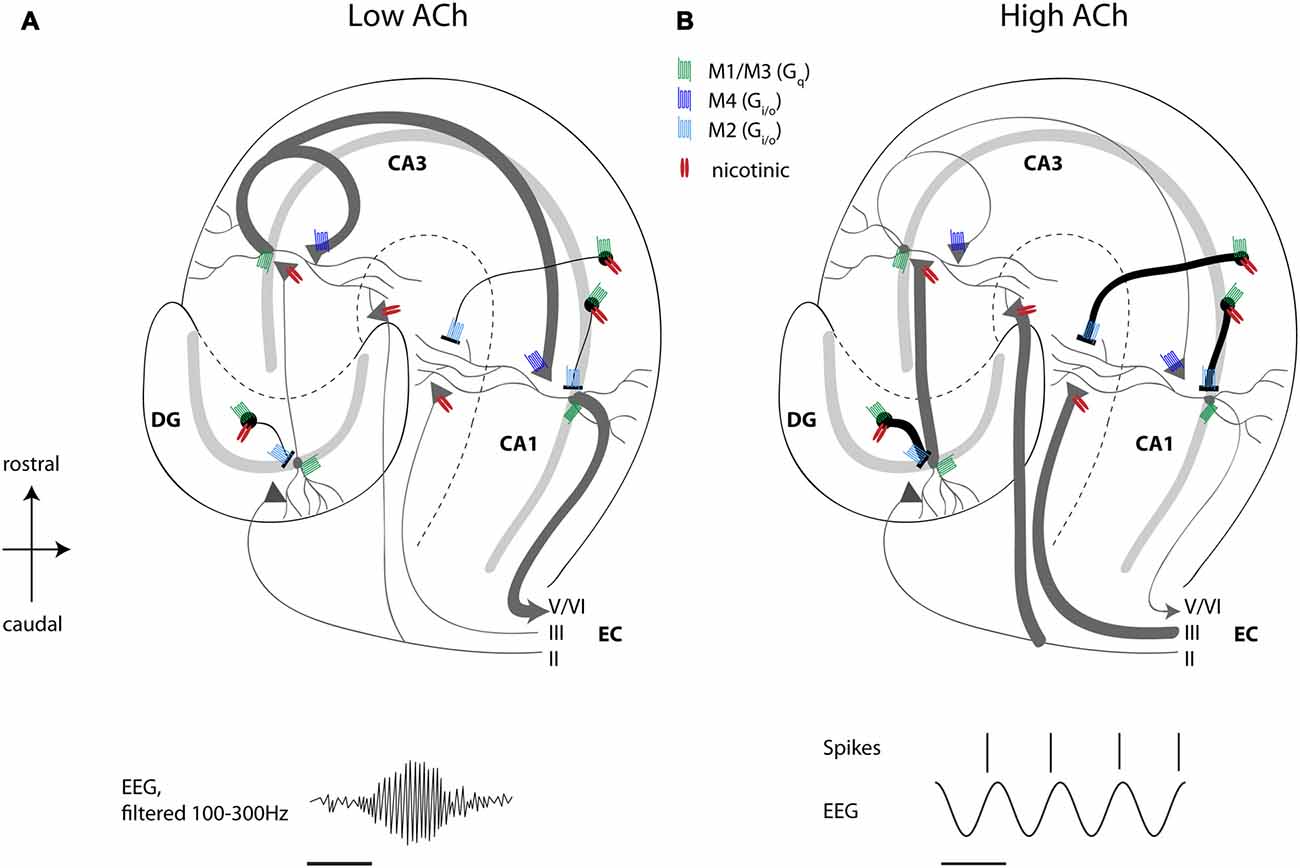
Figure 1. High acetylcholine (ACh) levels enhance encoding and suppress consolidation dynamics in the hippocampus. Schematic drawing of a transverse slice of hippocampus with the main circuit connections and locations of muscarinic and nicotinic receptors shown. (A) When ACh release is low, recurrent excitatory hippocampal activity leads to retrieval and consolidation of previously stored information, which can support the consolidation of memory during sharp wave ripples activity (ripple event schematically depicted in lower left panel). (B) High ACh levels result in nicotinic enhancement of mossy fiber and perforant path inputs, thereby potentiating afferent input synapses in the hippocampus, which favors the encoding of novel information. At the same time, muscarinic depolarization of interneurons and muscarinic presynaptic inhibition of synaptic potentials at recurrent and Schaffer collateral synapses result in suppression of recurrent excitation associated with retrieval of information. Concomitantly, muscarinic and nicotinic excitation of interneurons results in reduced, but temporally more precise spiking activity of pyramidal cells during ongoing theta oscillations (schematically depicted in lower right panel). See text for a more detailed description of receptor distributions and functions. Scale bar for EEG, 125 ms. Principal cells with dendrites schematically depicted in gray, black circles represent interneurons, triangles represent synaptic terminals. ACh, acetylcholine; DG, dentate gyrus; CA, cornu ammonis; EC, entorhinal cortex; EEG, electroencephalogram.
In addition to the modulation by cholinergic presynaptic inhibition of synaptic transmission, the dynamics of theta rhythm might also contribute to the separation of encoding and retrieval (Hasselmo et al., 2002). The trough of the local theta oscillation in stratum pyramidale is associated with hyperpolarization of the soma of hippocampal pyramidal cells (Kamondi et al., 1998), which could prevent postsynaptic spiking that mediates retrieval of memories previously stored in the autoassociative CA3 network (Hasselmo et al., 2002), while allowing novel sensory input from the EC to depolarize dendrites at the same time, inducing synaptic modification. In the light of the experimentally found theta phase-dependent synaptic plasticity (see above), this restricts LTP to the synaptic contacts active during entorhinal inputs at the theta peak. This model of separate phase of encoding and retrieval is supported by experimental data showing that CA1 ensemble firing in rats shifts closer to the theta peak in a novel environment, but scopolamine injections shift the ensemble firing closer to the trough (Douchamps et al., 2013). Further supporting experimental data comes from a study by Siegle and Wilson (2014) who showed that inhibition of CA1 principal cells by optogenetic stimulation of PV+ interneurons could either support encoding or retrieval in an end-to-end T maze task when inhibition was targeted to the putative retrieval or encoding cycle of the theta oscillation, respectively (Siegle and Wilson, 2014). Lower levels of ACh release the presynaptic inhibition of excitatory feedback within cortical structures (Hasselmo, 1999), which allows a stronger influence of hippocampus on neocortex that could underlie the consolidation of previously encoded memories (Dewar et al., 2014; Craig et al., 2016).
Cholinergic Modulation of Location Coding
Numerous studies have shown robust impairments of spatial memory behavior after lesions of the medial septum (Winson, 1978; Givens and Olton, 1990). Similarly, recordings of place cells in rats after lesions of the fimbria-fornix, the main fiber track containing septo-hippocampal projection fibers from cholinergic and other medial septal neurons, have revealed a reduction in the spatial specificity and reliability of firing of place cells, and have shown that place cells are more sensitive to maze rotation (Shapiro et al., 1989). However, lesions of the MSDB or the fimbria-fornix are not specific to the cholinergic subpopulation of medial septum neurons. But the development of an immunotoxin composed of the neurotoxin saporin coupled to an antibody against the nerve growth factor receptor, which is enriched on cholinergic neurons in the MSDB, allowed more specific lesions of the cholinergic subpopulation within the MSDB (Book et al., 1994). The behavioral effects of these selective lesions of cholinergic innervation appeared to be weaker than the effect of medial septum lesions (Parent and Baxter, 2004), but, interestingly, specifically influenced memory for the spatial location of objects in a where-which task in rats (Easton et al., 2011). In mice, saporin lesions of cholinergic MSDB neurons have been shown to cause impairments in the recognition of the spatial location of objects (Cai et al., 2012). Taken together, these data support the view that cholinergic signaling is important for the rapid updating of place cells when visual cues or object locations differ across spatial contexts or when spatial locations are relevant to memory-guided behavior.
Cholinergic Signaling Associated with Spatial Novelty
ACh levels have been shown to increase during learning of a spatial memory task (Stancampiano et al., 1999) and during object exploration (Stanley et al., 2012). Furthermore, focal injection of scopolamine, a muscarinic receptor antagonist, degrades the place fields of hippocampal place cells, which is mostly reversible (Brazhnik et al., 2003). Exposing animals to a novel spatial environment not only increases hippocampal ACh concentrations, but also reduces the frequency of hippocampal theta oscillations, an effect slowly disappearing with increasing familiarity (Jeewajee et al., 2008) providing further hints that ACh modulates hippocampal network activity to better match the environmental demand for processing novel behaviorally relevant information. As outlined above, theta oscillations consist of a slower frequency atropine-sensitive component and a higher frequency atropine-resistant component, also known as type II and type I theta. Thus, the shift to lower frequency theta oscillations during novelty exposure can be explained by higher cholinergic activity, which overall helps integrating sensory experiences into episodic memory. Cholinergic modulation could also be relevant to the effect of novelty on the firing properties of grid cells and place cells. Recordings from rats exploring a novel environment show a larger spacing between the firing fields exhibited by each individual grid cell compared to the baseline spacing observed in a familiar environment (Barry et al., 2012a,b). This expansion of spacing could underlie the shifts in the firing location of place cells (termed remapping) that occurs in novel environments. This expansion of spacing has been proposed to arise from the increase of ACh levels in novel environments (Barry et al., 2012b).
Effects of Acetylcholine on Synaptic Plasticity
Because ACh affects memory and learning, the question arises, how ACh modulates synaptic plasticity, which is generally assumed to be the cellular and molecular correlate of learning. A seminal article by Williams and Johnston (1988) showed muscarinic depression of LTP at the mossy fiber-CA3 synapse. Moreover, the same stimulus can induce either LTP or LTD depending on the precise timing in relation to the ongoing theta oscillation. One study analyzed these effects in slice preparations in which theta rhythm oscillations were induced by cholinergic modulation (Huerta and Lisman, 1995). In this study, a single burst given in vitro at the peak of theta measured in stratum radiatum near to the pyramidal cell layer induced homosynaptic LTP, whereas the same stimulus given at the theta trough induced homosynaptic LTD (Huerta and Lisman, 1995). These results were later confirmed with an experiment using similar burst stimulation in awake behaving rats (Hyman et al., 2003). In addition, single burst stimulation-induced LTP at basal dendrites of CA1 was significantly larger when it was induced during walking than during awake immobility, slow wave sleep, or REM sleep of rats (Leung et al., 2003). On the receptor level, pre- and postsynaptic nAChR and mAChR activity on principal cells and interneurons are involved in the modulation of synaptic plasticity in a complex manner. For instance, nAChR activity could enhance or depress synaptic plasticity with the form of the modulation depending on the location and timing of the nAChR activity relative to the electrical stimulation used for LTP induction in mouse hippocampal slices (Ji et al., 2001). Local puff application of ACh to the apical dendrites was sufficient to boost short term plasticity of Schaffer collateral synapses to LTP. But when the same stimulus was delayed until nAChR-mediated GABAergic inhibition reached the pyramidal neuron, LTP was prevented. In addition to nAChR activity, mAChR activation was shown to modulate the induction and amplitude of LTP at hippocampal Schaffer collateral synapses in slice preparations from rats (Huerta and Lisman, 1995; Buchanan et al., 2010) or mice (Shinoe et al., 2005). Similar results were obtained when cholinergic activity was evoked by tail pinch or electrical stimulation of the medial septum nuclei in anesthetized rats in vivo (Navarrete et al., 2012). Induction of LTP was blocked in this study by systemic atropine application, confirming the contribution of mAChRs. Mechanistically, Buchanan et al. (2010) showed that postsynaptic activation of the muscarinic M1 receptor subtype resulted in the inhibition of SK channels, allowing enhanced NMDA receptor activity and eventually leading to a facilitation of LTP induction.
Cholinergic receptor activation also enhances spike backpropagation, and thereby affects the amplitude and duration of spike train-evoked Ca2+ changes in apical dendrites, thus affecting synaptic integration and plasticity (Tsubokawa and Ross, 1997).
Acetylcholine Effects on Astrocytes
Besides its effects on neurons, ACh also acts on astrocytes. Calcium imaging from acute rat hippocampal slices demonstrated the presence of functional α7-containing nAChRs on astrocytes in the CA1 (Shen and Yakel, 2012) and CA3 region (Grybko et al., 2010). Although the current density is very low, the calcium response upon receptor activation is robust due to the calcium induced calcium release from the endoplasmic reticulum mediated via inositol trisphosphate (IP3) receptor activation (Sharma and Vijayaraghavan, 2001; Grybko et al., 2010). In contrast to these studies, optogenetic stimulation of ACh release from CA1 cholinergic fibers in rat hippocampal slice preparations did not reveal significant nicotinic receptor-mediated effects, but instead mobilized Ca2+ from intracellular stores via muscarinic receptor activation (Araque et al., 2002). In this study, different regions in the recorded astrocytes showed independent stimulus-induced Ca2+ variations, suggesting the existence of subcellular domains in the astrocytic responses evoked by the synaptic cholinergic activity. One caveat of this study, however, is that the potassium channel blocker 4-aminopyridine (4-AP) was added to the slice in order to enhance synaptic release of ACh. Imaging of calcium activity in the barrel cortex of mice in vivo revealed that astrocytes exhibited elevated intracellular calcium levels during the induction of LTP (Takata et al., 2011). Moreover, the induction of LTP could not be induced in IP3 receptor type 2 knockout mice, indicating that calcium release from intracellular stores in astrocytes might be necessary for LTP induction. Likewise, ACh release evoked by tail pinch or electrical stimulation of the medial septum nuclei in anesthetized rats increased Ca2+ in hippocampal astrocytes and induced LTP at Schaffer collateral synapses, an effect dependent on mAChR activation (Navarrete et al., 2012). Further follow-up experiments performed in vitro confirmed the necessity of Ca2+ elevations in astrocytes for LTP induction at the Schaffer collateral synapse in the hippocampus, as previously shown for synapses in the barrel cortex.
Astrocytic activity has also been shown to contribute to γ oscillatory activity and disrupting gliotransmitter release from astrocytes impairs novel object recognition (Lee et al., 2014), two phenomena closely linked to cholinergic activity. Furthermore, genetic deletion of α7 nicotinic receptors causes mild but significant deficits in spatial learning (Levin, 2012). Activation of the α7 nicotinic receptor on astrocytes has also been shown recently to be involved in the regulation of the sleep-wake cycle by ACh (Papouin et al., 2017). Astrocytes in the hippocampus sense the wakefulness-dependent activity of septal cholinergic fibers through the α7-nAChR, whose activation drives D-serine release, which acts as a co-agonist at the NMDA receptor. Thus, astrocytes provide a link between cholinergic activity and NMDA receptor function. This is particularly interesting for the understanding of schizophrenia, a neurological disorder characterized by NMDAR hypofunction.
Cholinergic Dysfunction in Neurological Disorders
Given the outstanding role of ACh for the modulation of cortical and subcortical brain regions, it is not surprising that cholinergic dysfunction is correlated with various neurological and psychiatric disorders including depression, schizophrenia, epilepsy and Alzheimer’s disease (AD). In this review we will focus our discussion on AD and epilepsy, but see Higley and Picciotto (2014) for a recent discussion of cholinergic dysfunction in depression and schizophrenia.
Cholinergic Dysfunction in Alzheimer’s Disease
AD is the most common form of dementia in the elderly with progressive episodic memory deficits and global impairment of cognitive function at later disease states. The definitive diagnosis of AD is still based on post-mortem histophathological examinations of the patients’ brains. AD is characterized anatomically by cortical and white matter atrophy and histologically by the presence of large numbers of extracellular amyloid β (Aβ) plaques, as well as intracellular neuropil threads and neurofibrillary tangles consisting of twisted filaments of hyperphosphorylated tau protein, which also accumulates in the extracellular space after neuronal death (for review, see Serrano-Pozo et al., 2011). The extent of neurofibrillary tangles and neuropil threads found in different brain areas of post-mortem brains correlate with disease states: neurofibrillary changes are first observed in the EC, spreading to the hippocampus, and finally found in all isocortical areas correlating with neuronal damage (Braak and Braak, 1991). Given the central roles of ACh and the hippocampal formation for learning and memory, a cholinergic deficit, particularly within the hippocampal formation, has been suggested to contribute to the memory deficits observed in the elderly and particularly in AD. Supporting this hypothesis, the number of ChAT+ neurons was found to be reduced along the entire length of the basal forebrain in aged vs. young rats (Smith et al., 1993), and the proportion of rhythmically bursting neurons inside the MSDB was lower in aged vs. young rats, especially during immobile arousal states associated with atropine-sensitive theta activity (Apartis et al., 2000). Furthermore, cholinergic synaptic transmission in the hippocampus declines with age (Taylor and Griffith, 1993). In post-mortem tissue of AD patients, a substantial decrease of AChE and ChAT enzyme activity in many cortical areas, including the hippocampus, has been observed (Davies, 1979), indicating loss of cholinergic function at the beginning of AD. Radioligand binding assays found binding of [3H]-labeled nicotine to the DG granule cell layer, the presubiculum and the parahippocampal gyrus 30% reduced in post-mortem tissue of AD patients relative to age-matched elderly control subjects (Perry et al., 1995), suggesting a decrease of nAChR expression in these areas. Moreover, Aβ1–42 peptide was found to bind to nicotinic receptors of both the α7 and the non-α7 subtype, with higher affinity to the α7 subtype (Wang et al., 2000a,b). On a functional level, this binding has been demonstrated to inhibit nicotinic currents in rat hippocampal slices (Pettit et al., 2001) and research focused recently on the role of nicotinic AChRs, especially of the α7 subtype, for possible treatment options in AD (Vallés et al., 2014). At the moment AChE inhibitors are the most used drugs for treatment of mild to moderate AD, although they only show small benefits at the early stages of AD and do not prevent further progression of the disease (Kaduszkiewicz et al., 2005).
Nevertheless, the cholinergic deficit observed in AD can have substantial effects on the structure and thereby function of microcircuits with important consequences for cognitive processes and behavior. A recent study by Schmid et al. (2016) shows that structural plasticity of dendritic spines on O-LM interneurons is impaired in transgenic APP/PS-1 mice. This mouse line is commonly used as a model for AD. It carries mutations in the amyloid precursor protein and the presenilin-1 protein of the γ-secretase complex, a combination of mutations which lead to elevated β-amyloid production associated with cognitive impairments and memory deficits while ageing. The impairment of structural plasticity found by Schmid et al. (2016) in this mouse model was due to a loss of cholinergic input onto O-LM interneurons. Moreover, septal cholinergic input onto O-LM interneurons was shown to be necessary for fear conditioning induced spine gain on O-LM interneurons and application of cevemeline, an M1 AChR agonist, significantly improved memory deficits in the APP/PS1 mice. Thus, decreased cholinergic drive onto O-LM interneurons contributes to rewiring and memory deficits under AD-like conditions.
Previous models suggested that lower levels of ACh resulting in reduced presynaptic inhibition by muscarinic receptors could lead to excessive synaptic modification that could contribute to the progression of AD (Hasselmo, 1994), which is consistent with data showing hyperactivity in the hippocampal formation in presymptomatic AD (Quiroz et al., 2010). This framework supports the use of M4 muscarinic agonists to boost presynaptic inhibition and potentially reduce the hyperactivation in AD (Newman et al., 2012). Understanding the physiological function of the septo-hippocampal cholinergic system thus remains an important step in basic research. This applies not only for AD, but also for epilepsy.
Cholinergic Dysfunction in Epilepsy
Epilepsy is not a singular disease entity, but a group of neurological disorders characterized clinically by an enduring predisposition to generate epileptic seizures (Fisher et al., 2005). An epileptic seizure is defined as a transient occurrence of signs and/or symptoms due to abnormal excessive or synchronous neuronal activity in the brain (Fisher et al., 2005). One widely used animal model of epilepsy in basic research is the pilocarpine-induced epilepsy model. In vivo application of the mAChR agonist pilocarpine (together with methyl-scopolamine to block the action of pilocarpine on AChRs in the periphery) readily induces epileptic seizures and may lead to status epilepticus, resulting in spontaneous recurrent seizures following a latent period of epileptogenesis (Friedman et al., 2007). However, under physiological conditions, septal cholinergic neurons appear to suppress seizure activity, as indicated by a study from Ferencz et al. (2001), in which the authors showed that cholinergic septo-hippocampal deafferentiation facilitated hippocampal kindling in rats. Interestingly, chronic epileptic rats show a neuronal loss in the medial and lateral septum, which is mainly due to the loss of GABAergic neurons (80%–97%), suggesting that the processing of information in the septo-hippocampal networks might be altered (Garrido Sanabria et al., 2006). In line with the hypothesized role of GABAergic MSDB neurons for pacing hippocampal theta rhythm, early deficits are observed in spatial memory and theta rhythmic activity in such chronic epileptic rats (Chauvière et al., 2009). Conversely, epileptic seizures are less frequent during behavioral states associated with hippocampal theta rhythmic activity, e.g., active wakefulness or REM sleep, and microinjections of the muscarinic agonist carbachol into the MSDB not only elicited theta rhythmic activity, but also stopped pentylenetetrazol induced facial-forelimb seizures in rats (Miller et al., 1994). Further highlighting the role of a theta rhythmic functional network state inhibiting seizure production, electrical stimulation of the MSDB at the theta frequency range had similar effects as the carbachol microinjection. Degeneration of septal neurons, as observed in AD, might also contribute to epileptic seizures, which have a very high prevalence of 10%–22% in AD patients (Mendez and Lim, 2003).
Conclusion
As reviewed here, physiological data demonstrates robust neuromodulatory effects of the activation of muscarinic and nicotinic receptors within cortical circuits including the hippocampal formation. These modulatory effects appear important to the encoding of new information, based on changes in network circuit dynamics as reviewed in previous articles (Hasselmo, 2006). The heterogeneity of these effects on different subtypes of neurons will require future computational modeling to develop more detailed computational hypotheses of the function of these modulatory effects. These can be tested in experimental studies exploring the functional role of the strong neuromodulatory effects observed in physiological studies.
Author Contributions
All authors conceived and wrote the review.
Funding
This research was supported by a grant from the National Institutes of Health (Grant No. MH060013, MH061492) and Office of Naval Research (Grant No. N00014-16-1-2832), and Deutsche Forschungsgemeinschaft (DFG; Project No. 322014644).
Conflict of Interest Statement
The authors declare that the research was conducted in the absence of any commercial or financial relationships that could be construed as a potential conflict of interest.
Acknowledgments
The article includes contents from the dissertation by Dannenberg (2015).
References
Acquas, E., Wilson, C., and Fibiger, H. C. (1996). Conditioned and unconditioned stimuli increase frontal cortical and hippocampal acetylcholine release: effects of novelty, habituation, and fear. J. Neurosci. 16, 3089–3096.
Alger, B. E., Nagode, D. A., and Tang, A.-H. (2014). Muscarinic cholinergic receptors modulate inhibitory synaptic rhythms in hippocampus and neocortex. Front. Synaptic Neurosci. 6:18. doi: 10.3389/fnsyn.2014.00018
Alkondon, M., and Albuquerque, E. X. (2004). The nicotinic acetylcholine receptor subtypes and their function in the hippocampus and cerebral cortex. Prog. Brain Res. 145, 109–120. doi: 10.1016/s0079-6123(03)45007-3
Alkondon, M., Pereira, E. F. R., Eisenberg, H. M., and Albuquerque, E. X. (1999). Choline and selective antagonists identify two subtypes of nicotinic acetylcholine receptors that modulate GABA release from CA1 interneurons in rat hippocampal slices. J. Neurosci. 19, 2693–2705.
Aloisi, A. M., Casamenti, F., Scali, C., Pepeu, G., and Carli, G. (1997). Effects of novelty, pain and stress on hippocampal extracellular acetylcholine levels in male rats. Brain Res. 748, 219–226. doi: 10.1016/s0006-8993(96)01304-2
Apartis, E., Poindessous-Jazat, F., Epelbaum, J., and Bassant, M. H. (2000). Age-related changes in rhythmically bursting activity in the medial septum of rats. Brain Res. 876, 37–47. doi: 10.1016/s0006-8993(00)02571-3
Araque, A., Martín, E. D., Perea, G., Arellano, J. I., and Buño, W. (2002). Synaptically released acetylcholine evokes Ca2+ elevations in astrocytes in hippocampal slices. J. Neurosci. 22, 2443–2450.
Atri, A., Sherman, S., Norman, K. A., Kirchhoff, B. A., Nicolas, M. M., Greicius, M. D., et al. (2004). Blockade of central cholinergic receptors impairs new learning and increases proactive interference in a word paired-associate memory task. Behav. Neurosci. 118, 223–236. doi: 10.1037/0735-7044.118.1.223
Barry, C., Ginzberg, L. L., O’Keefe, J., and Burgess, N. (2012a). Grid cell firing patterns signal environmental novelty by expansion. Proc. Natl. Acad. Sci. U S A 109, 17687–17692. doi: 10.1073/pnas.1209918109
Barry, C., Heys, J. G., and Hasselmo, M. E. (2012b). Possible role of acetylcholine in regulating spatial novelty effects on θ rhythm and grid cells. Front. Neural Circuits 6:5. doi: 10.3389/fncir.2012.00005
Behrends, J. C., and ten Bruggencate, G. (1993). Cholinergic modulation of synaptic inhibition in the guinea pig hippocampus in vitro: excitation of GABAergic interneurons and inhibition of GABA-release. J. Neurophysiol. 69, 626–629.
Bell, L. A., Bell, K. A., and McQuiston, A. R. (2013). Synaptic muscarinic response types in hippocampal CA1 interneurons depend on different levels of presynaptic activity and different muscarinic receptor subtypes. Neuropharmacology 73, 160–173. doi: 10.1016/j.neuropharm.2013.05.026
Bell, L. A., Bell, K. A., and McQuiston, A. R. (2015). Activation of muscarinic receptors by ACh release in hippocampal CA1 depolarizes VIP but has varying effects on parvalbumin-expressing basket cells. J. Physiol. 593, 197–215. doi: 10.1113/jphysiol.2014.277814
Bell, K. A., Shim, H., Chen, C.-K., and McQuiston, A. R. (2011). Nicotinic excitatory postsynaptic potentials in hippocampal CA1 interneurons are predominantly mediated by nicotinic receptors that contain α4 and β2 subunits. Neuropharmacology 61, 1379–1388. doi: 10.1016/j.neuropharm.2011.08.024
Belluscio, M. A., Mizuseki, K., Schmidt, R., Kempter, R., and Buzsáki, G. (2012). Cross-frequency phase-phase coupling between θ and γ oscillations in the hippocampus. J. Neurosci. 32, 423–435. doi: 10.1523/JNEUROSCI.4122-11.2012
Benardo, L. S., and Prince, D. A. (1982a). Cholinergic excitation of mammalian hippocampal pyramidal cells. Brain Res. 249, 315–331. doi: 10.1016/0006-8993(82)90066-x
Benardo, L. S., and Prince, D. A. (1982b). Cholinergic pharmacology of mammalian hippocampal pyramidal cells. Neuroscience 7, 1703–1712. doi: 10.1016/0306-4522(82)90028-8
Benardo, L. S., and Prince, D. A. (1982c). Ionic mechanisms of cholinergic excitation in mammalian hippocampal pyramidal cells. Brain Res. 249, 333–344. doi: 10.1016/0006-8993(82)90067-1
Benchenane, K., Peyrache, A., Khamassi, M., Tierney, P. L., Gioanni, Y., Battaglia, F. P., et al. (2010). Coherent θ oscillations and reorganization of spike timing in the hippocampal- prefrontal network upon learning. Neuron 66, 921–936. doi: 10.1016/j.neuron.2010.05.013
Bianchi, L., Ballini, C., Colivicchi, M. A., Della Corte, L., Giovannini, M. G., and Pepeu, G. (2003). Investigation on acetylcholine, aspartate, glutamate, and GABA extracellular levels from ventral hippocampus during repeated exploratory activity in the rat. Neurochem. Res. 28, 565–573. doi: 10.1023/A:1022881625378
Bloem, B., Poorthuis, R. B., and Mansvelder, H. D. (2014). Cholinergic modulation of the medial prefrontal cortex: the role of nicotinic receptors in attention and regulation of neuronal activity. Front. Neural Circuits 8:17. doi: 10.3389/fncir.2014.00017
Blokland, A., Honig, W., and Raaijmakers, W. G. (1992). Effects of intra-hippocampal scopolamine injections in a repeated spatial acquisition task in the rat. Psychopharmacology 109, 373–376. doi: 10.1007/bf02245886
Bohbot, V. D., Copara, M. S., Gotman, J., and Ekstrom, A. D. (2017). Low-frequency θ oscillations in the human hippocampus during real-world and virtual navigation. Nat. Commun. 8:14415. doi: 10.1038/ncomms14415
Book, A. A., Wiley, R. G., and Schweitzer, J. B. (1994). 192 IgG-saporin: I. Specific lethality for cholinergic neurons in the basal forebrain of the rat. J. Neuropathol. Exp. Neurol. 53, 95–102. doi: 10.1097/00005072-199401000-00012
Braak, H., and Braak, E. (1991). Neuropathological stageing of Alzheimer-related changes. Acta Neuropathol. 82, 239–259. doi: 10.1007/bf00308809
Bragin, A., Jandó, G., Nádasdy, Z., Hetke, J., Wise, K., and Buzsáki, G. (1995). γ (40–100 Hz) oscillation in the hippocampus of the behaving rat. J. Neurosci. 15, 47–60.
Brazhnik, E. S., Muller, R. U., and Fox, S. E. (2003). Muscarinic blockade slows and degrades the location-specific firing of hippocampal pyramidal cells. J. Neurosci. 23, 611–621.
Brown, D. A., and Adams, P. R. (1980). Muscarinic suppression of a novel voltage-sensitive K+ current in a vertebrate neurone. Nature 283, 673–676. doi: 10.1038/283673a0
Brunner, H., and Misgeld, U. (1994). Muscarinic amplification of fast excitation in hilar neurones and inhibition in granule cells in the guinea-pig hippocampus. J. Physiol. 480, 513–526. doi: 10.1113/jphysiol.1994.sp020380
Buchanan, K. A., Petrovic, M. M., Chamberlain, S. E. L., Marrion, N. V., and Mellor, J. R. (2010). Facilitation of long-term potentiation by muscarinic M1 receptors is mediated by inhibition of SK channels. Neuron 68, 948–963. doi: 10.1016/j.neuron.2010.11.018
Burmeister, J. J., Pomerleau, F., Huettl, P., Gash, C. R., Werner, C. E., Bruno, J. P., et al. (2008). Ceramic-based multisite microelectrode arrays for simultaneous measures of choline and acetylcholine in CNS. Biosens. Bioelectron. 23, 1382–1389. doi: 10.1016/j.bios.2007.12.013
Cai, L., Gibbs, R. B., and Johnson, D. A. (2012). Recognition of novel objects and their location in rats with selective cholinergic lesion of the medial septum. Neurosci. Lett. 506, 261–265. doi: 10.1016/j.neulet.2011.11.019
Cea-del Rio, C. A., Lawrence, J. J., Erdelyi, F., Szabo, G., and McBain, C. J. (2011). Cholinergic modulation amplifies the intrinsic oscillatory properties of CA1 hippocampal cholecystokinin-positive interneurons. J. Physiol. 589, 609–627. doi: 10.1113/jphysiol.2010.199422
Cea-del Rio, C. A., Lawrence, J. J., Tricoire, L., Erdelyi, F., Szabo, G., and McBain, C. J. (2010). M3 muscarinic acetylcholine receptor expression confers differential cholinergic modulation to neurochemically distinct hippocampal basket cell subtypes. J. Neurosci. 30, 6011–6024. doi: 10.1523/JNEUROSCI.5040-09.2010
Ceccarelli, I., Casamenti, F., Massafra, C., Pepeu, G., Scali, C., and Aloisi, A. M. (1999). Effects of novelty and pain on behavior and hippocampal extracellular ACh levels in male and female rats. Brain Res. 815, 169–176. doi: 10.1016/s0006-8993(98)01171-8
Changeux, J. P., Bertrand, D., Corringer, P. J., Dehaene, S., Edelstein, S., Léna, C., et al. (1998). Brain nicotinic receptors: structure and regulation, role in learning and reinforcement. Brain Res. Rev. 26, 198–216. doi: 10.1016/S0165-0173(97)00040-4
Chapman, C. A., and Lacaille, J. C. (1999). Cholinergic induction of θ-frequency oscillations in hippocampal inhibitory interneurons and pacing of pyramidal cell firing. J. Neurosci. 19, 8637–8645.
Chauvière, L., Rafrafi, N., Thinus-Blanc, C., Bartolomei, F., Esclapez, M., and Bernard, C. (2009). Early deficits in spatial memory and θ rhythm in experimental temporal lobe epilepsy. J. Neurosci. 29, 5402–5410. doi: 10.1523/JNEUROSCI.4699-08.2009
Cheng, Q., and Yakel, J. L. (2014). Presynaptic α7 nicotinic acetylcholine receptors enhance hippocampal mossy fiber glutamatergic transmission via PKA activation. J. Neurosci. 34, 124–133. doi: 10.1523/JNEUROSCI.2973-13.2014
Chiang, P. H., Yeh, W. C., Lee, C. T., Weng, J. Y., Huang, Y. Y., and Lien, C. C. (2010). M1-like muscarinic acetylcholine receptors regulate fast-spiking interneuron excitability in rat dentate gyrus. Neuroscience 169, 39–51. doi: 10.1016/j.neuroscience.2010.04.051
Chrobak, J. J., Stackman, R. W., and Walsh, T. J. (1989). Intraseptal administration of muscimol produces dose-dependent memory impairments in the rat. Behav. Neural Biol. 52, 357–369. doi: 10.1016/s0163-1047(89)90472-x
Cobb, S. R., Buhl, E. H., Halasy, K., Paulsen, O., and Somogyi, P. (1995). Synchronization of neuronal activity in hippocampus by individual GABAergic interneurons. Nature 378, 75–78. doi: 10.1038/378075a0
Cole, A. E., and Nicoll, R. A. (1984). Characterization of a slow cholinergic postsynaptic potential recorded in vitro from rat hippocampal pyramidal cells. J. Physiol. 352, 173–188. doi: 10.1113/jphysiol.1984.sp015285
Colgin, L. L., Denninger, T., Fyhn, M., Hafting, T., Bonnevie, T., Jensen, O., et al. (2009). Frequency of γ oscillations routes flow of information in the hippocampus. Nature 462, 353–357. doi: 10.1038/nature08573
Craig, M., Dewar, M., Harris, M. A., Della Sala, S., and Wolbers, T. (2016). Wakeful rest promotes the integration of spatial memories into accurate cognitive maps. Hippocampus 26, 185–193. doi: 10.1002/hipo.22502
Dannenberg, H. (2015). Direct and Indirect Cholinergic Septo-Hippocampal Pathways Cooperate to Structure Spiking Activity in the Hippocampus [Dissertation]. Bonn: Universitäts-und Landesbibliothek Bonn.
Dannenberg, H., Pabst, M., Braganza, O., Schoch, S., Niediek, J., Bayraktar, M., et al. (2015). Synergy of direct and indirect cholinergic septo-hippocampal pathways coordinates firing in hippocampal networks. J. Neurosci. 35, 8394–8410. doi: 10.1523/JNEUROSCI.4460-14.2015
Dasari, S., and Gulledge, A. T. (2011). M1 and M4 receptors modulate hippocampal pyramidal neurons. J. Neurophysiol. 105, 779–792. doi: 10.1152/jn.00686.2010
Davies, P. (1979). Neurotransmitter-related enzymes in senile dementia of the Alzheimer type. Brain Res. 171, 319–327. doi: 10.1016/0006-8993(79)90336-6
Daw, M. I., Tricoire, L., Erdelyi, F., Szabo, G., and McBain, C. J. (2009). Asynchronous transmitter release from CCK-containing inhibitory interneurons is widespread and target-cell independent. J. Neurosci. 29, 11112–11122. doi: 10.1523/JNEUROSCI.5760-08.2009
Descarries, L., Gisiger, V., and Steriade, M. (1997). Diffuse transmission by acetylcholine in the CNS. Prog. Neurobiol. 53, 603–625. doi: 10.1016/s0301-0082(97)00050-6
Dewar, M., Alber, J., Cowan, N., and Della Sala, S. (2014). Boosting long-term memory via wakeful rest: intentional rehearsal is not necessary, consolidation is sufficient. PLoS One 9:e109542. doi: 10.1371/journal.pone.0109542
Disney, A. A., Aoki, C., and Hawken, M. J. (2007). Gain modulation by nicotine in macaque v1. Neuron 56, 701–713. doi: 10.1016/j.neuron.2007.09.034
Douchamps, V., Jeewajee, A., Blundell, P., Burgess, N., and Lever, C. (2013). Evidence for encoding versus retrieval scheduling in the hippocampus by θ phase and acetylcholine. J. Neurosci. 33, 8689–8704. doi: 10.1523/JNEUROSCI.4483-12.2013
Dragoi, G., Carpi, D., Recce, M., Csicsvari, J., and Buzsaki, G. (1999). Interactions between hippocampus and medial septum during sharp waves and θ oscillation in the behaving rat. J. Neurosci. 19, 6191–6199.
Easton, A., Fitchett, A. E., Eacott, M. J., and Baxter, M. G. (2011). Medial septal cholinergic neurons are necessary for context-place memory but not episodic-like memory. Hippocampus 21, 1021–1027. doi: 10.1002/hipo.20814
Egorov, A. V., Hamam, B. N., Fransén, E., Hasselmo, M. E., and Alonso, A. A. (2002). Graded persistent activity in entorhinal cortex neurons. Nature 420, 173–178. doi: 10.1038/nature01171
Ekstrom, A. D., Caplan, J. B., Ho, E., Shattuck, K., Fried, I., and Kahana, M. J. (2005). Human hippocampal θ activity during virtual navigation. Hippocampus 15, 881–889. doi: 10.1002/hipo.20109
Ferencz, I., Leanza, G., Nanobashvili, A., Kokaia, Z., Kokaia, M., and Lindvall, O. (2001). Septal cholinergic neurons suppress seizure development in hippocampal kindling in rats: comparison with noradrenergic neurons. Neuroscience 102, 819–832. doi: 10.1016/s0306-4522(00)00499-1
Fernández-Ruiz, A., Oliva, A., Nagy, G. A., Maurer, A. P., Berényi, A., and Buzsáki, G. (2017). Entorhinal-CA3 dual-input control of spike timing in the hippocampus by θ-γ coupling. Neuron 93, 1213.e5–1226.e5. doi: 10.1016/j.neuron.2017.02.017
Fisher, R. S., van Emde Boas, W., Blume, W., Elger, C., Genton, P., Lee, P., et al. (2005). Epileptic seizures and epilepsy: definitions proposed by the International League Against Epilepsy (ILAE) and the International Bureau for Epilepsy (IBE). Epilepsia 46, 470–472. doi: 10.1111/j.0013-9580.2005.66104.x
Flynn, D. D., Ferrari-DiLeo, G., Mash, D. C., and Levey, A. I. (1995). Differential regulation of molecular subtypes of muscarinic receptors in Alzheimer’s disease. J. Neurochem. 64, 1888–1891. doi: 10.1046/j.1471-4159.1995.64041888.x
Frazier, C. J., Rollins, Y. D., Breese, C. R., Leonard, S., Freedman, R., and Dunwiddie, T. V. (1998). Acetylcholine activates an α-bungarotoxin-sensitive nicotinic current in rat hippocampal interneurons, but not pyramidal cells. J. Neurosci. 18, 1187–1195.
Frazier, C. J., Strowbridge, B. W., and Papke, R. L. (2003). Nicotinic receptors on local circuit neurons in dentate gyrus: a potential role in regulation of granule cell excitability. J. Neurophysiol. 89, 3018–3028. doi: 10.1152/jn.01036.2002
Freund, T. F., and Buzsáki, G. (1996). Interneurons of the hippocampus. Hippocampus 6, 347–470. doi: 10.1002/(sici)1098-1063(1996)6:4<347::aid-hipo1>3.0.co;2-i
Friedman, A., Behrens, C. J., and Heinemann, U. (2007). Cholinergic dysfunction in temporal lobe epilepsy. Epilepsia 48, 126–130. doi: 10.1111/j.1528-1167.2007.01300.x
Fu, Y., Tucciarone, J. M., Espinosa, J. S., Sheng, N., Darcy, D. P., Nicoll, R. A., et al. (2014). A cortical circuit for gain control by behavioral state. Cell 156, 1139–1152. doi: 10.1016/j.cell.2014.01.050
Fukudome, Y., Ohno-Shosaku, T., Matsui, M., Omori, Y., Fukaya, M., Tsubokawa, H., et al. (2004). Two distinct classes of muscarinic action on hippocampal inhibitory synapses: M2-mediated direct suppression and M1/M3-mediated indirect suppression through endocannabinoid signalling. Eur. J. Neurosci. 19, 2682–2692. doi: 10.1111/j.0953-816x.2004.03384.x
Garrido Sanabria, E. R., Castañeda, M. T., Banuelos, C., Perez-Cordova, M. G., Hernandez, S., and Colom, L. V. (2006). Septal GABAergic neurons are selectively vulnerable to pilocarpine-induced status epilepticus and chronic spontaneous seizures. Neuroscience 142, 871–883. doi: 10.1016/j.neuroscience.2006.06.057
Ghoneim, M. M., and Mewaldt, S. P. (1977). Studies on human memory: the interactions of diazepam, scopolamine, and physostigmine. Psychopharmacology 52, 1–6. doi: 10.1007/bf00426592
Gil, Z., Conners, B. W., and Amitai, Y. (1997). Differential regulation of neocortical synapses by neuromodulators and activity. Neuron 19, 679–686. doi: 10.1016/s0896-6273(00)80380-3
Giocomo, L. M., and Hasselmo, M. E. (2005). Nicotinic modulation of glutamatergic synaptic transmission in region CA3 of the hippocampus. Eur. J. Neurosci. 22, 1349–1356. doi: 10.1111/j.1460-9568.2005.04316.x
Giovannini, M. G., Rakovska, A., Benton, R. S., Pazzagli, M., Bianchi, L., and Pepeu, G. (2001). Effects of novelty and habituation on acetylcholine, GABA, and glutamate release from the frontal cortex and hippocampus of freely moving rats. Neuroscience 106, 43–53. doi: 10.1016/s0306-4522(01)00266-4
Givens, B. S., and Olton, D. S. (1990). Cholinergic and GABAergic modulation of the medial septal area: effect on working memory. Behav. Neurosci. 104, 849–855. doi: 10.1037/0735-7044.104.6.849
Gray, R., Rajan, A. S., Radcliffe, K. A., Yakehiro, M., and Dani, J. A. (1996). Hippocampal synaptic transmission enhanced by low concentrations of nicotine. Nature 383, 713–716. doi: 10.1038/383713a0
Green, J. D., and Arduini, A. A. (1954). Hippocampal electrical activity in arousal. J. Neurophysiol. 17, 533–557.
Green, A., Ellis, K. A., Ellis, J., Bartholomeusz, C. F., Ilic, S., Croft, R. J., et al. (2005). Muscarinic and nicotinic receptor modulation of object and spatial n-back working memory in humans. Pharmacol. Biochem. Behav. 81, 575–584. doi: 10.1016/j.pbb.2005.04.010
Gritton, H. J., Howe, W. M., Mallory, C. S., Hetrick, V. L., Berke, J. D., and Sarter, M. (2016). Cortical cholinergic signaling controls the detection of cues. Proc. Natl. Acad. Sci. U S A 113, E1089–E1097. doi: 10.1073/pnas.1516134113
Grybko, M., Sharma, G., and Vijayaraghavan, S. (2010). Functional distribution of nicotinic receptors in CA3 region of the hippocampus. J. Mol. Neurosci. 40, 114–120. doi: 10.1007/s12031-009-9266-8
Gulledge, A. T., and Kawaguchi, Y. (2007). Phasic cholinergic signaling in the hippocampus: functional homology with the neocortex? Hippocampus 17, 327–332. doi: 10.1002/hipo.20279
Gulledge, A. T., Park, S. B., Kawaguchi, Y., and Stuart, G. J. (2007). Heterogeneity of phasic cholinergic signaling in neocortical neurons. J. Neurophysiol. 97, 2215–2229. doi: 10.1152/jn.00493.2006
Haam, J., and Yakel, J. L. (2017). Cholinergic modulation of the hippocampal region and memory function. J. Neurochem. 142, 111–121. doi: 10.1111/jnc.14052
Hájos, N., Papp, E. C., Acsády, L., Levey, A. I., and Freund, T. F. (1998). Distinct interneuron types express m2 muscarinic receptor immunoreactivity on their dendrites or axon terminals in the hippocampus. Neuroscience 82, 355–376. doi: 10.1016/s0306-4522(97)00300-x
Hangya, B., Borhegyi, Z., Szilágyi, N., Freund, T. F., and Varga, V. (2009). GABAergic neurons of the medial septum lead the hippocampal network during θ activity. J. Neurosci. 29, 8094–8102. doi: 10.1523/JNEUROSCI.5665-08.2009
Hasselmo, M. E. (1994). Runaway synaptic modification in models of cortex: implications for Alzheimer’s disease. Neural Netw. 7, 13–40. doi: 10.1016/0893-6080(94)90053-1
Hasselmo, M. E. (1999). Neuromodulation: acetylcholine and memory consolidation. Trends Cogn. Sci. 3, 351–359. doi: 10.1016/s1364-6613(99)01365-0
Hasselmo, M. E. (2006). The role of acetylcholine in learning and memory. Curr. Opin. Neurobiol. 16, 710–715. doi: 10.1016/j.conb.2006.09.002
Hasselmo, M. E., Bodelón, C., and Wyble, B. P. (2002). A proposed function for hippocampal θ rhythm: separate phases of encoding and retrieval enhance reversal of prior learning. Neural Comput. 14, 793–817. doi: 10.1162/089976602317318965
Hasselmo, M. E., and Schnell, E. (1994). Laminar selectivity of the cholinergic suppression of synaptic transmission in rat hippocampal region CA1: computational modeling and brain slice physiology. J. Neurosci. 14, 3898–3914.
Hasselmo, M. E., Schnell, E., and Barkai, E. (1995). Dynamics of learning and recall at excitatory recurrent synapses and cholinergic modulation in rat hippocampal region CA3. J. Neurosci. 15, 5249–5262.
Herreras, O., Solís, J. M., Herranz, A. S., Martín del Río, R., and Lerma, J. (1988). Sensory modulation of hippocampal transmission. II. Evidence for a cholinergic locus of inhibition in the Schaffer-CA1 synapse. Brain Res. 461, 303–313. doi: 10.1016/0006-8993(88)90260-0
Higley, M. J., and Picciotto, M. R. (2014). Neuromodulation by acetylcholine: examples from schizophrenia and depression. Curr. Opin. Neurobiol. 29, 88–95. doi: 10.1016/j.conb.2014.06.004
Hounsgaard, J. (1978). Presynaptic inhibitory action of acetylcholine in area CA1 of the hippocampus. Exp. Neurol. 62, 787–797. doi: 10.1016/0014-4886(78)90284-4
Huerta, P. T., and Lisman, J. E. (1995). Bidirectional synaptic plasticity induced by a single burst during cholinergic θ oscillation in CA1 in vitro. Neuron 15, 1053–1063. doi: 10.1016/0896-6273(95)90094-2
Hyman, J. M., Wyble, B. P., Goyal, V., Rossi, C. A., and Hasselmo, M. E. (2003). Stimulation in hippocampal region CA1 in behaving rats yields long-term potentiation when delivered to the peak of θ and long-term depression when delivered to the trough. J. Neurosci. 23, 11725–11731.
Hyman, J. M., Zilli, E. A., Paley, A. M., and Hasselmo, M. E. (2005). Medial prefrontal cortex cells show dynamic modulation with the hippocampal θ rhythm dependent on behavior. Hippocampus 15, 739–749. doi: 10.1002/hipo.20106
Jeewajee, A., Lever, C., Burton, S., O’Keefe, J., and Burgess, N. (2008). Environmental novelty is signaled by reduction of the hippocampal θ frequency. Hippocampus 18, 340–348. doi: 10.1002/hipo.20394
Ji, D., Lape, R., and Dani, J. A. (2001). Timing and location of nicotinic activity enhances or depresses hippocampal synaptic plasticity. Neuron 31, 131–141. doi: 10.1016/s0896-6273(01)00332-4
Jochems, A., and Yoshida, M. (2013). Persistent firing supported by an intrinsic cellular mechanism in hippocampal CA3 pyramidal cells. Eur. J. Neurosci. 38, 2250–2259. doi: 10.1111/ejn.12236
Jones, M. W., and Wilson, M. A. (2005). θ rhythms coordinate hippocampal-prefrontal interactions in a spatial memory task. PLoS Biol. 3:e402. doi: 10.1371/journal.pbio.0030402
Jones, S., and Yakel, J. L. (1997). Functional nicotinic ACh receptors on interneurones in the rat hippocampus. J. Physiol. 504, 603–610. doi: 10.1111/j.1469-7793.1997.603bd.x
Kaduszkiewicz, H., Zimmermann, T., Beck-Bornholdt, H.-P., and van den Bussche, H. (2005). Cholinesterase inhibitors for patients with Alzheimer’s disease: systematic review of randomised clinical trials. BMJ 331, 321–327. doi: 10.1136/bmj.331.7512.321
Kahana, M. J., Sekuler, R., Caplan, J. B., Kirschen, M., and Madsen, J. R. (1999). Human θ oscillations exhibit task dependence during virtual maze navigation. Nature 399, 781–784. doi: 10.1038/21645
Kalappa, B. I., Gusev, A. G., and Uteshev, V. V. (2010). Activation of functional α7-containing nAChRs in hippocampal CA1 pyramidal neurons by physiological levels of choline in the presence of PNU-120596. PLoS One 5:e13964. doi: 10.1371/journal.pone.0013964
Kamondi, A., Acsády, L., Wang, X. J., and Buzsáki, G. (1998). θ oscillations in somata and dendrites of hippocampal pyramidal cells in vivo: activity-dependent phase-precession of action potentials. Hippocampus 8, 244–261. doi: 10.1002/(sici)1098-1063(1998)8:3<244::aid-hipo7>3.0.co;2-j
Karnani, M. M., Jackson, J., Ayzenshtat, I., Hamzehei Sichani, A., Manoocheri, K., Kim, S., et al. (2016). Opening holes in the blanket of inhibition: localized lateral disinhibition by VIP interneurons. J. Neurosci. 36, 3471–3480. doi: 10.1523/JNEUROSCI.3646-15.2016
Kawaguchi, Y. (1997). Selective cholinergic modulation of cortical GABAergic cell subtypes. J. Neurophysiol. 78, 1743–1747.
Kepecs, A., and Fishell, G. (2014). Interneuron cell types are fit to function. Nature 505, 318–326. doi: 10.1038/nature12983
Klausberger, T., and Somogyi, P. (2008). Neuronal diversity and temporal dynamics: the unity of hippocampal circuit operations. Science 321, 53–57. doi: 10.1126/science.1149381
Klink, R., and Alonso, A. (1997). Muscarinic modulation of the oscillatory and repetitive firing properties of entorhinal cortex layer II neurons. J. Neurophysiol. 77, 1813–1828.
Knauer, B., Jochems, A., Valero-Aracama, M. J., and Yoshida, M. (2013). Long-lasting intrinsic persistent firing in rat CA1 pyramidal cells: a possible mechanism for active maintenance of memory. Hippocampus 23, 820–831. doi: 10.1002/hipo.22136
Konopacki, J., MacIver, M. B., Bland, B. H., and Roth, S. H. (1987). Carbachol-induced EEG ‘theta’ activity in hippocampal brain slices. Brain Res. 405, 196–198. doi: 10.1016/0006-8993(87)91009-2
Kramis, R., Vanderwolf, C. H., and Bland, B. H. (1975). Two types of hippocampal rhythmical slow activity in both the rabbit and the rat: relations to behavior and effects of atropine, diethyl ether, urethane, and pentobarbital. Exp. Neurol. 49, 58–85. doi: 10.1016/0014-4886(75)90195-8
Kruglikov, I., and Rudy, B. (2008). Perisomatic GABA release and thalamocortical integration onto neocortical excitatory cells are regulated by neuromodulators. Neuron 58, 911–924. doi: 10.1016/j.neuron.2008.04.024
Kuchibhotla, K. V., Gill, J. V., Lindsay, G. W., Papadoyannis, E. S., Field, R. E., Sten, T. A., et al. (2017). Parallel processing by cortical inhibition enables context-dependent behavior. Nat. Neurosci. 20, 62–71. doi: 10.1038/nn.4436
Lambert, N. A., and Teyler, T. J. (1991). Cholinergic disinhibition in area CA1 of the rat hippocampus is not mediated by receptors located on inhibitory neurons. Eur. J. Pharmacol. 203, 129–131. doi: 10.1016/0014-2999(91)90801-v
Lawrence, J. J., Grinspan, Z. M., Statland, J. M., and McBain, C. J. (2006a). Muscarinic receptor activation tunes mouse stratum oriens interneurones to amplify spike reliability. J. Physiol. 571, 555–562. doi: 10.1113/jphysiol.2005.103218
Lawrence, J. J., Statland, J. M., Grinspan, Z. M., and McBain, C. J. (2006b). Cell type-specific dependence of muscarinic signalling in mouse hippocampal stratum oriens interneurones. J. Physiol. 570, 595–610. doi: 10.1113/jphysiol.2005.100875
Leão, R. N., Mikulovic, S., Leão, K. E., Munguba, H., Gezelius, H., Enjin, A., et al. (2012). OLM interneurons differentially modulate CA3 and entorhinal inputs to hippocampal CA1 neurons. Nat. Neurosci. 15, 1524–1530. doi: 10.1038/nn.3235
Lee, H. S., Ghetti, A., Pinto-Duarte, A., Wang, X., Dziewczapolski, G., Galimi, F., et al. (2014). Astrocytes contribute to γ oscillations and recognition memory. Proc. Natl. Acad. Sci. U S A 111, E3343–E3352. doi: 10.1073/pnas.1410893111
Leung, L. S., and Péloquin, P. (2010). Cholinergic modulation differs between basal and apical dendritic excitation of hippocampal CA1 pyramidal cells. Cereb. Cortex 20, 1865–1877. doi: 10.1093/cercor/bhp251
Leung, L. S., Shen, B., Rajakumar, N., and Ma, J. (2003). Cholinergic activity enhances hippocampal long-term potentiation in CA1 during walking in rats. J. Neurosci. 23, 9297–9304.
Leung, L. S., and Vanderwolf, C. H. (1980). Behavior-dependent evoked potentials in the hippocampal CA1 region of the rat. II. Effect of eserine, atropine, ether and pentobarbital. Brain Res. 198, 119–133. doi: 10.1016/0006-8993(80)90348-0
Levey, A. I., Edmunds, S. M., Koliatsos, V., Wiley, R. G., and Heilman, C. J. (1995). Expression of m1–m4 muscarinic acetylcholine receptor proteins in rat hippocampus and regulation by cholinergic innervation. J. Neurosci. 15, 4077–4092.
Levin, E. D. (2012). α7-Nicotinic receptors and cognition. Curr. Drug Targets 13, 602–606. doi: 10.2174/138945012800398937
Liebe, S., Hoerzer, G. M., Logothetis, N. K., and Rainer, G. (2012). θ coupling between V4 and prefrontal cortex predicts visual short-term memory performance. Nat. Neurosci. 15, 456–462, S1–S2. doi: 10.1038/nn.3038
Lisman, J. E., and Jensen, O. (2013). The θ-γ neural code. Neuron 77, 1002–1016. doi: 10.1016/j.neuron.2013.03.007
Lovett-Barron, M., Kaifosh, P., Kheirbek, M. A., Danielson, N., Zaremba, J. D., Reardon, T. R., et al. (2014). Dendritic inhibition in the hippocampus supports fear learning. Science 343, 857–863. doi: 10.1126/science.1247485
Madison, D. V., Lancaster, B., and Nicoll, R. A. (1987). Voltage clamp analysis of cholinergic action in the hippocampus. J. Neurosci. 7, 733–741.
Madison, D. V., and Nicoll, R. A. (1984). Control of the repetitive discharge of rat CA 1 pyramidal neurones in vitro. J. Physiol. 354, 319–331. doi: 10.1113/jphysiol.1984.sp015378
Manseau, F., Goutagny, R., Danik, M., and Williams, S. (2008). The hippocamposeptal pathway generates rhythmic firing of GABAergic neurons in the medial septum and diagonal bands: an investigation using a complete septohippocampal preparation in vitro. J. Neurosci. 28, 4096–4107. doi: 10.1523/JNEUROSCI.0247-08.2008
Marrosu, F., Portas, C., Mascia, M. S., Casu, M. A., Fà, M., Giagheddu, M., et al. (1995). Microdialysis measurement of cortical and hippocampal acetylcholine release during sleep-wake cycle in freely moving cats. Brain Res. 671, 329–332. doi: 10.1016/0006-8993(94)01399-3
Martin, L. A., and Alger, B. E. (1999). Muscarinic facilitation of the occurrence of depolarization-induced suppression of inhibition in rat hippocampus. Neuroscience 92, 61–71. doi: 10.1016/s0306-4522(98)00745-3
McBain, C., DiChiara, T., and Kauer, J. (1994). Activation of metabotropic glutamate receptors differentially affects two classes of hippocampal interneurons and potentiates excitatory synaptic transmission. J. Neurosci. 14, 4433–4445.
McBain, C. J., and Fisahn, A. (2001). Interneurons unbound. Nat. Rev. Neurosci. 2, 11–23. doi: 10.1038/35049047
McGaughy, J., and Sarter, M. (1998). Sustained attention performance in rats with intracortical infusions of 192 IgG-saporin-induced cortical cholinergic deafferentation: effects of physostigmine and FG 7142. Behav. Neurosci. 112, 1519–1525. doi: 10.1037/0735-7044.112.6.1519
McQuiston, A. R. (2014). Acetylcholine release and inhibitory interneuron activity in hippocampal CA1. Front. Synaptic Neurosci. 6:20. doi: 10.3389/fnsyn.2014.00020
McQuiston, A. R., and Madison, D. V. (1999a). Muscarinic receptor activity has multiple effects on the resting membrane potentials of CA1 hippocampal interneurons. J. Neurosci. 19, 5693–5702.
McQuiston, A. R., and Madison, D. V. (1999b). Muscarinic receptor activity induces an afterdepolarization in a subpopulation of hippocampal CA1 interneurons. J. Neurosci. 19, 5703–5710.
McQuiston, A. R., and Madison, D. V. (1999c). Nicotinic receptor activation excites distinct subtypes of interneurons in the rat hippocampus. J. Neurosci. 19, 2887–2896.
Mendez, M., and Lim, G. (2003). Seizures in elderly patients with dementia: epidemiology and management. Drugs Aging 20, 791–803. doi: 10.2165/00002512-200320110-00001
Miller, J. W., Turner, G. M., and Gray, B. C. (1994). Anticonvulsant effects of the experimental induction of hippocampal θ activity. Epilepsy Res. 18, 195–204. doi: 10.1016/0920-1211(94)90040-x
Minces, V., Pinto, L., Dan, Y., and Chiba, A. A. (2017). Cholinergic shaping of neural correlations. Proc. Natl. Acad. Sci. U S A 114, 5725–5730. doi: 10.1073/pnas.1621493114
Mizuseki, K., Sirota, A., Pastalkova, E., and Buzsáki, G. (2009). θ oscillations provide temporal windows for local circuit computation in the entorhinal-hippocampal loop. Neuron 64, 267–280. doi: 10.1016/j.neuron.2009.08.037
Monmaur, P., Collet, A., Puma, C., Frankel-Kohn, L., and Sharif, A. (1997). Relations between acetylcholine release and electrophysiological characteristics of θ rhythm: a microdialysis study in the urethane-anesthetized rat hippocampus. Brain Res. Bull. 42, 141–146. doi: 10.1016/s0361-9230(96)00200-6
Nagode, D. A., Tang, A.-H., Karson, M. A., Klugmann, M., and Alger, B. E. (2011). Optogenetic release of ACh induces rhythmic bursts of perisomatic IPSCs in hippocampus. PLoS One 6:e27691. doi: 10.1371/journal.pone.0027691
Nagode, D. A., Tang, A.-H., Yang, K., and Alger, B. E. (2014). Optogenetic identification of an intrinsic cholinergically driven inhibitory oscillator sensitive to cannabinoids and opioids in hippocampal CA1. J. Physiol. 592, 103–123. doi: 10.1113/jphysiol.2013.257428
Navarrete, M., Perea, G., Fernandez de Sevilla, D., Gómez-Gonzalo, M., Núñez, A., Martín, E. D., et al. (2012). Astrocytes mediate in vivo cholinergic-induced synaptic plasticity. PLoS Biol. 10:e1001259. doi: 10.1371/journal.pbio.1001259
Newman, E. L., Gillet, S. N., Climer, J. R., and Hasselmo, M. E. (2013). Cholinergic blockade reduces θ-γ phase amplitude coupling and speed modulation of θ frequency consistent with behavioral effects on encoding. J. Neurosci. 33, 19635–19646. doi: 10.1523/JNEUROSCI.2586-13.2013
Newman, E. L., Shay, C. F., and Hasselmo, M. E. (2012). Malignant synaptic growth and Alzheimer’s disease. Future Neurol. 7, 557–571. doi: 10.2217/fnl.12.47
Ohno, M., Yamamoto, T., and Watanabe, S. (1993). Blockade of hippocampal nicotinic receptors impairs working memory but not reference memory in rats. Pharmacol. Biochem. Behav. 45, 89–93. doi: 10.1016/0091-3057(93)90091-7
Ohno, M., Yamamoto, T., and Watanabe, S. (1994). Blockade of hippocampal M1 muscarinic receptors impairs working memory performance of rats. Brain Res. 650, 260–266. doi: 10.1016/0006-8993(94)91790-6
Pabst, M., Braganza, O., Dannenberg, H., Hu, W., Pothmann, L., Rosen, J., et al. (2016). Astrocyte intermediaries of septal cholinergic modulation in the hippocampus. Neuron 90, 853–865. doi: 10.1016/j.neuron.2016.04.003
Papouin, T., Dunphy, J. M., Tolman, M., Dineley, K. T., and Haydon, P. G. (2017). Septal cholinergic neuromodulation tunes the astrocyte-dependent gating of hippocampal NMDA receptors to wakefulness. Neuron 94, 840.e7–854.e7. doi: 10.1016/j.neuron.2017.04.021
Parent, M. B., and Baxter, M. G. (2004). Septohippocampal acetylcholine: involved in but not necessary for learning and memory? Learn. Mem. 11, 9–20. doi: 10.1101/lm.69104
Perry, E. K., Morris, C. M., Court, J. A., Cheng, A., Fairbairn, A. F., McKeith, I. G., et al. (1995). Alteration in nicotine binding sites in Parkinson’s disease, Lewy body dementia and Alzheimer’s disease: possible index of early neuropathology. Neuroscience 64, 385–395. doi: 10.1016/0306-4522(94)00410-7
Pettit, D. L., Shao, Z., and Yakel, J. L. (2001). β-amyloid(1–42) peptide directly modulates nicotinic receptors in the rat hippocampal slice. J. Neurosci. 21:RC120.
Pfeffer, C. K., Xue, M., He, M., Huang, Z. J., and Scanziani, M. (2013). Inhibition of inhibition in visual cortex: the logic of connections between molecularly distinct interneurons. Nat. Neurosci. 16, 1068–1076. doi: 10.1038/nn.3446
Pi, H.-J., Hangya, B., Kvitsiani, D., Sanders, J. I., Huang, Z. J., and Kepecs, A. (2013). Cortical interneurons that specialize in disinhibitory control. Nature 503, 521–524. doi: 10.1038/nature12676
Picciotto, M. R., Higley, M. J., and Mineur, Y. S. (2012). Acetylcholine as a neuromodulator: cholinergic signaling shapes nervous system function and behavior. Neuron 76, 116–129. doi: 10.1016/j.neuron.2012.08.036
Pinto, L., Goard, M. J., Estandian, D., Xu, M., Kwan, A. C., Lee, S.-H., et al. (2013). Fast modulation of visual perception by basal forebrain cholinergic neurons. Nat. Neurosci. 16, 1857–1863. doi: 10.1038/nn.3552
Pitler, T. A., and Alger, B. E. (1992). Cholinergic excitation of GABAergic interneurons in the rat hippocampal slice. J. Physiol. 450, 127–142. doi: 10.1113/jphysiol.1992.sp019119
Poorthuis, R. B., Bloem, B., Schak, B., Wester, J., de Kock, C. P., and Mansvelder, H. D. (2013). Layer-specific modulation of the prefrontal cortex by nicotinic acetylcholine receptors. Cereb. Cortex 23, 148–161. doi: 10.1093/cercor/bhr390
Pouille, F., and Scanziani, M. (2001). Enforcement of temporal fidelity in pyramidal cells by somatic feed-forward inhibition. Science 293, 1159–1163. doi: 10.1126/science.1060342
Power, J. M., and Sah, P. (2002). Nuclear calcium signaling evoked by cholinergic stimulation in hippocampal CA1 pyramidal neurons. J. Neurosci. 22, 3454–3462.
Quiroz, Y. T., Budson, A. E., Celone, K., Ruiz, A., Newmark, R., Castrillón, G., et al. (2010). Hippocampal hyperactivation in presymptomatic familial Alzheimer’s disease. Ann. Neurol. 68, 865–875. doi: 10.1002/ana.22105
Radcliffe, K. A., Fisher, J. L., Gray, R., and Dani, J. A. (1999). Nicotinic modulation of glutamate and GABA synaptic transmission of hippocampal neurons. Ann. N Y Acad. Sci. 868, 591–610. doi: 10.1111/j.1749-6632.1999.tb11332.x
Raiteri, M., Leardi, R., and Marchi, M. (1984). Heterogeneity of presynaptic muscarinic receptors regulating neurotransmitter release in the rat brain. J. Pharmacol. Exp. Ther. 228, 209–214.
Reece, L. J., and Schwartzkroin, P. A. (1991). Effects of cholinergic agonists on two non-pyramidal cell types in rat hippocampal slices. Brain Res. 566, 115–126. doi: 10.1016/0006-8993(91)91688-w
Robinson, D. A. (1972). On the nature of visual-oculomotor connections. Invest. Ophthalmol. 11, 497–503.
Rogers, J. L., and Kesner, R. P. (2003). Cholinergic modulation of the hippocampus during encoding and retrieval. Neurobiol. Learn. Mem. 80, 332–342. doi: 10.1016/s1074-7427(03)00063-7
Romo, R., Brody, C. D., Hernández, A., and Lemus, L. (1999). Neuronal correlates of parametric working memory in the prefrontal cortex. Nature 399, 470–473. doi: 10.1038/20939
Rouse, S. T., Edmunds, S. M., Yi, H., Gilmor, M. L., and Levey, A. I. (2000). Localization of M2 muscarinic acetylcholine receptor protein in cholinergic and non-cholinergic terminals in rat hippocampus. Neurosci. Lett. 284, 182–186. doi: 10.1016/s0304-3940(00)01011-9
Rouse, S. T., Gilmor, M. L., and Levey, A. I. (1998). Differential presynaptic and postsynaptic expression of m1–m4 muscarinic acetylcholine receptors at the perforant pathway/granule cell synapse. Neuroscience 86, 221–232. doi: 10.1016/s0306-4522(97)00681-7
Rovira, C., Ben-Ari, Y., and Cherubini, E. (1983). Dual cholinergic modulation of hippocampal somatic and dendritic field potentials by the septo-hippocampal pathway. Exp. Brain Res. 49, 151–155. doi: 10.1007/bf00235552
Rudy, B., Fishell, G., Lee, S., and Hjerling-Leffler, J. (2011). Three groups of interneurons account for nearly 100% of neocortical GABAergic neurons. Dev. Neurobiol. 71, 45–61. doi: 10.1002/dneu.20853
Rutishauser, U., Ross, I. B., Mamelak, A. N., and Schuman, E. M. (2010). Human memory strength is predicted by θ-frequency phase-locking of single neurons. Nature 464, 903–907. doi: 10.1038/nature08860
Sarter, M., Hasselmo, M. E., Bruno, J. P., and Givens, B. (2005). Unraveling the attentional functions of cortical cholinergic inputs: interactions between signal-driven and cognitive modulation of signal detection. Brain Res. Rev. 48, 98–111. doi: 10.1016/j.brainresrev.2004.08.006
Schmid, L. C., Mittag, M., Poll, S., Steffen, J., Wagner, J., Geis, H.-R., et al. (2016). Dysfunction of somatostatin-positive interneurons associated with memory deficits in an Alzheimer’s disease model. Neuron 92, 114–125. doi: 10.1016/j.neuron.2016.08.034
Schon, K., Atri, A., Hasselmo, M. E., Tricarico, M. D., LoPresti, M. L., and Stern, C. E. (2005). Scopolamine reduces persistent activity related to long-term encoding in the parahippocampal gyrus during delayed matching in humans. J. Neurosci. 25, 9112–9123. doi: 10.1523/jneurosci.1982-05.2005
Schon, K., Hasselmo, M. E., Lopresti, M. L., Tricarico, M. D., and Stern, C. E. (2004). Persistence of parahippocampal representation in the absence of stimulus input enhances long-term encoding: a functional magnetic resonance imaging study of subsequent memory after a delayed match-to-sample task. J. Neurosci. 24, 11088–11097. doi: 10.1523/jneurosci.3807-04.2004
Séguéla, P., Wadiche, J., Dineley-Miller, K., Dani, J. A., and Patrick, J. W. (1993). Molecular cloning, functional properties, and distribution of rat brain α7: a nicotinic cation channel highly permeable to calcium. J. Neurosci. 13, 596–604.
Serrano-Pozo, A., Frosch, M. P., Masliah, E., and Hyman, B. T. (2011). Neuropathological alterations in Alzheimer disease. Cold Spring Harb. Perspect. Med. 1:a006189. doi: 10.1101/cshperspect.a006189
Shapiro, M. L., Simon, D. K., Olton, D. S., Gage, F. H. III, Nilsson, O., and Björklund, A. (1989). Intrahippocampal grafts of fetal basal forebrain tissue alter place fields in the hippocampus of rats with fimbria-fornix lesions. Neuroscience 32, 1–18. doi: 10.1016/0306-4522(89)90103-6
Sharma, G., and Vijayaraghavan, S. (2001). Nicotinic cholinergic signaling in hippocampal astrocytes involves calcium-induced calcium release from intracellular stores. Proc. Natl. Acad. Sci. U S A 98, 4148–4153. doi: 10.1073/pnas.071540198
Sharma, G., and Vijayaraghavan, S. (2003). Modulation of presynaptic store calcium induces release of glutamate and postsynaptic firing. Neuron 38, 929–939. doi: 10.1016/s0896-6273(03)00322-2
Shen, J.-X., and Yakel, J. L. (2012). Functional α7 nicotinic ACh receptors on astrocytes in rat hippocampal CA1 slices. J. Mol. Neurosci. 48, 14–21. doi: 10.1007/s12031-012-9719-3
Shinoe, T., Matsui, M., Taketo, M. M., and Manabe, T. (2005). Modulation of synaptic plasticity by physiological activation of M1 muscarinic acetylcholine receptors in the mouse hippocampus. J. Neurosci. 25, 11194–11200. doi: 10.1523/jneurosci.2338-05.2005
Shirey, J. K., Xiang, Z., Orton, D., Brady, A. E., Johnson, K. A., Williams, R., et al. (2008). An allosteric potentiator of M4 mAChR modulates hippocampal synaptic transmission. Nat. Chem. Biol. 4, 42–50. doi: 10.1038/nchembio.2007.55
Siegle, J. H., and Wilson, M. A. (2014). Enhancement of encoding and retrieval functions through θ phase-specific manipulation of hippocampus. Elife 3:e03061. doi: 10.7554/elife.03061
Skaggs, W. E., McNaughton, B. L., Wilson, M. A., and Barnes, C. A. (1996). θ phase precession in hippocampal neuronal populations and the compression of temporal sequences. Hippocampus 6, 149–172. doi: 10.1002/(SICI)1098-1063(1996)6:2<149::AID-HIPO6>3.0.CO;2-K
Smith, M. L., Deadwyler, S. A., and Booze, R. M. (1993). 3-D reconstruction of the cholinergic basal forebrain system in young and aged rats. Neurobiol. Aging 14, 389–392. doi: 10.1016/0197-4580(93)90126-v
Somogyi, P., and Klausberger, T. (2005). Defined types of cortical interneurone structure space and spike timing in the hippocampus. J. Physiol. 562, 9–26. doi: 10.1113/jphysiol.2004.078915
Stancampiano, R., Cocco, S., Cugusi, C., Sarais, L., and Fadda, F. (1999). Serotonin and acetylcholine release response in the rat hippocampus during a spatial memory task. Neuroscience 89, 1135–1143. doi: 10.1016/s0306-4522(98)00397-2
Stanley, E. M., Wilson, M. A., and Fadel, J. R. (2012). Hippocampal neurotransmitter efflux during one-trial novel object recognition in rats. Neurosci. Lett. 511, 38–42. doi: 10.1016/j.neulet.2012.01.033
Stewart, M., and Fox, S. E. (1990). Do septal neurons pace the hippocampal θ rhythm? Trends Neurosci. 13, 163–168. doi: 10.1016/0166-2236(90)90040-h
Suzuki, W. A., Miller, E. K., and Desimone, R. (1997). Object and place memory in the macaque entorhinal cortex. J. Neurophysiol. 78, 1062–1081.
Szabó, G. G., Holderith, N., Gulyás, A. I., Freund, T. F., and Hájos, N. (2010). Distinct synaptic properties of perisomatic inhibitory cell types and their different modulation by cholinergic receptor activation in the CA3 region of the mouse hippocampus. Eur. J. Neurosci. 31, 2234–2246. doi: 10.1111/j.1460-9568.2010.07292.x
Tahvildari, B., Fransén, E., Alonso, A. A., and Hasselmo, M. E. (2007). Switching between “On” and “Off” states of persistent activity in lateral entorhinal layer III neurons. Hippocampus 17, 257–263. doi: 10.1002/hipo.20270
Takacs, V. T., Cserep, C., Schlingloff, D., Posfai, B., Szonyi, A., Sos, K. E., et al. (2017). Synaptic co-transmission of acetylcholine and GABA regulates hippocampal states. bioRxiv 193318. doi: 10.1101/193318
Takata, N., Mishima, T., Hisatsune, C., Nagai, T., Ebisui, E., Mikoshiba, K., et al. (2011). Astrocyte calcium signaling transforms cholinergic modulation to cortical plasticity in vivo. J. Neurosci. 31, 18155–18165. doi: 10.1016/j.neures.2011.07.1142
Taube, J. S., and Bassett, J. P. (2003). Persistent neural activity in head direction cells. Cereb. Cortex 13, 1162–1172. doi: 10.1093/cercor/bhg102
Taube, J. S., and Muller, R. U. (1998). Comparisons of head direction cell activity in the postsubiculum and anterior thalamus of freely moving rats. Hippocampus 8, 87–108. doi: 10.1002/(sici)1098-1063(1998)8:2<87::aid-hipo1>3.0.co;2-4
Taylor, L., and Griffith, W. H. (1993). Age-related decline in cholinergic synaptic transmission in hippocampus. Neurobiol. Aging 14, 509–515. doi: 10.1016/0197-4580(93)90110-w
Tóth, K., Freund, T. F., and Miles, R. (1997). Disinhibition of rat hippocampal pyramidal cells by GABAergic afferents from the septum. J. Physiol. 500, 463–474. doi: 10.1113/jphysiol.1997.sp022033
Tsubokawa, H., and Ross, W. N. (1997). Muscarinic modulation of spike backpropagation in the apical dendrites of hippocampal CA1 pyramidal neurons. J. Neurosci. 17, 5782–5791.
Umbriaco, D., Garcia, S., Beaulieu, C., and Descarries, L. (1995). Relational features of acetylcholine, noradrenaline, serotonin and GABA axon terminals in the stratum radiatum of adult rat hippocampus (CA1). Hippocampus 5, 605–620. doi: 10.1002/hipo.450050611
Valentino, R. J., and Dingledine, R. (1981). Presynaptic inhibitory effect of acetylcholine in the hippocampus. J. Neurosci. 1, 784–792.
Vallés, A. S., Borroni, M. V., and Barrantes, F. J. (2014). Targeting brain α7 nicotinic acetylcholine receptors in Alzheimer’s disease: rationale and current status. CNS Drugs 28, 975–987. doi: 10.1007/s40263-014-0201-3
Van Der Zee, E. A., De Jong, G. I., Strosberg, A. D., and Luiten, P. G. (1993). Muscarinic acetylcholine receptor-expression in astrocytes in the cortex of young and aged rats. Glia 8, 42–50. doi: 10.1002/glia.440080106
Vandecasteele, M., Varga, V., Berenyi, A., Papp, E., Bartho, P., Venance, L., et al. (2014). Optogenetic activation of septal cholinergic neurons suppresses sharp wave ripples and enhances θ oscillations in the hippocampus. Proc. Natl. Acad. Sci. U S A 111, 13535–13540. doi: 10.1073/pnas.1411233111
Varga, V., Hangya, B., Kránitz, K., Ludányi, A., Zemankovics, R., Katona, I., et al. (2008). The presence of pacemaker HCN channels identifies θ rhythmic GABAergic neurons in the medial septum. J. Physiol. 586, 3893–3915. doi: 10.1113/jphysiol.2008.155242
Vilaró, M. T., Palacios, J. M., and Mengod, G. (1990). Localization of M5 muscarinic receptor mRNA in rat brain examined by in situ hybridization histochemistry. Neurosci. Lett. 114, 154–159. doi: 10.1016/0304-3940(90)90064-g
Wall, S. J., Yasuda, R. P., Li, M., Ciesla, W., and Wolfe, B. B. (1992). Differential regulation of subtypes m1–m5 of muscarinic receptors in forebrain by chronic atropine administration. J. Pharmacol. Exp. Ther. 262, 584–588.
Wang, H.-Y., Lee, D. H., D’Andrea, M. R., Peterson, P. A., Shank, R. P., and Reitz, A. B. (2000a). β-amyloid1–42 binds to α7 nicotinic acetylcholine receptor with high affinity. Implications for Alzheimer’s disease pathology. J. Biol. Chem. 275, 5626–5632. doi: 10.1074/jbc.275.8.5626
Wang, H.-Y., Lee, D. H., Davis, C. B., and Shank, R. P. (2000b). Amyloid peptide Aβ(1–42) binds selectively and with picomolar affinity to α7 nicotinic acetylcholine receptors. J. Neurochem. 75, 1155–1161. doi: 10.1046/j.1471-4159.2000.0751155.x
Wess, J. (2003). Novel insights into muscarinic acetylcholine receptor function using gene targeting technology. Trends Pharmacol. Sci. 24, 414–420. doi: 10.1016/s0165-6147(03)00195-0
Widmer, H., Ferrigan, L., Davies, C. H., and Cobb, S. R. (2006). Evoked slow muscarinic acetylcholinergic synaptic potentials in rat hippocampal interneurons. Hippocampus 16, 617–628. doi: 10.1002/hipo.20191
Williams, S., and Johnston, D. (1988). Muscarinic depression of long-term potentiation in CA3 hippocampal neurons. Science 242, 84–87. doi: 10.1126/science.2845578
Winson, J. (1978). Loss of hippocampal θ rhythm results in spatial memory deficit in the rat. Science 201, 160–163. doi: 10.1126/science.663646
Xiang, Z., Huguenard, J. R., and Prince, D. A. (1998). Cholinergic switching within neocortical inhibitory networks. Science 281, 985–988. doi: 10.1126/science.281.5379.985
Xu, M., Chung, S., Zhang, S., Zhong, P., Ma, C., Chang, W.-C., et al. (2015). Basal forebrain circuit for sleep-wake control. Nat. Neurosci. 18, 1641–1647. doi: 10.1038/nn.4143
Yamasaki, M., Matsui, M., and Watanabe, M. (2010). Preferential localization of muscarinic M1 receptor on dendritic shaft and spine of cortical pyramidal cells and its anatomical evidence for volume transmission. J. Neurosci. 30, 4408–4418. doi: 10.1523/jneurosci.5719-09.2010
Yi, F., Ball, J., Stoll, K. E., Satpute, V. C., Mitchell, S. M., Pauli, J. L., et al. (2014). Direct excitation of parvalbumin-positive interneurons by M1 muscarinic acetylcholine receptors: roles in cellular excitability, inhibitory transmission and cognition. J. Physiol. 592, 3463–3494. doi: 10.1113/jphysiol.2014.275453
Yoshida, M., Fransén, E., and Hasselmo, M. E. (2008). mGluR-dependent persistent firing in entorhinal cortex layer III neurons. Eur. J. Neurosci. 28, 1116–1126. doi: 10.1111/j.1460-9568.2008.06409.x
Yoshida, M., and Hasselmo, M. E. (2009). Persistent firing supported by an intrinsic cellular mechanism in a component of the head direction system. J. Neurosci. 29, 4945–4952. doi: 10.1523/jneurosci.5154-08.2009
Young, B. J., Otto, T., Fox, G. D., and Eichenbaum, H. (1997). Memory representation within the parahippocampal region. J. Neurosci. 17, 5183–5195.
Yu, A. J., and Dayan, P. (2005). Uncertainty, neuromodulation, and attention. Neuron 46, 681–692. doi: 10.1016/j.neuron.2005.04.026
Zhang, H., Lin, S.-C., and Nicolelis, M. A. L. (2010). Spatiotemporal coupling between hippocampal acetylcholine release and θ oscillations in vivo. J. Neurosci. 30, 13431–13440. doi: 10.1523/jneurosci.1144-10.2010
Keywords: acetylcholine, presynaptic inhibition, tonic depolarization, cholinergic fibers, volume transmission
Citation: Dannenberg H, Young K and Hasselmo M (2017) Modulation of Hippocampal Circuits by Muscarinic and Nicotinic Receptors. Front. Neural Circuits 11:102. doi: 10.3389/fncir.2017.00102
Received: 01 October 2017; Accepted: 27 November 2017;
Published: 13 December 2017.
Edited by:
Oscar Herreras, Consejo Superior de Investigaciones Científicas (CSIC), SpainReviewed by:
Jerrel Yakel, National Institute of Environmental Health Sciences (NIH), United StatesHeiko J. Luhmann, Johannes Gutenberg-Universität Mainz, Germany
Copyright © 2017 Dannenberg, Young and Hasselmo. This is an open-access article distributed under the terms of the Creative Commons Attribution License (CC BY). The use, distribution or reproduction in other forums is permitted, provided the original author(s) or licensor are credited and that the original publication in this journal is cited, in accordance with accepted academic practice. No use, distribution or reproduction is permitted which does not comply with these terms.
*Correspondence: Holger Dannenberg, aGRhbm5lbmJAZ21haWwuY29t