- 1Corporate Member of Freie Universität Berlin and Humboldt-Universität zu Berlin, Institute of Clinical Pharmacology and Toxicology, Charité—Universitätsmedizin Berlin, Berlin, Germany
- 2Lipidomix GmbH, Berlin, Germany
Background: Glomerular hyperfiltration (GH) is an important mechanism in the development of albuminuria in hypertension. The Munich Wistar Frömter (MWF) rat is a non-diabetic model of chronic kidney disease (CKD) with GH due to inherited low nephron number resulting in spontaneous albuminuria and podocyte injury. In MWF rats, we identified prostaglandin (PG) E2 (PGE2) signaling as a potential causative mechanism of albuminuria in GH.
Method: For evaluation of the renal PGE2 metabolic pathway, time-course lipidomic analysis of PGE2 and its downstream metabolites 15-keto-PGE2 and 13-14-dihydro-15-keto-PGE2 was conducted in urine, plasma and kidney tissues of MWF rats and albuminuria-resistant spontaneously hypertensive rats (SHR) by liquid chromatography electrospray ionization tandem mass spectrometry (LC/ESI-MS/MS).
Results: Lipidomic analysis revealed no dysregulation of plasma PGs over the time course of albuminuria development, while glomerular levels of PGE2 and 15-keto-PGE2 were significantly elevated in MWF compared to albuminuria-resistant SHR. Overall, averaged PGE2 levels in glomeruli were up to ×150 higher than the corresponding 15-keto-PGE2 levels. Glomerular metabolic ratios of 15-hydroxyprostaglandin dehydrogenase (15-PGDH) were significantly lower, while metabolic ratios of prostaglandin reductases (PTGRs) were significantly higher in MWF rats with manifested albuminuria compared to SHR, respectively.
Conclusion: Our data reveal glomerular dysregulation of the PGE2 metabolism in the development of albuminuria in GH, resulting at least partly from reduced PGE2 degradation. This study provides first insights into dynamic changes of the PGE2 pathway that support a role of glomerular PGE2 metabolism and signaling for early albuminuria manifestation in GH.
1 Introduction
Albuminuria is a leading symptom of the progression of chronic kidney disease (CKD) and early kidney injury (Srivastava et al., 2010; Carrero et al., 2017). Elevated fluid flow shear stress (FFSS) in Bowman’s space causes podocyte damage during glomerular hyperfiltration (GH) (Sharma et al., 2017). Prostaglandins (PGs) and particularly prostaglandin E2 (PGE2) play various complex roles in the manifestation and progression of CKD (Nasrallah et al., 2014; Sharma et al., 2017). In this context, COX2 (cyclooxygenase 2) and PGE2 activation contributes to impairment of the glomerular filtration barrier (GFB), i.e., foot process effacement of podocytes and slit diaphragm damage in albuminuria development (Srivastava et al., 2014). Furthermore, PGE2 signaling via the prostaglandin receptors (EP) 2 and EP4 is connected to podocyte responses during FFSS, and to kidney injury in the solitary kidney (Srivastava et al., 2014). Recently, we demonstrated a potential association between glomerular PGE2 accumulation and albuminuria development due to podocyte damage (Mangelsen et al., 2020). Thus, PGE2 degrading enzymes may play a critical role in the autocrine/paracrine COX2/PGE2 pathway in the development of albuminuria. Arachidonic acid is converted into PGE2 by cyclooxygenase enzymes 1 and 2 (COX1/COX2) and prostaglandin E synthases (PGES) (Smith, 1989; Park et al., 2006). These enzymes are expressed in all main cell types of the glomeruli (Sraer et al., 1979; Petrulis et al., 1981; Hirose et al., 1998; Harding et al., 2006; Xu et al., 2006; Yu et al., 2016; Ma et al., 2019; Yao et al., 2019). PGE2 is metabolized by 15-prostaglandin dehydrogenase (15-PGDH) to 15-keto-PGE2. The latter is terminally degraded by prostaglandin reductases PTGR1, PTGR2, and PTGR3 to 13,14-dihydro-15-keto-PGE2 (Figure 1A) (Tai et al., 2002; Wu et al., 2008; Yu et al., 2013; Chen et al., 2018).
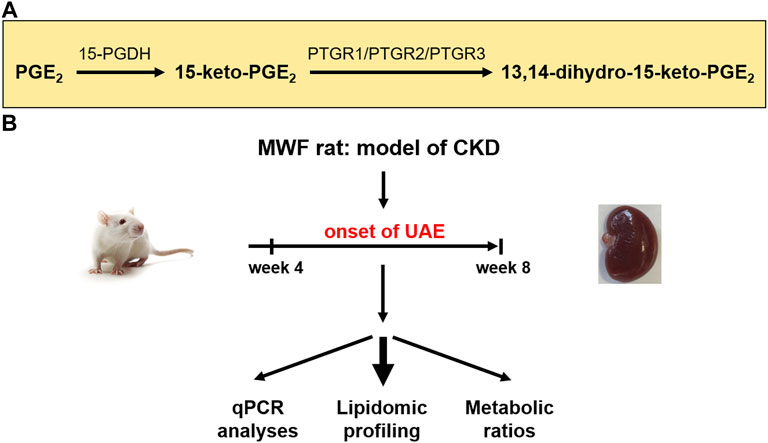
FIGURE 1. Study design for evaluation of prostaglandin metabolism in the chronic kidney disease (CKD) rat model Munich Wistar Frömter (MWF). (A) Schematic representation of enzymatic reduction of both prostaglandin E2 (PGE2) and 15-keto-PGE2 by prostaglandin dehydrogenase (15-PGDH) and prostaglandin reductases 1–3 (PTGR1-3) (Wu et al., 2008). (B) Young male MWF rats were studied between 4 weeks and 8 weeks of age and were compared to the reference strain spontaneously hypertensive rats (SHR). Therefore, lipidomic profiling and transcriptomic analysis of the PGE2 metabolic pathway were conducted. UAE = urinary albumin excretion.
To further investigate the renal PGE2 metabolic pathway we used the Munich Wistar Frömter (MWF) inbred rat strain as a suitable model for GH (Ulu et al., 2009; Schulz and Kreutz, 2012), which mirrors several phenotypes observed in patients with albuminuria and CKD. The glomerular hyperfiltration phenotype of MWF rats is due to an inherited nephron deficit with increased single nephron glomerular filtration rate and hemodynamically associated with dilatation of the afferent arteriole and normal glomerular capillary pressures (Remuzzi et al., 1988; Fassi et al., 1998) Overall glomerular filtration in young rats, as studied in this report is similar to normal rat strains (Rovira-Halbach et al., 1986; Fassi et al., 1998; van Es et al., 2011) MWF rats develop moderate hypertension, spontaneous albuminuria of early onset, and a congenital nephron deficit (Schulz and Kreutz, 2012). Podocyte damage with focal and segmental foot process effacement and a reduced podocyte number were early found in young adult MWF animals (Ijpelaar et al., 2008; Kourpa et al., 2023). With increasing age further significant structural renal abnormalities such as glomerulosclerosis and renal interstitial fibrosis with progressive albuminuria development were observed (Schulz and Kreutz, 2012).
So far, elevated plasma levels of circulating PGE2 were mostly measured by enzyme-linked immunosorbent assays (ELISA) and have been implicated in the MWF rat models of GH (Ulu et al., 2009). ELISA are often used despite their limitations including low specificity and selectivity as well as a lack of standardization across different ELISA kits (Faupel-Badger et al., 2010; Gandhi et al., 2017). Therefore, we previously developed a modified liquid chromatography electrospray ionization tandem mass spectrometry (LC/ESI-MS/MS) protocol that allows more precise quantification of PG levels (Mangelsen et al., 2020). Preliminary measurements in plasma and glomeruli of MWF rats at the onset of albuminuria suggest an implication of the PGE2 pathway for albuminuria development (Mangelsen et al., 2020). This supports the need to conduct comparative analysis of PGE2 and its downstream metabolites in more detail with state-of-the-art LC/ESI-MS/MS methodology in different kidney tissues and body fluids in the time window of albuminuria development in MWF rats (Figure 1B).
In this study, we aim to elucidate the PGE2 pathway in plasma, urine and glomerular tissue to clarify the impact of PGE2 metabolism in the context of GH during the onset of albuminuria.
2 Methods
2.1 Animals and phenotyping
Male MWF rats were obtained from our MWF/Rkb (RRID:RGD_724569, laboratory code Rkb, http://dels.nas.edu/ilar/). Male rats were used because a sexual dimorphism is conspicuous in the MWF strain with a more severe manifestation and progression of albuminuria and subsequent renal failure compared with females (Schulz and Kreutz, 2012; Herlan et al., 2015).
As a contrasting model we used male spontaneously hypertensive rats (SHR) from our SHR/Rkb (RRID:RGD_631696, laboratory code Rkb, http://dels.nas.edu/ilar/) colonies at the Charité—Universitätsmedizin Berlin, Germany (Schulz and Kreutz, 2012). SHR rats exhibit similar blood pressure values in early life (Schulz et al., 2003), but an albuminuria-resistant phenotype compared to MWF rats (Schulz and Kreutz, 2012).
Rats were grouped under conditions of regular 12 h diurnal cycles with an automated light switching device and climate-controlled conditions at a room temperature of 22°C. The rats were fed a normal diet containing 0.2% NaCl and had free access to food and water.
Urinary albumin excretion (UAE) was determined in metabolic cages for 24 h at 4 and 8 weeks of age as reported (n = 10–18, each) (Kreutz et al., 2000) and urinary creatinine levels (Supplementary Figure S1) were determined by the Jaffé method (Labor Berlin–Charité Vivantes GmbH, Berlin, Germany). Systolic blood pressure (SBP) was determined by a tail-cuff method in awake animals at 8 weeks of age (n = 5–8, each) using a computer-assisted oscillatory detection device (TSE, Bad Homburg, Germany) (Kreutz et al., 2000). Animals were sacrificed under ketamine-xylazine anesthesia (87 and 13 mg/kg body weight, respectively). For quantitative real-time PCR analysis (qPCR) and lipidomic profiling by LC/ESI-MS/MS (Mangelsen et al., 2020) cortex dissections of left kidneys, isolated glomeruli, plasma, and urine of MWF and SHR at both 4 and 8 weeks of age were snap-frozen and stored at −80°C.
2.2 Ethics approval statement
All experimental work in rats was performed in accordance with the guidelines of the Charité—Universitätsmedizin Berlin and the local authority for animal protection (Landesamt für Gesundheit und Soziales, Berlin, Germany) for the use of laboratory animals. The registration numbers for the rat experiments are G 0255/09, G 0309/19, G 0130/16, and T 0189/02.
2.3 Glomeruli and kidney cortex isolation
Different protocols were used for glomeruli isolation from rats at 4 and 8 weeks of age, due to the different body size (Schulz et al., 2019). Rats were anesthetized with ketamine-xylazine (87 and 13 mg/kg body weight, respectively). In 4-week-old rats, the abdominal artery was catheterized and kidneys were perfused with 10 mL 1x phosphate buffered saline (PBS) and subsequently with 20 mL ferrous solution 12.5 g ferric oxide [Iron (II/III) powder <5 micron, 98%; Sigma- Aldrich Chemie GmbH] suspended in 1,000 mL 1x PBS. Kidneys were removed and decapsulated. For cortical analysis the cortex of the left kidney was dissected and immediately snap-frozen and stored at 80°C. For glomeruli isolation decapsulated kidneys were passed through a 125 µm steel sieve (Retsch GmbH) with 1x PBS. The glomeruli containing ferrous particles were gathered by a magnet, snap-frozen and stored at −80°C. Kidneys of 8-week-old rats were removed, decapsulated and passed through a 125 µm steel sieve with 1x PBS. The filtrate was put on a 71 µm steel sieve (Retsch GmbH) to separate glomeruli from the flow-through. Glomeruli were washed off the sieve with 1x PBS, centrifuged, immediately snap-frozen and stored at −80°C.
2.4 Reverse transcription and qPCR
Isolated glomeruli preparations of rat strains were analyzed at week 4 and week 8 (n = 5–11, each). Total RNA of glomeruli preparations was isolated and DNase-treated by RNeasy Purification Kits (Qiagen), according to the manufacturer’s instructions. First-strand cDNA synthesis was carried out on 2 µg of total RNA using the First Strand cDNA Synthesis Kit (Fermentas Life Sciences) following the manufacturer’s protocol. qPCR analysis of mRNA expression of PGE2 degrading enzymes 15-Pgdh, Ptgr1, Ptgr2, and Ptgr3 was performed using the comparative quantification cycle method as reported (Schulz et al., 2019). In addition, different glomerular compartments in isolated glomeruli were evaluated by qPCR. We assessed alpha actinin 4 (Actn4) as a podocyte marker, platelet derived growth factor receptor beta (Pdgfrb) as a mesangial marker, and platelet endothelial cell adhesion molecule 1 (Pecam1, CD31) as an endothelial marker (Supplementary Figure S2). The extent of contamination with tubular material in isolated glomeruli was assessed by evaluating the tubular marker solute carrier family 5 member 1 (Slc5a1, Sglt1) (Supplementary Figure S2). Primers are listed in Table 1. Normalization of expression data was done by the reference gene hydroxymethylbilane synthase (Hmbs, Pbgd) (Schulz et al., 2008). All analyses were performed in three technical replicates for each animal or experiment (n = 5, each).
2.5 LC/ESI-MS/MS for prostaglandin profiling
2.5.1 Sample preparation
Sample preparation was described previously in detail (Mangelsen et al., 2020). Briefly, for lipidomic analyses 100 µL rat urine (n = 8–10 animals per strain, each) were spiked with an internal standard. 500 μL methanol and 5 µL 2,6-Di-tert-butyl-4-methylphenol (BHT 10 mg/mL) was added and shaked vigorously. The samples were brought to pH 6. After centrifugation, the obtained supernatant was added to the Bond Elute Certify II columns (Agilent Technologies) for Solid phase Extraction SPE, which were preconditioned with 3 mL methanol, followed by 3 mL of 0.1 mol/L phosphate buffer containing 5% methanol (pH 6). The SPE-columns were then washed with 3 mL methanol/H2O (40/50, vol/vol). For elution 2 mL of n-hexane: ethyl acetate 25:75 with 1% acetic acid was used. The eluate was evaporated on a heating block at 40 °C under a stream of nitrogen to obtain solids, which were dissolved in 100 µL methanol/water 60:40 and transferred in an HPLC autosampler vial. Plasma (n = 6–11 animals per strain, each) and isolated glomeruli (n = 6–10 animals per strain, each) were prepared as previously described (Mangelsen et al., 2020). For kidney cortex preparation (n = 6–9 animals per strain, each) approximately 5–20 mg of tissue was accurately weighed and homogenized with 500 µL water. 50 μL aliquots were taken for protein measurement following Lowry protocol (Lowry et al., 1951) with primary alkaline hydrolysis (Mangelsen et al., 2020). For alkaline hydrolysis, 50 µL tissue suspensions were mixed with 16 µL 10 mol/L sodium hydroxide solution and incubated for 1 hour at 60°C. The pH values were adjusted to pH = 10 using 58% acetic acid. For prostaglandin measurement, the tissue suspensions were added with internal standard and BHT and further processed like plasma.
2.5.2 LC/ESI-MS/MS
The residues were analyzed using the liquid chromatography tandem mass spectrometry protocol in an Agilent 1290 HPLC system with binary pump, multisampler and column thermostat with a Zorbax Eclipse plus C-18, 2.1 × 150 mm, 1.8 µm column using a solvent system of aqueous acetic acid (0.05%) and acetonitrile. The HPLC was coupled with an Agilent 6,495 Triplequad mass spectrometer (Agilent Technologies, Santa Clara, CA, United States) with electrospray ionisation source. Further details were given in Mangelsen et al. (2020) and Mangelsen et al. (2020). The analytes PGE2, 15-keto-PGE2, and 13,14-dihydro-15-keto-PGE2 were assessed. Glomerular and cortical values are normalized to protein, plasma values to ml of plasma and urinary values to creatinine. Analytes in isolated glomeruli and plasma in MWF rats and SHR at week 8, respectively, were recently published (Mangelsen et al., 2020).
2.5.3 Metabolic ratios
For determination of metabolic ratios the quotient of PGE2/15-keto-PGE2 was calculated as a surrogate for 15-PGDH enzyme activity and the quotient of 15-keto-PGE2/13,14-dihydro-15-keto-PGE2 was determined as a surrogate for PTGRs enzyme activities. Low values represent higher enzymatic conversions and high values lower enzymatic conversions.
2.6 Statistics
Statistical analysis was performed using the SPSS Statistics 28.0.0.0. Data are presented as mean ± SD, and p < 0.05 was considered as statistically significant. Normal distribution was tested with the Shapiro-Wilk test. Data are normally distributed unless otherwise specified. Normally distributed data were compared by ANOVA. Results not normally distributed were analyzed by Kruskal–Wallis test and by Mann-Whitney U test as indicated. For identification of outliers, Grubbs’ outliers test (α = 0.05) was performed.
3 Results
3.1 Rat phenotypic characteristics
MWF rats and the contrasting albuminuria resistant SHR strain showed both similar UAE levels below 0.33 mg/24 h at 4 weeks of age (Figure 2A). In contrast, UAE at week 8 was significantly higher in MWF rats compared to the reference strain (p < 0.0001). Mean SBP values were, albeit numerically higher in SHR, not significantly different between MWF rats vs. SHR (148.4 ± 6.0 mmHg vs. 158.0 ± 12.8 mmHg) at 8 weeks of age (Figure 2B).
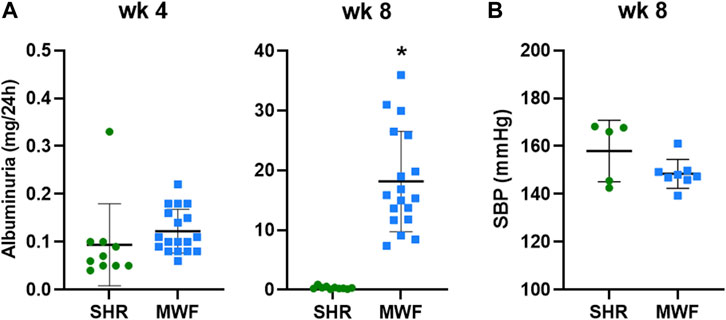
FIGURE 2. Phenotypic characteristics of Munich Wistar Frömter (MWF) and spontaneously hypertensive rats (SHR). (A) Urinary albumin excretion (UAE) at 4 and 8 weeks of age. MWF (n = 18), SHR (n = 10). (B) Systolic blood pressure (SBP) at 8 weeks of age. MWF (n = 8), SHR (n = 5). Analyte data were tested for normal distribution using Shapiro-Wilk test and analyzed by Mann-Whitney U test. Values shown as mean ± SD; *p < 0.0001 vs. SHR. UAE values were recently published (Schulz et al., 2019).
3.2 Lipidomic profile of PGE2 analytes and metabolic ratios
3.2.1 PGE2 in kidney tissue, plasma and urine
Glomerular levels of PGE2 were increased in MWF at week 4 and 8 (p < 0.006, respectively), whereas no difference for cortical levels was observed (Figure 3). Plasma levels of PGE2 did not differ between strains, while urinary PGE2 was significantly lower in MWF rats compared to SHR at both time points (p < 0.005, respectively; Figure 3).
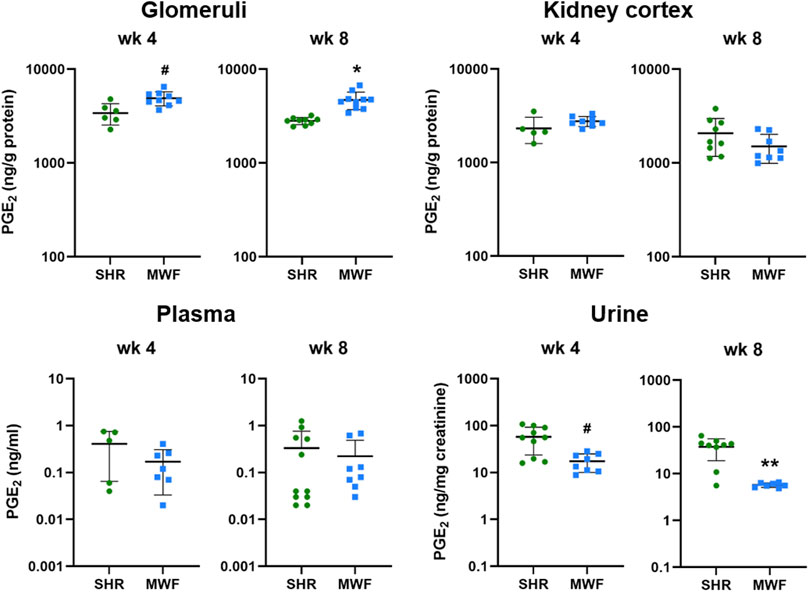
FIGURE 3. Metabolic levels of PGE2 in Munich Wistar Frömter (MWF) compared to spontaneously hypertensive rats (SHR). Analyte was assessed by LC/ESI-MS/MS in isolated glomeruli kidney cortex, plasma, and urine in 4 and 8 week old animals. n = 5–11 animals per rat strain. Data plotted as mean ± SD; logarithmic scale is used for visualization. *p < 0.0001, **p < 0.001, and #p < 0.02, respectively. Analyte data were tested for normal distribution using Shapiro-Wilk test. Normally distributed analytes were analyzed by one-way ANOVA, not normally distributed analytes by Kruskal–Wallis test and by Mann-Whitney U test.
3.2.2 15-keto-PGE2 and 13,14-dihydro-15-keto-PGE2 metabolites in kidney tissue, plasma and urine
Glomerular levels of 15-keto-PGE2 were significantly higher in MWF at week 4 and 8 (p < 0.006, respectively; Figure 4). In contrast, 13,14-dihydro-15-keto-PGE2 was only significantly higher at week 4 compared to SHR (p = 0.0071). In kidney cortex, 15-keto-PGE2 and 13,14-dihydro-15-keto-PGE2 were increased in MWF at week 4 (p < 0.0004, respectively), whereas no difference for cortical levels of PGE2 metabolites was observed at week 8 (Figure 4). Glomerular PGE2 levels were remarkably up to 150-fold higher than the respective 15-keto-PGE2 levels, whereas cortical PGE2 levels were even up to 200-fold higher than the corresponding 15-keto-PGE2 levels. Glomerular 13,14-dihydro-15-keto-PGE2 levels were elevated up to 15-fold compared to the 15-keto-PGE2 levels, whereas cortical levels were up to 46-fold higher than the corresponding 15-keto-PGE2 levels (Figures 3, 4).
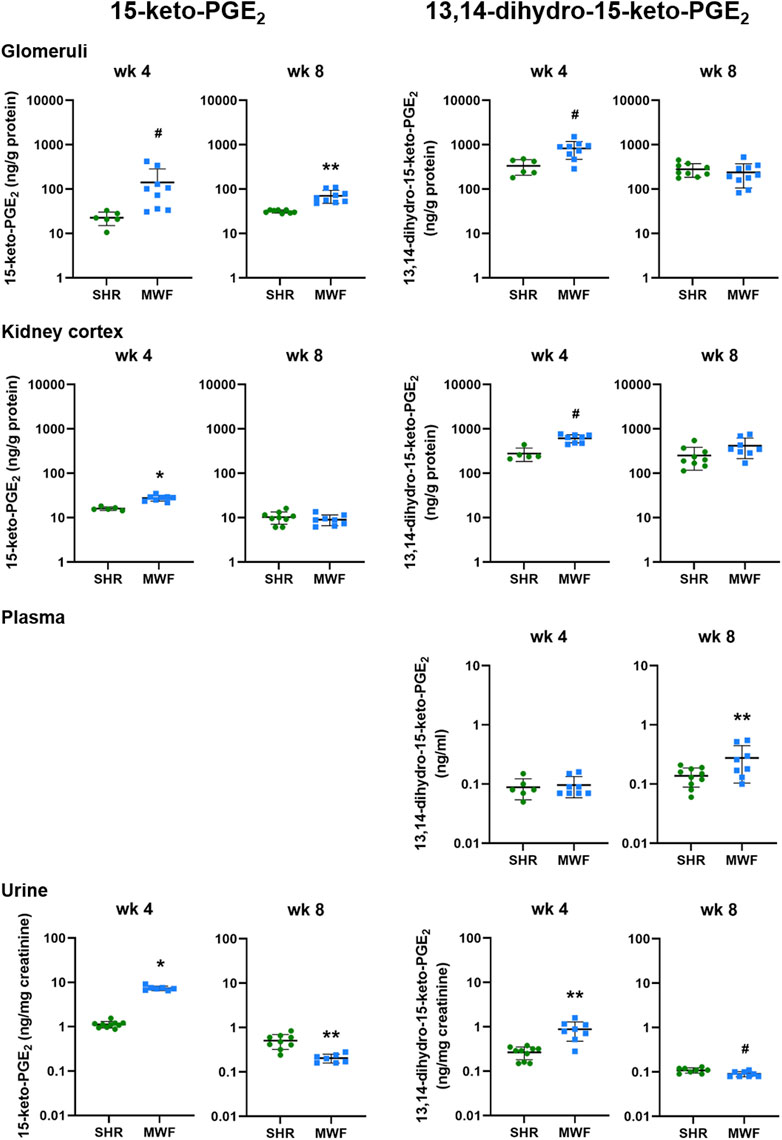
FIGURE 4. Metabolic levels of 15-keto-PGE2 and 13,14-dihydro-15-keto-PGE2 in Munich Wistar Frömter (MWF) rats compared to spontaneously hypertensive rats (SHR). Analytes were assessed by LC/ESI-MS/MS in isolated glomeruli kidney cortex, plasma, and urine in 4 and 8 week old animals. Plasma values for 15-keto-PGE2 were under the detection limit. n = 5–10 animals per rat strain. Data plotted as mean ± SD; logarithmic scale is used for visualization. *p < 0.0001, **p < 0.001, and #p < 0.02, respectively. Analyte data were tested for normal distribution using Shapiro-Wilk test. Normally distributed analytes were analyzed by one-way ANOVA, not normally distributed analytes by Kruskal–Wallis test and by Mann-Whitney U test.
Plasma levels of 13,14-dihydro-15-keto-PGE2 did not differ between strains at week 4 but significantly increased in MWF at week 8 (p < 0.03; Figure 4). 15-keto-PGE2 was below the detection limit in plasma.
Urinary 15-keto-PGE2 and 13,14-dihydro-15-keto-PGE2 levels were significantly increased in MWF rats compared to SHR at week 4 (p < 0.0005, respectively; Figure 4). Both analytes were significantly decreased in urine of MWF with increased albuminuria at week 8 (p < 0.02 vs. SHR, respectively; Figures 2, 4).
3.2.3 Glomerular metabolic ratios of 15-PGDH and PTGRs
Glomerular metabolic ratio of PGE2/15-keto-PGE2 as a surrogate for 15-PGDH enzyme activity showed significantly lower metabolic ratios in MWF compared to SHR at week 4 and week 8 (p < 0.006, respectively; Figure 5). Metabolic ratios of 15-keto-PGE2/13,14-dihydro-15-keto-PGE2 as a surrogate for PTGRs enzyme activities were similar between the strains at week 4, but significantly increased in MWF compared to SHR at week 8 (p < 0.0001, respectively; Figure 5).
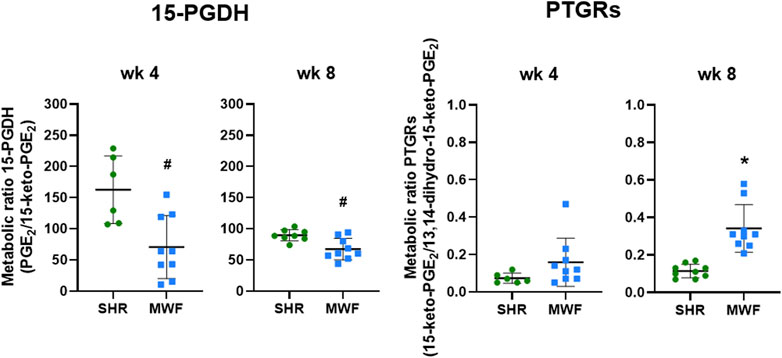
FIGURE 5. Metabolic ratios of 15-hydroxyprostaglandin dehydrogenase (15-PGDH) and prostaglandin reductases (PTGRs) in isolated glomeruli of Munich Wistar Frömter (MWF) rats compared to spontaneously hypertensive rats (SHR). Metabolic ratios of PGE2/15-keto-PGE2 were calculated as a surrogate for 15-PGDH activity and metabolic ratios of 15-keto-PGE2/13,14-dihydro-15-keto-PGE2 were assessed as a surrogate for PTGRs activities at 4 and 8 weeks of age. Values were plotted as mean ± SD. *p < 0.0001, #p < 0.04, respectively. Data were tested by one-way ANOVA.
3.3 Glomerular mRNA expression analysis of PGE2 degrading enzymes
mRNA expression of Ptgr1, Ptgr2, and Ptgr3 demonstrated significantly lower expression levels in MWF rats as compared to SHR at both time points (p < 0.02, respectively; Figure 6). In contrast, 15-Pgdh mRNA expression was similar between the strains.
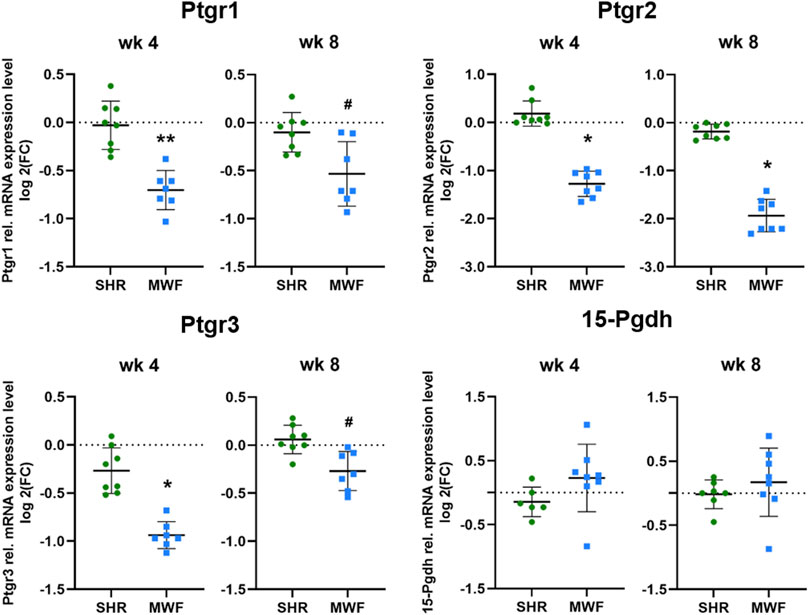
FIGURE 6. Targeted gene expression analyses of prostaglandin E2 (PGE2) degrading enzymes. Quantitative real-time PCR (qPCR) was performed for prostaglandin reductase (Ptgr)1-3 and 15-hydroxyprostaglandin dehydrogenase (15-Pgdh) in isolated glomeruli tissue. Ptgr1-3 showed significantly reduced expression values in Munich Wistar Frömter (MWF) compared to spontaneously hypertensive rats (SHR) at 4 and 8 weeks of age. 15-Pgdh was similar between the strains. n = 6–8, each; values are plotted as mean ± SD. *p < 0.0001, **p < 0.001, and #p < 0.02, respectively. Gene data were tested for normal distribution using Shapiro-Wilk test: all genes were normally distributed and were analyzed by one-way ANOVA. Quantitative mRNA levels were normalized by the reference gene hydroxymethylbilane synthase (Hmbs, Pbgd).
4 Discussion
Within the different signaling mechanisms of PGs in the kidney, PGE2 increases GFB permeability (Nasrallah et al., 2014) and its upregulation in podocytes is linked to a response to increased FFSS as observed during GH (Srivastava et al., 2010; Srivastava et al., 2020). Moreover, PGE2 upregulation in podocytes also associates with actin cytoskeleton rearrangement (Martineau et al., 2004) and the latter contributes to podocyte foot process effacement (Kaplan et al., 2000) and slit diaphragm damage (Tryggvason and Wartiovaara, 2005), which is crucial for albuminuria development. A pathogenic role of signaling via the EP2 and possibly with lesser relevance via EP4 has been also suggested (Penn et al., 2001). We recently demonstrated in a yeast model that PGE2 and its downstream metabolite 15-keto-PGE2 bind to both EP2 and EP4 in vitro (Kourpa et al., 2022). Moreover, we showed in lipidomic analyses by LC/ESI-MS/MS that concerted EP2 and EP4 signaling mediates autocrine PGE2 signaling in human podocytes (Mangelsen et al., 2020). In a diabetic mouse model, expression of EP4 was detected in glomeruli and EP4 blockade could significantly ameliorated albuminuria development (Guan et al., 2022). Moreover, selective EP4 blockade is protective in a model of subtotal nephrectomy (Thieme et al., 2017). In contrast, inhibition of EP2 and activation of EP4 has the strongest effect in decreasing albuminuria in a hyperfiltration-induced injury mouse model with unilaterally nephrectomy (Srivastava et al., 2022). In the non-diabetic MWF rat model of CKD with GH, we showed a renoprotective effect of combined EP2/EP4 receptor inhibition of the COX2-PGE2-EP2/EP4 axis, since dual receptor blockade during onset of albuminuria development ameliorated albuminuria in this model, while systemic arterial blood pressure and GFR were not affected (Kourpa et al., 2023). Taken together, EP2 and EP4 receptors are potential targets for therapeutical intervention in hyperfiltration-induced glomerular injury.
15-keto-PGE2 and 13,14-dihydro-15-keto-PGE2 have been considered biologically inactive for a long time. Recently, a bioactive role of 15-keto-PGE2 has been identified and its signaling through activation of the peroxisome proliferator activated receptor gamma (PPAR-γ) pathway investigated (Chou et al., 2007; Lu et al., 2014; Chang et al., 2016; Chen et al., 2018). Effects of 15-keto-PGE2 are mediated via EP2 and EP4 receptors in vitro and in vivo (Endo et al., 2020; Kourpa et al., 2022). Previously, it has been shown that 15-keto-PGE2 affects the glomerular morphology of zebrafish embryonic kidney (Kourpa et al., 2023). However, the activity of PGE2 degrading enzymes and thus the metabolic pathway of COX2-PGE2 have not yet been implicated in renal physiology nor their potential contribution to the initiation and/or progression of CKD, i.e., albuminuria (Nasrallah et al., 2014; Srivastava et al., 2014; Chen et al., 2018). This indicates the need to analyze the metabolic downstream pathway of COX2-PGE2 in more detail. To this end we used the LC/ESI-MS/MS methodology for exact quantification in different kidney tissues and body fluids in the time window of albuminuria development in the MWF model system.
Our lipidomic profiling revealed elevated glomerular PGE2 levels, which were accompanied with reduced urinary PGE2 levels in MWF rats compared to SHR (Figure 3). In contrast, elevated urinary PGE2 levels were previously linked to glomerular injury and considered as a potential biomarker for early stages of adaptive hyperfiltration-induced injury preceding albuminuria in children (Srivastava et al., 2014; Srivastava et al., 2020). Notable, urinary 13-14-dihydro-15-keto-PGE2 levels were downregulated in an unilateral nephrectomized mouse model for GH with albuminuria reduction due to EP2 antagonist and EP4 agonist treatment (Srivastava et al., 2022). It should be however noted here, that the evaluation of urinary differences of PG levels are difficult to interpret. Hence, the origin of PGs in urine is unclear, since it is not possible to dissect which fractions of PGs are possibly generated in the tubular/tissue compartment and how much is attributable to glomerular filtration. However, to clarify this, further investigations including lipidomic analysis of PGE2 metabolites in tubular compartment could complement our findings.
Differences between glomerular and cortical PGE2 analytes highlight the importance of choosing the right compartment, i.e., glomerular tissue, when addressing glomerular questions. We detected elevated PGE2 levels in isolated glomeruli of MWF rats at both investigated time points compared to SHR. Cortical analyses did not show those significant changes between rat strains emphasizing the need to isolate glomeruli. We observed remarkably higher glomerular and cortical PGE2 levels than the respective 15-keto-PGE2 levels at both time points and elevated 13-14-dihydro-15-keto-PGE2 levels compared to the 15-keto-PGE2 levels, respectively. Hence, we conclude that the PTGRs are not the rate-limiting enzymes in the PGE2 degradation and inactivation in the kidney, rather 15-PGDH seems to be rate limiting in this process.
In contrast to the analysis of glomerular tissue, lipidomic analysis in plasma was not informative when comparing MWF rats and SHR during onset of albuminuria development. Comparing these results with previously reported PGE2 plasma levels in older MWF rats as measured by ELISA technique (Ulu et al., 2009), we measured almost four times lower average PGE2 plasma level. This discrepancy emphasizes the importance to use sensitive and precise state-of-the-art LC/ESI-MS/MS methodology to measure PGE2 lipids. Mass spectrometric analyses are preferable due to their higher specificity and selectivity compared to ELISA and standardized measurement procedures are lacking within different ELISA kits (Faupel-Badger et al., 2010; Gandhi et al., 2017). In conclusion, we observed a dysregulation of glomerular PGs but no dysregulation in plasma levels.
Metabolic ratios as a surrogate for enzyme activity revealed reduced glomerular 15-PGDH activity in MWF compared to SHR (Figure 5), while glomerular mRNA expression of 15-Pgdh was similar between strains. The increased glomerular PGE2 values in MWF rats could be thus at least partially due to the lower 15-PGDH activity and a reduced PGE2 degradation.
In summary, our study highlights the importance of utilizing sensitive mass spectrometry technology for PG measurement in body liquids and tissues. Furthermore, we emphasized the importance of investigating the PGE2 pathway in kidney tissues and more precisely in glomeruli as analysis of plasma and urine samples have limiting relevance here. We demonstrated for the first-time age-dependent dynamic changes in the PGE2 metabolic pathway, which is involved in GH in the MWF rat model. Enzymatic functions of PGE2 degrading enzymes support a potential causative mechanism for kidney physiology and albuminuria onset in the setting of GH.
Data availability statement
All relevant data is contained within the article: The original contributions presented in the study are included in the article/supplementary material, further inquiries can be directed to the corresponding author.
Ethics statement
The animal study was approved by the Landesamt für Gesundheit und Soziales Berlin, Germany. The study was conducted in accordance with the local legislation and institutional requirements.
Author contributions
DK-G: Conceptualization, Data curation, Formal Analysis, Supervision, Validation, Visualization, Writing–original draft, Writing–review and editing. AS: Conceptualization, Data curation, Formal Analysis, Investigation, Methodology, Supervision, Validation, Visualization, Writing–original draft, Writing–review and editing. EM: Data curation, Investigation, Methodology, Writing–review and editing. MR: Data curation, Investigation, Methodology, Writing–review and editing. JB: Supervision, Writing–review and editing. RK: Conceptualization, Funding acquisition, Project administration, Resources, Supervision, Writing–review and editing.
Funding
The author(s) declare financial support was received for the research, authorship, and/or publication of this article. This research was funded by the Deutsche Forschungsgemeinschaft (DFG)—Project number 394046635—SFB 1365.
Acknowledgments
We acknowledge the contributions of Claudia Plum, Karen Böhme, and Bettina Bublath for excellent laboratory or animal assistance.
Conflict of interest
Author MR was employed by Lipidomix GmbH.
The remaining authors declare that the research was conducted in the absence of any commercial or financial relationships that could be construed as a potential conflict of interest.
Publisher’s note
All claims expressed in this article are solely those of the authors and do not necessarily represent those of their affiliated organizations, or those of the publisher, the editors and the reviewers. Any product that may be evaluated in this article, or claim that may be made by its manufacturer, is not guaranteed or endorsed by the publisher.
Supplementary material
The Supplementary Material for this article can be found online at: https://www.frontiersin.org/articles/10.3389/fnetp.2023.1271042/full#supplementary-material
References
Carrero, J. J., Grams, M. E., Sang, Y., Arnlov, J., Gasparini, A., Matsushita, K., et al. (2017). Albuminuria changes are associated with subsequent risk of end-stage renal disease and mortality. Kidney Int. 91 (1), 244–251. doi:10.1016/j.kint.2016.09.037
Chang, E. Y., Chang, Y. C., Shun, C. T., Tien, Y. W., Tsai, S. H., Hee, S. W., et al. (2016). Inhibition of prostaglandin reductase 2, a putative oncogene overexpressed in human pancreatic adenocarcinoma, induces oxidative stress-mediated cell death involving xCT and CTH gene expressions through 15-keto-PGE2. PLoS One 11 (1), e0147390. doi:10.1371/journal.pone.0147390
Chen, I. J., Hee, S. W., Liao, C. H., Lin, S. Y., Su, L., Shun, C. T., et al. (2018). Targeting the 15-keto-PGE2-PTGR2 axis modulates systemic inflammation and survival in experimental sepsis. Free Radic. Biol. Med. 115, 113–126. doi:10.1016/j.freeradbiomed.2017.11.016
Chou, W. L., Chuang, L. M., Chou, C. C., Wang, A. H., Lawson, J. A., FitzGerald, G. A., et al. (2007). Identification of a novel prostaglandin reductase reveals the involvement of prostaglandin E2 catabolism in regulation of peroxisome proliferator-activated receptor gamma activation. J. Biol. Chem. 282 (25), 18162–18172. doi:10.1074/jbc.M702289200
Endo, S., Suganami, A., Fukushima, K., Senoo, K., Araki, Y., Regan, J. W., et al. (2020). 15-Keto-PGE(2) acts as a biased/partial agonist to terminate PGE(2)-evoked signaling. J. Biol. Chem. 295 (38), 13338–13352. doi:10.1074/jbc.RA120.013988
Fassi, A., Sangalli, F., Maffi, R., Colombi, F., Mohamed, E. I., Brenner, B. M., et al. (1998). Progressive glomerular injury in the MWF rat is predicted by inborn nephron deficit. J. Am. Soc. Nephrol. 9 (8), 1399–1406. doi:10.1681/ASN.V981399
Faupel-Badger, J. M., Fuhrman, B. J., Xu, X., Falk, R. T., Keefer, L. K., Veenstra, T. D., et al. (2010). Comparison of liquid chromatography-tandem mass spectrometry, RIA, and ELISA methods for measurement of urinary estrogens. Cancer Epidemiol. Biomarkers Prev. 19 (1), 292–300. doi:10.1158/1055-9965.Epi-09-0643
Gandhi, A. S., Budac, D., Khayrullina, T., Staal, R., and Chandrasena, G. (2017). Quantitative analysis of lipids: a higher-throughput LC-MS/MS-based method and its comparison to ELISA. Future Sci. OA 3 (1), Fso157. doi:10.4155/fsoa-2016-0067
Guan, Y., Davis, L., Breyer, M. D., and Hao, C. M. (2022). Cyclooxygenase-2 contributes to diabetic nephropathy through glomerular EP4 receptor. Prostagl. Other Lipid Mediat 159, 106621. doi:10.1016/j.prostaglandins.2022.106621
Harding, P., Balasubramanian, L., Swegan, J., Stevens, A., and Glass, W. F. (2006). Transforming growth factor beta regulates cyclooxygenase-2 in glomerular mesangial cells. Kidney Int. 69 (9), 1578–1585. doi:10.1038/sj.ki.5000323
Herlan, L., Unland, J., Langer, S., Schulte, L., Schutten, S., Garcia-Prieto, C. F., et al. (2015). Development of progressive albuminuria in male Munich Wistar Fromter rats is androgen dependent. Physiol. Genomics 47 (7), 281–289. doi:10.1152/physiolgenomics.00008.2015
Hirose, S., Yamamoto, T., Feng, L., Yaoita, E., Kawasaki, K., Goto, S., et al. (1998). Expression and localization of cyclooxygenase isoforms and cytosolic phospholipase A2 in anti-Thy-1 glomerulonephritis. J. Am. Soc. Nephrol. 9 (3), 408–416. doi:10.1681/asn.V93408
Ijpelaar, D. H., Schulz, A., Koop, K., Schlesener, M., Bruijn, J. A., Kerjaschki, D., et al. (2008). Glomerular hypertrophy precedes albuminuria and segmental loss of podoplanin in podocytes in Munich-Wistar-Fromter rats. Am. J. Physiol. Ren. Physiol. 294 (4), F758–F767. doi:10.1152/ajprenal.00457.2007
Kaplan, J. M., Kim, S. H., North, K. N., Rennke, H., Correia, L. A., Tong, H. Q., et al. (2000). Mutations in ACTN4, encoding alpha-actinin-4, cause familial focal segmental glomerulosclerosis. Nat. Genet. 24 (3), 251–256. doi:10.1038/73456
Kourpa, A., Kaiser-Graf, D., Sporbert, A., Philippe, A., Catar, R., Rothe, M., et al. (2022). 15-keto-Prostaglandin E(2) exhibits bioactive role by modulating glomerular cytoarchitecture through EP2/EP4 receptors. Life Sci. 310, 121114. doi:10.1016/j.lfs.2022.121114
Kourpa, A., Schulz, A., Mangelsen, E., Kaiser-Graf, D., Koppers, N., Stoll, M., et al. (2023). Studies in zebrafish and rat models support dual blockade of EP2 and EP4 (prostaglandin E(2) receptors type 2 and 4) for renoprotection in glomerular hyperfiltration and albuminuria. Hypertension 80 (4), 771–782. doi:10.1161/hypertensionaha.122.20392
Kreutz, R., Kovacevic, L., Schulz, A., Rothermund, L., Ketteler, M., and Paul, M. (2000). Effect of high NaCl diet on spontaneous hypertension in a genetic rat model with reduced nephron number. J. Hypertens. 18 (6), 777–782. doi:10.1097/00004872-200018060-00017
Lowry, O. H., Rosebrough, N. J., Farr, A. L., and Randall, R. J. (1951). Protein measurement with the Folin phenol reagent. J. Biol. Chem. 193 (1), 265–275. doi:10.1016/s0021-9258(19)52451-6
Lu, D., Han, C., and Wu, T. (2014). 15-PGDH inhibits hepatocellular carcinoma growth through 15-keto-PGE2/PPARγ-mediated activation of p21WAF1/Cip1. Oncogene 33 (9), 1101–1112. doi:10.1038/onc.2013.69
Ma, K. L., Liu, L., Zhang, Y., Wang, G. H., Hu, Z. B., Chen, P. P., et al. (2019). Aspirin attenuates podocyte injury in diabetic rats through overriding cyclooxygenase-2-mediated dysregulation of LDL receptor pathway. Int. Urol. Nephrol. 51 (3), 551–558. doi:10.1007/s11255-018-2059-7
Mangelsen, E., Rothe, M., Schulz, A., Kourpa, A., Panáková, D., Kreutz, R., et al. (2020). Concerted EP2 and EP4 receptor signaling stimulates autocrine prostaglandin E2 activation in human podocytes. Cells 9 (5), 1256. doi:10.3390/cells9051256
Martineau, L. C., McVeigh, L. I., Jasmin, B. J., and Kennedy, C. R. (2004). p38 MAP kinase mediates mechanically induced COX-2 and PG EP4 receptor expression in podocytes: implications for the actin cytoskeleton. Am. J. Physiol. Ren. Physiol. 286 (4), F693–F701. doi:10.1152/ajprenal.00331.2003
Nasrallah, R., Hassouneh, R., and Hebert, R. L. (2014). Chronic kidney disease: targeting prostaglandin E2 receptors. Am. J. Physiol. Ren. Physiol. 307 (3), F243–F250. doi:10.1152/ajprenal.00224.2014
Park, J. Y., Pillinger, M. H., and Abramson, S. B. (2006). Prostaglandin E2 synthesis and secretion: the role of PGE2 synthases. Clin. Immunol. 119 (3), 229–240. doi:10.1016/j.clim.2006.01.016
Penn, R. B., Pascual, R. M., Kim, Y. M., Mundell, S. J., Krymskaya, V. P., Panettieri, R. A., et al. (2001). Arrestin specificity for G protein-coupled receptors in human airway smooth muscle. J. Biol. Chem. 276 (35), 32648–32656. doi:10.1074/jbc.M104143200
Petrulis, A. S., Aikawa, M., and Dunn, M. J. (1981). Prostaglandin and thromboxane synthesis by rat glomerular epithelial cells. Kidney Int. 20 (4), 469–474. doi:10.1038/ki.1981.163
Remuzzi, A., Puntorieri, S., Mazzoleni, A., and Remuzzi, G. (1988). Sex related differences in glomerular ultrafiltration and proteinuria in Munich-Wistar rats. Kidney Int. 34 (4), 481–486. doi:10.1038/ki.1988.206
Rovira-Halbach, G., Alt, J. M., Brunkhorst, R., Frei, U., Kuhn, K., and Stolte, H. (1986). Single nephron hyperfiltration and proteinuria in a newly selected rat strain with superficial glomeruli. Ren. Physiol. 9 (6), 317–325. doi:10.1159/000173097
Schulz, A., and Kreutz, R. (2012). Mapping genetic determinants of kidney damage in rat models. Hypertens. Res. 35 (7), 675–694. doi:10.1038/hr.2012.77
Schulz, A., Muller, N. V., van de Lest, N. A., Eisenreich, A., Schmidbauer, M., Barysenka, A., et al. (2019). Analysis of the genomic architecture of a complex trait locus in hypertensive rat models links Tmem63c to kidney damage. Elife 8, e42068. doi:10.7554/eLife.42068
Schulz, A., Schlesener, M., Weiss, J., Hänsch, J., Wendt, N., Kossmehl, P., et al. (2008). Protective effect of female gender on the development of albuminuria in a polygenetic rat model is enhanced further by replacement of a major autosomal QTL. Clin. Sci. (Lond) 114 (4), 305–311. doi:10.1042/CS20070300
Schulz, A., Standke, D., Kovacevic, L., Mostler, M., Kossmehl, P., Stoll, M., et al. (2003). A major gene locus links early onset albuminuria with renal interstitial fibrosis in the MWF rat with polygenetic albuminuria. J. Am. Soc. Nephrol. 14 (12), 3081–3089. doi:10.1097/01.asn.0000100126.62370.25
Sharma, M., Sharma, R., McCarthy, E. T., Savin, V. J., and Srivastava, T. (2017). Hyperfiltration-associated biomechanical forces in glomerular injury and response: potential role for eicosanoids. Prostagl. Other Lipid Mediat 132, 59–68. doi:10.1016/j.prostaglandins.2017.01.003
Smith, W. L. (1989). The eicosanoids and their biochemical mechanisms of action. Biochem. J. 259 (2), 315–324. doi:10.1042/bj2590315
Sraer, J., Foidart, J., Chansel, D., Mahieu, P., Kouznetzova, B., and Ardaillou, R. (1979). Prostaglandin synthesis by mesangial and epithelial glomerular cultured cells. FEBS Lett. 104 (2), 420–424. doi:10.1016/0014-5793(79)80866-2
Srivastava, T., Alon, U. S., Cudmore, P. A., Tarakji, B., Kats, A., Garola, R. E., et al. (2014). Cyclooxygenase-2, prostaglandin E2, and prostanoid receptor EP2 in fluid flow shear stress-mediated injury in the solitary kidney. Am. J. Physiol. Ren. Physiol. 307 (12), F1323–F1333. doi:10.1152/ajprenal.00335.2014
Srivastava, T., Garola, R. E., Zhou, J., Boinpelly, V. C., Priya, L., Ali, M. F., et al. (2022). Prostanoid receptors in hyperfiltration-mediated glomerular injury: novel agonists and antagonists reveal opposing roles for EP2 and EP4 receptors. Faseb J. 36 (10), e22559. doi:10.1096/fj.202200875R
Srivastava, T., Ju, W., Milne, G. L., Rezaiekhaligh, M. H., Staggs, V. S., Alon, U. S., et al. (2020). Urinary prostaglandin E(2) is a biomarker of early adaptive hyperfiltration in solitary functioning kidney. Prostagl. Other Lipid Mediat 146, 106403. doi:10.1016/j.prostaglandins.2019.106403
Srivastava, T., McCarthy, E. T., Sharma, R., Cudmore, P. A., Sharma, M., Johnson, M. L., et al. (2010). Prostaglandin E(2) is crucial in the response of podocytes to fluid flow shear stress. J. Cell Commun. Signal 4 (2), 79–90. doi:10.1007/s12079-010-0088-9
Tai, H. H., Ensor, C. M., Tong, M., Zhou, H., and Yan, F. (2002). Prostaglandin catabolizing enzymes. Prostagl. Other Lipid Mediat 68-69, 483–493. doi:10.1016/s0090-6980(02)00050-3
Thieme, K., Majumder, S., Brijmohan, A. S., Batchu, S. N., Bowskill, B. B., Alghamdi, T. A., et al. (2017). EP4 inhibition attenuates the development of diabetic and non-diabetic experimental kidney disease. Sci. Rep. 7 (1), 3442. doi:10.1038/s41598-017-03237-3
Tryggvason, K., and Wartiovaara, J. (2005). How does the kidney filter plasma? Physiol. (Bethesda) 20, 96–101. doi:10.1152/physiol.00045.2004
Ulu, N., Schoemaker, R. G., Henning, R. H., Buikema, H., Teerlink, T., Zijlstra, F. J., et al. (2009). Proteinuria-associated endothelial dysfunction is strain dependent. Am. J. Nephrol. 30 (3), 209–217. doi:10.1159/000218062
Untergasser, A., Cutcutache, I., Koressaar, T., Ye, J., Faircloth, B. C., Remm, M., et al. (2012). Primer3 - new capabilities and interfaces. Nucleic Acids Res. 40 (15), e115. doi:10.1093/nar/gks596
van Es, N., Schulz, A., Ijpelaar, D., van der Wal, A., Kuhn, K., Schütten, S., et al. (2011). Elimination of severe albuminuria in aging hypertensive rats by exchange of 2 chromosomes in double-consomic rats. Hypertension 58 (2), 219–224. doi:10.1161/HYPERTENSIONAHA.111.170621
Wu, Y. H., Ko, T. P., Guo, R. T., Hu, S. M., Chuang, L. M., and Wang, A. H. (2008). Structural basis for catalytic and inhibitory mechanisms of human prostaglandin reductase PTGR2. Structure 16 (11), 1714–1723. doi:10.1016/j.str.2008.09.007
Xu, Z. G., Li, S. L., Lanting, L., Kim, Y. S., Shanmugam, N., Reddy, M. A., et al. (2006). Relationship between 12/15-lipoxygenase and COX-2 in mesangial cells: potential role in diabetic nephropathy. Kidney Int. 69 (3), 512–519. doi:10.1038/sj.ki.5000137
Yao, L., He, J., Li, B., Yan, M., Wang, H., Tan, L., et al. (2019). Regulation of YAP by mammalian target of rapamycin complex 1 in endothelial cells controls blood pressure through COX-2/mPGES-1/PGE(2) cascade. Hypertension 74 (4), 936–946. doi:10.1161/hypertensionaha.119.12834
Yu, J., Gong, W., Wu, Y., Li, S., Cui, Y., Ma, Y., et al. (2016). mPGES-1-derived PGE2 contributes to adriamycin-induced podocyte injury. Am. J. Physiol. Ren. Physiol. 310 (6), F492–F498. doi:10.1152/ajprenal.00499.2015
Keywords: albuminuria, glomerular filtration, glomerular hyperfiltration, chronic kidney disease, lipidomic profiling, prostaglandin E2 pathway, Munich Wistar Frömter rats
Citation: Kaiser-Graf D, Schulz A, Mangelsen E, Rothe M, Bolbrinker J and Kreutz R (2023) Tissue lipidomic profiling supports a mechanistic role of the prostaglandin E2 pathway for albuminuria development in glomerular hyperfiltration. Front. Netw. Physiol. 3:1271042. doi: 10.3389/fnetp.2023.1271042
Received: 01 August 2023; Accepted: 21 November 2023;
Published: 22 December 2023.
Edited by:
Niels-Henrik Holstein-Rathlou, University of Copenhagen, DenmarkReviewed by:
Roberto Boi, University of Gothenburg, SwedenMukut Sharma, KCVA Medical Center, United States
Jan Michael Williams, University of Mississippi Medical Center School of Dentistry, United States
Copyright © 2023 Kaiser-Graf, Schulz, Mangelsen, Rothe, Bolbrinker and Kreutz. This is an open-access article distributed under the terms of the Creative Commons Attribution License (CC BY). The use, distribution or reproduction in other forums is permitted, provided the original author(s) and the copyright owner(s) are credited and that the original publication in this journal is cited, in accordance with accepted academic practice. No use, distribution or reproduction is permitted which does not comply with these terms.
*Correspondence: Angela Schulz, YW5nZWxhLW1hcnRpbmEuc2NodWx6QGNoYXJpdGUuZGU=