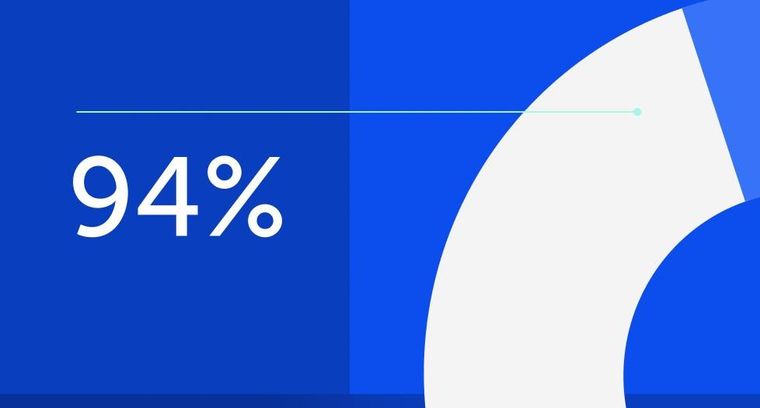
94% of researchers rate our articles as excellent or good
Learn more about the work of our research integrity team to safeguard the quality of each article we publish.
Find out more
REVIEW article
Front. Neural Circuits, 08 April 2014
Volume 8 - 2014 | https://doi.org/10.3389/fncir.2014.00033
This article is part of the Research TopicNeuromodulation of Executive CircuitsView all 20 articles
A commentary has been posted on this article:
Corrigendum: “The role of prefrontal catecholamines in attention and working memory”
While much progress has been made in identifying the brain regions and neurochemical systems involved in the cognitive processes disrupted in mental illnesses, to date, the level of detail at which neurobiologists can describe the chain of events giving rise to cognitive functions is very rudimentary. Much of the intense interest in understanding cognitive functions is motivated by the hope that it might be possible to understand these complex functions at the level of neurons and neural circuits. Here, we review the current state of the literature regarding how modulations in catecholamine levels within the prefrontal cortex (PFC) alter the neuronal and behavioral correlates of cognitive functions, particularly attention and working memory.
Attention, working memory, impulse control, and other “top-down” cognitive functions have long been known to depend on the prefrontal cortex (PFC) (Ghent et al., 1962; Chao and Knight, 1998; D'Esposito and Postle, 1999). Many of these cognitive functions are disrupted in mental disorders such as attention deficit hyperactivity disorder (ADHD), Parkinson's disease, and schizophrenia. Studies in human and non-human primates have implicated prefrontal catecholamines in control of cognitive functions. Notably, drugs altering catecholamine signaling have been used to treat the symptoms of some of these mental illnesses. Consequently, an imbalance in prefrontal catecholamines has long been a suspected cause of the cognitive component of these mental illnesses. Our goal is to review studies examining the contribution of prefrontal catecholamines to cognitive tasks and their dysfunction. Due to known differences between rodents and primates (Berger et al., 1991), this review will be focused on studies in human and non-human primates. Among catecholamines, the main focus will be on dopamine (DA), however the role of norepinephrine (NE) will also be briefly addressed. We survey the evidence implicating prefrontal catecholamines as the neurochemical mediator of the neural and behavioral signatures of attention and working memory, and link these neurobiological findings to the etiology and treatment of cognitive impairments in mental disorders.
The importance of prefrontal DA in delayed-response tasks was established very early on (Brozoski et al., 1979), and much work has since gone into unraveling the details of this dependence (see Table 1). The PFC receives DA-ergic projections from both the ventral tegmental area (VTA) and the substantia nigra (Porrino and Goldman-Rakic, 1982; Levitt et al., 1984; Goldman-Rakic et al., 1992). DA neurons in the VTA and substantia nigra exhibit both tonic activity, and phasic responses associated with the expectation of reward (Schultz et al., 1993) or reward prediction errors (Schultz, 1998). While DA neurons are activated by the spatial cue in the working memory tasks discussed below (since it signals the availability of a reward in the near future), this activation differs from that observed in PFC itself in that it does not reflect the cue position, nor does it continue throughout the delay period (Schultz et al., 1993). Therefore, the incoming DA-ergic input to PFC does not directly encode the remembered stimulus, but could potentially serve to “tune” the prefrontal network for optimal activity.
Table 1. Studies examining the contribution of prefrontal catecholamines to the behavioral and neural correlates of working memory in non-human primates.
In order to understand the effects of prefrontal DA release on neural activity, first let us consider DA receptors and the anatomy of DA-ergic terminals in PFC. DA receptors are G-protein-coupled receptors, modulating neuronal activity via intracellular signaling cascades rather than directly inducing either excitatory or inhibitory postsynaptic currents (Yang and Seamans, 1996; Lachowicz and Sibley, 1997; Missale et al., 1998). The five types of DA receptor are commonly divided into two classes: the D1 family (comprised of D1 and D5 receptors) and the D2 family (D2, D3, and D4 receptors) (Missale et al., 1998; Seamans and Yang, 2004). Expression for D1 receptors (D1Rs) is enriched in the PFC of both primates and rodents, suggesting an important role in specifically prefrontal circuit functions (Lidow et al., 1991; Goldman-Rakic et al., 1992). Within PFC, D1Rs are expressed in both superficial and deep cortical layers, while expression of the less abundant D2Rs is limited to the infragranular layers (Lidow et al., 1991). Although this bilaminar distribution pattern is evident at birth, layer three undergoes a dramatic post-natal increase in the density of DA innervation, which is then subject to layer-specific remodeling and decreases in DA axon density during adolescence (Lewis and Harris, 1991; Rosenberg and Lewis, 1995; Lewis, 1997); during this period performance on delayed response tasks improves and becomes more dependent on the PFC (Alexander and Goldman, 1978). Goldman-Rakic and colleagues used immunohistological staining, Golgi impregnation and electron microscopy to examine DA-ergic synapses in the PFC. They found that DA-ergic boutons were part of synaptic triads, in which the DA-positive bouton formed a symmetric synapse, while an unlabeled asymmetric synapse (of the type associated with excitatory inputs) contacted the same dendritic spine (Figure 1A). Many of the postsynaptic neurons appear to be pyramidal cells. However, targets of DA-ergic projections include both pyramidal cells and fast-spiking interneurons (Goldman-Rakic et al., 1989; Sesack et al., 1995, 1998). D1Rs can also be located outside of synapses (Smiley et al., 1994), suggesting at least some slow timecourse effects as DA diffuses to these more remote sites of action. Some DA axonal varicosities also appear to be localized outside of synaptic specializations (Smiley and Goldman-Rakic, 1993), and may contribute to extrasynaptic “volume transmission” effects (Zoli et al., 1998). This anatomy seems conducive to dopamine playing a modulatory role, regulating the efficacy or strength of prefrontal signals originating elsewhere.
Figure 1. DA modulates the efficacy of synaptic connections within PFC. (A) Cartoon illustrates a characteristic “synaptic triad” in monkey PFC: the same spine (S) is postsynaptic to both a DA-positive axon (DA) and a non-immunoreactive axon terminal (non-DA). The synapse of the DA axon is symmetric, while the non-DA axon forms an asymmetric synapse. Based on electron micrography and immunostaining of synaptic connections in layer II of monkey PFC (from Goldman-Rakic et al., 1989). (B) D1R signaling alters synaptic efficacy, a measured by changes in the synchronous firing of pairs of PFC neurons. Castner and Williams (2007) simultaneously recorded from pairs of neurons in monkey PFC before and after delivering a D1R agonist or antagonist using in vivo iontophoresis. Cross-correlograms depict the number of spikes occurring at a particular time-lag relative to spikes of the other neuron in the pair: a peak at 0 ms indicates simultaneous firing due to common input. The top pair of PFC neurons exhibited a 0 ms peak prior to drug infusion (gray): application of a D1R agonist eliminated this peak (red), presumably by disrupting the efficacy of common input. In the bottom plot, the neurons did not show evidence of common input during the control recordings (gray), but a peak emerged following application of a D1R antagonist (red), reflecting stronger or more reliable excitation from their common inputs. (C) Effects of D1R agonists and antagonists on working memory performance will depend on initial levels of PFC DA. Gray curves illustrate working memory performance as a function of PFC DA level: performance is greatest at an intermediate level, with insufficient or excessive DA leading to impaired performance. Basal DA levels (illustrated by the dashed lines) are usually tuned for optimal performance (middle curve), but are sub-optimal in aged animals (back curve), and above optimal in the case of stress (front curve). D1R agonists (red arrows) and antagonists (blue arrows) effect working memory performance differently based on initial DA levels: if initial DA levels are supra-optimal, as in stress, then D1R antagonists will move DA signaling toward the optimal level, improving performance, while agonists will further impair performance. If initial DA levels are below optimal, as in aged animals, then D1R agonists will increase DA signaling back toward optimal levels, improving performance; D1R antagonists will move DA levels further from optimal, impairing performance.
The role of dopamine in modulating glutamatergic activity, suggested by the presence of synaptic triads, has been directly tested in slices using dual whole-cell patch clamp recordings, examining the effect of DA application on synaptic transmission between neurons. These experiments revealed that DA reduces the reliability of excitatory neurotransmission by reducing the probability of glutamate release presynaptically (Gao et al., 2001). Consistent with this finding, the consequences of this reduced reliability of synaptic transmission can be read out in the synchronous activity of neighboring prefrontal neurons in vivo (Figure 1B): iontophoretic application of a D1R antagonist introduces a peak in the cross-correlogram between prefrontal pyramidal neurons, as the reliability of transmission from mutual inputs increases (Castner and Williams, 2007). Conversely, iontophoretic application of a D1R agonist decreases synaptic efficacy, disrupting common excitatory input and eliminate existing cross-correlogram peaks for neighboring prefrontal neurons. Interestingly, DA may have different direct effects on excitatory and inhibitory neurons within PFC (Gao and Goldman-Rakic, 2003; Jacob et al., 2013). Using iontophoresis to examine the effects of DA on prefrontal visual responses during a visual detection task, Jacob and colleagues found two distinct types of DA-ergic modulation (Jacob et al., 2013) One group of PFC neurons, which included all the modulated narrow-spiking, putatively inhibitory neurons, was inhibited by DA; these showed short onset latency of DA effects (~10 ms), with no change in signal-to-noise ratio (SNR) or inter-trial variability. A second set of prefrontal neurons was excited by DA application, displaying an increase in SNR and decrease in inter-trial variability; this effect was slower (~200 ms) and observed only in broad-spiking, putatively pyramidal neurons. These direct effects of DA on the excitability of individual neurons of different types will then interact at the population level—for example, the activity of inhibitory neurons helps shape the tuning of excitatory neurons during working memory (Rao et al., 2000; Constantinidis et al., 2002).
In addition to modulating neural activity within PFC, DA also alters activity-dependent plasticity (Gurden et al., 2000; Pawlak and Kerr, 2008; Zhang et al., 2009). Such plasticity has long been the focus of study in relation to addiction; however, these changes may also play a more general role in associative learning. Recent work in rodent PFC suggests that both D1R and D2R signaling contribute to changes in plasticity, with D2R actions on inhibitory interneurons gating potentiation, and D1Rs on postsynaptic neurons controlling the size of the temporal window during which coincident spikes induce potentiation (Xu and Yao, 2010). These effects of DA on plasticity, and the known firing of DA inputs to PFC in response to prediction errors, suggest an influence of prefrontal DA on learning, and indeed a recent study reveals just such an effect (Puig and Miller, 2012). Local injection of a D1R antagonist into ventrolateral prefrontal cortex (vlPFC) was found to impair monkeys' acquisition of novel visuomotor associations, without impairing performance on familiar associations. This behavioral effect was accompanied by changes in prefrontal activity, again observed for novel but not familiar cues: selectivity of individual neurons for the upcoming motor response decreased, while synchronous discharge and low-frequency LFP power increased. Human experiments have also linked phasic activity in midbrain DA nuclei and PFC with context-dependent working memory performance (D'Ardenne et al., 2012). Thus, phasic discharge of prefrontal DA inputs may be particularly important during the learning of novel associations and tasks, or contextual switching between rules.
Williams and Goldman-Rakic used iontophoresis in a behaving monkey to extend these findings on the cellular effects of DA to its role in prefrontal circuits during a spatial working memory task (Williams and Goldman-Rakic, 1995). Iontophoretic application of a D1R antagonist during spatial working memory selectively enhances delay-period activity representing the remembered location. The effect is dose-dependent, with enhanced delay activity for an intermediate level of D1R antagonist, but suppression of both delay and visual activity in the same cell when a greater concentration of the drug is applied. This “inverted-U” dose-dependency, in which an intermediate level of DA signaling produces more selective memory activity, has also been observed for a D1R agonist: low doses suppressed only responses to non-preferred locations, enhancing the spatial tuning of delay activity, while higher doses suppressed activity altogether (Vijayraghavan et al., 2007). How to reconcile the apparently contradictory findings that a low dose of either a D1R agonist (Vijayraghavan et al., 2007) or a D1R antagonist (Williams and Goldman-Rakic, 1995) improves the selectivity of PFC delay activity? Presumably the answer lies in the original level of DA-ergic tone in the PFC neurons being studied (Figure 1C), although it remains unclear why these studies would have a systematic difference in baseline prefrontal DA-ergic stimulation. The known elevation of prefrontal DA by stress (Thierry et al., 1976; Roth et al., 1988; Abercrombie et al., 1989) further raises questions as to how the stresses affecting laboratory animals may impact their baseline DA-ergic tone, and thus the effects of pharmacological agents. Also note that the basis for improved delay selectivity appears to differ for the two agents: D1R antagonists may improve tuning by increasing the level of delay firing for the preferred location, while the D1R agonist selectively reduces firing for the non-preferred cue locations.
Several biologically-plausible neurocomputational models have been developed to incorporate DA or NE-ergic modulation of prefrontal activity (Servan-Schreiber et al., 1998; Durstewitz et al., 2000; Brunel and Wang, 2001; Chadderdon and Sporns, 2006; Eckhoff et al., 2009; Avery et al., 2013). One such model (Chadderdon and Sporns, 2006) of task-oriented behavioral selection incorporates such disparate brain regions as early visual areas, inferotemporal cortex, PFC, basal ganglia, and anterior cingulate cortex. At the heart of this model is a mechanism that simulates exogenously induced changes in prefrontal DA release, which is thought to underlie the updating and maintenance functions of working memory. More recently, Avery et al. (2013) constructed a model of PFC designed to capture the effects of signaling through both DA (D1) and NE (alpha-2A and alpha-1) receptors. Both of these models were able to reproduce the “inverted-U” effect of catecholamine signaling, with impaired working memory representations when levels were too high or too low. The former model incorporated changes in prefrontal DA levels over the course of a delayed match to sample task, using these changes to switch the prefrontal network between states of updating based on current inputs vs. maintaining previous inputs, while the latter instead examined the effects of tonic DA and NE tone on network behavior. The extent to which fluctuations in PFC DA levels during different task epochs occur or contribute to task performance remains experimentally unproven.
The effect of DA on the activity of prefrontal neurons is complicated, involving multiple mechanisms of direct and indirect action through D1Rs and D2Rs, affecting presynaptic release, NMDA, GABA, AMPA, Na+, Ca2+, and K+ currents, among others (Seamans and Yang, 2004). Various studies have reported either primarily inhibitory (e.g., Pirot et al., 1992), excitatory (e.g., Henze et al., 2000), or heterogenous (Jacob et al., 2013) effects of DA on PFC neurons. The main points we wish to emphasize here are that DA acts as a neuromodulator, altering the efficacy of synaptic input to prefrontal neurons, and that there is some optimal level of DA-ergic stimulation for a neuron to experience, with greater or lesser DA signaling leading to an erosion of task related activity.
Given the known firing of prefrontal DA afferents in response to reward expectation (Schultz, 2013), and the ability of expected reward to modulate responses throughout the brain, we cannot discuss the role of DA in prefrontal control of cognitive functions without considering the effects of reward and to what extent they can be separated from the other roles of prefrontal DA signaling. In the following sections we first discuss the difficulties in parsing the behavioral effects and neural signatures of attention and reward. We review the known role of both prefrontal DA and reward in modulating responses in visual cortex, and the evidence for and against prefrontal neurons receiving DA input themselves representing reward value. The evidence suggests that prefrontal DA contributes to both representations of target value and to the behavioral and neural signatures of attention, although further studies will be needed to determine if DA's roles in these processes are dissociable.
DA release is associated with reward cues or expectation (Schultz, 2002). The involvement of DA signaling in both attention and reward raises the question of how these mechanisms overlap or diverge. Indeed, many behavioral tasks manipulate attention or reward in such a way that these two properties cannot truly be distinguished from one another (Maunsell, 2004). Consider a typical study seeking to identify a neural correlate of reward size (Figure 2A). One stimulus is placed within the neuron's response field (RF), a second outside it; the relative size of the reward associated with the two locations is then varied, either in blocks or from trial to trial based on some cue. A neuron that displays greater activity when the high-reward stimulus appears in its RF is typically reported as encoding reward expectation or value. The same logic applies to reward probability, although this manipulation must be done in blocks. Now consider a typical “attention” task: multiple stimuli appear onscreen, and one of them must be monitored for a behavioral response—again, the selected location may be held constant over a block of trials or varied from trial to trial based on a cue. Sometimes the task occurs only or more frequently at the cued stimulus—in other cases the animal is explicitly trained not to respond to changes at the uncued location; in either version of the attention task, reward is exclusively or predominantly associated with the stimulus at the attended location, and yet in these studies a difference in firing rate is attributed to the locus of attention rather than an expected reward. Conversely, the “reward” activity we described in the previous experimental design could be attributed to attentional modulation, given that on a behavioral level the expectation of a reward attracts attention (Posner, 1980). Importantly, many areas reflecting attentional modulation in their neural activity also exhibit reward-dependent activity (Figure 2B). A recent study of the effects of reward on activity in primary visual cortex showed that the strength of reward-size modulation across cells was strongly correlated with their modulation by attention, suggesting that the neural sources of these effects may be overlapping, if not identical (Stănişor et al., 2013, discussed further in the next section). Since this critique originally appeared a decade ago, many studies that experimentally manipulate reward values acknowledge the potential confound, or even explicitly attribute their findings to attention (Kennerley and Wallis, 2009), but few attempt to dissociate the two processes. Even studies using paradigms designed to differentiate representations of reward from general behavioral salience (Leathers and Olson, 2012) have proven controversial (Leathers and Olson, 2013; Newsome et al., 2013), or generated results that suggest reward cues can drive attentional allocation in ways that prove detrimental to task performance (Peck et al., 2009).
Figure 2. Interactions between attention and reward. (A) A schematic illustration of typical tasks used to study reward and attention, and how the differences in potential reward and neural activity are similar between the two paradigms. Consider two studies conducted in V1 (Stănişor et al., 2013 and McAdams and Reid, 2005). To study the effect of reward size in the Stănişor task (schematically illustrated in the top panel), two potential targets appear, with colors indicating different reward values. Neural activity recorded at this point in the task reflects the relative value of the target in the RF (higher activity when the RF target offered a greater reward than the non-RF target); a subsequent cue instructs the monkey which target to saccade to. In the McAdams and Reid attentional paradigm (bottom panel), a cue indicates which of two stimuli should be monitored for a change, which instructs an eye movement response to a separate location. Changes at the uncued location must be ignored, and will never lead to rewards. Neural activity is higher when the stimulus in the RF is cued. In both cases higher expected reward value for the stimulus in the RF is associated with greater neural activity. (B) An overview of brain areas in which neural activity reflecting both attentional modulation and reward value has been reported. Only a single study is cited for each area; reward studies are in gray, attention studies in black. Dotted outlines represent structures not located on the cortical surface, either within sulci or deeper within the brain. Abbreviations: PMC, premotor cortex; vlPFC, ventrolateral prefrontal cortex; dlPFC, dorsolateral prefrontal cortex; SC, superior colliculus; BG, basal ganglia; LIP, lateral intraparietal area; SEF, supplementary eye field; ACC, anterior cingulate cortex; FEF, frontal eye field.
Although many studies have examined the effect of DA-ergic agents on prefrontal activity, and prefrontal activity has long been believed to modulate responses in visual cortex during attention and working memory, until recently no one had directly examined the effect of locally manipulating prefrontal DA signaling on visual responses in other cortical areas. Noudoost and Moore (2011b) examined the long-range effects of altering prefrontal DA signaling on visual responses in extrastriate area V4. V4, like much of visual cortex, receives direct projections from the Frontal Eye Field (FEF) part of the PFC, an area strongly implicated in controlling spatial attention (Moore and Fallah, 2004; Armstrong et al., 2009; Clark et al., 2012), and it is believed that these projections may be the source of the changes in activity observed in V4 during the deployment of covert attention (Moore and Armstrong, 2003; Awh et al., 2006; Noudoost et al., 2010, 2014; Squire et al., 2013; Clark et al., 2014). Noudoost and Moore examined the effects of manipulating either D1Rs or D2Rs on V4 visual responses during a passive fixation task, and their effect on saccadic target selection in a free-choice task (Noudoost and Moore, 2011a,b). While both D1R and D2R manipulations increased the monkey's tendency to choose the saccade target in the affected region of space, biasing saccadic target selection, only D1Rs had an impact on V4 visual responses. Local injection of a D1R antagonist into the FEF enhanced the strength of visual signals in V4: response magnitude increased, orientation selectivity was enhanced, and trial-to-trial variability decreased (Figure 3). All of these changes are also observed in V4 when covert spatial attention is directed to the V4 neuron's RF (Moran and Desimone, 1985; McAdams and Maunsell, 2000; Reynolds et al., 2000; Mitchell et al., 2007). The reason for the differing effects of FEF D1R and D2R manipulations on V4 activity, but common effects on target selection, may lie in the patterns of receptor expression within the FEF. D1Rs are expressed in both the supragranular layers, which project to V4, and infragranular layers, which contain neurons projecting to motor areas such as the superior colliculus. In contrast, D2Rs are primarily expressed in the infragranular layers. This pattern of expression could account for both receptors influencing target selection, while only D1Rs alter V4 responses (Noudoost and Moore, 2011c).
Figure 3. The effects of PFC DA on visual cortical activity. Manipulating D1R-mediated FEF activity enhances visual representations in area V4. Noudoost and Moore (2011b) infused a D1R antagonist into the FEF while recording from V4 neurons with RFs either overlapping or not overlapping the area of space represented at the site of drug infusion; the visual responses of the same V4 neurons were recorded before and after infusion of drugs into the FEF. FEF RF center was estimated based on the endpoints of microstimulation-evoked saccades. FEF D1R manipulation caused an increase in orientation selectivity, increase in response magnitude, and decrease in response variability at overlapping V4 sites (orange bars); no effect was seen for non-overlapping V4 sites (green), or saline infusions (gray). These changes in V4 responses with FEF D1R manipulation mimic those seen during covert attention. *p <0.05.
Neurophysiological experiments in V1 have provided a direct comparison of the effects of attention and reward on visual cortical responses (Stănişor et al., 2013). Visual responses were shown to be modulated by the relative reward value of the RF stimulus; moreover, the magnitude of this modulation was strongly correlated with the strength of atttentional modulation during a later time window in the same task, and the onset latencies of the two effects were indistinguishable. Like attentional modulation, the neural effects of reward value were dramatically enhanced in the presence of a second stimulus. Human fMRI experiments have also demonstrated a D1R-dependent reward modulation of visual cortical activity (Arsenault et al., 2013). These effects of reward on visual cortex may not be attributable to the PFC—they could result from a bottom-up influence of DA-ergic changes in LGN signaling (Zhao et al., 2002), or via direct DA release from midbrain projections (Lewis et al., 1987). However, several aspects of the findings argue in favor of a prefrontal origin to these effects: the strong correlation with attention in the Stănişor case, the presence of this modulation even in trials without a visual stimulus in the Arsenault paper, the lower density of DA-ergic projections to visual cortex (Berger et al., 1988), and the proven ability of DA-ergic PFC activity to modulate representations in visual cortex (Noudoost and Moore, 2011b), make PFC a likely source of this reward-induced modulation.
Multiple studies have looked for representations of reward value in PFC. Leon and Shadlen (1999) examined the effect of centrally cued reward size on FEF and dlPFC responses during a memory-guided saccade task. They found an effect on reward size on responses in dlPFC, but not FEF; this dlPFC reward-size dependent activity continued throughout the delay period. Interestingly, the presence of reward-size information in dlPFC responses was dependent on the simultaneous maintenance of a spatial memory: in a variant of the task in which the reward cue appeared before the spatial cue, no reward size information was present until after the subsequent spatial cue appeared. However, findings by Ding and Hikosaka suggest that the FEF will also represent reward size information under certain conditions: specifically when the reward is tied to a particular location (Ding and Hikosaka, 2006). Using an asymmetrically rewarded memory-guided saccade paradigm, in which the relative value of the two target locations varied between blocks of trials, they found that about 1/3 of FEF neurons were selective for the location of the larger reward during the cue period. This may reflect the stronger retinotopic organization of the FEF in comparison to dlPFC (Suzuki and Azuma, 1983; Bruce et al., 1985; Funahashi et al., 1989). Interestingly, this reward modulation did not persist into the delay period—precisely the time in which the dlPFC representation of reward was observed by Leon and Shadlen, and the period whose activity predicts an FEF neuron's ability to distinguish targets from distractors (Armstrong et al., 2009). This pattern of reward modulation contrasts starkly with the response properties of the DA neurons projecting to PFC, again emphasizing the role of DA-ergic activity as a modulator rather than a simple driver or inhibitor of prefrontal activity.
Spatially-specific representation of reward values in the FEF and rich DA-ergic inputs to this area raise the hypothesis that FEF DA could serve as a mechanism for reward-dependent selection of visual targets. Indeed, Soltani et al. pursued this idea and tested the behavioral effects of perturbing DA-ergic activity within the FEF of monkeys performing a saccadic choice task and simulated the effects using a biologically-plausible cortical network (Soltani et al., 2013). They found that manipulation of FEF activity either by blocking D1Rs or by stimulating D2Rs increased the tendency to choose targets in the RF of the affected site. These effects of DA manipulation could be described purely in terms of motor biases; however, DA manipulation also altered the influence of choice history, and hence reward history, on subsequent target choices. The effects of choice history were also differently altered by the two DA receptors: D1R manipulation decreased the tendency to repeat choices on subsequent trials, whereas the D2R manipulation increased that tendency. This altered impact of choice history indicates that manipulating FEF DA influences the value of saccadic targets based on prior reward experience. The network simulation results suggest that D1Rs influence target selection mainly through their effects on the strength of inputs to the FEF and on recurrent connectivity, whereas D2Rs influence the excitability of FEF output neurons. Altogether, these results reveal dissociable DA-ergic mechanisms influencing target selection in which D1Rs and D2Rs differentially alter saccadic target selection by virtue of their effects in different cortical layers (Noudoost and Moore, 2011c). The network model revealed that DA-ergic modulation of the afferents to the FEF could alter reward-dependent choice. Based on this model one might predict, for example, that after blocking D1Rs within the FEF, the form and time constant of reward integration would be altered such that the impact of previous rewards on current choices could be increased or decreased.
DA is a neuromodulator known to play a crucial role in reward-dependent behavior. Prefrontal neurons, which receive rich DA-ergic input from areas representing expected rewards, play a pivotal role in top-down modulation of cortical activity. Prefrontal DA (Noudoost and Moore, 2011b) and reward (Stănişor et al., 2013) can both modulate representation of targets within visual areas, mimicking some of the signatures of top-down visual attention. The questions of whether manipulation of PFC DA changes reward-dependent behavior, the degree to which signatures of attention and reward expectation in visual areas are dissociable, and whether DA-mediated PFC activity is the link for established behavioral interactions between attention and reward, remain to be answered.
DA is not the only neuromodulator whose levels are critical for prefrontal function during cognitive tasks: NE also appears to be crucial to normal PFC activity. The PFC receives NE input from the locus coeruleus (Porrino and Goldman-Rakic, 1982; Levitt et al., 1984). The tonic firing of locus coeruleus NE neurons reflects arousal state, with low rates during slow wave sleep or drowsiness, moderate rates during waking, and high rates in response to acute stress. They also display phasic firing in response to behaviorally relevant stimuli during normal waking, but this phasic firing can extend to irrelevant distractors during fatigue or stress (Aston-Jones et al., 1999). Like the DA projections described above, NE inputs to PFC show a bilaminar targeting pattern (Morrison et al., 1982; Levitt et al., 1984; Lewis and Morrison, 1989). NE binds to high affinity alpha-2 adrenoreceptors, and to lower affinity alpha-1 and beta receptors (Molinoff, 1984). Alpha-2 receptors are found on dendritic spines in the superficial layers of PFC; although they can function both pre- and post-synaptically, their postsynaptic activity appears to underlie the benefits of alpha-2A agonists on working memory and other cognitive tasks (Arnsten and Cai, 1993; Wang et al., 2007). Like dopamine, there appears to be an optimal, intermediate level of NE signaling in PFC. The higher levels of NE associated with stress may impair PFC function through actions at the lower affinity alpha-1 receptors in the superficial layers (Arnsten et al., 1999; Birnbaum et al., 1999; Mao et al., 1999), and beta receptors localized on dendritic spines in the intermediate layers (Aoki et al., 1998; Ramos et al., 2005). Intracellularly, the actions of D1 (Vijayraghavan et al., 2007), alpha-2A (Wang et al., 2007), and beta1 receptors may converge on the cAMP signaling pathway (Gamo and Arnsten, 2011). Studies of the contributions of prefrontal NE to cognitive function led to the development of alpha-2A agonist guanfacine as a treatment for ADHD (Hunt et al., 1995; Taylor and Russo, 2001; Biederman et al., 2008; Gamo and Arnsten, 2011).
One of the reasons for focusing on prefrontal catecholamines—as opposed to, for example, prefrontal N-methyl-D-aspartate receptor (NMDA) or gamma-Aminobutyric acid (GABA) signaling, the proper functioning of which are certainly also vital to working memory and other prefrontal functions—is that these systems appear to be implicated in multiple disorders involving prefrontal dysfunction. Here we briefly canvas the literature linking prefrontal catecholamines to Parkinson's, schizophrenia, and ADHD, before turning to studies of their contribution to normal cognition in humans.
The loss of DA neurons in Parkinson's disease produces cognitive deficits in addition to the more outwardly apparent motor symptoms (Lees and Smith, 1983; Taylor et al., 1986; Morris et al., 1988; Owen et al., 1992, 1993; Postle et al., 1997). It seems likely that at least some of these cognitive effects are directly due to a loss of DA-ergic input to PFC, and can thus provide insight into the normal contribution of DA to these functions. Accordingly, multiple studies use the withdrawal of L-dopa or other dopaminergic medications in Parkinson's patients to evaluate the effect of reduced DA signaling on various cognitive tasks (Table 2). Results generally indicate impaired spatial working memory in the absence of sufficient DA (Lange et al., 1992; Mattay et al., 2002). They also confirm findings suggesting that increased prefrontal activity, measured with fMRI or blood flow, may reflect less efficient processing in these tasks, showing greater dlPFC activation in the hypo-DA-ergic state (Cools et al., 2002), and a correlation between increases in PFC activity and error rates on the working memory task (Mattay et al., 2002). Interestingly, in early Parkinson's disease patients DA loss is more pronounced in specific anatomical regions, with dramatic DA depletion in the putamen and dorsal caudate, while DA levels in the ventral striatum are relatively spared (Kish et al., 1988; Agid et al., 1993). These regions of the basal ganglia also differ in their prefrontal connectivity, the dorsal regions forming a circuit with dlPFC while the ventral striatum is connected to orbitofrontal cortex (Alexander et al., 1986). The consequences of this segregation and differential susceptibility to Parkinson's-induced DA losses can be seen in the effect of medication withdrawal on two tasks selected to differentially engage the dlPFC and the orbitofrontal cortex (Dias et al., 1996; Cools et al., 2001). Performance on task-set switching, which is thought to depend on dlPFC and parietal circuits, was impaired following medication withdrawal; in contrast, patients' performance on a reversal learning which depends upon orbitofrontal cortex actually improved when off of medication. This reinforces the notion of an optimal level of DA signaling: when disease-induced DA depletion affects circuits to different degrees, medication that increases DA globally and optimizes the level in one circuit may produce above-optimal levels in other areas, with corresponding behavioral deficits.
Table 2. Studies examining the contribution of prefrontal catecholamines to the behavioral and neural correlates of working memory in human subjects.
DA is also implicated in the etiology of schizophrenia (origins of this idea reviewed in Baumeister and Francis, 2002). Although the “dopamine hypothesis” of schizophrenia has existed for decades, development of theoretical frameworks to link the pharmacological and neurobiological findings to the phenomenology of the disorder is ongoing, for example the aberrant salience theory of psychosis (Kapur, 2003; Kapur et al., 2005). Clinically effective antipsychotics appear to primarily target the D2 receptor (Seeman and Lee, 1975), and hyperstimulation of subcortical D2Rs is still considered a likely cause of the positive symptoms of the disorder; in contrast, a cortical, and specifically prefrontal, DA deficit may contribute to the cognitive symptoms (Abi-Dargham, 2004; Guillin et al., 2007). Associations have been found between schizophrenia and genetic variations in DA receptors (Glatt et al., 2003; Jönsson et al., 2004), and the COMT gene discussed below (Egan et al., 2001, reviewed in Harrison and Weinberger, 2005). COMT genotype has also been associated with the ability of antipsychotics to improve working memory performance (Weickert et al., 2004). (However, DA is not the only neurochemical system genetically linked to schizophrenia—see Mowry and Gratten, 2013). Schizophrenic patients display deficits in working memory tasks (Park and Holzman, 1992; Fleming et al., 1995; Morice and Delahunty, 1996; Keefe et al., 1997), and neurocognitive deficits have been shown to predict clinical outcomes (Green, 1996). Patients also show abnormal, typically excessive, PFC activation during these tasks (Manoach et al., 1999; Callicott, 2000; Barch et al., 2001; Perlstein et al., 2001). The laminar distribution of DA-ergic innervation of PFC appears altered (Akil et al., 1999), and there is some evidence for changes in prefrontal D1R density (Abi-Dargham et al., 2002, 2012)—although the absence of such effects in postmortem studies may indicate that expression levels are normalized by medication (Laruelle et al., 1990; Meador-Woodruff et al., 1997).
ADHD is one of the most common psychiatric disorders, affecting ~3–7% of the US population. Clinically, ADHD is characterized by inattention, impulsivity, and hyperactivity (American Psychiatric Association, 2013). In laboratory settings, ADHD patients' inattention and impulsivity lead to deficits in tasks measuring spatial attention (Friedman-Hill et al., 2010), working memory (Alderson et al., 2013), and oculomotor response inhibition (Rommelse et al., 2008; Goto et al., 2010). These cognitive tasks have long been linked to prefrontal function (D'Esposito and Postle, 1999; Miller, 2000). Given this link, it is unsurprising that patients with ADHD show structural and functional differences in prefrontal size, projection strength, resting connectivity, and activity during cognitive tasks (Seidman et al., 2005; Arnsten, 2006; Kieling et al., 2008). Several lines of evidence more specifically implicate prefrontal catecholamine function as an underlying cause and potential therapeutic target. Genetic linkage studies confirm potential contributions of both DA and NE to the disorder (reviewed in Gizer et al., 2009). Associated genes include DA receptors D1, D4, and D5 (Sunohara et al., 2000; Tahir et al., 2000; Kustanovich et al., 2004; Bobb et al., 2005; Mill et al., 2006; Wu et al., 2012), the DA transporter (DAT) (Durston et al., 2005; Mill et al., 2006), the NE transporter, the NE alpha-2A receptor (Xu et al., 2001; Roman et al., 2003), and DA beta-hydroxylase, an enzyme which coverts DA to NE (Daly et al., 1999; Roman et al., 2002; Kopecková et al., 2006). Many of the medications currently prescribed to treat ADHD alter catecholamine transmission (Arnsten, 2009). Stimulants such as amphetamine, lisdexamphetamine, and methylphenidrate block both DA and NE transporters. In rats, methylphenidrate (Ritalin®) has been shown to increase DA and NE release, particularly in the PFC (Berridge et al., 2006), and improve performance on a delayed alternation task used to assess prefrontal function in rodents. These performance benefits were blocked by co-administration of either an alpha-2A or D1R antagonist, neither of which impaired performance in isolation, suggesting that both DA-ergic and noradrenergic signaling contribute to the methylphenidate's cognitive effects (Arnsten and Dudley, 2005). Atomoxetine blocks the NE transporter, producing increases in both NE and DA in the PFC (Bymaster et al., 2002), while guanfacine is an alpha-2A receptor agonist.
Numerous studies have examined dopamine's contribution to cognitive performance by administering various DA agonists or antagonists to healthy volunteers (see Table 2). Unfortunately there is no D1R-selective drug available for use in humans; D1R effects have had to be inferred by comparing the effects of mixed agonists to those of D2R selective agents. A number of studies have reported the ability of DA-ergic drugs to alter performance on spatial working memory or delayed response tasks, although the studies' findings differ with respect to the relative contribution of D1Rs and D2Rs (Luciana et al., 1998; Müller et al., 1998) and whether the effects are limited to spatial working memory or apply to a broader range of memory and attention tasks (Luciana et al., 1998; Kimberg and D'Esposito, 2003). Some of this variability is probably attributable to an interaction between drug action and subjects' baseline DA-ergic tone (see discussion of the “inverted-U” action of DA above). Indeed, the action of these drugs in healthy volunteers has been shown to depend on their baseline working memory capacity (Kimberg and D'Esposito, 2003; Mattay et al., 2003). It may even depend on the subject's recent behavior: training on a working memory task, half an hour a day for 5 weeks, is sufficient to improve capacity measurements and decrease prefrontal D1R binding potential, suggesting DA receptor expression may be modulated by the demands of habitual tasks (McNab et al., 2009).
Genetic polymorphisms related to DA processing or signaling have also been linked to cognitive phenotypes, in both neurotypical and patient populations. One of the most extensively studied is a polymorphism in the catechol-O-methyltransferase (COMT) gene. COMT is an enzyme that breaks down DA following synaptic release; its activity is especially important for determining DA levels in the PFC, which has comparatively few DATs (Gogos et al., 1998). A common polymorphism producing a valine-to-methionine substitution alters enzyme activity: the Val-allele has higher enzymatic activity, presumably reducing prefrontal DA levels, while the Met-allele has lower activity, theoretically resulting in higher basal DA (Chen et al., 2004); however these presumed effects of COMT genotype on basal PFC DA levels have never been directly verified in humans. It should also be noted that the effects of many DA-related polymorphisms on working memory may be mediated by the striatum in addition to the PFC (Cools et al., 2008). Met-allele homozygotes show lower prefrontal activity during an n-back working memory task than heterozygotes, who in turn have lower prefrontal activation than Val-allele homozygotes (Egan et al., 2001). Amphetamine, which like other stimulants causes release of DA and NE in PFC (Kuczenski and Segal, 1992; Moghaddam et al., 1993; Berridge et al., 2006; Narendran et al., 2014), reduces prefrontal activity during the 3-back task in Val homozygotes, while increasing prefrontal activity and impairing performance for Met homozygotes on the same task (Mattay et al., 2003). These results are consistent with an inverted-U relationship between prefrontal DA levels and function, where Val homozygotes have slightly sub-optimal basal DA levels due to their increased enzymatic breakdown of DA, while Met homozygotes have higher basal DA levels, such that the additional DA release following amphetamine administration is detrimental to PFC function. Interestingly, Val-allele homozygotes show more perseverative errors on the Wisconsin card-sorting task, but no overall differences in working memory performance or other cognitive measures (Egan et al., 2001; Mattay et al., 2003; Zilles et al., 2012); this absence of baseline differences in working memory based on COMT genotype suggests compensatory changes in other aspects of DA signaling (although see Goldberg et al., 2003). The effects of COMT genotype on prefrontal activity during working memory have been shown to interact additively with another polymorphism, a variable number tandem repeat polymorphisms identified in the 3' untranslated region of the DAT gene (Bertolino et al., 2006; Caldú et al., 2007).
Performance in attention and working memory tasks is impaired in ADHD, Parkinson's disease, and schizophrenia, as well as under stress or in normal aging. Considering the evidence for a contribution of prefrontal catecholamines to these cognitive functions, imbalance in the prefrontal level of these neuromodulators has long been a suspected cause of the cognitive impairments observed in these disorders. More recently, genetic association studies have demonstrated links between prefrontal catecholamines and the etiology of these diseases, as well as how patients respond to treatment. Despite numerous studies examining the link between prefrontal DA or NE and cognitive function in these disorders, we are still far from treatments that fully restore cognitive function. This gap may be partly due to individual variation in the underlying pathology, but also partly as a result of our own incomplete understanding of the neural mechanisms underlying normal cognitive function. Even in cases where the mechanisms are well understood, clinically we lack the means to target specific anatomical or chemical subsets of neurons. However, basic research on the mechanisms of prefrontal function has produced some therapeutic advances, e.g., the introduction of guanfacine for the treatment of ADHD patients, and a more complete understanding of how prefrontal catecholamine signaling underlies cognition may produce further clinical applications.
The link between prefrontal catecholamines and cognitive deficits in multiple neurological disorders makes understanding their role in prefrontal function particularly critical. While much progress has been made in elucidating the role of prefrontal catecholamines' role in cognitive function, crucial questions still remain. Is the effect of prefrontal DA mediated entirely via reward expectation, or do basal PFC DA levels modulate working memory and attention performance in a manner dissociable from upcoming rewards? Do PFC DA levels fluctuate significantly over the course of attention and working memory tasks, and do these fast changes in DA signaling contribute to behavioral performance? Although the true “neural mechanism” of working memory maintenance or covert attentional deployment is the pattern of task-related neural activity, driven by spatially tuned glutamatergic and GABA-ergic responses, these population dynamics are enabled by appropriate DA and NE “tone” within these prefrontal circuits; whether more temporally or spatially localized changes in catecholamine signaling also contribute to task performance (Chadderdon and Sporns, 2006) remains uncertain. More reliable, temporally precise and continuous measures of local DA levels would be an important first step in addressing these questions.
The authors declare that the research was conducted in the absence of any commercial or financial relationships that could be construed as a potential conflict of interest.
Aalto, S., Brück, A., Laine, M., Någren, K., and Rinne, J. O. (2005). Frontal and temporal dopamine release during working memory and attention tasks in healthy humans: a positron emission tomography study using the high-affinity dopamine D2 receptor ligand [11C]FLB 457. J. Neurosci. 25, 2471–2477. doi: 10.1523/JNEUROSCI.2097-04.2005
Abercrombie, E. D., Keefe, K. A., DiFrischia, D. S., and Zigmond, M. J. (1989). Differential effect of stress on in vivo dopamine release in striatum, nucleus accumbens, and medial frontal cortex. J. Neurochem. 52, 1655–1658. doi: 10.1111/j.1471-4159.1989.tb09224.x
Abi-Dargham, A. (2004). Do we still believe in the dopamine hypothesis? New data bring new evidence. Int. J. Neuropsychopharmacol. 7(Suppl. 1), S1–S5. doi: 10.1017/S1461145704004110
Abi-Dargham, A., Mawlawi, O., Lombardo, I., Gil, R., Martinez, D., Huang, Y., et al. (2002). Prefrontal dopamine D1 receptors and working memory in schizophrenia. J. Neurosci. 22, 3708–3719.
Abi-Dargham, A., Xu, X., Thompson, J. L., Gil, R., Kegeles, L. S., Urban, N., et al. (2012). Increased prefrontal cortical D1 receptors in drug naive patients with schizophrenia: a PET study with [11C]NNC112. J. Psychopharmacol. 26, 794–805. doi: 10.1177/0269881111409265
Agid, Y., Ruberg, M., Javoy-Agid, F., Hirsch, E., Raisman-Vozari, R., Vyas, S., et al. (1993). Are dopaminergic neurons selectively vulnerable to Parkinson's disease? Adv. Neurol. 60, 148–164.
Akil, M., Pierri, J. N., Whitehead, R. E., Edgar, C. L., Mohila, C., Sampson, A. R., et al. (1999). Lamina-specific alterations in the dopamine innervation of the prefrontal cortex in schizophrenic subjects. Am. J. Psychiatry 156, 1580–1589.
Alderson, R. M., Hudec, K. L., Patros, C. H. G., and Kasper, L. J. (2013). Working memory deficits in adults with attention-deficit/hyperactivity disorder (ADHD): an examination of central executive and storage/rehearsal processes. J. Abnorm. Psychol. 122, 532–541. doi: 10.1037/a0031742
Alexander, G. E., DeLong, M. R., and Strick, P. L. (1986). Parallel organization of functionally segregated circuits linking basal ganglia and cortex. Annu. Rev. Neurosci. 9, 357–381. doi: 10.1146/annurev.ne.09.030186.002041
Alexander, G. E., and Goldman, P. S. (1978). Functional development of the dorsolateral prefrontal cortex: an analysis utlizing reversible cryogenic depression. Brain Res. 143, 233–249. doi: 10.1016/0006-8993(78)90566-8
American Psychiatric Association. (2013). Diagnostic and Statistical Manual of Mental Disorders, 5th Edn. Arlington, VA: American Psychiatric Publishing.
Aoki, C., Venkatesan, C., and Kurose, H. (1998). Noradrenergic modulation of the prefrontal cortex as revealed by electron microscopic immunocytochemistry. Adv. Pharmacol. 42, 777–780. doi: 10.1016/S1054-3589(08)60862-5
Armstrong, K. M., Chang, M. H., and Moore, T. (2009). Selection and maintenance of spatial information by frontal eye field neurons. J. Neurosci. 29, 15621–15629. doi: 10.1523/JNEUROSCI.4465-09.2009
Arnsten, A. F. (2006). Fundamentals of attention-deficit/hyperactivity disorder: circuits and pathways. J. Clin. Psychiatry 67(Suppl 8), 7–12.
Arnsten, A. F. (2009). The emerging neurobiology of attention deficit hyperactivity disorder: the key role of the prefrontal association cortex. J. Pediatr. 154, I–S43. doi: 10.1016/j.jpeds.2009.01.018
Arnsten, A. F., and Cai, J. X. (1993). Postsynaptic alpha-2 receptor stimulation improves memory in aged monkeys: indirect effects of yohimbine versus direct effects of clonidine. Neurobiol. Aging 14, 597–603. doi: 10.1016/0197-4580(93)90044-C
Arnsten, A. F., Cai, J. X., and Goldman-Rakic, P. S. (1988). The alpha-2 adrenergic agonist guanfacine improves memory in aged monkeys without sedative or hypotensive side effects: evidence for alpha-2 receptor subtypes. J. Neurosci. 8, 4287–4298.
Arnsten, A. F., Cai, J. X., Murphy, B. L., and Goldman-Rakic, P. S. (1994). Dopamine D1 receptor mechanisms in the cognitive performance of young adult and aged monkeys. Psychopharmacology 116, 143–151. doi: 10.1007/BF02245056
Arnsten, A. F., Cai, J. X., Steere, J. C., and Goldman-Rakic, P. S. (1995). Dopamine D2 receptor mechanisms contribute to age-related cognitive decline: the effects of quinpirole on memory and motor performance in monkeys. J. Neurosci. 15(5 Pt 1), 3429–3439.
Arnsten, A. F., and Contant, T. A. (1992). Alpha-2 adrenergic agonists decrease distractibility in aged monkeys performing the delayed response task. Psychopharmacology 108, 159–169. doi: 10.1007/BF02245302
Arnsten, A. F., and Dudley, A. (2005). Methylphenidate improves prefrontal cortical cognitive function through alpha2 adrenoceptor and dopamine D1 receptor actions: relevance to therapeutic effects in Attention Deficit Hyperactivity Disorder. Behav. Brain Func. 1, 2. doi: 10.1186/1744-9081-1-2
Arnsten, A. F., and Goldman-Rakic, P. S. (1985). Alpha 2-adrenergic mechanisms in prefrontal cortex associated with cognitive decline in aged nonhuman primates. Science 230, 1273–1276. doi: 10.1126/science.2999977
Arnsten, A. F., Mathew, R., Ubriani, R., Taylor, J. R., and Li, B. M. (1999). Alpha-1 noradrenergic receptor stimulation impairs prefrontal cortical cognitive function. Biol. Psychiatry 45, 26–31. doi: 10.1016/S0006-3223(98)00296-0
Arsenault, J. T., Nelissen, K., Jarraya, B., and Vanduffel, W. (2013). Dopaminergic reward signals selectively decrease fMRI activity in primate visual cortex. Neuron 77, 1174–1186. doi: 10.1016/j.neuron.2013.01.008
Aston-Jones, G., Rajkowski, J., and Cohen, J. D. (1999). Role of locus coeruleus in attention and behavioral flexibility. Biol. Psychiatry 46, 1309–1320. doi: 10.1016/S0006-3223(99)00140-7
Avery, M. C., Dutt, N., and Krichmar, J. L. (2013). A large-scale neural network model of the influence of neuromodulatory levels on working memory and behavior. Front. Comput. Neurosci. 7:133. doi: 10.3389/fncom.2013.00133
Avery, R. A., Franowicz, J. S., Studholme, C., van Dyck, C. H., and Arnsten, A. F. (2000). The alpha-2A-adrenoceptor agonist, guanfacine, increases regional cerebral blood flow in dorsolateral prefrontal cortex of monkeys performing a spatial working memory task. Neuropsychopharmacology 23, 240–249. doi: 10.1016/S0893-133X(00)00111-1
Awh, E., Armstrong, K. M., and Moore, T. (2006). Visual and oculomotor selection: links, causes and implications for spatial attention. Trends Cogn. Sci. 10, 124–130. doi: 10.1016/j.tics.2006.01.001
Barch, D. M., Carter, C. S., Braver, T. S., Sabb, F. W., MacDonald, A., Noll, D. C., et al. (2001). Selective deficits in prefrontal cortex function in medication-naive patients with schizophrenia. Arch. Gen. Psychiatry 58, 280–288. doi: 10.1001/archpsyc.58.3.280
Baumeister, A. A., and Francis, J. L. (2002). Historical development of the dopamine hypothesis of schizophrenia. J. Hist. Neurosci. 11, 265–277. doi: 10.1076/jhin.11.3.265.10391
Berger, B., Gaspar, P., and Verney, C. (1991). Dopaminergic innervation of the cerebral cortex: unexpected differences between rodents and primates. Trends Neurosci. 14, 21–27. doi: 10.1016/0166-2236(91)90179-X
Berger, B., Trottier, S., Verney, C., Gaspar, P., and Alvarez, C. (1988). Regional and laminar distribution of the dopamine and serotonin innervation in the macaque cerebral cortex: a radioautographic study. J. Comp. Neurol. 273, 99–119. doi: 10.1002/cne.902730109
Berridge, C. W., Devilbiss, D. M., Andrzejewski, M. E., Arnsten, A. F., Kelley, A. E., Schmeichel, B., et al. (2006). Methylphenidate preferentially increases catecholamine neurotransmission within the prefrontal cortex at low doses that enhance cognitive function. Biol. Psychiatry 60, 1111–1120. doi: 10.1016/j.biopsych.2006.04.022
Bertolino, A., Blasi, G., Latorre, V., Rubino, V., Rampino, A., Sinibaldi, L., et al. (2006). Additive effects of genetic variation in dopamine regulating genes on working memory cortical activity in human brain. J. Neurosci. 26, 3918–3922. doi: 10.1523/JNEUROSCI.4975-05.2006
Biederman, J., Melmed, R. D., Patel, A., McBurnett, K., Donahue, J., and Lyne, A. (2008). Long-term, open-label extension study of guanfacine extended release in children and adolescents with ADHD. CNS Spectr. 13, 1047–1055.
Birnbaum, S., Gobeske, K. T., Auerbach, J., Taylor, J. R., and Arnsten, A. F. (1999). A role for norepinephrine in stress-induced cognitive deficits: alpha-1-adrenoceptor mediation in the prefrontal cortex. Biol. Psychiatry 46, 1266–1274. doi: 10.1016/S0006-3223(99)00138-9
Bobb, A. J., Addington, A. M., Sidransky, E., Gornick, M. C., Lerch, J. P., Greenstein, D. K., et al. (2005). Support for association between ADHD and two candidate genes: NET1 and DRD1. Am. J. Med. Genet. B Neuropsychiatr. Genet. 134B, 67–72. doi: 10.1002/ajmg.b.30142
Bon, L., and Lucchetti, C. (1997). Attention-related neurons in the supplementary eye field of the macaque monkey. Exp. Brain Res. 113, 180–185. doi: 10.1007/BF02454156
Brozoski, T. J., Brown, R. M., Rosvold, H. E., and Goldman, P. S. (1979). Cognitive deficit caused by regional depletion of dopamine in prefrontal cortex of rhesus monkey. Science 205, 929–932. doi: 10.1126/science.112679
Bruce, C. J., Goldberg, M. E., Bushnell, M. C., and Stanton, G. B. (1985). Primate frontal eye fields. II. Physiological and anatomical correlates of electrically evoked eye movements. J. Neurophysiol. 54, 714–734.
Brunel, N., and Wang, X. J. (2001). Effects of neuromodulation in a cortical network model of object working memory dominated by recurrent inhibition. J. Comput. Neurosci. 11, 63–85. doi: 10.1023/A:1011204814320
Buschman, T. J., and Miller, E. K. (2007). Top-down versus bottom-up control of attention in the prefrontal and posterior parietal cortices. Science 315, 1860–1862. doi: 10.1126/science.1138071
Bymaster, F. P., Katner, J. S., Nelson, D. L., Hemrick-Luecke, S. K., Threlkeld, P. G., Heiligenstein, J. H., et al. (2002). Atomoxetine increases extracellular levels of norepinephrine and dopamine in prefrontal cortex of rat: a potential mechanism for efficacy in attention deficit/hyperactivity disorder. Neuropsychopharmacology 27, 699–711. doi: 10.1016/S0893-133X(02)00346-9
Cai, J. X., and Arnsten, A. F. (1997). Dose-dependent effects of the dopamine D1 receptor agonists A77636 or SKF81297 on spatial working memory in aged monkeys. J. Pharmacol. Exp. Ther. 283, 183–189.
Cai, J. X., Ma, Y. Y., Xu, L., and Hu, X. T. (1993). Reserpine impairs spatial working memory performance in monkeys: reversal by the alpha 2-adrenergic agonist clonidine. Brain Res. 614, 191–196. doi: 10.1016/0006-8993(93)91034-P
Caldú, X., Vendrell, P., Bartrés-Faz, D., Clemente, I., Bargalló, N., Jurado, M. A., et al. (2007). Impact of the COMT Val108/158 Met and DAT genotypes on prefrontal function in healthy subjects. Neuroimage 37, 1437–1444. doi: 10.1016/j.neuroimage.2007.06.021
Callicott, J. H. (2000). Physiological dysfunction of the dorsolateral prefrontal cortex in schizophrenia revisited. Cereb. Cortex 10, 1078–1092. doi: 10.1093/cercor/10.11.1078
Castner, S. A., and Williams, G. V. (2007). Tuning the engine of cognition: a focus on NMDA/D1 receptor interactions in prefrontal cortex. Brain Cogn. 63, 94–122. doi: 10.1016/j.bandc.2006.11.002
Chadderdon, G. L., and Sporns, O. (2006). A large-scale neurocomputational model of task-oriented behavior selection and working memory in prefrontal cortex. J. Cogn. Neurosci. 18, 242–257. doi: 10.1162/jocn.2006.18.2.242
Chao, L. L., and Knight, R. T. (1998). Contribution of human prefrontal cortex to delay performance. J. Cogn. Neurosci. 10, 167–177. doi: 10.1162/089892998562636
Chen, J., Lipska, B. K., Halim, N., Ma, Q. D., Matsumoto, M., Melhem, S., et al. (2004). Functional analysis of genetic variation in catechol-O-methyltransferase (COMT): effects on mRNA, protein, and enzyme activity in postmortem human brain. Am. J. Hum. Genet. 75, 807–821. doi: 10.1086/425589
Clark, K. L., Noudoost, B., and Moore, T. (2012). Persistent spatial information in the frontal eye field during object-based short-term memory. J. Neurosci. 32, 10907–10914. doi: 10.1523/JNEUROSCI.1450-12.2012
Clark, K. L., Noudoost, B., Schafer, R. J., and Moore, T. (2014). “Neuronal mechanisms of attentional control: Frontal cortex,” in Handbook of Attention, eds S. Kastner and A. C. Nobre (Oxford: Oxford University Press), 375–398.
Colby, C. L., Duhamel, J.-R., and Goldberg, M. E. (1996). Visual, presaccadic, and cognitive activation of single neurons in monkey lateral intraparietal area. J. Neurophysiol. 76, 2841–2852.
Constantinidis, C., Williams, G. V., and Goldman-Rakic, P. S. (2002). A role for inhibition in shaping the temporal flow of information in prefrontal cortex. Nat. Neurosci. 5, 175–180. doi: 10.1038/nn799
Cools, R., Barker, R. A., Sahakian, B. J., and Robbins, T. W. (2001). Enhanced or impaired cognitive function in Parkinson's disease as a function of dopaminergic medication and task demands. Cereb. Cortex 11, 1136–1143. doi: 10.1093/cercor/11.12.1136
Cools, R., Gibbs, S. E., Miyakawa, A., Jagust, W., and D'Esposito, M. (2008). Working memory capacity predicts dopamine synthesis capacity in the human striatum. J. Neurosci. 28, 1208–1212. doi: 10.1523/JNEUROSCI.4475-07.2008
Cools, R., Miyakawa, A., Sheridan, M., and D'Esposito, M. (2010). Enhanced frontal function in Parkinson's disease. Brain 133(Pt 1), 225–233. doi: 10.1093/brain/awp301
Cools, R., Stefanova, E., Barker, R. A., Robbins, T. W., and Owen, A. M. (2002). Dopaminergic modulation of high-level cognition in Parkinson's disease: the role of the prefrontal cortex revealed by PET. Brain 125(Pt 3), 584–594. doi: 10.1093/brain/awf052
D'Ardenne, K., Eshel, N., Luka, J., Lenartowicz, A., Nystrom, L. E., and Cohen, J. D. (2012). Role of prefrontal cortex and the midbrain dopamine system in working memory updating. Proc. Natl. Acad. Sci. U.S.A. 109, 19900–19909. doi: 10.1073/pnas.1116727109
Daly, G., Hawi, Z., Fitzgerald, M., and Gill, M. (1999). Mapping susceptibility loci in attention deficit hyperactivity disorder: preferential transmission of parental alleles at DAT1, DBH and DRD5 to affected children. Mol. Psychiatry 4, 192–196. doi: 10.1038/sj.mp.4000510
Decamp, E., and Schneider, J. S. (2004). Attention and executive function deficits in chronic low-dose MPTP-treated non-human primates. Eur. J. Neurosci. 20, 1371–1378. doi: 10.1111/j.1460-9568.2004.03586.x
D'Esposito, M., and Postle, B. R. (1999). The dependence of span and delayed-response performance on prefrontal cortex. Neuropsychologia 37, 1303–1315. doi: 10.1016/S0028-3932(99)00021-4
Dias, R., Robbins, T. W., and Roberts, A. C. (1996). Dissociation in prefrontal cortex of affective and attentional shifts. Nature 380, 69–72. doi: 10.1038/380069a0
Ding, L., and Hikosaka, O. (2006). Comparison of reward modulation in the frontal eye field and caudate of the macaque. J. Neurosci. 26, 6695–6703. doi: 10.1523/JNEUROSCI.0836-06.2006
di Pellegrino, G., and Wise, S. P. (1993). Effects of attention on visuomotor activity in the premotor and prefrontal cortex of a primate. Somatosens. Mot. Res. 10, 245–262. doi: 10.3109/08990229309028835
Durstewitz, D., Seamans, J. K., and Sejnowski, T. J. (2000). Dopamine-mediated stabilization of delay-period activity in a network model of prefrontal cortex. J. Neurophysiol. 83, 1733–1750.
Durston, S., Fossella, J. A., Casey, B. J., Hulshoff Pol, H. E., Galvan, A., Schnack, H. G., et al. (2005). Differential effects of DRD4 and DAT1 genotype on fronto-striatal gray matter volumes in a sample of subjects with attention deficit hyperactivity disorder, their unaffected siblings, and controls. Mol. Psychiatry 10, 678–685. doi: 10.1038/sj.mp.4001649
Eckhoff, P., Wong-Lin, K. F., and Holmes, P. (2009). Optimality and robustness of a biophysical decision-making model under norepinephrine modulation. J. Neurosci. 29, 4301–4311. doi: 10.1523/JNEUROSCI.5024-08.2009
Egan, M. F., Goldberg, T. E., Kolachana, B. S., Callicott, J. H., Mazzanti, C. M., Straub, R. E., et al. (2001). Effect of COMT Val108/158 Met genotype on frontal lobe function and risk for schizophrenia. Proc. Natl. Acad. Sci. U.S.A. 98, 6917–6922. doi: 10.1073/pnas.111134598
Elliott, R., Sahakian, B. J., Matthews, K., Bannerjea, A., Rimmer, J., and Robbins, T. W. (1997). Effects of methylphenidate on spatial working memory and planning in healthy young adults. Psychopharmacology 131, 196–206. doi: 10.1007/s002130050284
Fernandez-Ruiz, J., Doudet, D. J., and Aigner, T. G. (1995). Long-term cognitive impairment in MPTP-treated rhesus monkeys. Neuroreport 7, 102–104. doi: 10.1097/00001756-199512290-00024
Fernández-Ruiz, J., Doudet, D., and Aigner, T. G. (1999). Spatial memory improvement by levodopa in parkinsonian MPTP-treated monkeys. Psychopharmacology 147, 104–107. doi: 10.1007/s002130051148
Fischer, H., Nyberg, L., Karlsson, S., Karlsson, P., Brehmer, Y., Rieckmann, A., et al. (2010). Simulating neurocognitive aging: effects of a dopaminergic antagonist on brain activity during working memory. Biol. Psychiatry 67, 575–580. doi: 10.1016/j.biopsych.2009.12.013
Fleming, K., Goldberg, T. E., Gold, J. M., and Weinberger, D. R. (1995). Verbal working memory dysfunction in schizophrenia: use of a Brown-Peterson paradigm. Psychiatry Res. 56, 155–161. doi: 10.1016/0165-1781(95)02589-3
Frank, M. J., Moustafa, A. A., Haughey, H. M., Curran, T., and Hutchison, K. E. (2007). Genetic triple dissociation reveals multiple roles for dopamine in reinforcement learning. Proc. Natl. Acad. Sci. U.S.A. 104, 16311–16316. doi: 10.1073/pnas.0706111104
Friedman-Hill, S. R., Wagman, M. R., Gex, S. E., Pine, D. S., Leibenluft, E., and Ungerleider, L. G. (2010). What does distractibility in ADHD reveal about mechanisms for top-down attentional control? Cognition 115, 93–103. doi: 10.1016/j.cognition.2009.11.013
Funahashi, S., Bruce, C. J., and Goldman-Rakic, P. S. (1989). Mnemonic coding of visual space in the monkey's dorsolateral prefrontal cortex. J. Neurophysiol. 61, 331–349.
Gamo, N. J., and Arnsten, A. F. (2011). Molecular modulation of prefrontal cortex: rational development of treatments for psychiatric disorders. Behav. Neurosci. 125, 282–296. doi: 10.1037/a0023165
Gao, W. J., and Goldman-Rakic, P. S. (2003). Selective modulation of excitatory and inhibitory microcircuits by dopamine. Proc. Natl. Acad. Sci. U.S.A. 100, 2836–2841. doi: 10.1073/pnas.262796399
Gao, W. J., Krimer, L. S., and Goldman-Rakic, P. S. (2001). Presynaptic regulation of recurrent excitation by D1 receptors in prefrontal circuits. Proc. Natl. Acad. Sci. U.S.A. 98, 295–300. doi: 10.1073/pnas.98.1.295
Ghent, L., Mishkin, M., and Teuber, H. L. (1962). Short-term memory after frontal-lobe injury in man. J. Comp. Physiol. Pysch. 55, 705–709. doi: 10.1037/h0047520
Gibbs, S. E. B., and D'Esposito, M. (2005). Individual capacity differences predict working memory performance and prefrontal activity following dopamine receptor stimulation. Cogn. Affect. Behav. Neurosci. 5, 212–221. doi: 10.3758/CABN.5.2.212
Gizer, I. R., Ficks, C., and Waldman, I. D. (2009). Candidate gene studies of ADHD: a meta-analytic review. Hum. Genet. 126, 51–90. doi: 10.1007/s00439-009-0694-x
Glatt, S. J., Faraone, S. V., and Tsuang, M. T. (2003). Meta-analysis identifies an association between the dopamine D2 receptor gene and schizophrenia. Mol. Psychiatry 8, 911–915. doi: 10.1038/sj.mp.4001321
Gogos, J. A., Morgan, M., Luine, V., Santha, M., Ogawa, S., Pfaff, D., et al. (1998). Catechol-O-methyltransferase-deficient mice exhibit sexually dimorphic changes in catecholamine levels and behavior. Proc. Natl. Acad. Sci. U.S.A. 95, 9991–9996. doi: 10.1073/pnas.95.17.9991
Goldberg, M. E., and Wurtz, R. H. (1972). Activity of superior colliculus in behaving monkey. II. Effect of attention on neuronal responses. J. Neurophysiol. 35, 560–574.
Goldberg, T. E., Egan, M. F., Gscheidle, T., Coppola, R., Weickert, T., Kolachana, B. S., et al. (2003). Executive subprocesses in working memory: relationship to catechol-O-methyltransferase Val158Met genotype and schizophrenia. Arch. Gen. Psychiatry 60, 889–896. doi: 10.1001/archpsyc.60.9.889
Goldman-Rakic, P. S., Leranth, C., Williams, S. M., Mons, N., and Geffard, M. (1989). Dopamine synaptic complex with pyramidal neurons in primate cerebral cortex. Proc. Natl. Acad. Sci. U.S.A. 86, 9015–9019. doi: 10.1073/pnas.86.22.9015
Goldman-Rakic, P. S., Lidow, M. S., Smiley, J. F., and Williams, M. S. (1992). The anatomy of dopamine in monkey and human prefrontal cortex. J. Neural Transm. Suppl. 36, 163–177. doi: 10.1007/978-3-7091-9211-5_8
Gonzalez-Burgos, G., Kroener, S., Seamans, J. K., Lewis, D. A., and Barrionuevo, G. (2005). Dopaminergic modulation of short-term synaptic plasticity in fast-spiking interneurons of primate dorsolateral prefrontal cortex. J. Neurophysiol. 94, 4168–4177. doi: 10.1152/jn.00698.2005
González-Burgos, G., Kröner, S., Krimer, L. S., Seamans, J. K., Urban, N. N., Henze, D. A., et al. (2002). Dopamine modulation of neuronal function in the monkey prefrontal cortex. Physiol. Behav. 77, 537–543. doi: 10.1016/S0031-9384(02)00940-X
Gordon, E. M., Devaney, J. M., Bean, S., and Vaidya, C. J. (2013). Resting-state striato-frontal functional connectivity is sensitive to DAT1 genotype and predicts executive function. Cereb. Cortex doi: 10.1093/cercor/bht229. [Epub ahead of print].
Goto, Y., Hatakeyama, K., Kitama, T., Sato, Y., Kanemura, H., Aoyagi, K., et al. (2010). Saccade eye movements as a quantitative measure of frontostriatal network in children with ADHD. Brain Dev. 32, 347–355. doi: 10.1016/j.braindev.2009.04.017
Green, M. F. (1996). What are the functional consequences of neurocognitive deficits in schizophrenia? Am. J. Psychiatry 153, 321–330.
Guillin, O., Abi-Dargham, A., and Laruelle, M. (2007). Neurobiology of dopamine in schizophrenia. Int. Rev. Neurobiol. 78, 1–39. doi: 10.1016/S0074-7742(06)78001-1
Gurden, H., Takita, M., and Jay, T. M. (2000). Essential role of D1 but not D2 receptors in the NMDA receptor-dependent long-term potentiation at hippocampal-prefrontal cortex synapses in vivo. J. Neurosci. 20:RC106.
Harrison, P. J., and Weinberger, D. R. (2005). Schizophrenia genes, gene expression, and neuropathology: on the matter of their convergence. Mol. Psychiatry 10, 40–68; image 5. doi: 10.1038/sj.mp.4001558
Henze, D. A., González-Burgos, G. R., Urban, N. N., Lewis, D. A., and Barrionuevo, G. (2000). Dopamine increases excitability of pyramidal neurons in primate prefrontal cortex. J. Neurophysiol. 84, 2799–2809.
Hollerman, J. R., Tremblay, L., and Schultz, W. (1998). Influence of reward expectation on behavior-related neuronal activity in primate striatum. J. Neurophysiol. 80, 947–963.
Hunt, R. D., Arnsten, A. F., and Asbell, M. D. (1995). An open trial of guanfacine in the treatment of attention-deficit hyperactivity disorder. J. Am. Acad. Child Adolesc. Psychiatry 34, 50–54. doi: 10.1097/00004583-199501000-00013
Ikeda, T., and Hikosaka, O. (2003). Reward-dependent gain and bias of visual responses in primate superior colliculus. Neuron 39, 693–700. doi: 10.1016/S0896-6273(03)00464-1
Jacob, S. N., Ott, T., and Nieder, A. (2013). Dopamine regulates two classes of primate prefrontal neurons that represent sensory signals. J. Neurosci. 33, 13724–13734. doi: 10.1523/JNEUROSCI.0210-13.2013
Jäkälä, P., Riekkinen, M., Sirviö, J., Koivisto, E., Kejonen, K., Vanhanen, M., et al. (1999). Guanfacine, but not clonidine, improves planning and working memory performance in humans. Neuropsychopharmacology 20, 460–470. doi: 10.1016/S0893-133X(98)00127-4
Jönsson, E. G., Kaiser, R., Brockmöller, J., Nimgaonkar, V. L., and Crocq, M.-A. (2004). Meta-analysis of the dopamine D3 receptor gene (DRD3) Ser9Gly variant and schizophrenia. Psychiatr. Genet. 14, 9–12. doi: 10.1097/00041444-200403000-00002
Kaping, D., Vinck, M., Hutchison, R. M., Everling, S., and Womelsdorf, T. (2011). Specific contributions of ventromedial, anterior cingulate, and lateral prefrontal cortex for attentional selection and stimulus valuation. PLoS Biol. 9:e1001224. doi: 10.1371/journal.pbio.1001224
Kapur, S. (2003). Psychosis as a state of aberrant salience: a framework linking biology, phenomenology, and pharmacology in schizophrenia. Am. J. Psychiatry 160, 13–23. doi: 10.1176/appi.ajp.160.1.13
Kapur, S., Mizrahi, R., and Li, M. (2005). From dopamine to salience to psychosis–linking biology, pharmacology and phenomenology of psychosis. Schizophr. Res. 79, 59–68. doi: 10.1016/j.schres.2005.01.003
Keefe, R. S., Lees-Roitman, S. E., and Dupre, R. L. (1997). Performance of patients with schizophrenia on a pen and paper visuospatial working memory task with short delay. Schizophr. Res. 26, 9–14. doi: 10.1016/S0920-9964(97)00037-6
Kennerley, S. W., and Wallis, J. D. (2009). Reward-dependent modulation of working memory in lateral prefrontal cortex. J. Neurosci. 29, 3259–3270. doi: 10.1523/JNEUROSCI.5353-08.2009
Kieling, C., Goncalves, R. R. F., Tannock, R., and Castellanos, F. X. (2008). Neurobiology of attention deficit hyperactivity disorder. Child Adolesc. Psychiatr. Clin. N. Am. 17, 285–307. doi: 10.1016/j.chc.2007.11.012
Kimberg, D. Y., and D'Esposito, M. (2003). Cognitive effects of the dopamine receptor agonist pergolide. Neuropsychologia 41, 1020–1027. doi: 10.1016/S0028-3932(02)00317-2
Kimberg, D. Y., D'Esposito, M., and Farah, M. J. (1997). Effects of bromocriptine on human subjects depend on working memory capacity. Neuroreport 8, 3581–3585. doi: 10.1097/00001756-199711100-00032
Kish, S. J., Shannak, K., and Hornykiewicz, O. (1988). Uneven pattern of dopamine loss in the striatum of patients with idiopathic Parkinson's disease. Pathophysiologic and clinical implications. N. Engl. J. Med. 318, 876–880. doi: 10.1056/NEJM198804073181402
Kodaka, Y., Mikami, A., and Kubota, K. (1997). Neuronal activity in the frontal eye field of the monkey is modulated while attention is focused on to a stimulus in the peripheral visual field, irrespective of eye movement. Neurosci. Res. 28, 291–298. doi: 10.1016/S0168-0102(97)00055-2
Kopecková, M., Paclt, I., and Goetz, P. (2006). Polymorphisms of dopamine-beta-hydroxylase in ADHD children. Folia Biol. (Praha) 52, 194–201.
Kuczenski, R., and Segal, D. S. (1992). Regional norepinephrine response to amphetamine using dialysis: comparison with caudate dopamine. Synapse 11, 164–169. doi: 10.1002/syn.890110210
Kustanovich, V., Ishii, J., Crawford, L., Yang, M., McGough, J. J., McCracken, J. T., et al. (2004). Transmission disequilibrium testing of dopamine-related candidate gene polymorphisms in ADHD: confirmation of association of ADHD with DRD4 and DRD5. Mol. Psychiatry 9, 711–717. doi: 10.1038/sj.mp.4001466
Lachowicz, J. E., and Sibley, D. R. (1997). Molecular characteristics of mammalian dopamine receptors. Pharmacol. Toxicol. 81, 105–113. doi: 10.1111/j.1600-0773.1997.tb00039.x
Lange, K. W., Robbins, T. W., Marsden, C. D., James, M., Owen, A. M., and Paul, G. M. (1992). L-dopa withdrawal in Parkinson's disease selectively impairs cognitive performance in tests sensitive to frontal lobe dysfunction. Psychopharmacology 107, 394–404. doi: 10.1007/BF02245167
Laruelle, M., Casanova, M., Weinberger, D. R., and Kleinman, J. (1990). Postmortem study of the dopaminergic d1 receptors in the dorsolateral prefrontal cortex of schizophrenics and controls. Schizophr. Res.3, 30–31. doi: 10.1016/0920-9964(90)90097-Q
Leathers, M. L., and Olson, C. R. (2012). In monkeys making value-based decisions, LIP neurons encode cue salience and not action value. Science 338, 132–135. doi: 10.1126/science.1226405
Leathers, M. L., and Olson, C. R. (2013). Response to comment on “In monkeys making value-based decisions, LIP neurons encode cue salience and not action value.” Science 340, 430. doi: 10.1126/science.1233367
Lebedev, M. A., Messinger, A., Kralik, J. D., and Wise, S. P. (2004). Representation of attended versus remembered locations in prefrontal cortex. PLoS Biol. 2:e365. doi: 10.1371/journal.pbio.0020365
Lees, A. J., and Smith, E. (1983). Cognitive deficits in the early stages of Parkinson's disease. Brain 106(Pt 2), 257–270. doi: 10.1093/brain/106.2.257
Leon, M. I., and Shadlen, M. N. (1999). Effect of expected reward magnitude on the response of neurons in the dorsolateral prefrontal cortex of the macaque. Neuron 24, 415–425. doi: 10.1016/S0896-6273(00)80854-5
Levitt, P., Rakic, P., and Goldman-Rakic, P. (1984). Region-specific distribution of catecholamine afferents in primate cerebral cortex: a fluorescence histochemical analysis. J. Comp. Neurol. 227, 23–36. doi: 10.1002/cne.902270105
Lewis, D. A. (1997). Development of the prefrontal cortex during adolescence: insights into vulnerable neural circuits in schizophrenia. Neuropsychopharmacology 16, 385–398. doi: 10.1016/S0893-133X(96)00277-1
Lewis, D. A., Campbell, M. J., Foote, S. L., Goldstein, M., and Morrison, J. H. (1987). The distribution of tyrosine hydroxylase-immunoreactive fibers in primate neocortex is widespread but regionally specific. J. Neurosci. 7, 279–290.
Lewis, D. A., and Harris, H. W. (1991). Differential laminar distribution of tyrosine hydroxylase-immunoreactive axons in infant and adult monkey prefrontal cortex. Neurosci. Lett. 125, 151–154. doi: 10.1016/0304-3940(91)90014-K
Lewis, D. A., and Morrison, J. H. (1989). Noradrenergic innervation of monkey prefrontal cortex: a dopamine-beta-hydroxylase immunohistochemical study. J. Comp. Neurol. 282, 317–330. doi: 10.1002/cne.902820302
Li, B. M., Mao, Z. M., Wang, M., and Mei, Z. T. (1999). Alpha-2 adrenergic modulation of prefrontal cortical neuronal activity related to spatial working memory in monkeys. Neuropsychopharmacology 21, 601–610. doi: 10.1016/S0893-133X(99)00070-6
Li, B. M., and Mei, Z. T. (1994). Delayed-response deficit induced by local injection of the alpha 2-adrenergic antagonist yohimbine into the dorsolateral prefrontal cortex in young adult monkeys. Behav. Neural Biol. 62, 134–139. doi: 10.1016/S0163-1047(05)80034-2
Lidow, M. S., Goldman-Rakic, P. S., Gallager, D. W., and Rakic, P. (1991). Distribution of dopaminergic receptors in the primate cerebral cortex: quantitative autoradiographic analysis using [3H]raclopride, [3H]spiperone and [3H]SCH23390. Neuroscience 40, 657–671. doi: 10.1016/0306-4522(91)90003-7
Liu, B., Song, M., Li, J., Liu, Y., Li, K., Yu, C., et al. (2010). Prefrontal-related functional connectivities within the default network are modulated by COMT val158met in healthy young adults. J. Neurosci. 30, 64–69. doi: 10.1523/JNEUROSCI.3941-09.2010
Luciana, M., Collins, P. F., and Depue, R. A. (1998). Opposing roles for dopamine and serotonin in the modulation of human spatial working memory functions. Cereb. Cortex 8, 218–226. doi: 10.1093/cercor/8.3.218
Luciana, M., Depue, R. A., Arbisi, P., and Leon, A. (1992). Facilitation of working memory in humans by a d2 dopamine receptor agonist. J. Cogn. Neurosci. 4, 58–68. doi: 10.1162/jocn.1992.4.1.58
Manoach, D. S., Press, D. Z., Thangaraj, V., Searl, M. M., Goff, D. C., Halpern, E., et al. (1999). Schizophrenic subjects activate dorsolateral prefrontal cortex during a working memory task, as measured by fMRI. Biol. Psychiatry 45, 1128–1137. doi: 10.1016/S0006-3223(98)00318-7
Mao, Z. M., Arnsten, A. F., and Li, B. M. (1999). Local infusion of an alpha-1 adrenergic agonist into the prefrontal cortex impairs spatial working memory performance in monkeys. Biol. Psychiatry 46, 1259–1265. doi: 10.1016/S0006-3223(99)00139-0
Mattay, V. S., Callicott, J. H., Bertolino, A., Heaton, I., Frank, J. A., Coppola, R., et al. (2000). Effects of dextroamphetamine on cognitive performance and cortical activation. Neuroimage 12, 268–275. doi: 10.1006/nimg.2000.0610
Mattay, V. S., Goldberg, T. E., Fera, F., Hariri, A. R., Tessitore, A., Egan, M. F., et al. (2003). Catechol O-methyltransferase val158-met genotype and individual variation in the brain response to amphetamine. Proc. Natl. Acad. Sci. U.S.A. 100, 6186–6191. doi: 10.1073/pnas.0931309100
Mattay, V. S., Tessitore, A., Callicott, J. H., Bertolino, A., Goldberg, T. E., Chase, T. N., et al. (2002). Dopaminergic modulation of cortical function in patients with Parkinson's disease. Ann. Neurol. 51, 156–164. doi: 10.1002/ana.10078
Maunsell, J. H. R. (2004). Neuronal representations of cognitive state: reward or attention? Trends Cogn. Sci. 8, 261–265. doi: 10.1016/j.tics.2004.04.003
McAdams, C. J., and Maunsell, J. H. R. (2000). Attention to both space and feature modulates neuronal responses in macaque area V4. J. Neurophysiol. 83, 1751–1755.
McAdams, C. J., and Reid, R. C. (2005). Attention modulates the responses of simple cells in monkey primary visual cortex. J. Neurosci. 25, 11023–11033. doi: 10.1523/JNEUROSCI.2904-05.2005
McNab, F., Varrone, A., Farde, L., Jucaite, A., Bystritsky, P., Forssberg, H., et al. (2009). Changes in cortical dopamine D1 receptor binding associated with cognitive training. Science 323, 800–802. doi: 10.1126/science.1166102
Meador-Woodruff, J. H., Haroutunian, V., Powchik, P., Davidson, M., Davis, K. L., and Watson, S. J. (1997). Dopamine receptor transcript expression in striatum and prefrontal and occipital cortex. Focal abnormalities in orbitofrontal cortex in schizophrenia. Arch. Gen. Psychiatry 54, 1089–1095. doi: 10.1001/archpsyc.1997.01830240045007
Mehta, M. A., Manes, F. F., Magnolfi, G., Sahakian, B. J., and Robbins, T. W. (2004). Impaired set-shifting and dissociable effects on tests of spatial working memory following the dopamine D2 receptor antagonist sulpiride in human volunteers. Psychopharmacology 176, 331–342. doi: 10.1007/s00213-004-1899-2
Mehta, M. A., Owen, A. M., Sahakian, B. J., Mavaddat, N., Pickard, J. D., and Robbins, T. W. (2000). Methylphenidate enhances working memory by modulating discrete frontal and parietal lobe regions in the human brain. J. Neurosci. 20, RC65.
Mehta, M. A., Sahakian, B. J., McKenna, P. J., and Robbins, T. W. (1999). Systemic sulpiride in young adult volunteers simulates the profile of cognitive deficits in Parkinson's disease. Psychopharmacology 146, 162–174. doi: 10.1007/s002130051102
Mehta, M. A., Swainson, R., Ogilvie, A. D., Sahakian, J., and Robbins, T. W. (2001). Improved short-term spatial memory but impaired reversal learning following the dopamine D(2) agonist bromocriptine in human volunteers. Psychopharmacology 159, 10–20. doi: 10.1007/s002130100851
Meyer-Lindenberg, A., Kohn, P. D., Kolachana, B., Kippenhan, S., McInerney-Leo, A., Nussbaum, R., et al. (2005). Midbrain dopamine and prefrontal function in humans: interaction and modulation by COMT genotype. Nat. Neurosci. 8, 594–596. doi: 10.1038/nn1438
Miller, E. K. (2000). The prefrontal cortex and cognitive control. Nat. Rev. Neurosci. 1, 59–65. doi: 10.1038/35036228
Mill, J., Caspi, A., Williams, B. S., Craig, I., Taylor, A., Polo-Tomas, M., et al. (2006). Prediction of heterogeneity in intelligence and adult prognosis by genetic polymorphisms in the dopamine system among children with attention-deficit/hyperactivity disorder: evidence from 2 birth cohorts. Arch. Gen. Psychiatry 63, 462–469. doi: 10.1001/archpsyc.63.4.462
Missale, C., Nash, S. R., Robinson, S. W., Jaber, M., and Caron, M. G. (1998). Dopamine receptors: from structure to function. Physiol. Rev. 78, 189–225.
Mitchell, J. F., Sundberg, K. A., and Reynolds, J. H. (2007). Differential attention-dependent response modulation across cell classes in macaque visual area V4. Neuron 55, 131–141. doi: 10.1016/j.neuron.2007.06.018
Moghaddam, B., Berridge, C. W., Goldman-Rakic, P. S., Bunney, B. S., and Roth, R. H. (1993). In vivo assessment of basal and drug-induced dopamine release in cortical and subcortical regions of the anesthetized primate. Synapse 13, 215–222. doi: 10.1002/syn.890130304
Molinoff, P. B. (1984). Alpha- and beta-adrenergic receptor subtypes properties, distribution and regulation. Drugs 28(Suppl. 2), 1–15. doi: 10.2165/00003495-198400282-00002
Moore, T., and Armstrong, K. M. (2003). Selective gating of visual signals by microstimulation of frontal cortex. Nature 421, 370–373. doi: 10.1038/nature01341
Moore, T., and Fallah, M. (2004). Microstimulation of the frontal eye field and its effects on covert spatial attention. J. Neurophysiol. 91, 152–162. doi: 10.1152/jn.00741.2002
Moran, J., and Desimone, R. (1985). Selective attention gates visual processing in the extrastriate cortex. Science 229, 782–784. doi: 10.1126/science.4023713
Morice, R., and Delahunty, A. (1996). Frontal/executive impairments in schizophrenia. Schizophr. Bull. 22, 125–137. doi: 10.1093/schbul/22.1.125
Morris, R. G., Downes, J. J., Sahakian, B. J., Evenden, J. L., Heald, A., and Robbins, T. W. (1988). Planning and spatial working memory in Parkinson's disease. J. Neurol. Neurosurg. Psychiatry 51, 757–766. doi: 10.1136/jnnp.51.6.757
Morrison, J. H., Foote, S. L., O'Connor, D., and Bloom, F. E. (1982). Laminar, tangential and regional organization of the noradrenergic innervation of monkey cortex: dopamine-beta-hydroxylase immunohistochemistry. Brain Res. Bull. 9, 309–319. doi: 10.1016/0361-9230(82)90144-7
Motter, B. C. (1993). Focal attention produces spatially selective processing in visual cortical areas V1, V2, and V4 in the presence of competing stimuli. J. Neurophysiol. 70, 909–919.
Mowry, B. J., and Gratten, J. (2013). The emerging spectrum of allelic variation in schizophrenia: current evidence and strategies for the identification and functional characterization of common and rare variants. Mol. Psychiatry 18, 38–52. doi: 10.1038/mp.2012.34
Müller, U., von Cramon, D. Y., and Pollmann, S. (1998). D1- versus D2-receptor modulation of visuospatial working memory in humans. J. Neurosci. 18, 2720–2728.
Narendran, R., Jedema, H. P., Lopresti, B. J., Mason, N. S., Gurnsey, K., Ruszkiewicz, J., et al. (2014). Imaging dopamine transmission in the frontal cortex: a simultaneous microdialysis and [(11)C]FLB 457 PET study. Mol. Psychiatry 19, 302–310. doi: 10.1038/mp.2013.9
Newsome, W. T., Glimcher, P. W., Gottlieb, J., Lee, D., and Platt, M. L. (2013). Comment on “In monkeys making value-based decisions, LIP neurons encode cue salience and not action value.” Science 340, 430. doi: 10.1126/science.1233214
Noudoost, B., Chang, M. H., Steinmetz, N. A., and Moore, T. (2010). Top-down control of visual attention. Curr. Opin. Neurobiol. 20, 183–190. doi: 10.1016/j.conb.2010.02.003
Noudoost, B., Clark, K. L., and Moore, T. (2014). A distinct contribution of the frontal eye field to the visual representation of saccadic targets. J. Neurosci. 34, 3687–3698. doi: 10.1523/JNEUROSCI.3824-13.2014
Noudoost, B., and Moore, T. (2011a). A reliable microinjectrode system for use in behaving monkeys. J. Neurosci. Methods 194, 218–223. doi: 10.1016/j.jneumeth.2010.10.009
Noudoost, B., and Moore, T. (2011b). Control of visual cortical signals by prefrontal dopamine. Nature 474, 372–375. doi: 10.1038/nature09995
Noudoost, B., and Moore, T. (2011c). The role of neuromodulators in selective attention. Trends Cogn. Sci. 15, 585–591. doi: 10.1016/j.tics.2011.10.006
O'Neill, J., Fitten, L. J., Siembieda, D. W., Ortiz, F., and Halgren, E. (2000). Effects of guanfacine on three forms of distraction in the aging macaque. Life Sci. 67, 877–885. doi: 10.1016/S0024-3205(00)00681-0
Owen, A. M., Beksinska, M., James, M., Leigh, P. N., Summers, B. A., Marsden, C. D., et al. (1993). Visuospatial memory deficits at different stages of Parkinson's disease. Neuropsychologia 31, 627–644. doi: 10.1016/0028-3932(93)90135-M
Owen, A. M., James, M., Leigh, P. N., Summers, B. A., Marsden, C. D., Quinn, N. P., et al. (1992). Fronto-striatal cognitive deficits at different stages of Parkinson's disease. Brain 115, 1727–1751. doi: 10.1093/brain/115.6.1727
Park, S., and Holzman, P. S. (1992). Schizophrenics show spatial working memory deficits. Arch. Gen. Psychiatry 49, 975–982. doi: 10.1001/archpsyc.1992.01820120063009
Pawlak, V., and Kerr, J. N. D. (2008). Dopamine receptor activation is required for corticostriatal spike-timing-dependent plasticity. J. Neurosci. 28, 2435–2446. doi: 10.1523/JNEUROSCI.4402-07.2008
Peck, C. J., Jangraw, D. C., Suzuki, M., Efem, R., and Gottlieb, J. (2009). Reward modulates attention independently of action value in posterior parietal cortex. J. Neurosci. 29, 11182–11191. doi: 10.1523/JNEUROSCI.1929-09.2009
Perlstein, W. M., Carter, C. S., Noll, D. C., and Cohen, J. D. (2001). Relation of prefrontal cortex dysfunction to working memory and symptoms in schizophrenia. Am. J. Psychiatry 158, 1105–1113. doi: 10.1176/appi.ajp.158.7.1105
Pirot, S., Godbout, R., Mantz, J., Tassin, J. P., Glowinski, J., and Thierry, A. M. (1992). Inhibitory effects of ventral tegmental area stimulation on the activity of prefrontal cortical neurons: evidence for the involvement of both dopaminergic and GABAergic components. Neuroscience 49, 857–865. doi: 10.1016/0306-4522(92)90362-6
Platt, M. L., and Glimcher, P. W. (1999). Neural correlates of decision variables in parietal cortex. Nature 400, 233–238. doi: 10.1038/22268
Porrino, L. J., and Goldman-Rakic, P. S. (1982). Brainstem innervation of prefrontal and anterior cingulate cortex in the rhesus monkey revealed by retrograde transport of HRP. J. Comp. Neurol. 205, 63–76. doi: 10.1002/cne.902050107
Posner, M. I. (1980). Orienting of attention. Q. J. Exp. Psychol. 32, 3–25. doi: 10.1080/00335558008248231
Postle, B. R., Jonides, J., Smith, E. E., Corkin, S., and Growdon, J. H. (1997). Spatial, but not object, delayed response is impaired in early Parkinson's disease. Neuropsychology 11, 171–179. doi: 10.1037/0894-4105.11.2.171
Puig, M. V., and Miller, E. K. (2012). The role of prefrontal dopamine D1 receptors in the neural mechanisms of associative learning. Neuron 74, 874–886. doi: 10.1016/j.neuron.2012.04.018
Rämä, P., Linnankoski, I., Tanila, H., Pertovaara, A., and Carlson, S. (1996). Medetomidine, atipamezole, and guanfacine in delayed response performance of aged monkeys. Pharmacol. Biochem. Behav. 55, 415–422. doi: 10.1016/S0091-3057(96)00111-6
Ramos, B. P., Colgan, L., Nou, E., Ovadia, S., Wilson, S. R., and Arnsten, A. F. (2005). The beta-1 adrenergic antagonist, betaxolol, improves working memory performance in rats and monkeys. Biol. Psychiatry 58, 894–900. doi: 10.1016/j.biopsych.2005.05.022
Rao, S. G., Williams, G. V., and Goldman-Rakic, P. S. (2000). Destruction and creation of spatial tuning by disinhibition: GABA(A) blockade of prefrontal cortical neurons engaged by working memory. J. Neurosci. 20, 485–494.
Reynolds, J. H., Pasternak, T., and Desimone, R. (2000). Attention increases sensitivity of V4 neurons. Neuron 26, 703–714. doi: 10.1016/S0896-6273(00)81206-4
Roesch, M. R., and Olson, C. R. (2003). Impact of expected reward on neuronal activity in prefrontal cortex, frontal and supplementary eye fields and premotor cortex. J. Neurophysiol. 90, 1766–1789. doi: 10.1152/jn.00019.2003
Roman, T., Schmitz, M., Polanczyk, G. V., Eizirik, M., Rohde, L. A., and Hutz, M. H. (2002). Further evidence for the association between attention-deficit/hyperactivity disorder and the dopamine-beta-hydroxylase gene. Am. J. Med. Genet. 114, 154–158. doi: 10.1002/ajmg.10194
Roman, T., Schmitz, M., Polanczyk, G. V., Eizirik, M., Rohde, L. A., and Hutz, M. H. (2003). Is the alpha-2A adrenergic receptor gene (ADRA2A) associated with attention-deficit/hyperactivity disorder? Am. J. Med. Genet. B Neuropsychiatr. Genet. 120B, 116–120. doi: 10.1002/ajmg.b.20018
Rommelse, N. N. J., Van der Stigchel, S., Witlox, J., Geldof, C., Deijen, J.-B., Theeuwes, J., et al. (2008). Deficits in visuo-spatial working memory, inhibition and oculomotor control in boys with ADHD and their non-affected brothers. J. Neural Trans. 115, 249–260. doi: 10.1007/s00702-007-0865-7
Rosenberg, D. R., and Lewis, D. A. (1995). Postnatal maturation of the dopaminergic innervation of monkey prefrontal and motor cortices: a tyrosine hydroxylase immunohistochemical analysis. J. Comp. Neurol. 358, 383–400. doi: 10.1002/cne.903580306
Roth, R. H., Tam, S. Y., Ida, Y., Yang, J. X., and Deutch, A. Y. (1988). Stress and the mesocorticolimbic dopamine systems. Ann. N.Y. Acad. Sci. 537, 138–147. doi: 10.1111/j.1749-6632.1988.tb42102.x
Sawaguchi, T. (1998). Attenuation of delay-period activity of monkey prefrontal neurons by an alpha2-adrenergic antagonist during an oculomotor delayed-response task. J. Neurophysiol. 80, 2200–2205.
Sawaguchi, T., and Goldman-Rakic, P. S. (1991). D1 dopamine receptors in prefrontal cortex: involvement in working memory. Science 251, 947–950. doi: 10.1126/science.1825731
Sawaguchi, T., and Goldman-Rakic, P. S. (1994). The role of D1-dopamine receptor in working memory: local injections of dopamine antagonists into the prefrontal cortex of rhesus monkeys performing an oculomotor delayed-response task. J. Neurophysiol. 71, 515–528.
Sawaguchi, T., Matsumura, M., and Kubota, K. (1988). Dopamine enhances the neuronal activity of spatial short-term memory task in the primate prefrontal cortex. Neurosci. Res. 5, 465–473. doi: 10.1016/0168-0102(88)90030-2
Sawaguchi, T., Matsumura, M., and Kubota, K. (1990a). Effects of dopamine antagonists on neuronal activity related to a delayed response task in monkey prefrontal cortex. J. Neurophysiol. 63, 1401–1412.
Sawaguchi, T., Matsumura, M., and Kubota, K. (1990b). Catecholaminergic effects on neuronal activity related to a delayed response task in monkey prefrontal cortex. J. Neurophysiol. 63, 1385–1400.
Schneider, J. S., and Kovelowski, C. J. (1990). Chronic exposure to low doses of MPTP. I. Cognitive deficits in motor asymptomatic monkeys. Brain Res. 519, 122–128. doi: 10.1016/0006-8993(90)90069-N
Schneider, J. S., and Roeltgen, D. P. (1993). Delayed matching-to-sample, object retrieval, and discrimination reversal deficits in chronic low dose MPTP-treated monkeys. Brain Res. 615, 351–354. doi: 10.1016/0006-8993(93)90049-S
Schneider, J. S., Sun, Z. Q., and Roeltgen, D. P. (1994a). Effects of dopamine agonists on delayed response performance in chronic low-dose MPTP-treated monkeys. Pharmacol. Biochem. Behav. 48, 235–240. doi: 10.1016/0091-3057(94)90522-3
Schneider, J. S., Sun, Z. Q., and Roeltgen, D. P. (1994b). Effects of dihydrexidine, a full dopamine D-1 receptor agonist, on delayed response performance in chronic low dose MPTP-treated monkeys. Brain Res. 663, 140–144. doi: 10.1016/0006-8993(94)90471-5
Schultz, W. (2002). Getting formal with dopamine and reward. Neuron 36, 241–263. doi: 10.1016/S0896-6273(02)00967-4
Schultz, W. (2013). Updating dopamine reward signals. Curr. Opin. Neurobiol. 23, 229–238. doi: 10.1016/j.conb.2012.11.012
Schultz, W., Apicella, P., and Ljungberg, T. (1993). Responses of monkey dopamine neurons to reward and conditioned stimuli during successive steps of learning a delayed response task. J. Neurosci. 13, 900–913.
Seamans, J. K., and Yang, C. R. (2004). The principal features and mechanisms of dopamine modulation in the prefrontal cortex. Prog. Neurobiol. 74, 1–58. doi: 10.1016/j.pneurobio.2004.05.006
Seeman, P., and Lee, T. (1975). Antipsychotic drugs: direct correlation between clinical potency and presynaptic action on dopamine neurons. Science 188, 1217–1219. doi: 10.1126/science.1145194
Seidman, L. J., Valera, E. M., and Makris, N. (2005). Structural brain imaging of attention-deficit/hyperactivity disorder. Biol. Psychiatry 57, 1263–1272. doi: 10.1016/j.biopsych.2004.11.019
Serences, J. T. (2008). Value-based modulations in human visual cortex. Neuron 60, 1169–1181. doi: 10.1016/j.neuron.2008.10.051
Servan-Schreiber, D., Bruno, R. M., Carter, C. S., and Cohen, J. D. (1998). Dopamine and the mechanisms of cognition: part I. A neural network model predicting dopamine effects on selective attention. Biol. Psychiatry 43, 713–722. doi: 10.1016/S0006-3223(97)00448-4
Sesack, S. R., Hawrylak, V. A., Melchitzky, D. S., and Lewis, D. A. (1998). Dopamine innervation of a subclass of local circuit neurons in monkey prefrontal cortex: ultrastructural analysis of tyrosine hydroxylase and parvalbumin immunoreactive structures. Cereb. Cortex 8, 614–622. doi: 10.1093/cercor/8.7.614
Sesack, S. R., Snyder, C. L., and Lewis, D. A. (1995). Axon terminals immunolabeled for dopamine or tyrosine hydroxylase synapse on GABA-immunoreactive dendrites in rat and monkey cortex. J. Comp. Neurol. 363, 264–280. doi: 10.1002/cne.903630208
Shidara, M., and Richmond, B. J. (2002). Anterior cingulate: single neuronal signals related to degree of reward expectancy. Science 296, 1709–1711. doi: 10.1126/science.1069504
Slovin, H., Abeles, M., Vaadia, E., Haalman, I., Prut, Y., and Bergman, H. (1999). Frontal cognitive impairments and saccadic deficits in low-dose MPTP-treated monkeys. J. Neurophysiol. 81, 858–874.
Smiley, J. F., and Goldman-Rakic, P. S. (1993). Heterogeneous targets of dopamine synapses in monkey prefrontal cortex demonstrated by serial section electron microscopy: a laminar analysis using the silver-enhanced diaminobenzidine sulfide (SEDS) immunolabeling technique. Cereb. Cortex 3, 223–238. doi: 10.1093/cercor/3.3.223
Smiley, J. F., Levey, A. I., Ciliax, B. J., and Goldman-Rakic, P. S. (1994). D1 dopamine receptor immunoreactivity in human and monkey cerebral cortex: predominant and extrasynaptic localization in dendritic spines. Proc. Natl. Acad. Sci. U.S.A. 91, 5720–5724. doi: 10.1073/pnas.91.12.5720
Soltani, A., Noudoost, B., and Moore, T. (2013). Dissociable dopaminergic control of saccadic target selection and its implications for reward modulation. Proc. Natl. Acad. Sci. U.S.A. 110, 3579–3584. doi: 10.1073/pnas.1221236110
Squire, R. F., Noudoost, B., Schafer, R. J., and Moore, T. (2013). Prefrontal contributions to visual selective attention. Annu. Rev. Neurosci. 36, 451–466. doi: 10.1146/annurev-neuro-062111-150439
Stănişor, L., van der Togt, C., Pennartz, C. M. A., and Roelfsema, P. R. (2013). A unified selection signal for attention and reward in primary visual cortex. Proc. Natl. Acad. Sci. U.S.A. 110, 9136–9141. doi: 10.1073/pnas.1300117110
Sunohara, G. A., Roberts, W., Malone, M., Schachar, R. J., Tannock, R., Basile, V. S., et al. (2000). Linkage of the dopamine D4 receptor gene and attention-deficit/hyperactivity disorder. J. Am. Acad. Child Adolesc. Psychiatry 39, 1537–1542. doi: 10.1097/00004583-200012000-00017
Suzuki, H., and Azuma, M. (1983). Topographic studies on visual neurons in the dorsolateral prefrontal cortex of the monkey. Exp. Brain Res. 53, 47–58. doi: 10.1007/BF00239397
Tahir, E., Yazgan, Y., Cirakoglu, B., Ozbay, F., Waldman, I., and Asherson, P. J. (2000). Association and linkage of DRD4 and DRD5 with attention deficit hyperactivity disorder (ADHD) in a sample of Turkish children. Mol. Psychiatry 5, 396–404. doi: 10.1038/sj.mp.4000744
Taylor, A. E., Saint-Cyr, J. A., and Lang, A. E. (1986). Frontal lobe dysfunction in Parkinson's disease. The cortical focus of neostriatal outflow. Brain 109(Pt 5), 845–883. doi: 10.1093/brain/109.5.845
Taylor, F. B., and Russo, J. (2001). Comparing guanfacine and dextroamphetamine for the treatment of adult attention-deficit/hyperactivity disorder. J. Clin. Psychopharmacol. 21, 223–228. doi: 10.1097/00004714-200104000-00015
Thierry, A. M., Tassin, J. P., Blanc, G., and Glowinski, J. (1976). Selective activation of mesocortical DA system by stress. Nature 263, 242–244. doi: 10.1038/263242a0
Tian, T., Qin, W., Liu, B., Jiang, T., and Yu, C. (2013). Functional connectivity in healthy subjects is nonlinearly modulated by the COMT and DRD2 polymorphisms in a functional system-dependent manner. J. Neurosci. 33, 17519–17526. doi: 10.1523/JNEUROSCI.2163-13.2013
Vijayraghavan, S., Wang, M., Birnbaum, S. G., Williams, G. V., and Arnsten, A. F. (2007). Inverted-U dopamine D1 receptor actions on prefrontal neurons engaged in working memory. Nat. Neurosci. 10, 376–384. doi: 10.1038/nn1846
Wang, M., Ramos, B. P., Paspalas, C. D., Shu, Y., Simen, A., Duque, A., et al. (2007). Alpha2A-adrenoceptors strengthen working memory networks by inhibiting cAMP-HCN channel signaling in prefrontal cortex. Cell 129, 397–410. doi: 10.1016/j.cell.2007.03.015
Wang, M., Vijayraghavan, S., and Goldman-Rakic, P. S. (2004). Selective D2 receptor actions on the functional circuitry of working memory. Science 303, 853–856. doi: 10.1126/science.1091162
Weickert, T. W., Goldberg, T. E., Mishara, A., Apud, J. A., Kolachana, B. S., Egan, M. F., et al. (2004). Catechol-O-methyltransferase val108/158met genotype predicts working memory response to antipsychotic medications. Biol. Psychiatry 56, 677–682. doi: 10.1016/j.biopsych.2004.08.012
Williams, G. V., and Goldman-Rakic, P. S. (1995). Modulation of memory fields by dopamine D1 receptors in prefrontal cortex. Nature 376, 572–575. doi: 10.1038/376572a0
Wu, J., Xiao, H., Sun, H., Zou, L., and Zhu, L. Q. (2012). Role of dopamine receptors in ADHD: a systematic meta-analysis. Mol. Neurobiol. 45, 605–620. doi: 10.1007/s12035-012-8278-5
Xu, C., Schachar, R., Tannock, R., Roberts, W., Malone, M., Kennedy, J. L., et al. (2001). Linkage study of the alpha2A adrenergic receptor in attention-deficit hyperactivity disorder families. Am. J. Med. Genet. 105, 159–162. doi: 10.1002/1096-8628(2001)9999:9999<::AID-AJMG1160>3.0.CO;2-B
Xu, T. X., and Yao, W. D. (2010). D1 and D2 dopamine receptors in separate circuits cooperate to drive associative long-term potentiation in the prefrontal cortex. Proc. Natl. Acad. Sci. U.S.A. 107, 16366–16371. doi: 10.1073/pnas.1004108107
Yang, C. R., and Seamans, J. K. (1996). Dopamine D1 receptor actions in layers V-VI rat prefrontal cortex neurons in vitro: modulation of dendritic-somatic signal integration. J. Neurosci. 16, 1922–1935.
Zhang, J. C., Lau, P. M., and Bi, G. Q. (2009). Gain in sensitivity and loss in temporal contrast of STDP by dopaminergic modulation at hippocampal synapses. Proc. Natl. Acad. Sci. U.S.A. 106, 13028–13033. doi: 10.1073/pnas.0900546106
Zhang, Y., Bertolino, A., Fazio, L., Blasi, G., Rampino, A., Romano, R., et al. (2007). Polymorphisms in human dopamine D2 receptor gene affect gene expression, splicing, and neuronal activity during working memory. Proc. Natl. Acad. Sci. U.S.A. 104, 20552–20557. doi: 10.1073/pnas.0707106104
Zhao, Y., Kerscher, N., Eysel, U., and Funke, K. (2002). D1 and D2 receptor-mediated dopaminergic modulation of visual responses in cat dorsal lateral geniculate nucleus. J. Physiol. 539(Pt 1), 223–238. doi: 10.1113/jphysiol.2001.012721
Zilles, D., Meyer, J., Schneider-Axmann, T., Ekawardhani, S., Gruber, E., Falkai, P., et al. (2012). Genetic polymorphisms of 5-HTT and DAT but not COMT differentially affect verbal and visuospatial working memory functioning. Eur. Arch. Psychiatry Clin. Neurosci. 262, 667–676. doi: 10.1007/s00406-012-0312-0
Keywords: dopamine, reward, top-down control, pathophysiology, frontal eye field, V4, extrastriate cortex
Citation: Clark KL and Noudoost B (2014) The role of prefrontal catecholamines in attention and working memory. Front. Neural Circuits 8:33. doi: 10.3389/fncir.2014.00033
Received: 13 January 2014; Accepted: 19 March 2014;
Published online: 08 April 2014.
Edited by:
M. Victoria Puig, Massachusetts Institute of Technology, USAReviewed by:
Albert Compte, IDIBAPS (Institut d'Investigacions Biomèdiques August Pi i Sunyer), SpainCopyright © 2014 Clark and Noudoost. This is an open-access article distributed under the terms of the Creative Commons Attribution License (CC BY). The use, distribution or reproduction in other forums is permitted, provided the original author(s) or licensor are credited and that the original publication in this journal is cited, in accordance with accepted academic practice. No use, distribution or reproduction is permitted which does not comply with these terms.
*Correspondence: Behrad Noudoost, Department of Cell Biology and Neuroscience, Montana State University, Room 01 Lewis Hall, Bozeman, MT 59717, USA e-mail:Ym5vdWRvb3N0QG1vbnRhbmEuZWR1
Disclaimer: All claims expressed in this article are solely those of the authors and do not necessarily represent those of their affiliated organizations, or those of the publisher, the editors and the reviewers. Any product that may be evaluated in this article or claim that may be made by its manufacturer is not guaranteed or endorsed by the publisher.
Research integrity at Frontiers
Learn more about the work of our research integrity team to safeguard the quality of each article we publish.