- 1Translational Hepatology, Department of Biomedicine, University of Basel, Basel, Switzerland
- 2Department of Biomedicine, University of Basel and University Centre for Gastrointestinal and Liver Diseases, Basel, Switzerland
Cirrhosis of the liver is a systemic condition with raising prevalence worldwide. Patients with cirrhosis are highly susceptible to develop bacterial infections leading to acute decompensation and acute-on-chronic liver failure both associated with a high morbidity and mortality and sparse therapeutic options other than transplantation. Mononuclear phagocytes play a central role in innate immune responses and represent a first line of defence against pathogens. Their function includes phagocytosis, killing of bacteria, antigen presentation, cytokine production as well as recruitment and activation of immune effector cells. Liver injury and development of cirrhosis induces activation of liver resident Kupffer cells and recruitment of monocytes to the liver. Damage- and pathogen-associated molecular patterns promote systemic inflammation which involves multiple compartments besides the liver, such as the circulation, gut, peritoneal cavity and others. The function of circulating monocytes and tissue macrophages is severely impaired and worsens along with cirrhosis progression. The underlying mechanisms are complex and incompletely understood. Recent ‘omics’ technologies help to transform our understanding of cellular diversity and function in health and disease. In this review we point out the current state of knowledge on phenotypical and functional changes of monocytes and macrophages during cirrhosis evolution in different compartments and their role in disease progression. We also discuss the value of potential prognostic markers for cirrhosis-associated immuneparesis, and future immunotherapeutic strategies that may reduce the need for transplantation and death.
1 Introduction
Cirrhosis of the liver is a global health burden with increasing prevalence and a high morbidity and mortality causing more than one million deaths per year worldwide (Mokdad et al., 2014; Tsochatzis et al., 2014). Cirrhosis represents a multisystemic disease, that not only involves the liver, but affects multiple organs, diverse immune cells and soluble factors (Ber nsmeier et al., 2020). Chronic liver injury is most frequently caused by alcohol use, nutritive fat accumulation and viral infections and associated with the activation and alteration of liver resident and infiltrating immune cells. It leads to replacement of healthy parenchyma by fibrotic tissue. This results in progressive fibrosis, disrupted liver architecture, aberrant regeneration, inflammation and vascular changes (Marcellin and Kutala, 2018). These processes moreover lead to portal hypertension (PH), an increase in the pressure within the portal vein, which carries blood from the digestive organs to the liver, and porto-systemic shunting, and results in pathological bacterial translocation (BT) (D'Amico et al., 2018; Mandorfer et al., 2020). The clinical course of cirrhosis when patients progress from compensated to decompensated cirrhosis relates to adverse prognosis. In compensated mostly asymptomatic cirrhosis mortality remains low, but dramatically increases in decompensated cirrhosis when ascites, variceal bleeding, bacterial infections, and organ failure develop (Arvaniti et al., 2010; D'Amico et al., 2006). Therapeutic options are limited to the treatment of the underlying cause of cirrhosis and in end stage liver disease transplantation remains the last option (Asrani et al., 2019). Donor organs are limited and don’t meet the demand, therefore the development of new effective therapies for cirrhosis patients preventing disease progression could improve clinical outcomes. Immune dysfunction seen in cirrhosis starts with the onset of chronic inflammation originating from damage associated molecular patters (DAMPs) released from the injured liver, and worsens with systemic pathogen associated molecular pattern (PAMP) exposure due to pathological BT. The underlying mechanisms remain incompletely understood, severe immune exhaustion develops following factors precipitating acute decompensation (AD) or acute-on-chronic liver failures (ACLF), mainly infection (Moreau et al., 2013). The liver is a tolerogenic organ with antimicrobial surveillance function between the portal and systemic circulation, governed by innate, and amplified by adaptive immunity. A better understanding of monocyte and macrophages dysfunction at a molecular level is required in order to evaluate potential immunotherapeutic approaches for the future treatment of cirrhosis.
In this article we aim to point out the importance of monocytes and macrophages in the context of cirrhosis. We review the current state of knowledge which mechanisms contribute to the progressing immune dysfunction from early asymptomatic stages to end-stage liver disease by dissecting the phenotypical and functional changes of monocytes and macrophages from patients with cirrhosis in different compartments. In the following we 1) summarise the current concept of the pathophysiology of cirrhosis as systemic inflammatory condition, 2) dissect the differentiation of monocytes and macrophages in context of cirrhosis and 3) discuss potential biomarkers and potential future immunotherapeutic targets modulating monocyte and macrophage function.
2 Cirrhosis
The main underlying aetiologies for cirrhosis in developed countries are alcohol use causing alcohol-related liver disease (ARLD), non-alcoholic fatty liver disease (NAFLD)—recently also referred to as metabolic-associated fatty liver disease (MAFLD) (Eslam et al., 2020)—and viral infections such as chronic hepatitis B and C (HBV/HCV) (Asrani et al., 2019). In Europe the aetiologies causing cirrhosis are changing due to the therapeutic cure of HCV and the control of chronic HBV, the increasingly widespread unhealthy use of alcohol, the growing epidemic of obesity, and undiagnosed or untreated liver disease (Karlsen et al., 2022). It is believed that NAFLD will become the most common aetiology for liver transplantation in end stage cirrhotic patients (Haldar et al., 2019; Younossi et al., 2019). Cirrhosis has been defined by severe parenchymal loss due to necrosis, inflammation and fibrogenesis as a consequence of diverse underlying aetiologies. It is histopathologically characterised by diffuse nodular regeneration fibrous septa, parenchymal loss and vascular remodelling (Tsochatzis et al., 2014). Pathophysiologically, it involves the accumulation of fibrous tissue due to the activation of heaptic stellate cells (HSCs), as well as changes in the vascular architecture of the liver, leading to increased resistance to portal blood flow and progressive PH (Wanless, 2020). Over the previous 2 decades, it has been shown that under certain circumstances fibrosis and even cirrhosis may be reversible. These conditions must involve an effective and sustainable treatment of the underlying cause of liver injury (Wanless et al., 2000; Lee et al., 2015a; Hytiroglou and Theise, 2018) e.g., successful treatment of chronic viral hepatitis (Chang et al., 2010; Marcellin et al., 2013).
2.1 Disease stages of cirrhosis
Liver cirrhosis can roughly be categorized into a typically asymptomatic compensated stage and a decompensated stage, marked by the occurrence of complications and reduced survival (D'Amico et al., 2006; Moreau et al., 2013; Planas et al., 2006; Arroyo et al., 2020). However, this classification does not discriminate between the prognostic subgroups that characterise the course of decompensation, which depends on the type and number of decompensating events (D’Amico et al., 2022) (Figure 1). Decompensation of cirrhosis was recently defined by the presence or history of any complication of ascites, bleeding, hepatic encephalopathy (HE) or jaundice (D’Amico et al., 2022), and may be characterised by an acute onset (AD) (Moreau et al., 2013) or by a progressive, non-acute onset (NAD) (D’Amico et al., 2022) (Figure 1). NAD involves slow ascites formation, mild HE, or progressive jaundice in non-cholestatic cirrhosis free of other complications and is mostly represented by first decompensation events that do not require hospitalisation (D’Amico et al., 2022) (Figure 1). In contrast, AD is defined as any first or recurrent moderate or severe ascites within less than 2 weeks, first or recurrent acute HE in patients with previous normal consciousness, acute gastrointestinal bleeding, and any type of acute bacterial infection (D’Amico et al., 2022; Trebicka et al., 2021; Arroyo et al., 2021). AD may manifest itself as unstable decompensated cirrhosis (UDC), stable decompensated cirrhosis (SDC), pre-ACLF or ACLF (D’Amico et al., 2022). UDC involves a 3-months mortality of 21–35,6%, while SDC occurs with a 3-months mortality of 0–9.5% (D’Amico et al., 2022). In a pre-ACLF state ACLF develops within a 3-months period and shows a 3-months mortality of 53,7–67,4% (D’Amico et al., 2022) (Figure 1). ACLF, the most severe stage of cirrhosis with a 28-days mortality of up to 77% (D’Amico et al., 2022), is defined by the development of organ failures (liver, kidney, brain, circulation, coagulation and lung) (Moreau et al., 2013; Gustot et al., 2015; Arroyo et al., 2020; Bajaj et al., 2021; Trebicka et al., 2021). Overall, the classification of cirrhosis stages has recently developed and remains debated. Consequently, not all publications refer to the same classification. The exact assignment to the stages of cirrhosis studied is highly relevant regarding the interpretation of experimental research data in the field.
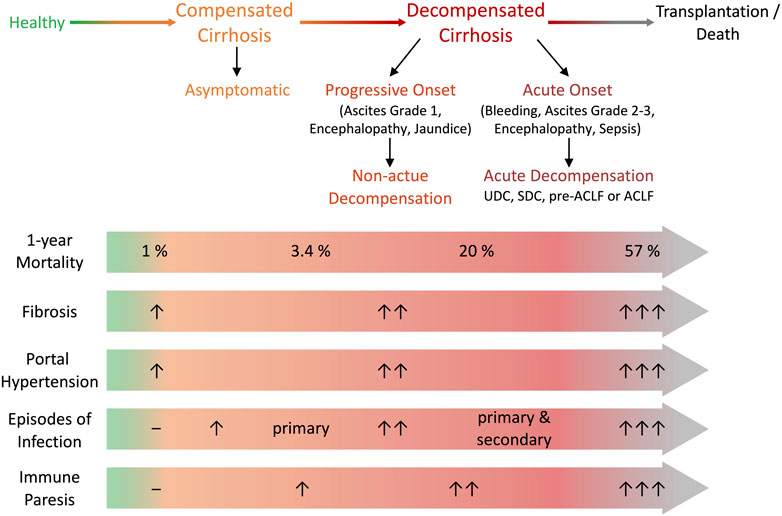
FIGURE 1. Definitions of decompensation and clinical stage of cirrhosis. Cirrhosis can be categorized in an asymptomatic compensated stage and decompensated stages with the onset of various complications. Decompensation can be characterised by progressive non-acute onset (non-acute decompensation, NAD) or acute onset (acute decompensation, AD). Progressive onset is defined as slow formation of ascites, low grade encephalopathy or progressive jaundice and doesn’t necessarily require hospitalization. AD is defined as rapid ascites formation within less than 2 weeks, acute hepatic encephalopathy, gastrointestinal bleeding or bacterial infection and usually requires hospitalization. AD presents as SDC with stable decrease of systemic inflammation and no further AD for at least 1 year, UDC characterised by persistent albeit unstable inflammatory status resulting in further AD events within 1 year, pre-ACLF with ACLF occurring within 3 months and ACLF defined by development of organ failure. During progression of cirrhosis mortality, fibrosis, portal hypertension and its associated clinical manifestations, the risk for episodes of infection increases and relates to the development of immune paresis. Adapted from (D’Amico et al., Journal of Hepatology 2022 and Bernsmeier et al., Journal of Hepatology 2020). ACLF, acute-on-chronic liver failure; SDC, stable decompensated cirrhosis; UDC, unstable decompensated cirrhosis.
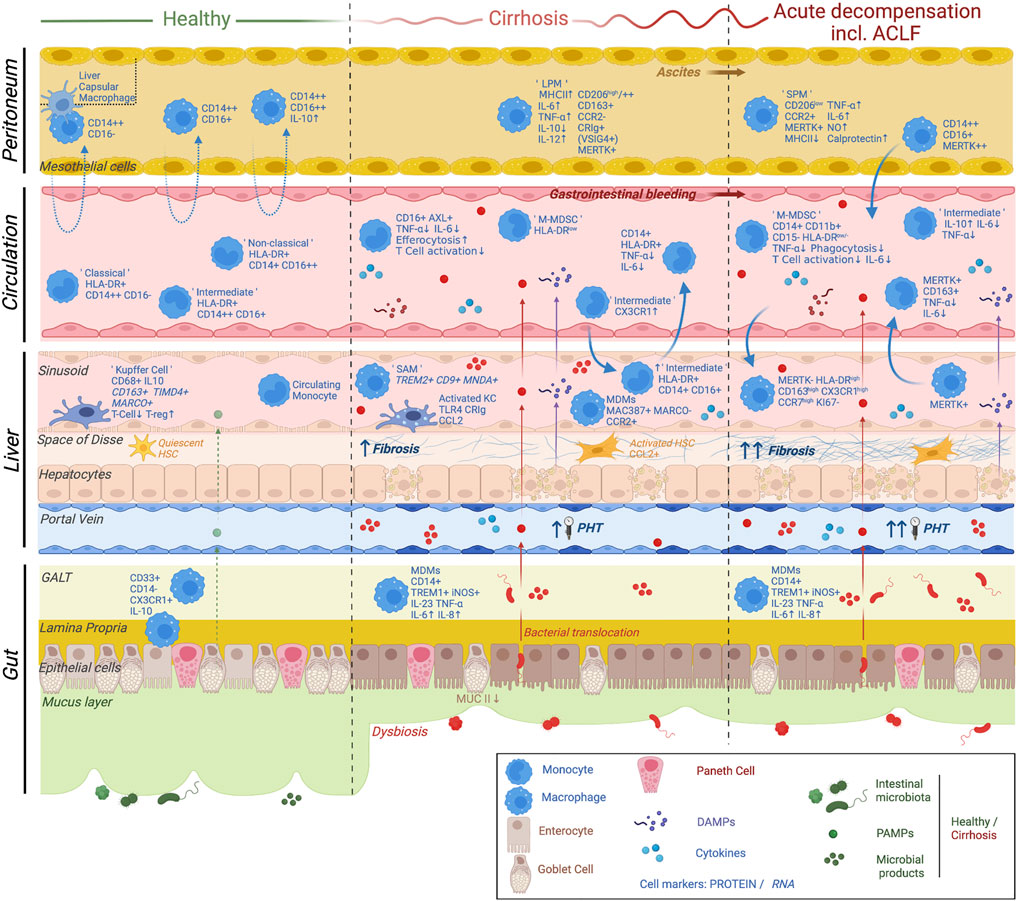
FIGURE 2. Current knowledge on monocyte and macrophage plasticity in relation to the multi-compartmental pathophysiological mechanisms underlying cirrhosis progression and decompensation. The main pathophysiological mechanisms underlying cirrhosis progression and acute decompensation are shown. These include liver fibrosis, hepatic endothelial dysfunction, parenchymal damage, portal hypertension, dysbiosis, bacterial translocation as well as activation of both local and systemic inflammatory responses. A variety of cellular and non-cellular players involved in these mechanisms are depicted (see symbol legend). In summary, homeostatic monocyte and macrophage populations display a distinct phenotype and function in a compartment-specific manner. Changes in monocyte/macrophage differentiation and migration patterns with progression of cirrhosis and acute decompensation are due to multiple factors involving chronic inflammation, exposure to DAMPs (released by injured hepatocytes) as well as exposure to PAMPs/microbial products due to dysbiosis and pathological bacterial translocation. Distinct cell subsets are shown in gut, liver, circulation and peritoneum, underlining the multi-compartmental nature of the disease. Adapted from Bernsmeier et al., Journal of hepatology 2020. ACLF, acute-on-chronic liver failure; LPM, large peritoneal macrophage; SPM, small peritoneal macrophage; M-MDSC, monocytic myeloid-derived suppressor cell; SAM, scar-associated macrophage; MDM, monocyte-derived macrophage; HSC, hepatic stellate cell; PHT, portal hypertension; GALT, gut-associated lymphoid tissue; DAMP; damage-associated molecular patterns; PAMP, pathogen-associated molecular pattern. Created with BioRender.com.
2.2 Pathophysiology of cirrhosis—the systemic inflammation hypothesis
The most important factors in the pathophysiology of immuneparesis in cirrhosis are DAMPs, PAMPs and the systemic inflammatory response (SIRS): Chronic liver injury results in release and exposure to DAMPs, with cirrhosis progression pathologic BT develops and can lead to infections subsequently resulting in SIRS.
2.2.1 Chronic liver injury and DAMPs
Importantly, the pathophysiology of cirrhosis is not restricted to the liver, rather it represents a systemic condition involving various compartments, immune cells and soluble factors (Albillos et al., 2014; Ber nsmeier et al., 2020). Upon chronic liver injury fibrosis occurs and its formation involves activated resident or recruited macrophages, hepatocytes and HSCs. HSCs are key players in fibrosis development as they remain quiescent under homeostatic conditions but become activated following hepatic injury and transform towards myofibroblasts which secrete extracellular matrix proteins (Tsuchida and Friedman, 2017; Kisseleva and Brenner, 2021). Immune dysfunction in cirrhosis initiates with the onset of chronic hepatic inflammation and worsens along with the progression of cirrhosis and the development of PH (Figure 1). It involves components of the innate immune system, which constitutes the first line of defence against pathogens as it plays a key role in maintaining homeostasis by phagocytosis and killing of bacteria, antigen presentation, inflammatory cytokine production, activation of adaptive immune cells and providing physical and chemical barriers. In cirrhosis chronic inflammation, parenchymal damage and scarring of the liver activate local tissue inflammation and contribute to a systemic inflammatory response mediated by DAMPs, cytokines and migration of immunoregulatory innate immune cells (Ber nsmeier et al., 2020). The liver sinusoidal endothelium is highly affected in cirrhosis due to its capillarization and angioarchitectural change (DeLeve, 2015; Su et al., 2021).
2.2.2 Bacterial translocation and PAMPs
The gut-liver axis is an operative unit protecting the body against harmful substances and microorganisms, maintaining homeostasis of the immune system. Liver cirrhosis profoundly alters this complex system and the intestine becomes more permeable allowing the translocation of bacteria and bacterial products (PAMPs) into the portal circulation (Ponziani et al., 2018). In the gut, dysbiosis, i.e. the reduction of beneficial taxa and increase in pathogenic bacteria, and reduction of the mucus layer is observed (Bonnel et al., 2011; Albillos et al., 2014; Bajaj et al., 2014; Wiest et al., 2017; Fernández et al., 2018; Fernández et al., 2019; Piano et al., 2019; Albillos et al., 2020; Bajaj et al., 2021; Van der Merwe et al., 2021). Gut endothelial and vascular barrier dysfunction results in BT, endotoxemia as well as mucosal immune cell alteration (Zapater et al., 2008; Ber nsmeier et al., 2020; Haderer et al., 2022). Pathological BT, present in advanced cirrhosis, is defined by the increased rate of translocation of bacteria or bacterial products from the gut to mesenteric lymph nodes, the portal and systemic circulation as well as other tissues. Gut derived PAMPs further drive hepatic inflammation and immune cell differentiation in the gut, liver, circulation and other organs (Zapater et al., 2008; Ber nsmeier et al., 2020; Stengel et al., 2020; Haderer et al., 2022).
2.2.3 Onset of infections and acute decompensation
Patients with cirrhosis are at high risk of bacterial infections, which are associated with the development of AD, hospitalisation, sepsis and the need for intensive care and organ support involving a substantial mortality (Arvaniti et al., 2010; Moreau et al., 2013; Jalan et al., 2014; Van der Merwe et al., 2021; Triantos et al., 2022) (Figure 1). The most common infections include spontaneous bacterial peritonitis (SBP), urinary tract infection, pneumonia, spontaneous bacteraemia, skin, and soft tissue infections (Arvaniti et al., 2010; Bajaj et al., 2012; Fernández et al., 2018; Piano et al., 2019; Arroyo et al., 2021; Trebicka et al., 2021). Bacterial infections in hospitalised patients are much more frequent in patients with cirrhosis (32–34%) compared to those without (5–7%). Remarkably, around 45% of hospitalised cirrhosis patients with gastrointestinal bleeding develop infections (Borzio et al., 2001; Tandon and Garcia-Tsao, 2008; Arvaniti et al., 2010). Of note, it has been observed that a dual infection (bacterial and fungal) increases the mortality of cirrhosis patients compared to patients with a single infection (Bajaj et al., 2018; Bajaj et al., 2021). As in the general population, infections caused by multi-drug-resistant organisms are progressively increasing in cirrhotic patients and represent up to 30–35% of all infections (Fernández et al., 2012; Fernández et al., 2019; Piano et al., 2019; Onorato et al., 2022).
Pathological BT in the context of immuneparesis can lead to SBP (Assimakopoulos et al., 2012; Wiest et al., 2014). SBP is diagnosed in patients with an elevated neutrophil count in the ascitic fluid (Runyon and Aasld, 2013), but no differences in mortality were observed independently of whether the bacterial culture of ascites was positive or negative (Runyon and Aasld, 2013). Moreover, BT and gut barrier impairment are directly related to the risk of variceal bleeding (Triantos et al., 2022). The plasticity of this process is accompanied by SIRS activation in ACLF and implicates changes in neural regulation, circulating inflammatory mediators and hormones (Bosmann and Ward, 2013; Bernsmeier et al., 2015a). Sepsis and SIRS are associated with hyperinflammation, impaired innate immune function, immunosuppression and complement activation, collectively leading to septic shock and organ failure (Bosmann and Ward, 2013).
Infection susceptibility in cirrhosis has been attributed to a state of immuneparesis, defined by inadequate immune responses to microbial challenge (Bonnel et al., 2011; Bajaj et al., 2012; Albillos et al., 2014). The pathophysiology of immuneparesis is very complex and incompletely understood, involving diverse defects in immune cell function, including monocytes and macrophages in multiple compartments (Bonnel et al., 2011). This suggests that the disease specific differentiation and function of monocytes and macrophages actively contribute to inflammation and infection susceptibility in cirrhosis patients. The following sections focus on the plasticity of mononuclear phagocytes in the context of cirrhosis.
3 Immunepathophysiology of mononuclear phagocytes in cirrhosis
Mononuclear phagocytes (monocytes and macrophages) play a crucial role in initiating immune responses. Their functions include phagocytosis, killing of bacteria, antigen presentation, cytokine production, immune cell recruitment to sites of infection, and inflammation and activation of immune effector cells. Phagocytes regulate the antimicrobial defence, inflammation in tissue injury, fibrogenesis and tumourigenesis (Yona et al., 2013; Ziegler-Heitbrock and Hofer, 2013). Related to these functions, monocytes and macrophages are essential in the pathophysiology of immune dysfunction in patients with cirrhosis of the liver as a systemic inflammatory condition (Ber nsmeier et al., 2020; Singanayagam and Triantafyllou, 2021) (Figure 2).
3.1 Circulating monocytes
Principally, monocytes can be divided into classical (CD14++CD16−), intermediate (CD14++CD16+) and non-classical (CD14+CD16++) subsets (Passlick et al., 1989; Jakubzick et al., 2017; Patel et al., 2017; Guilliams et al., 2018; Kapellos et al., 2019) and further identified by their human leukocyte antigen DR (HLA-DR) expression (Guilliams et al., 2018). The intermediate and non-classical monocyte subsets emerge sequentially from the pool of classical monocytes (Patel et al., 2017). It has been found in mice and confirmed in human that CD14+CD16++ non-classical monocytes fulfil a continuous monitoring of the vasculature by a crawling mechanism on endothelial cells (Cros et al., 2010). This behaviour permits the efficient scavenging of luminal microparticles under homeostatic conditions. While this established classification was based on protein assays which involved mainly flow cytometry-based techniques, recent unbiased single-cell RNA sequencing (scRNA-Seq) studies have suggested broader heterogeneity within the intermediate population of monocytes, through the identification of two distinct intermediate monocyte subsets, one expressing classical monocyte and cytotoxic genes, while the other one with so far unknown functions (Villani et al., 2017).
3.1.1 Monocyte development
The development of classical monocytes was firstly discovered with the use of mouse models and some of these findings could subsequently be transferred to humans. In mice, haematopoietic stem cells in the bone marrow give rise to a monocyte/dendritic cell progenitor (MDP) (Guilliams et al., 2018). This MDP population was proposed to give rise to monocytes and classical dendtritic cells (DCs) in both mice (Olsson et al., 2016; Guilliams et al., 2018) and humans (Lee et al., 2015b; Guilliams et al., 2018), by following a binary trajectory towards either dedicated common DC precursors (CDP) or into unipotent common monocyte progenitors (cMoP) (Lee et al., 2015b; Guilliams et al., 2018). Accordingly, the cMoP population has also been identified in human bone marrow (BM) (Kawamura et al., 2017). An important transcription factor maintaining commitment towards the monocyte lineage in humans is interferon regulatory factor 8 (IRF8) (Hambleton et al., 2011). An autosomal recessive IRF8 deficiency in humans leads to reduced numbers of circulating monocytes (Hambleton et al., 2011). Interestingly, investigations into cMoP biology have uncovered that this population possesses a high proliferative capacity and is characterized by CD14 expression in humans (Chong et al., 2016). Further differentiation of cMoP into mature monocytes involves a transient pre-monocyte stage, discriminated by the expression of CXC-motiv chemokinereceptor 4 (CXCR4) in mice and humans (Chong et al., 2016). In mice the downregulation of Cxcr4 expression is accompanied by the up-regulation of C-C chemokine receptor 2 (Ccr2), indicating that Ccr2 regulates the BM exit of murine monocytes (Serbina and Pamer, 2006; Chong et al., 2016). While it remains unclear whether CCR2 also plays a role in BM exit of human monocytes to the circulation (Guilliams et al., 2018), it was shown that CXCR4 participates in the homing of classical and non-classical monocytes to central (BM) and peripheral (spleen) monocyte reservoirs (Chong et al., 2016). In mice, following release of classical monocytes into the circulation from the BM under healthy homeostasis, these cells remain in the circulation and may subsequently either traffic to repopulate a proportion of tissue-resident macrophages (Tamoutounour et al., 2012; Bain et al., 2013; Jakubzick et al., 2013; Bain et al., 2014; Epelman et al., 2014; Calderon et al., 2015; Guilliams et al., 2018) or alternatively convert into non-classical monocytes (Yona et al., 2013; Patel et al., 2017). In an analogous manner, it was observed in humans that the vast majority of classical monocytes are recruited to peripheral tissues where they potentially differentiate into monocyte-derived cells or enter reservoirs of undifferentiated monocytes (Tak et al., 2017a; Patel et al., 2017). The transition of classical monocytes to non-classical monocytes has been observed in different species including mouse (Sunderkötter et al., 2004; Tacke et al., 2006; Varol et al., 2007; Yona et al., 2013), rat (Yrlid et al., 2006), macaque (Sugimoto et al., 2015) as well as in humans (Patel et al., 2017) and therefore represents an evolutionarily conserved program (Guilliams et al., 2018).
3.1.2 Monocyte differentiation in the context of inflammation and cirrhosis
Proportions of circulating monocytes vary depending on monopoiesis, tissue infiltration and their release from central (BM) or peripheral reservoirs. In humans, endotoxin induces a rapid yet transient monocytopenia during the first 2 h after lipopolysaccharide (LPS) injection followed by the sequential reappearance of CD14++CD16− classical monocytes, followed by CD14+CD16+ intermediate cells and finally CD14+ CD16++ non-classical monocytes (Thaler et al., 2016; Tak et al., 2017b; Patel et al., 2017). The number of non-classical monocytes is thus strongly linked to the physiological status of the organism and therefore represents a potential diagnostic tool (Selimoglu-Buet et al., 2015). Not only do cytokines directly provoke functional changes in monocytes, but they also influence the cellular outcome of haematopoietic stem cells, a phenomenon called “trained immunity” (Quintin et al., 2012; Netea et al., 2016). A number of cytokines have been proposed to play a key role in trained immunity, including interferon-ɣ (INF-ɣ) and interleukine-1β (IL-1β), suggesting that activation of haematopoietic stem cells by cytokines produced by immune or non-immune cells in the BM is crucial for these long-lasting training effects (Boettcher and Manz, 2017). Inflammation-induced emergency haematopoiesis can result in trained immunity characterized by long-term epigenetic effects on haematopoietic stem cells. The epigenetic changes associated with monocyte training involve histone modification of genes encoding pro-inflammatory cytokines such as IL-6, tumor necrosis factor-α (TNF-α) but also genes of the mammalian target of rapamycin (mTOR) pathway (Quintin et al., 2012; Netea et al., 2016). Under pathological conditions, monocytes gain distinct non-redundant functions that often cannot be fulfilled by resident macrophages and DCs. These non-exclusive and sometimes overlapping effector functions comprise pro-inflammatory activities, antigen-presentation, tissue remodelling, or anti-inflammatory abilities (Patel et al., 2017; Guilliams et al., 2018). Furthermore, in mouse models effector monocytes actively participate in fibrosis development (Satoh et al., 2017) and can also differentiate into pathogenic foam cells (Guilliams et al., 2018). This highlights that monocytes are not simply precursors that get recruited to temporarily increase the number of tissue resident macrophages but in fact give rise to functionally distinct monocyte-derived cells (Guilliams et al., 2018).
In patients with cirrhosis of the liver the number of circulating monocytes increases gradually with the progression of disease (Hassner et al., 1979; Zimmermann et al., 2010; Korf et al., 2019a; Brenig et al., 2020). An expansion of the intermediate monocyte population was observed in the circulation and the liver in the context of cirrhosis progression, as a consequence of enhanced recruitment from blood and local differentiation from classical monocytes (Zimmermann et al., 2010; Liaskou et al., 2013; Korf et al., 2019a). This indicates the pathological condition present in patients with cirrhosis is reflected by monocyte differentiation. Intermediate CD14++CD16+ monocytes accumulated in chronically inflamed and cirrhotic human liver as a consequence of enhanced recruitment from the blood (Zimmermann et al., 2010; Liaskou et al., 2013; Zimmermann et al., 2016). These monocytes displayed a high level of phagocytosis, antigen presentation, T cell proliferation and secretion of cytokines (TNF-a, IL-6, IL-8, and IL-1b) as well as profibrogenic cytokines (IL-13) and growth factors (granulocyte-colony stimulating factor G-CSF, granulocyte-macrophage-colony stimulating factor GM-CSF) (Liaskou et al., 2013).
3.1.3 Expansion of M-MDSC in cirrhosis
One of the first discoveries of monocyte dysfunction in patients with cirrhosis was their impaired phagocytosis capacity (Sanchez-Tapias et al., 1977; Sherlock, 1977; Hassner et al., 1979; Holdstock et al., 1982). In line with this finding, a reduced expression of major histocompatibility complex class II (MHC II) molecules was observed on monocytes from patients with ACLF and was associated with adverse prognosis (Hassner et al., 1979; Wasmuth et al., 2005). Confirming these findings, it was shown that MHC II molecule expression gradually decreased with cirrhosis progression (Lin et al., 2007; Xing et al., 2007; Bernsmeier et al., 2018; Brenig et al., 2020). HLA-DR, a MHC II family member, can be considered as a monocyte activation marker, due to the increased expression upon immune stimulation and its function in inducing T cell responses (Gordon and Taylor, 2005). On the contrary, the downregulation of HLA-DR appears in acquired immunosuppressive monocyte differentiation (Gordon and Taylor, 2005).
The reduced HLA-DR expression on monocytes in cirrhosis was associated with the expansion of mononuclear myeloid-derived suppressor cells (M-MDSC, CD11b+CD14+HLA-DRlo/−CD15−) (Bronte et al., 2016) in the circulation of patients with cirrhosis (Bernsmeier et al., 2018). The term MDSCs was first introduced in the year 2007 in a cancer-related context (Gabrilovich et al., 2007). MDSCs develop from myeloid cell expansion and conditioning in the BM and spleen with subsequent conversion of monocytes into pathologically activated MDSCs (Condamine et al., 2015; Wang et al., 2019; Ostrand-Rosenberg et al., 2020). Human M-MDSCs are distinct from monocytes by their low expression of MHC II (Bronte et al., 2016). The main characteristic of MDSCs is the inhibition of immune responses, including those mediated by T cells, B cells and NK cells (Gabrilovich, 2017). The suppression of immune responses is mediated by upregulation of signal transducer and activator of transcription 3 (STAT3) expression, induction of endoplasmic reticulum (ER) stress, expression of arginase 1 (Arg1) and expression of S100A8/A9 (Veglia et al., 2021). To fulfil their immunosuppressive function M-MDSCs use nitric oxide (NO), immunosuppressive cytokines such as interleukin-10 (IL-10) and transforming growth factor β (TGF-β) and the expression of immune regulatory molecules like programmed death-ligand 1 (PD-L1) (Gabrilovich, 2017). Recently, trajectory analysis showed that M-MDSCs are also molecularly distinct from M1-like and M2-like macrophages (Song et al., 2019), indicating that M-MDSCs represent a subset distinct from monocytes and macrophages.
In the context of cirrhosis, endotoxemia (the presence of bacteria-derived endotoxins in the blood stream) is observed in advanced stages of disease and is associated with the loss of HLA-DR (Zapater et al., 2008; Bernsmeier et al., 2018). Moreover, loss of HLA-DR expression affects the antigen presentation capacity of monocytes and their ability to induce activation of adaptive immune responses and is associated with adverse clinical outcomes in patients with cirrhosis (Bernsmeier et al., 2018; Brenig et al., 2020). M-MDSCs in AD/ACLF patients displayed immunosuppressive properties in regard to their pro-inflammatory cytokine production (TNF-α, IL-6) (Wasmuth et al., 2005; Lin et al., 2007; Berres et al., 2009; Berry et al., 2011; Bernsmeier et al., 2018; Korf et al., 2019a; Brenig et al., 2020) as well as their reduced T cell activation and decreased phagocytosis capacity, contributing to the dampened innate immune responses of circulating monocytes (Bernsmeier et al., 2018). Sequentially increasing numbers of M-MDSC with disease progression from compensated cirrhosis to AD/ACLF (Bernsmeier et al., 2018) underlie the low TNF-α/IL-6 responses in AD/ACLF patients. Furthermore CD8+ T cells from cirrhotic patients displayed high HLA-DR, T cell immunoglobulin and mucin-domain containing-3 (TIM-3), cytotoxic T-lymphocyte-associated protein 4 (CTLA-4) and programmed cell death protein 1 (PD-1) expression associated with concomitant infections and disease severity (Lebossé et al., 2019).
In vitro exposure of monocytes from healthy controls to plasma isolated from AD/ACLF patients reduced monocytic HLA-DR expression as well as suppressed TNF-α/IL-6 responses. (Bernsmeier et al., 2018; O'Brien et al., 2014; Bernsmeier et al., 2015b). In a similar fashion healthy human monocytes exposed to activated primary human HSCs, converted mature CD14+ monocytes into M-MDSCs in a contact-dependent manner (Höchst et al., 2013; Resheq et al., 2015). Recently it has been shown that MDMCs from patients with non-cirrhotic chronic HBV infection had profound suppressive ability, expressing Arg1/iNOS/PD-L1/CTLA-4/CD40 at significantly greater levels relative to healthy controls (Pal et al., 2022).
3.1.4 TAM receptor expression on monocytes in cirrhosis
Lately, TAM receptors (TYRO3, AXL, and MERTK), which are tyrosine kinase receptors expressed on monocytes and macrophages and essential for the regulation of immune homeostasis, have been shown to play a central role in cirrhosis and contribute to the systemic immune paresis in patients with cirrhosis and liver failure (Roth lin et al., 2007; Bernsmeier et al., 2015b; Triantafyllou et al., 2018; Brenig et al., 2020; Flint et al., 2022). They maintain immune homeostasis by regulating innate immune responses via suppression of inflammatory toll-like receptor (TLR) signalling cascades and promotion of tissue resolution thorough the clearance of apoptotic cells (efferocytosis) (Flint et al., 2022). Immuneregulatory AXL-expressing monocytes expanded along with the progression of cirrhosis, representing <5% in early stages and up to 40% of monocytes in later stages, but were scarce following an event triggering AD (<5%) (Brenig et al., 2020). In compensated cirrhosis and NAD, the expansion of AXL-expressing circulating monocytes correlated with the occurrence of infectious episodes, onset of subsequent AD within 4 months and 1-year mortality (Brenig et al., 2020). On a functional level, AXL-expressing monocytes exhibited a reduced T cell activation potential and dampened TNF-α and IL-6 responses upon TLR4 stimulation through LPS treatment. However, AXL+ monocytes showed preserved capacity to phagocytose pathogens and enhanced capacity to phagocyte apoptotic cells, i.e. efferocytosis, when compared to AXL− monocytes (Brenig et al., 2020). Another TAM receptor family member, MERTK was found to be up-regulated on circulating monocytes in the stage of AD/ACLF in cirrhosis patients (Bernsmeier et al., 2015b; Brenig et al., 2020). MERTK-expressing monocytes represented 14.4% of all circulating monocytes in patients with AD and even 35.7% in ACLF, respectively, and showed dampened innate immune responses to LPS in close association with clinical disease severity scores and the need for transplantation or death (Bernsmeier et al., 2015b).
3.1.5 Functional consequences of monocyte plasticity following acute decompensation
Monocytes from patients with ACLF showed polarisation towards an immunotolerant state by reduced HLA-DR expression, reduced TNF-α/IL-6 production but elevated IL-10 production (Wasmuth et al., 2005; Lin et al., 2007; Berres et al., 2009; Berry et al., 2011; Bernsmeier et al., 2018; Korf et al., 2019a; Brenig et al., 2020), and impaired phagocytic capacity. As indicated, the phagocytic capacity of monocytes was maintained during disease progression, but diminished following insults leading to AD/ACLF stages (Gadd et al., 2016; Bernsmeier et al., 2018).
Under pathological conditions the number, phenotype and function of circulating monocytes changes, indicating that monocytes are not only macrophage precursors, but in fact form a separate cell population giving rise to functionally distinct cells. Immunosuppressive M-MDSCs increase in late stages of cirrhosis either developing earlier in the BM and spleen or originating from mature circulating monocytes in the disease-specific milieu. The appearance of distinct monocyte subsets at early and late stages of disease indicates that monocyte subsets are susceptible to phenotypic and functional changes not only on a mature circulating monocyte level, but already on a developmental level. Thus, monocyte differentiation is continually adapting to the inflammatory milieu in response to PAMPs, DAMPs and SIRS. In NAD, AD, and ACLF stages of disease, immunoregulatory monocytic populations expanding and replace substantial proportions of functionally regular counterparts. In order to prevent infection, decompensation and death from cirrhosis, it is desirable to further explore therapeutic options aimed at restoring systemic innate immune homeostasis by reconstituting monocyte function, while also assessing the risk of therapy-related side effects (see Section 5).
3.2 Macrophages in the liver
Monocytes are considered to be precursors of macrophages polarizing and generating subpopulations traditionally called activated, pro-inflammatory macrophages “M1” and alternatively activated, anti-inflammatory macrophages “M2” (Nathan et al., 1983; Mosmann et al., 1986; Murray et al., 2014; Chávez-Galán et al., 2015). This categorization of macrophages cannot be applied to hepatic macrophages since they simultaneously express M1 and M2 markers (Gao et al., 2019) and recent evidence using mouse models suggested that liver resident Kupffer cells (KCs) are self-renewing and originate from the yolk sack (Li et al., 2017). These cells exhibit great plasticity which is dependent on their ontogeny, local and systemic microenvironmental mediators and epigenetic programming (Guillot and Tacke, 2019; Wen et al., 2021). To date, markers that distinctly identify KCs in humans and allow to distinguish these cells from monocyte-derived macrophages (MDMs) have been reviewed. Undoubtedly, new technical opportunities provided by ‘omics’ technologies (genomics, transcriptomics, proteomics, and metabolomics) will allow for the re-evaluation and further exploration of distinct KC and MDM markers (Guilliams et al., 2022). The liver drains blood from abdominal organs through the portal vein and receives systemic blood via the hepatic artery (Jenne and Kubes, 2013). It is a highly organised tolerogenic organ with a large sinusoidal network. These so-called sinusoids are lined by permeable fenestrated liver sinusoidal endothelial cells (LSECs) enabling the exposure of plasma content such as gut-derived bacterial products to hepatocytes and non-parenchymal cells of the liver (Su et al., 2021) and harbour KCs (Figure 2).
3.2.1 Kupffer cells
In homeostatic conditions immune activation is prevented by KCs, LSEC, and DCs, which together promote immune tolerance. KCs are the most abundant hepatic immune cells maintaining liver homeostasis and immune tolerance, due to their continuous exposure to harmless gut-derived antigens and their ability to rapidly identify and eliminate pathogens (Wen et al., 2021). The key role of liver resident KCs is to phagocytose and scavenge DAMPs, recognize PAMPs and remove circulating bacteria to prevent systemic immune activation by secreting IL-10 (Kubes and Jenne, 2018). Furthermore, KCs are capable of self-renewal and show a specific transcriptional program defined by their unique niche (Zigmond et al., 2014; Beattie et al., 2016; Krenkel and Tacke, 2017). KCs are located within the sinusoidal lumen, in continuous contact with LSECs and can also interact with HSCs and hepatocytes across the space of Disse (Jenne and Kubes, 2013; Bonnardel et al., 2019; Sutti et al., 2019). Liver resident KCs are capable of removing bacterial products and secreting tolerogenic factors, regulating this microenvironment (Sutti et al., 2019). KCs have the ability to inhibit T cell expansion and induce regulatory T cells, that may reprogram liver infiltrating monocytes to regulatory IL-10+ DCs that further promote tolerance (Heymann et al., 2015; Heymann and Tacke, 2016). Furthermore, hepatocytes, HSCs and endothelial cells shift infiltrating monocytes towards a more tolerogenic phenotype (Blériot and Ginhoux, 2019; Bonnardel et al., 2019).
The balance between immunity and tolerance is essential to liver function (Kubes and Jenne, 2018) and in cirrhosis the mechanisms to maintain tolerance fail (Wu et al., 2010). Chronic liver injury and inflammation lead to progressive liver fibrosis and ultimately to the development of cirrhosis with formation of fibrous septa, regenerative nodules, sinusoidal resistance, intrahepatic shunting and PH (Koyama and Brenner, 2017; Laleman et al., 2018). The liver, which drains the intestinal venous blood, forms a vascular “firewall” that captures gut commensal bacteria entering the blood stream during intestinal pathology (Balmer et al., 2014). The compartmentalization of intestinal microbes was found to be defective in animal models of liver disease due to failure of the hepatic vascular “firewall” that is required to clear microbes from the mesenteric and systemic vasculature efficiently (Balmer et al., 2014). Mononuclear phagocytic cells in the liver can be categorized into liver resident KCs (Kubes and Jenne, 2018), circulating monocytes trafficking through the sinusoids or migrating monocytes, which may persist as monocytes in the tissue, acquire antigen-presenting capability or mature into macrophages (Strauss et al., 2015; Jakubzick et al., 2017; Weston et al., 2019). During the progression from inflammation with fibrosis to cirrhosis, KCs are continuously activated by DAMPs released by dying hepatocytes. Activated TLR4/complement receptor of the immunoglobulin superfamily (CRIg)-expressing KCs lose their tolerogenic phenotype and secrete pro-inflammatory cytokines, amplifying immune responses and recruiting immune cells to the liver (Kolios, 2006; Weston et al., 2019). Experimental models of acute liver injury in rodents have been used to better understand macrophage function during liver disease (Weston et al., 2019). These models showed that extensive hepatocyte damage releases DAMPs which are sensed by KCs leading to the release of cytokines, chemokines and pro-inflammatory macrophage recruitment (Krenkel and Tacke, 2017). During acetaminophen-induced acute liver injury in humans a phenotypic switch in macrophage phenotype is observed, where MAC387 (S100A9) serves as a marker to identify MDMs in contrast to CD68+ resident KCs in the liver (Antoniades et al., 2012). Recently, using scRNA-Seq KCs were phenotypically described as CD68+CD163+MARCO+TIMD4+ (MacParland et al., 2018; Aizarani et al., 2019; Ramachandran et al., 2019).
3.2.2 Capsular macrophages
Furthermore, a distinct population of MHC II-expressing cells was observed in the hepatic capsule (PRICKE TT et al., 1988). In mice it has been shown that this population represented a macrophage population (liver capsular macrophages LCMs) that is distinct from KCs (Sierro et al., 2017). LCMs in mice are CX3CR1hiTIM4- and replenished in the steady state from blood-borne monocytes (Sierro et al., 2017). Recent spatial transcriptomic experiments in mice have shown that these capsule macrophages can be further characterised by the expression of CD207 and CX3CR1 (Guilliams et al., 2022), but could not be identified in human liver biopsies due to the lack of capsule tissue (Guilliams et al., 2022). In mice, LCMs form a cellular network in the hepatic capsule and potentially sense the blood and the peritoneal cavity due to their extended dendrites (Sierro et al., 2017). It has been suggested that LCMs sense peritoneal bacteria accessing the liver capsule and prevent them from breaching the liver capsule through the recruitment of neutrophils (Sierro et al., 2017).
3.2.3 Monocyte-derived macrophages
In cirrhotic livers the number of intermediate CD14++CD16+ monocytes increases, these cells accumulate at sites of inflammation and fibrosis and are characterised by enhanced phagocytosis capacity, antigen presentation capacity, activation potential for T cell proliferation and secretion of numerous soluble factors like chemokines, growth factors, pro-inflammatory, and pro-fibrogenic mediators (Zimmermann et al., 2010; Liaskou et al., 2013; Tsuchida and Friedman, 2017). Intermediate monocytes contribute to the perpetuation of hepatic inflammation and fibrogenesis and show an increased CX3CR1-dependent migratory potential towards liver tissue, while also displaying the ability to transmigrate back to the circulation and contribute to SIRS (Aspinall et al., 2010; Zimmermann et al., 2010). In the context of cirrhosis capillarization of LSECs, formation of basement membranes and activation of HSCs contributes to increased migration of immune cells across the sinusoidal endothelial barrier (Bataller and Brenner, 2005; Henderson et al., 2006; Li et al., 2012; Ramachandran et al., 2012; Shetty et al., 2018; Su et al., 2021). Both, activated KCs and HSCs produce CCL2 and CCL5 promoting the recruitment of MDMs to the liver through CCR2/CCL2 or CCR5/CCL5 signalling (Heymann and Tacke, 2016; Ju and Tacke, 2016; Krenkel and Tacke, 2017; Sasaki et al., 2017; Ramachandran et al., 2020). In addition, activated HSCs can also recruit monocytes through the CCR8/CCL1 and CXCR3/CXCL10 axes (Heymann et al., 2012; Tomita et al., 2016; Zhang et al., 2016). However CCR2 expression on KCs is absent under healthy conditions, but increases in parallel to disease evolution, serving as diagnostic marker in the context of NAFLD (Krenkel et al., 2018). CCR2+ macrophages are also seen in patients with acetaminophen (APAP)-induced liver failure (Mossanen et al., 2016) and increased serum CCL2 levels are associated with adverse prognosis (Antoniades et al., 2012) serving as a potential biomarker also for ALF, mitigating its specificity. MDMs have been shown to regulate a number of aspects of liver injury, including perpetuation of inflammation (Wu et al., 2016; Krenkel and Tacke, 2017), but also promotion of fibrosis (Karlmark et al., 2009; Krenkel et al., 2018; Weston et al., 2019; Ramachandran et al., 2020). Both KCs and MDMs can adopt context-dependent fibrogenic and fibrolytic roles due to their heterogeneity and plasticity (Weston et al., 2019).
ScRNA-Seq revealed MDMs as CD68+MARCO− in comparison to CD68+CD163+MARCO+TIMD4+ KCs (MacParland et al., 2018; Aizarani et al., 2019; Ramachandran et al., 2019). A cross-species comparison suggested a conserved transcriptional gene signature among human and mouse KCs (Ramachandran et al., 2019; Ramachandran et al., 2020). Recent publications revealed that expression of TREM-1, an amplifier of inflammation, on macrophages promoted hepatic inflammation and fibrosis in mice and humans (Nguye n-Lefebvre et al., 2018; Perugorria et al., 2019). Moreover, TREM-1 promoted pro-inflammatory cytokine production and mobilization of inflammatory cells to the site of injury (Nguye n-Lefebvre et al., 2018). In human liver samples from patients with severe fibrosis an increase in the number of TREM-1+ cells in fibrotic areas was observed, compared to only slight TREM-1 expression in and around the hepatic sinusoid in normal liver tissue (Nguye n-Lefebvre et al., 2018). Interestingly, TREM-2 expression on monocytes and macrophages was located around fibrous septa, sinusoids and areas of inflammation in human cirrhotic liver (Perugorria et al., 2019). In a recent study, an unbiased scRNA-Seq approach helped to further characterize the heterogenous population of MDMs in humans. A new pro-fibrogenic scar-associated TREM2+CD9+MNDA+ subpopulation of macrophages (scar-associated macrophages, SAMs) was identified, which expanded in the liver in the context of fibrosis, originated from differentiating circulating monocytes (Ramachandran et al., 2019) and was distinct from KCs (Ramachandran et al., 2020). Spatial analysis located SAMs at fibrotic niches in livers of cirrhosis patients and showed expansion of SAMs (Ramachandran et al., 2012; Ramachandran et al., 2019). SAMs also promoted HSC collagen production and proliferation (Ramachandran et al., 2019; Ramachandran et al., 2020). Strikingly, mouse injury-associated macrophages show overlap in distinct marker genes observed in human SAMs, such as TREM2 and CD9, which are conserved across species (Ramachandran et al., 2019; Xiong et al., 2019). Recent scRNA-Seq combined with cellular indexing of transcriptomes and epitopes by sequencing (CITE-Seq) and spatial proteogenomics experiments on NAFLD mouse models showed the expansion of lipid-associated macrophages (LAMs) in the liver, originating from the BM prior to the development of fibrosis and cirrhosis (Remmerie et al., 2020a; Guilliams et al., 2022). The hepatic LAMs also showed some overlap with the SAMs in expression of Trem-2 and Cd9, but could be distinctively characterised by their expression of Spp1 (Remmerie et al., 2020a).
3.2.4 TAM receptor expression on macrophages
Moreover, macrophages expressing MERTK accumulated in the liver, but also the peritoneal cavity and mesenteric lymph nodes, in patients with decompensated cirrhosis and ACLF (Bernsmeier et al., 2015b). MERTK-expressing monocytes were characterised by increased expression of pro-resolution/anti-inflammatory and tissue- and lymph node-homing markers: HLA-DRhiCD163hiCX3CR1hiCCR7hi and Ki-67 negative, which suggests an origin from recruited monocytes (Bernsmeier et al., 2015b). MERTK-expressing monocytes showed attenuated cytokine responses and an enhanced migratory potential across endothelial layers, suggesting their capacity to infiltrate the liver and reverse migrate back to the circulation where they aggravate the systemic immuneparesis (Bernsmeier et al., 2015b; Triantafyllou et al., 2018). In the context of ALF, it has been shown that these MERTK-expressing macrophages in the liver exhibited enhanced efferocytosis and played a role in resolution of inflammation and tissue restoration (Bernsmeier et al., 2015b; Triantafyllou et al., 2018). Yet, MERTK expressing cells were essential to maintaining homeostasis in the cirrhotic liver, but aggravated systemic immuneparesis (Bernsmeier et al., 2015b; Triantafyllou et al., 2018). In a mouse model for non-alcoholic steatohepatitis (NASH) it was shown that AXL signalling in primary fibroblasts, hepatocytes and liver macrophages promoted fibrosis, while GAS6 or MERTK activation protected primary hepatocytes against lipid toxicity, and the AXL inhibitor bemcentinib diminished liver inflammation and fibrosis in mice (Tutusaus et al., 2020).
3.2.5 Function of macrophages in cirrhosis
The impact of aetiologies on the function of monocytes and macrophages at the stage of cirrhosis has not been extensively studied. NAFLD- and ARLD-related cirrhosis display a similar histopathology, pathophysiology, shared genetic and epigenetic factors and frequently coexist (Idalsoaga et al., 2020). Prior to the presence of cirrhosis, in ARLD and NAFLD, hepatic macrophages become activated by LPS, IFN-ɣ, and GM-CSF signalling and show pro-inflammatory cytokine/chemokine secretion (IL-6, IL-1β, and CCL2) (Mandrekar and Szabo, 2009; Gao et al., 2019; Remmerie et al., 2020b). In NAFLD, free fatty acids derived from white adipose tissue promoted hepatocyte triglyceride synthesis and storage as well as lipotoxicity with increased production of both pro-inflammatory cytokines such as TNF-α and IL-6 and macrophage recruiting chemokines such as CCL2, CCL5, and CXCL10 (Kazankov et al., 2019). NAFLD- and ARLD-related cirrhosis was associated with steatosis and hepatocyte cell death (apoptosis, necrosis and pyroptosis) promoting tissue inflammation and injury (Itoh et al., 2013; Gao et al., 2019; Miyata and Nagy, 2020). Autophagy can protect against ARLD tissue damage (Gao et al., 2019) by degrading interferon regulatory factor 1 (IRF1) and damaged mitochondria in hepatic macrophages (Ilyas et al., 2019; Liang et al., 2019; Williams and Ding, 2020). In non-cirrhotic viral hepatitis, KC activation led to the expression of IL-6, IFN-ɣ, and ROS, which in turn inhibited HCV replication and induced apoptosis of infected hepatocytes (Broering et al., 2008; Boltjes et al., 2014). In the setting of non-cirrhotic chronic HBV infection, an impaired immune response was associated with the release of IL-10 (Liu et al., 2018), reduced IL-12 expression (Wang et al., 2013) or increased expression of PD-L1 (Tian et al., 2016). However, in the context of cirrhosis, HCV also triggered the secretion of CCL5 by human macrophages and induced CCR5-dependent activation of HSCs (Sasaki et al., 2017). Patients with cirrhosis due to viral hepatitis showed increased numbers of hepatic macrophages associated with the infiltration of other pro-inflammatory CD14+HLA-DRhiCD206+ myeloid cells (Tan-Garcia et al., 2017), which in turn produced pro-inflammatory cytokines such as IL-1β, IL-18, and TNF-α (Weston et al., 2019).
Hepatic macrophages show tolerance during liver homeostasis and protect the liver and subsequently the systemic circulation from bacteria and bacterial products. In chronic liver disease and cirrhosis, KCs become activated and MDMs are recruited to the liver. Recently, due to new ‘omics’ technologies a list of macrophage markers associated with fibrosis, steatosis and monocyte recruitment could be identified. Hepatic macrophages show a very heterogenous phenotype expressing pro-inflammatory cytokines, but switching to a more immunoregulatory phenotype characterised by pro- and anti-inflammatory cytokines in advanced stages especially AD/ACLF. The further delineation of macrophages in relation to distinct aetiologies underlying cirrhosis and to specific stages of disease is the focus of ongoing research and the basis of potential future therapies.
3.3 Intestinal macrophages
Cirrhosis is a systemic disease that displays immunological alterations in immune cells beyond the liver. The gut connects to the liver by the biliary tract and portal vein, allowing for direct transfer of gut-derived components that impact liver pathophysiology (Hartmann et al., 2015) (Figure 2). In healthy conditions the intestinal mucosal and vascular barrier serve as an intersection for the interactions between the gut and the liver in order to limit systemic dissemination of microbes and toxins as well as to allow nutrients to access the circulation and liver (Albillos et al., 2020). Intestinal macrophages are a subset of CD33+CD14− lamina propria immune cells and are hyporesponsive to LPS (Smythies et al., 2005; Smith et al., 2011). Gut-resident CX3CR1+ macrophages contribute to host defence and barrier integrity through their phagocytic capacity (Ber nsmeier et al., 2020). Immunosuppressive cytokine IL-10 plays a key role in the regulatory functions of intestinal macrophages (Scott and Mann, 2020).
In cirrhosis, pathological BT from the gut to mesenteric lymph nodes is driven by a suppressed intestinal immune system with small intestinal bacterial overgrowth and reduced microbial diversity (Albillos et al., 2020; Chopyk and Grakoui, 2020). Following pathological BT in patients with decompensated cirrhosis, CD14+TREM1+iNOS+ intestinal monocyte-derived macrophages were recruited from the circulation into the duodenum via MCP-1 secreted by intestinal epithelial cells (Du Plessis et al., 2013). Functionally these activated macrophages were responsive to microbial challenges, which resulted in the production of pro-inflammatory cytokines (IL-23, IL-6, and TNF-α) and contribute to mucosal, epithelial, vascular and immunological barrier dysfunction in cirrhosis (Kamada et al., 2008; Wiest et al., 2017; Sarin et al., 2019; De Muynck et al., 2021). If all defence mechanisms fail, these processes manifest as infection e.g., SBP, bacteraemia and sepsis (Albillos et al., 2020; Ber nsmeier et al., 2020). In murine models of cirrhosis, the mucus layer was reduced in thickness, with a loss of goblet cells, decreased MUC2 expression and bacterial overgrowth. In a NAFLD murine model recent data indicated that a high-fat diet altered the microbiome and impaired the intestinal barrier and the gut vascular barrier, which was confirmed in human gut biopsies (Mouries et al., 2019). Also chronic alcohol intake in humans leads to intestinal inflammation and increased numbers of TNF-α producing monocytes and macrophages in the lamina propria enhancing the intestinal permeability (Chen et al., 2015). It can further lead to intestinal gut microbiota dysbiosis and impaired intestinal barrier integrity (Gao et al., 2019; Sarin et al., 2019). It was shown in humans and mice that chronic alcohol use suppressed intestinal regeneration through reduced antimicrobial Reg3b and Reg3g secretion by Paneth cells (Yan et al., 2011; Hartmann et al., 2013), which led to increased bacteria adhesion to mucosal surfaces, intestinal bacterial overgrowth, enhanced BT of viable bacteria and worsening of liver inflammation (Albillos et al., 2020). Analogously, in cirrhosis patients with chronic HBV and HCV an increased gut permeability and resulting BT was observed (Sandler et al., 2011), indicating shared mechanisms in NAFLD, ARLD and viral-related cirrhosis of the liver. The mechanisms leading to increased gut permeability are not fully understood, but nitration and oxidation of tubulin, damage to microtubule cytoskeleton and activation of iNOS and NF-κB have been shown to impact tight and adherens junctions in the intestinal epithelium (Rao, 2009; Yona et al., 2013; Hartmann et al., 2015; Gao et al., 2019).
In cirrhosis, dysfunctional immune cells are not limited to the liver and the circulation but extend to the innate immune barriers of the intestine. Dysbiosis in the gut and the compromised intestinal barrier led to BT to the liver and circulation, prompting and maintaining systemic inflammation, immuneparesis and bacterial infection susceptibility.
3.4 Peritoneal macrophages
Under healthy conditions peritoneal macrophages (PMs), i.e. macrophages in the peritoneal cavity fluid (PF) are constantly exchanged between blood and PF and clear debris as well as pathogens (Heel and Hall, 2005) (Figure 2). In cirrhosis ascites, the accumulation of fluid in the peritoneal cavity, and SBP may develop. SBP results from intestinal and peritoneal barrier failure, which allows viable bacteria to enter the peritoneal cavity (Van der Merwe et al., 2021) and can induce AD of the liver, ACLF, multiorgan failure and death. Three subsets of PMs have been defined: Classical CD14++CD16−, intermediate CD4++CD16+ and large granular CD14hiCD16hi (Ruiz-Alcaraz et al., 2016; Ruiz-Alcaraz et al., 2018). PMs highly express pattern recognition receptors (CD14, CD16), as well as phagocytosis receptor CD11b, cytokine receptors (CD116, CD119), T cell receptor ligand (HLA-DR), and co-stimulatory molecules (CD40, CD80) compared to circulating blood monocytes (Ruiz-Alcaraz et al., 2016; Ruiz-Alcaraz et al., 2018). Pathogens and bacterial products originating from BT from the gut may be absorbed by the peritoneal cavity and elicit an inflammatory reaction (Capobianco et al., 2017).
In regard to the aetiology, in ARLD cirrhosis PMs showed a more pro-inflammatory profile, while in hepatitis C higher levels of IL-12 and lower IL-10 levels were found (Tapia-Abellán et al., 2012). The expression of complement receptor of the immunoglobulin superfamily (CRIg) and CCR2 were found to define two phenotypically and functionally distinct peritoneal macrophage subpopulations (Irvine et al., 2016). CRIghi macrophages showed higher expression of efferocytosis receptors MERTK and TIMD4 and were on a functional level highly phagocytic and displayed enhanced antimicrobial effector activity compared to CRIglo macrophages (Irvine et al., 2016). Consequently, a high proportion of CRIghi macrophages was associated with reduced morbidity and mortality (Irvine et al., 2016). Another group defined PM subsets as large PMs (LPMs, CD206+CD163+) and small PMs (SPMs, CD206-), which differed in granularity and maturation markers in ascites samples from patients with cirrhosis (Stengel et al., 2020). LPMs had an inflammatory phenotype, were less susceptible to tolerance induction and released more TNF-α than SPMs. TLR stimulation and live bacteria altered levels of CD206 on the surface and resulted in release of soluble CD206 (sCD206) (Stengel et al., 2020). In the early phase of SBP, LPMs were lost, but their abundance was reversible and increased after treatment (Stengel et al., 2020). PMs co-expressed classical M1 and M2 markers illustrating their functional plasticity (Ruiz‐Alcaraz et al., 2020) and the potential of the ascitic microenvironment to dynamically alter the macrophage phenotype in order to suit the insult. In the event of BT, increased pro-inflammatory cytokine production and increased iNOS/NO production (Van der Merwe et al., 2021) and a reduced HLA-DR expression in CD14+ PMs was associated with adverse prognosis (Frances, 2004; Fagan et al., 2015). A counter-regulatory process resulted in ascitic IL-10, resin and reduced expression of CD14, CD16, HLA-DR, CD86, and CD206 on PMs (Lesińska et al., 2014; Nieto et al., 2015; Nieto et al., 2018; Hadjivasilis et al., 2021). The reduced CD14 expression on PMs in SBP conditions was associated with impaired phagocytosis (Nieto et al., 2015; Nieto et al., 2018). When comparing peripheral circulating monocytes in stable cirrhosis conditions to PMs of patient with ACLF increased immunoregulatory MERTK-expression population was found, indicating a more suppressive phenotype of PMs in ACLF patients (Bernsmeier et al., 2015b; Flint et al., 2022).
Taken together, in the peritoneal fluid from patients with decompensated cirrhosis complicated by ascites, two distinct PM populations have been identified. LPMs have a pro-inflammatory phenotype (CD14+CD206+CCR2−CRIg+MERTK+), are less susceptible to tolerance induction, and release more pro-inflammatory cytokines (TNF-α) compared to SPMs. In SBP conditions LPMs release sCD206 and the loss of LPMs occurs, while the number of SPMs (CD14+CD206−CCR2+/−MERTK-) increases. Due to the technical challenge to date, PM from patients with compensated cirrhosis without ascitic fluid have not been characterised phenotypically and functionally. This comparison may help to identify immunological and barrier switches in the progression from compensated to decompensated stages of cirrhosis, since ascites is considered as the hallmark of decompensation (D’Amico et al., 2022).
3.5 Macrophages in other tissues
As a systemic disorder, cirrhosis can involve a number of other organs beyond the circulation, liver, gut and peritoneum.
Lymphoid organs like the spleen and lymph nodes harbour mononuclear phagocytes among other immune cells, which are also be affected in cirrhosis patients. In cirrhosis defective immune cells in mesenteric lymph nodes facilitate BT in decompensation stage. A study showed the accumulation of immune-suppressive MERTK-expressing macrophages in the subcapsular sinus and medullary cord in the lymph nodes of patients with AD/ACLF (Bernsmeier et al., 2015b). In the spleen of patients with cirrhosis and PH phagocytosis activity of macrophages in the red pulp and marginal zone was enhanced and related to hypersplenism and cytopenia (Yongxiang et al., 2002). There is evidence also for a spleen-liver crosstalk, given a study showed reduced fibrosis, monocyte infiltration within the injured liver and CCL2 secretion by hepatic macrophages in cirrhosis patients that had undergone splenectomy (Li et al., 2018).
Adipose tissue macrophages (ATMs) have been intensively investigated over the last years in mouse models or in patients with obesity and NAFLD, however not in the context of cirrhosis. In obesity, the adipose tissue mechanism for lipid containment fails leading to dyslipidemia and insulin resistance (Korf et al., 2019b; Remmerie et al., 2020a). Dying adipocytes release DAMPs and cause adipose tissue macrophage (ATM) activation, which form crown like structures around necrotic adipocytes to engulf cell debris (Korf et al., 2019b; Remmerie et al., 2020a). ATMs secrete pro-inflammatory cytokines (TNF-α, IL-6, and IL-1β), promote monocyte recruitment via MCP-1, increase insulin resistance, hepatic lipid flux and lipid accumulation and are associated with disease progression of NAFLD (Du Plessis et al., 2015; Lefere and Tacke, 2019). The CCL2-CCR2 and CCL5-CCR5 chemokine signalling axes stimulates monocyte recruitment to insulted adipose tissue sites and has been suggested as therapeutic target to resolve inflammation in NAFLD (Kazankov et al., 2019).
Overall, the study of macrophages in the context of cirrhosis in organs other than the liver, circulation or peritoneum remain underrepresented. This may be a result of the difficulty to obtain these biological materials such as lymph nodes, spleen, adipose and other tissue from patients. There is a substantial need to investigate immune processes systematically in diverse tissues in order to understand the plasticity of monocytes and macrophages in the human body in the context of cirrhosis.
4 Potential monocyte and macrophage derived markers of immuneparesis in cirrhosis
Immuneparesis in patients with cirrhosis develops as a consequence of persisting systemic inflammation, which attenuates the responses of immune cells required to combat infections (Bonnel et al., 2011; Bajaj et al., 2012; Albillos et al., 2014). Monocyte and macrophage plasticity in tissues and the systemic circulation play a key role in the regulation of tissue homeostasis and defence against infection. Therefore, future prognostic markers indicating the state of immuneparesis would be helpful in clinical decision making.
The soluble form of two scavenger receptors CD163 and CD206 (sCD163 and sMR/sCD206), expressed by circulating blood monocytes and macrophages, were present in plasma, ascites and other body fluids (Grønbæk et al., 2020; Nielsen et al., 2020) and associated with severity and prognosis of chronic liver diseases (Grønbæk et al., 2016; Kazankov et al., 2016; Rainer et al., 2018; Grønbæk et al., 2020; Nielsen et al., 2020; Stengel et al., 2020) and cirrhosis (Holland-Fischer et al., 2011; Grønbaek et al., 2012; Rode et al., 2013; Waidmann et al., 2013). High sCD163 levels were additionally associated with variceal bleeding, but also predicted mortality in alcoholic hepatitis (Waidmann et al., 2013; Saha et al., 2019). However, sCD163 and sMR plasma/serum levels have been associated with a variety of other diseases and infection conditions (Andersen et al., 2018; Suzuki et al., 2018; Gong et al., 2021; Arneth and Kraus, 2022; Davidsson et al., 2022) as shedding of CD163 and CD206 is associated with macrophage activation (Grønbæk et al., 2020; Nielsen et al., 2020) in general and does not specifically indicate immuneparesis in patients with cirrhosis. Moreover, sCD163 plasma levels differed in relation to the underlying aetiologies (Wang et al., 2015) further mitigating their clinical significance. Given their secretion by LPM, sCD163, and sMR may potentially enhance the diagnostic criteria for SBP in ascitic fluid of cirrhosis patients (Stengel et al., 2020).
AXL expressing immune-regulatory monocytes have been shown to increase in patients during progression of cirrhosis independent of the aetiology and prior to AD and related infectious complications, as well as the future onset of AD and mortality (Brenig et al., 2020). Therefore, the flow cytometry-based analysis of AXL expression on monocytes in patients with cirrhosis might serve as a marker of immuneparesis and identify patients at risk for infection and deterioration. Also its soluble form sAXL was increased in the plasma of cirrhosis patients correlating with cirrhosis progression (Brenig et al., 2020). However, sAXL has been suggested not only as a marker for advanced fibrosis/cirrhosis but also HCC development (Dengler et al., 2017; Staufer et al., 2017). Elevated sAXL levels were not detected in patients with chronic liver diseases of diverse underlying aetiologies, yet patients with advanced fibrosis (F3), cirrhosis (F4) (54.67–94.74 ng/ml) and HCC (82.7–114.5 ng/ml) displayed higher levels compared to healthy controls (40.15 ng/ml) (Dengler et al., 2017). By contrast, MERTK expression has been identified as a marker both for immunosuppressive circulating monocytes and for macrophages in the liver, peritoneum and mesenteric lymph nodes in acute decompensation of cirrhosis (AD/ACLF) (Bernsmeier et al., 2015b). Thus, in addition to AXL expression, flow cytometry-based MERTK expression on monocytes may represent a valuable marker of immuneparesis and indicating patients with AD/ACLF.
The progression from compensated to decompensated stages of cirrhosis is associated with a poor prognosis and a high mortality (D’Amico et al., 2022). Given the increase in circulating neutrophils and concomitant decrease in lymphocyte numbers is a response to stress including sepsis (de Jager et al., 2010), the neutrophil-lymphocyte ratio (NLR) and monocyte-lymphocyte ratio (MLR) have been developed as prognostic markers for patient stratification. Patients with AD and ACLF who died during hospitalisation displayed elevated NLR and MLR (Bernsmeier et al., 2020). In ACLF patients, an NLR >30 was associated with an 80% 3-months mortality risk (Bernsmeier et al., 2020). Thus, NLR and MLR may be used as rapidly available robust initial diagnostic tools for patient stratification in AD/ACLF.
5 Therapeutic modulation of monocyte and macrophage function in immuneparesis
Along cirrhosis progression patients develop multiple complications (PH, inflammation, BT, gut dysbiosis) that predispose them to decompensation and subsequent precipitating events (infection, variceal haemorrhage) lead to AD (Gustot et al., 2021).
The association of immuneparesis with infectious complications, decompensation and mortality has evoked the concept of immunomodulatory therapies as an adjuvant concept in order to prevent decompensation, save organs and reduce mortality in patients with cirrhosis. Over the previous decade, several potential targets for immunotherapy have been defined (Table 1).
Firstly, therapies with G-CSF have gained interest. These therapies aim to improve liver regeneration and innate immune responses in cirrhosis through the release of bone marrow-derived CD34+ haematopoietic stem cells in order to replace the dysfunctional circulating monocytes and have been studied intensively in ACLF patients (Kedarisetty et al., 2015; Newsome et al., 2018; Verma et al., 2018). However, distinct clinical studies showed differing outcomes. On one hand G-CSF improved the survival of patients (Garg et al., 2012; Verma et al., 2018), while on the other hand G-CSF with haemopoietic stem-cell infusion did not improve liver dysfunction and was associated with increased frequency of adverse events (Newsome et al., 2018). Most recently, a large prospective multicentre trial failed to show beneficial effects of G-CSF over standard of care alone (Engelmann et al., 2021). Thus, G-CSF treatment cannot be recommended at any stage of cirrhosis to date and may require further detailed investigation.
Another therapeutic approach may involve inhibition of macrophage activation via pattern recognition receptors by TLR4 inhibition using TAK-242 or SERELAXIN. Proof of concept studies of TLR4 inhibition previously revealed reduced liver injury and inflammation in rodent models (Seki et al., 2007; Shah et al., 2013; Bennett et al., 2017; Engelmann et al., 2020). In a similar fashion the NLRP3 inflammasome inhibitor MCC950 reduced liver inflammation and fibrosis in experimental NAFLD models (Mridha et al., 2017). These concepts await further evaluation in human in vitro models and clinical studies.
Ex vivo data obtained from patients at different stages of cirrhosis support the potential strategy of targeting TAM receptors AXL and MERTK (Bernsmeier et al., 2015b; Brenig et al., 2020). The TAM receptor inhibitors UNC569 (MERTK) and BGB324 (AXL) augmented pro-inflammatory cytokine response in ex vivo models (Bernsmeier et al., 2015b; Brenig et al., 2020) and therefore deserve evaluation in vivo models of cirrhosis and ACLF. TAM receptor inhibitors are in clinical evaluation (phase 2 studies) for different malignant diseases and may eventually be translated to liver disease given their known safety profiles. Another candidate is the established diabetes drug metformin, which led to reduced AXL expression on monocytes and enhanced inflammatory cytokine responses in vitro (Brenig et al., 2020). Moreover, the use of metformin in patients with cirrhosis and diabetes appeared safe and was associated with reduced mortality, HCC or decompensation (Kaplan et al., 2021).
Another candidate for immunotherapy may be the TLR3 agonist poly (I:C), which reduced proportions of M-MDSCs and improved their anti-microbial function in vitro (Bernsmeier et al., 2018). Poly (I:C) has been used as an adjuvant for vaccination and thus in vivo studies for the treatment of cirrhosis may be considered.
In addition, feeding of glutamine into the tricarboxylic acid cycle by using a pharmacological inhibitor of glutamine synthetase (GLUL) restored innate immune responses, in particular the phagocytic and inflammatory capacities of monocytes from patients with ACLF (Korf et al., 2019a).
The therapeutic effects of inhibiting monocyte infiltration by using cenicriviroc (CVC), an oral dual chemokine receptor CCR2/CCR5 antagonist, were assessed using mouse models (Krenkel et al., 2018). In all murine models for steatohepatitis, as well as liver fibrosis progression and fibrosis regression, CVC treatment reduced the recruitment of MDMs (Krenkel et al., 2018). Furthermore, CVC did not affect macrophage polarization, hepatocyte fatty acid metabolism or HSC activation (Krenkel et al., 2018). The inhibition of CCR2+ monocyte recruitment shows potential to improve fibrosis in patients with NASH (Krenkel et al., 2018), but needs further evaluation for the potential of cirrhosis reversibility and its effect on cirrhosis due to other underlying liver diseases.
PAMP-mediated macrophage activation may also be prevented by the restoration of a normal gut microbiome using antibiotics and probiotics (Mazagova et al., 2015; Irvine et al., 2019; Wen et al., 2021). Novel approaches to antibiotic prophylaxis have been explored, including strategies targeting intestinal dysbiosis, including non-selective decontamination (rifaximin), probiotics and faecal microbiota transplantation. Moreover, intestinal motility and barrier function may be improved by the use of prokinetics, beta-blockers, and bile acids (Yan and Garcia-Tsao, 2016). Treatments improving intestinal homeostasis could beneficially impact systemic immune function by reducing exposure to pathological taxa and chronic immune stimulation. Many antibiotics, including those prophylactically used in patients with decompensated cirrhosis, have direct impact on the immune system, although the underlying mechanisms are not well-defined (Zapater et al., 2015).
In addition, the cyclooxygenase (COX)-derived eicosanoid prostaglandin E2 (PGE2) was elevated in the plasma of decompensated cirrhosis patients in association with suppressed cytokine responses and bacterial killing by macrophages in vitro (O'Brien et al., 2014). Albumin and COX inhibitors modulated endosomal TLR signalling and improved pro-inflammatory cytokine production (O'Brien et al., 2014; China et al., 2018; Casulleras et al., 2020). An in vitro study revealed that the augmentation of serum albumin above 30 g/L was associated with the reversibility of plasma-mediated immune dysfunction by binding and inactivation PGE2 (China et al., 2018). Intravenous albumin had however no effect on systemic inflammation, albumin function, cardiovascular mediators and biomarkers compared with standard of care in hospitalized decompensated cirrhosis (China et al., 2022). To date, the standard of care remains the treatment with albumin in addition to appropriate antibiotics in SBP (Bajaj et al., 2021) as well as in acute kidney injury. Further studies may evaluate the additive use of COX inhibitors and their potential to improve immuneparesis and restore cytokine production in cirrhosis patients.
In summary, there are a variety of immunomodulatory strategies in clinical evaluation which may shape the future therapy of patients with cirrhosis by improving their antimicrobial defence, and hereby hopefully save organs and reduce mortality.
6 Conclusion
In conclusion, research over the last decade has significantly improved our understanding of the plasticity of monocyte and macrophage differentiation in relation to different compartments and clinical conditions. Their relevance in immuneparesis development in patients with cirrhosis as well as their prognostic value is substantial, and thus supports the concept of adjuvant immune-modulatory treatments. This review specifically summarizes findings originating from patient data or distinctly describing the translation from mouse to human findings. To date immune-pathophysiological findings in humans with cirrhosis are little due to the limitation in assessing samples. Furthermore, translation of mouse related findings to human have to be drawn carefully due to the lack of a proper cirrhosis mouse model. Methodological advances such as single-cell based technologies have enabled the identification of tissue specific mononuclear phagocytic subsets in the context of their functions in limited samples from patients with liver cirrhosis. However, further studies are needed to clarify monocyte and macrophage function also in relation to the diverse underlying aetiologies and in diverse compartments other than the liver and the circulation. Decompensation of cirrhosis is accompanied with the development of diverse complications, such as ascites and infections. Infections, which are due to a dysfunctional immune response, in turn represent a great risk to patients with decompensated cirrhosis. Most of these infections originate from barrier dysfunction in the gut and peritoneum, which result in BT to the circulation. In addition to the inflamed liver, BT has the potential to change the milieu in the gut, liver, circulation and peritoneum and affects tissue specific differentiation of monocytes and macrophages. Treatment with antibiotics and albumin have reduced the mortality in case of infection, but antibiotics should be prescribed with caution given the increasing prevalence of MDRO in cirrhosis patients. Research over the past years has identified not only potential prognostic molecular markers to better predict cirrhosis progression and immuneparesis development in patients, but also molecules on immune cells which may be targeted in order to restore immune function and prevent infectious episodes. The development of immune-modulatory treatments however is challenging given the tissue-specific plasticity of immune cells and requires careful evaluation in vivo. At present, experimental studies and clinical trials aimed at either identifying novel immunotherapeutic targets or delineating beneficial effects of distinct immune-modulatory treatments from their off-target effects are ongoing.
Author contributions
AG and CB contributed to the conception of the review. EF designed Figure 2 and Table 1. AG wrote the first draft of the manuscript under supervision of CB. All authors read and approved the submitted version.
Funding
CB is supported by the Swiss National Foundation (SNF), project numbers 320030_159984 and 320030_189072.
Conflict of interest
The authors declare that the research was conducted in the absence of any commercial or financial relationships that could be construed as a potential conflict of interest.
Publisher’s note
All claims expressed in this article are solely those of the authors and do not necessarily represent those of their affiliated organizations, or those of the publisher, the editors and the reviewers. Any product that may be evaluated in this article, or claim that may be made by its manufacturer, is not guaranteed or endorsed by the publisher.
References
Aizarani, N., Saviano, A., Sagar, M., Mailly, L., Durand, S., Herman, J. S., et al. (2019). A human liver cell atlas reveals heterogeneity and epithelial progenitors. Nature 572 (7768), 199–204. doi:10.1038/s41586-019-1373-2
Albillos, A., De Gottardi, A., and Rescigno, M. (2020). The gut-liver axis in liver disease: Pathophysiological basis for therapy. J. Hepatology 72 (3), 558–577. doi:10.1016/j.jhep.2019.10.003
Albillos, A., Lario, M., and Álvarez-Mon, M. (2014). Cirrhosis-associated immune dysfunction: Distinctive features and clinical relevance. J. Hepatology 61 (6), 1385–1396. doi:10.1016/j.jhep.2014.08.010
Andersen, M. N., Hønge, B. L., Jespersen, S., Medina, C., da Silva Té, D., Laursen, A., et al. (2018). Soluble macrophage mannose receptor (sCD206/sMR) as a biomarker in human immunodeficiency virus infection. J. Infect. Dis. 218 (8), 1291–1295. doi:10.1093/infdis/jiy318
Antoniades, C. G., Quaglia, A., Taams, L. S., Mitry, R. R., Hussain, M., Abeles, R., et al. (2012). Source and characterization of hepatic macrophages in acetaminophen-induced acute liver failure in humans. Hepatology 56 (2), 735–746. doi:10.1002/hep.25657
Arneth, B., and Kraus, J. (2022). Experimental laboratory biomarkers in multiple sclerosis. Wien Med. Wochenschr 7. doi:10.1007/s10354-022-00920-7
Arroyo, V., Angeli, P., Moreau, R., Jalan, R., Clària, J., Trebicka, J., et al. (2021). The systemic inflammation hypothesis: Towards a new paradigm of acute decompensation and multiorgan failure in cirrhosis. J. Hepatology 74 (3), 670–685. doi:10.1016/j.jhep.2020.11.048
Arroyo, V., Moreau, R., and Jalan, R. (2020). Acute-on-Chronic liver failure. N. Engl. J. Med. 382 (22), 2137–2145. doi:10.1056/nejmra1914900
Arvaniti, V., D'Amico, G., Fede, G., Manousou, P., Tsochatzis, E., Pleguezuelo, M., et al. (2010). Infections in patients with cirrhosis increase mortality four-fold and should Be used in determining prognosis. Gastroenterology 139 (4), 1246–1256. e5. doi:10.1053/j.gastro.2010.06.019
Aspinall, A. I., Curbishley, S. M., Lalor, P. F., Weston, C. J., Miroslava, B, Liaskou, E., et al. (2010). CX3CR1 and vascular adhesion protein-1-dependent recruitment of CD16+ monocytes across human liver sinusoidal endothelium. Hepatology 51 (6), 2030–2039. doi:10.1002/hep.23591
Asrani, S. K., Devarbhavi, H., Eaton, J., and Kamath, P. S. (2019). Burden of liver diseases in the world. J. Hepatology 70 (1), 151–171. doi:10.1016/j.jhep.2018.09.014
Assimakopoulos, S. F., Tsamandas, A. C., Tsiaoussis, G. I., Karatza, E., Triantos, C., Vagianos, C. E., et al. (2012). Altered intestinal tight junctions' expression in patients with liver cirrhosis: A pathogenetic mechanism of intestinal hyperpermeability. Eur. J. Clin. Investigation 42 (4), 439–446. doi:10.1111/j.1365-2362.2011.02609.x
Bain, C. C., Bravo-Blas, A., Scott, C. L., Gomez Perdiguero, E., Geissmann, F., Henri, S., et al. (2014). Constant replenishment from circulating monocytes maintains the macrophage pool in the intestine of adult mice. Nat. Immunol. 15 (10), 929–937. doi:10.1038/ni.2967
Bain, C. C., Scott, C. L., Uronen-Hansson, H., Gudjonsson, S., Jansson, O., Grip, O., et al. (2013). Resident and pro-inflammatory macrophages in the colon represent alternative context-dependent fates of the same Ly6Chi monocyte precursors. Mucosal Immunol. 6 (3), 498–510. doi:10.1038/mi.2012.89
Bajaj, J. S., Heuman, D. M., Hylemon, P. B., Sanyal, A. J., White, M. B., Monteith, P., et al. (2014). Altered profile of human gut microbiome is associated with cirrhosis and its complications. J. Hepatology 60 (5), 940–947. doi:10.1016/j.jhep.2013.12.019
Bajaj, J. S., Kamath, P. S., and Reddy, K. R. (2021). The evolving challenge of infections in cirrhosis. N. Engl. J. Med. 384 (24), 2317–2330. doi:10.1056/nejmra2021808
Bajaj, J. S., O'Leary, J. G., Reddy, K. R., Wong, F., Olson, J. C., Subramanian, R. M., et al. (2012). Second infections independently increase mortality in hospitalized patients with cirrhosis: The north American consortium for the study of end-stage liver disease (NACSELD) experience. Hepatology 56 (6), 2328–2335. doi:10.1002/hep.25947
Bajaj, J. S., Reddy, R. K., Tandon, P., Wong, F., Kamath, P. S., Biggins, S. W., et al. (2018). Prediction of fungal infection development and their impact on survival using the NACSELD cohort. Am. J. Gastroenterol. 113 (4), 556–563. doi:10.1038/ajg.2017.471
Balmer, M. L., Slack, E., de Gottardi, A., Lawson, M. A., Hapfelmeier, S., Miele, L., et al. (2014). The liver may act as a firewall mediating mutualism between the host and its gut commensal microbiota. Sci. Transl. Med. 6 (6), 237ra66. doi:10.1126/scitranslmed.3008618
Bataller, R., and Brenner, D. A. (2005). Liver fibrosis. J. Clin. Invest. 115 (2), 209–218. doi:10.1172/jci24282
Beattie, L., Sawtell, A., Mann, J., Frame, T. C. M., Teal, B., de Labastida Rivera, F., et al. (2016). Bone marrow-derived and resident liver macrophages display unique transcriptomic signatures but similar biological functions. J. Hepatology 65 (4), 758–768. doi:10.1016/j.jhep.2016.05.037
Bennett, R. G., Simpson, R. L., and Hamel, F. G. (2017). Serelaxin increases the antifibrotic action of rosiglitazone in a model of hepatic fibrosis. Wjg 23 (22), 3999. doi:10.3748/wjg.v23.i22.3999
Bernsmeier, C., van der Merwe, S., and Périanin, A. (2020). Innate immune cells in cirrhosis. J. Hepatology 73 (1), 186–201. doi:10.1016/j.jhep.2020.03.027
Bernsmeier, C., Cavazza, A., Fatourou, E. M., Theocharidou, E., Akintimehin, A., Baumgartner, B., et al. (2020). Leucocyte ratios are biomarkers of mortality in patients with acute decompensation of cirrhosis and acute-on-chronic liver failure. Aliment. Pharmacol. Ther. 52 (5), 855–865. doi:10.1111/apt.15932
Bernsmeier, C., Pop, O. T., Singanayagam, A., Triantafyllou, E., Patel, V. C., Weston, C. J., et al. (2015). Patients with acute-on-chronic liver failure have increased numbers of regulatory immune cells expressing the receptor tyrosine kinase MERTK. Gastroenterology 148 (3), 603–615. e14. doi:10.1053/j.gastro.2014.11.045
Bernsmeier, C., Singanayagam, A., Patel, V. C., Wendon, J., and Antoniades, C. G. (2015). Immunotherapy in the treatment and prevention of infection in acute-on-chronic liver failure. Immunotherapy 7 (6), 641–654. doi:10.2217/imt.15.27
Bernsmeier, C., Triantafyllou, E., Brenig, R., Lebosse, F. J., Singanayagam, A., Patel, V. C., et al. (2018). CD14+ CD15− HLA-DR− myeloid-derived suppressor cells impair antimicrobial responses in patients with acute-on-chronic liver failure. Gut 67 (6), 1155–1167. doi:10.1136/gutjnl-2017-314184
Berres, M.-L., Schnyder, B., Yagmur, E., Inglis, B., Stanzel, S., Tischendorf, J. J. W., et al. (2009). Longitudinal monocyte Human leukocyte antigen-DR expression is a prognostic marker in critically ill patients with decompensated liver cirrhosis. Liver Int. 29 (4), 536–543. doi:10.1111/j.1478-3231.2008.01870.x
Berry, P. A., Antoniades, C. G., Carey, I., McPhail, M. J. W., Hussain, M. J., Davies, E. T., et al. (2011). Severity of the compensatory anti-inflammatory response determined by monocyte HLA-DR expression may assist outcome prediction in cirrhosis. Intensive Care Med. 37 (3), 453–460. doi:10.1007/s00134-010-2099-7
Blériot, C., and Ginhoux, F. (2019). Understanding the heterogeneity of resident liver macrophages. Front. Immunol. 10, 2694. doi:10.3389/fimmu.2019.02694
Boettcher, S., and Manz, M. G. (2017). Regulation of inflammation- and infection-driven hematopoiesis. Trends Immunol. 38 (5), 345–357. doi:10.1016/j.it.2017.01.004
Boltjes, A., Movita, D., Boonstra, A., and Woltman, A. M. (2014). The role of Kupffer cells in Hepatitis B and hepatitis C virus infections. J. Hepatology 61 (3), 660–671. doi:10.1016/j.jhep.2014.04.026
Bonnardel, J., T’Jonck, W., Gaublomme, D., Browaeys, R., Scott, C. L., Martens, L., et al. (2019). Stellate cells, hepatocytes, and endothelial cells imprint the kupffer cell identity on monocytes colonizing the liver macrophage niche. Immunity 51 (4), 638–654. e9. doi:10.1016/j.immuni.2019.08.017
Bonnel, A. R., Bunchorntavakul, C., and Reddy, K. R. (2011). Immune dysfunction and infections in patients with cirrhosis. Clin. Gastroenterology Hepatology 9 (9), 727–738. doi:10.1016/j.cgh.2011.02.031
Borzio, M., Salerno, F., Piantoni, L., Cazzaniga, M., Angeli, P., Bissoli, F., et al. (2001). Bacterial infection in patients with advanced cirrhosis: A multicentre prospective study. Dig. Liver Dis. 33 (1), 41–48. doi:10.1016/s1590-8658(01)80134-1
Bosmann, M., and Ward, P. A. (2013). The inflammatory response in sepsis. Trends Immunol. 34 (3), 129–136. doi:10.1016/j.it.2012.09.004
Brenig, R., Pop, O. T., Triantafyllou, E., Geng, A., Singanayagam, A., Perez-Shibayama, C., et al. (2020). Expression of AXL receptor tyrosine kinase relates to monocyte dysfunction and severity of cirrhosis. Life Sci. Alliance 3 (1), e201900465. doi:10.26508/lsa.201900465
Broering, R., Wu, J., Meng, Z., Hilgard, P., Lu, M., Trippler, M., et al. (2008). Toll-like receptor-stimulated non-parenchymal liver cells can regulate hepatitis C virus replication. J. Hepatology 48 (6), 914–922. doi:10.1016/j.jhep.2008.01.028
Bronte, V., Brandau, S., Chen, S.-H., Colombo, M. P., Frey, A. B., Greten, T. F., et al. (2016). Recommendations for myeloid-derived suppressor cell nomenclature and characterization standards. Nat. Commun. 7, 12150. doi:10.1038/ncomms12150
Calderon, B., Carrero, J. A., Ferris, S. T., Sojka, D. K., Moore, L., Epelman, S., et al. (2015). The pancreas anatomy conditions the origin and properties of resident macrophages. J. Exp. Med. 212 (10), 1497–1512. doi:10.1084/jem.20150496
Capobianco, A., Cottone, L., Monno, A., Manfredi, A. A., and Rovere-Querini, P. (2017). The peritoneum: Healing, immunity, and diseases. J. Pathol. 243 (2), 137–147. doi:10.1002/path.4942
Casulleras, M., Flores-Costa, R., Duran-Güell, M., Alcaraz-Quiles, J., Sanz, S., Titos, E., et al. (2020). Albumin internalizes and inhibits endosomal TLR signaling in leukocytes from patients with decompensated cirrhosis. Sci. Transl. Med. 12 (566). doi:10.1126/scitranslmed.aax5135
Chang, T.-T., Liaw, Y.-F., Wu, S.-S., Schiff, E., Han, K.-H., Lai, C.-L., et al. (2010). Long-term entecavir therapy results in the reversal of fibrosis/cirrhosis and continued histological improvement in patients with chronic Hepatitis B. Hepatology 52 (3), 886–893. doi:10.1002/hep.23785
Chávez-Galán, L., Olleros, M. L., Vesin, D., and Garcia, I. (2015). Much more than M1 and M2 macrophages, there are also CD169(+) and TCR(+) macrophages. Front. Immunol. 6, 263. doi:10.3389/fimmu.2015.00263
Chen, P., Stärkel, P., Turner, J. R., Ho, S. B., and Schnabl, B. (2015). Dysbiosis‐induced intestinal inflammation activates tumor necrosis factor receptor I and mediates alcoholic liver disease in mice. Hepatology 61 (3), 883–894. doi:10.1002/hep.27489
China, L., Becares, N., Rhead, C., Tittanegro, T., Freemantle, N., and O'Brien, A. (2022). Targeted albumin infusions do not improve systemic inflammation or cardiovascular function in decompensated cirrhosis. Clin. Transl. Gastroenterology 13 (5), e00476. doi:10.14309/ctg.0000000000000476
China, L., Maini, A., Skene, S. S., Shabir, Z., Sylvestre, Y., Colas, R. A., et al. (2018). Albumin counteracts immune-suppressive effects of lipid mediators in patients with advanced liver disease. Clin. Gastroenterology Hepatology 16 (5), 738–747. e7. doi:10.1016/j.cgh.2017.08.027
Chong, S. Z., Evrard, M., Devi, S., Chen, J., Lim, J. Y., See, P., et al. (2016). CXCR4 identifies transitional bone marrow premonocytes that replenish the mature monocyte pool for peripheral responses. J. Exp. Med. 213 (11), 2293–2314. doi:10.1084/jem.20160800
Chopyk, D. M., and Grakoui, A. (2020). Contribution of the intestinal microbiome and gut barrier to hepatic disorders. Gastroenterology 159 (3), 849–863. doi:10.1053/j.gastro.2020.04.077
Condamine, T., Mastio, J., and Gabrilovich, D. I. (2015). Transcriptional regulation of myeloid-derived suppressor cells. J. Leukoc. Biol. 98 (6), 913–922. doi:10.1189/jlb.4ri0515-204r
Cros, J., Cagnard, N., Woollard, K., Patey, N., Zhang, S.-Y., Senechal, B., et al. (2010). Human CD14dim monocytes patrol and sense nucleic acids and viruses via TLR7 and TLR8 receptors. Immunity 33 (3), 375–386. doi:10.1016/j.immuni.2010.08.012
D'Amico, G., Garcia-Tsao, G., and Pagliaro, L. (2006). Natural history and prognostic indicators of survival in cirrhosis: A systematic review of 118 studies. J. Hepatology 44 (1), 217–231. doi:10.1016/j.jhep.2005.10.013
D'Amico, G., Morabito, A., D'Amico, M., Pasta, L., Malizia, G., Rebora, P., et al. (2018). Clinical states of cirrhosis and competing risks. J. Hepatology 68 (3), 563–576. doi:10.1016/j.jhep.2017.10.020
D’Amico, G., Bernardi, M., and Angeli, P. (2022). Towards a new definition of decompensated cirrhosis. J. Hepatology 76 (1), 202–207.
Davidsson, S., Huotilainen, S., Carlsson, J., and Sundqvist, P. (2022). Soluble levels of CD163, PD-L1, and IL-10 in renal cell carcinoma patients. Diagn. (Basel) 12 (2). doi:10.3390/diagnostics12020336
de Jager, C. P., van Wijk, P. T., Mathoera, R. B., de Jongh-Leuvenink, J., van der Poll, T., and Wever, P. C. (2010). Lymphocytopenia and neutrophil-lymphocyte count ratio predict bacteremia better than conventional infection markers in an emergency care unit. Crit. Care 14 (5), R192. doi:10.1186/cc9309
De Muynck, K., Vanderborght, B., Van Vlierberghe, H., and Devisscher, L. (2021). The gut-liver Axis in chronic liver disease: A macrophage perspective. Cells 10 (11). doi:10.3390/cells10112959
DeLeve, L. D. (2015). Liver sinusoidal endothelial cells in hepatic fibrosis. Hepatology 61 (5), 1740–1746. doi:10.1002/hep.27376
Dengler, M., Staufer, K., Huber, H., Stauber, R., Bantel, H., Weiss, K. H., et al. (2017). Soluble axl is an accurate biomarker of cirrhosis and hepatocellular carcinoma development: Results from a large scale multicenter analysis. Oncotarget 8 (28), 46234–46248. doi:10.18632/oncotarget.17598
Du Plessis, J., van Pelt, J., Korf, H., Mathieu, C., van der Schueren, B., Lannoo, M., et al. (2015). Association of adipose tissue inflammation with histologic severity of nonalcoholic fatty liver disease. Gastroenterology 149 (3), 635–648. e14. doi:10.1053/j.gastro.2015.05.044
Du Plessis, J., Vanheel, H., Janssen, C. E. I., Roos, L., Slavik, T., Stivaktas, P. I., et al. (2013). Activated intestinal macrophages in patients with cirrhosis release NO and IL-6 that may disrupt intestinal barrier function. J. Hepatology 58 (6), 1125–1132. doi:10.1016/j.jhep.2013.01.038
Engelmann, C., Herber, A., Franke, A., Bruns, T., Reuken, P., Schiefke, I., et al. (2021). Granulocyte-colony stimulating factor (G-CSF) to treat acute-on-chronic liver failure: A multicenter randomized trial (graft study). J. Hepatology 75 (6), 1346–1354. doi:10.1016/j.jhep.2021.07.033
Engelmann, C., Sheikh, M., Sharma, S., Kondo, T., Loeffler-Wirth, H., Zheng, Y. B., et al. (2020). Toll-like receptor 4 is a therapeutic target for prevention and treatment of liver failure. J. Hepatology 73 (1), 102–112. doi:10.1016/j.jhep.2020.01.011
Epelman, S., Lavine, K. J., Beaudin, A. E., Sojka, D. K., Carrero, J. A., Calderon, B., et al. (2014). Embryonic and adult-derived resident cardiac macrophages are maintained through distinct mechanisms at steady state and during inflammation. Immunity 40 (1), 91–104. doi:10.1016/j.immuni.2013.11.019
Eslam, M., Newsome, P. N., Sarin, S. K., Anstee, Q. M., Targher, G., Romero-Gomez, M., et al. (2020). A new definition for metabolic dysfunction-associated fatty liver disease: An international expert consensus statement. J. Hepatology 73 (1), 202–209. doi:10.1016/j.jhep.2020.03.039
Fagan, K. J., Rogers, G. B., Melino, M., Arthur, D. M., Costello, M.-E., Morrison, M., et al. (2015). Ascites bacterial burden and immune cell profile Are associated with poor clinical outcomes in the absence of overt infection. PLOS ONE 10 (3), e0120642. doi:10.1371/journal.pone.0120642
Fernández, J., Acevedo, J., Castro, M., Garcia, O., Rodríguez de Lope, C., Roca, D., et al. (2012). Prevalence and risk factors of infections by multiresistant bacteria in cirrhosis: A prospective study. Hepatology 55 (5), 1551–1561. doi:10.1002/hep.25532
Fernández, J., Acevedo, J., Wiest, R., Gustot, T., Amoros, A., Deulofeu, C., et al. (2018). Bacterial and fungal infections in acute-on-chronic liver failure: Prevalence, characteristics and impact on prognosis. Gut 67 (10), 1870–1880. doi:10.1136/gutjnl-2017-314240
Fernández, J., Prado, V., Trebicka, J., and Amoros, A. (2019). Multidrug-resistant bacterial infections in patients with decompensated cirrhosis and with acute-on-chronic liver failure in Europe. J. Hepatology 70 (3), 398–411.
Flint, E., Triantafyllou, E., and Bernsmeier, C. (2022). TAM receptors in the pathophysiology of liver disease. Livers 2 (1), 15–29. doi:10.3390/livers2010002
Frances, R. (2004). Bacterial DNA activates cell mediated immune response and nitric oxide overproduction in peritoneal macrophages from patients with cirrhosis and ascites. Gut 53 (6), 860–864. doi:10.1136/gut.2003.027425
Gabrilovich, D. I., Bronte, V., Chen, S.-H., Colombo, M. P., Ochoa, A., Ostrand-Rosenberg, S., et al. (2007). The terminology issue for myeloid-derived suppressor cells. Cancer Res. 67 (1), 425. doi:10.1158/0008-5472.can-06-3037
Gabrilovich, D. I. (2017). Myeloid-derived suppressor cells. Cancer Immunol. Res. 5 (1), 3–8. doi:10.1158/2326-6066.cir-16-0297
Gadd, V. L., Patel, P. J., Jose, S., Horsfall, L., Powell, E. E., and Irvine, K. M. (2016). Altered peripheral blood monocyte phenotype and function in chronic liver disease: Implications for hepatic recruitment and systemic inflammation. PLOS ONE 11 (6), e0157771. doi:10.1371/journal.pone.0157771
Gao, B., Ahmad, M. F., Nagy, L. E., and Tsukamoto, H. (2019). Inflammatory pathways in alcoholic steatohepatitis. J. Hepatology 70 (2), 249–259. doi:10.1016/j.jhep.2018.10.023
Garg, V., Garg, H., Khan, A., Trehanpati, N., Kumar, A., Sharma, B. C., et al. (2012). Granulocyte colony-stimulating factor mobilizes CD34+ cells and improves survival of patients with acute-on-chronic liver failure. Gastroenterology 142 (3), 505–512. doi:10.1053/j.gastro.2011.11.027
Gong, X., Li, T., Wan, R., and Sha, L. (2021). Cordycepin attenuates high-fat diet-induced non-alcoholic fatty liver disease via down-regulation of lipid metabolism and inflammatory responses. Int. Immunopharmacol. 91, 107173. doi:10.1016/j.intimp.2020.107173
Gordon, S., and Taylor, P. R. (2005). Monocyte and macrophage heterogeneity. Nat. Rev. Immunol. 5 (12), 953–964. doi:10.1038/nri1733
Grønbaek, H., Sandahl, T. D., Vilstrup, H., and Møller, S. (2012). Soluble CD163, a marker of Kupffer cell activation, is related to portal hypertension in patients with liver cirrhosis. Alimentary Pharmacol. Ther. 36 (2), 173–180. doi:10.1111/j.1365-2036.2012.05134.x
Grønbæk, H., Gantzel, R. H., and Laursen, T. L. (2020). Macrophage markers and innate immunity in cirrhosis. J. Hepatology 73 (6), 1586–1588. doi:10.1016/j.jhep.2020.07.033
Grønbæk, H., Rødgaard-Hansen, S., Aagaard, N. K., Arroyo, V., Moestrup, S. K., Garcia, E., et al. (2016). Macrophage activation markers predict mortality in patients with liver cirrhosis without or with acute-on-chronic liver failure (ACLF). J. Hepatology 64 (4), 813–822. doi:10.1016/j.jhep.2015.11.021
Guilliams, M., Bonnardel, J., Haest, B., Vanderborght, B., Wagner, C., Remmerie, A., et al. (2022). Spatial proteogenomics reveals distinct and evolutionarily conserved hepatic macrophage niches. Cell 185 (2), 379–396. e38. doi:10.1016/j.cell.2021.12.018
Guilliams, M., Mildner, A., and Yona, S. (2018). Developmental and functional heterogeneity of monocytes. Immunity 49 (4), 595–613. doi:10.1016/j.immuni.2018.10.005
Guillot, A., and Tacke, F. (2019). Liver macrophages: Old dogmas and new insights. Hepatol. Commun. 3 (6), 730–743. doi:10.1002/hep4.1356
Gustot, T., Fernandez, J., Garcia, E., Morando, F., Caraceni, P., Alessandria, C., et al. (2015). Clinical Course of acute-on-chronic liver failure syndrome and effects on prognosis. Hepatology 62 (1), 243–252. doi:10.1002/hep.27849
Gustot, T., Stadlbauer, V., Laleman, W., Alessandria, C., and Thursz, M. (2021). Transition to decompensation and acute-on-chronic liver failure: Role of predisposing factors and precipitating events. J. Hepatology 75 (Suppl. 1), S36–S48. doi:10.1016/j.jhep.2020.12.005
Haderer, M., Neubert, P., Rinner, E., Scholtis, A., Broncy, L., Gschwendtner, H., et al. (2022). Novel pathomechanism for spontaneous bacterial peritonitis: Disruption of cell junctions by cellular and bacterial proteases. Gut 71 (3), 580–592. doi:10.1136/gutjnl-2020-321663
Hadjivasilis, A., Tzanis, A., Ioakim, K. J., Poupoutsi, I., Agouridis, A. P., and Kouis, P. (2021). The diagnostic accuracy of ascitic calprotectin for the early diagnosis of spontaneous bacterial peritonitis: Systematic review and meta-analysis. Eur. J. Gastroenterol. Hepatol. 33 (3), 312–318. doi:10.1097/meg.0000000000001813
Haldar, D., Kern, B., Hodson, J., Armstrong, M. J., Adam, R., Berlakovich, G., et al. (2019). Outcomes of liver transplantation for non-alcoholic steatohepatitis: A European liver transplant registry study. J. Hepatology 71 (2), 313–322. doi:10.1016/j.jhep.2019.04.011
Hambleton, S., Salem, S., Bustamante, J., Bigley, V., Boisson-Dupuis, S., Azevedo, J., et al. (2011). IRF8Mutations and human dendritic-cell immunodeficiency. N. Engl. J. Med. 365, 127–138. doi:10.1056/nejmoa1100066
Hartmann, P., Chen, P., Wang, H. J., Wang, L., McCole, D. F., Brandl, K., et al. (2013). Deficiency of intestinal mucin-2 ameliorates experimental alcoholic liver disease in mice. Hepatology 58 (1), 108–119. doi:10.1002/hep.26321
Hartmann, P., Seebauer, C. T., and Schnabl, B. (2015). Alcoholic liver disease: The gut microbiome and liver cross talk. Alcohol Clin. Exp. Res. 39 (5), 763–775. doi:10.1111/acer.12704
Hassner, A., Kletter, Y., Jedvab, M., Aronson, M., and Shibolet, S. (1979). Impaired monocyte function in liver cirrhosis. Lancet 313 (8111), 329–330. doi:10.1016/s0140-6736(79)90745-1
Heel, K. A., and Hall, J. C. (2005). Peritoneal defences and peritoneum-associated lymphoid tissue. Br. J. Surg. 83 (8), 1031–1036. doi:10.1002/bjs.1800830804
Henderson, N. C., Mackinnon, A. C., Farnworth, S. L., Poirier, F., Russo, F. P., Iredale, J. P., et al. (2006). Galectin-3 regulates myofibroblast activation and hepatic fibrosis. Proc. Natl. Acad. Sci. U.S.A. 103 (13), 5060–5065. doi:10.1073/pnas.0511167103
Heymann, F., Hammerich, L., Storch, D., Bartneck, M., Huss, S., Rüsseler, V., et al. (2012). Hepatic macrophage migration and differentiation critical for liver fibrosis is mediated by the chemokine receptor C-C motif chemokine receptor 8 in mice. Hepatology 55 (3), 898–909. doi:10.1002/hep.24764
Heymann, F., Peusquens, J., Ludwig‐Portugall, I., Kohlhepp, M., Ergen, C., Niemietz, P., et al. (2015). Liver inflammation abrogates immunological tolerance induced by Kupffer cells. Hepatology 62 (1), 279–291. doi:10.1002/hep.27793
Heymann, F., and Tacke, F. (2016). Immunology in the liver - From homeostasis to disease. Nat. Rev. Gastroenterol. Hepatol. 13 (2), 88–110. doi:10.1038/nrgastro.2015.200
Höchst, B., Schildberg, F. A., and Sauerborn, P. (2013). Activated human hepatic stellate cells induce myeloid derived suppressor cells from peripheral blood monocytes in a CD44-dependent fashion. J. Hepatology 59 (3), 528–535. doi:10.1016/j.jhep.2013.04.033
Holdstock, G., Leslie, B., Hill, S., Tanner, A., and Wright, R. (1982). Monocyte function in cirrhosis. J. Clin. Pathology 35 (9), 972–979. doi:10.1136/jcp.35.9.972
Holland-Fischer, P., Gronbaek, H., Sandahl, T. D., Moestrup, S. K., Riggio, O., Ridola, L., et al. (2011). Kupffer cells are activated in cirrhotic portal hypertension and not normalised by TIPS. Gut 60 (10), 1389–1393. doi:10.1136/gut.2010.234542
Hytiroglou, P., and Theise, N. D. (2018). Regression of human cirrhosis: An update, 18 years after the pioneering article by wanless et al. Virchows Arch. 473 (1), 15–22. doi:10.1007/s00428-018-2340-2
Idalsoaga, F., Kulkarni, A. V., Mousa, O. Y., Arrese, M., and Arab, J. P. (2020). Non-alcoholic fatty liver disease and alcohol-related liver disease: Two intertwined entities. Front. Med. 7, 448. doi:10.3389/fmed.2020.00448
Ilyas, G., Cingolani, F., Zhao, E., Tanaka, K., and Czaja, M. J. (2019). Decreased macrophage autophagy promotes liver injury and inflammation from alcohol. Alcohol Clin. Exp. Re 43 (7), 1403–1413. doi:10.1111/acer.14041
Irvine, K. M., Banh, X., Gadd, V. L., Wojcik, K. K., Ariffin, J. K., Jose, S., et al. (2016). CRIg-expressing peritoneal macrophages are associated with disease severity in patients with cirrhosis and ascites. JCI Insight 1 (8), e86914. doi:10.1172/jci.insight.86914
Irvine, K. M., Ratnasekera, I., Powell, E. E., and Hume, D. A. (2019). Causes and consequences of innate immune dysfunction in cirrhosis. Front. Immunol. 10, 293. doi:10.3389/fimmu.2019.00293
Itoh, M., Kato, H., Suganami, T., Konuma, K., Marumoto, Y., Terai, S., et al. (2013). Hepatic crown-like structure: A unique histological feature in non-alcoholic steatohepatitis in mice and humans. PLoS ONE 8 (12), e82163. doi:10.1371/journal.pone.0082163
Jakubzick, C., Gautier, E. L., Gibbings, S. L., Sojka, D. K., Schlitzer, A., Johnson, T. E., et al. (2013). Minimal differentiation of classical monocytes as they survey steady-state tissues and transport antigen to lymph nodes. Immunity 39 (3), 599–610. doi:10.1016/j.immuni.2013.08.007
Jakubzick, C. V., Randolph, G. J., and Henson, P. M. (2017). Monocyte differentiation and antigen-presenting functions. Nat. Rev. Immunol. 17 (6), 349–362. doi:10.1038/nri.2017.28
Jalan, R., Fernandez, J., Wiest, R., Schnabl, B., Moreau, R., Angeli, P., et al. (2014). Bacterial infections in cirrhosis: A position statement based on the EASL special conference 2013. J. Hepatology 60 (6), 1310–1324. doi:10.1016/j.jhep.2014.01.024
Jenne, C. N., and Kubes, P. (2013). Immune surveillance by the liver. Nat. Immunol. 14 (10), 996–1006. doi:10.1038/ni.2691
Ju, C., and Tacke, F. (2016). Hepatic macrophages in homeostasis and liver diseases: From pathogenesis to novel therapeutic strategies. Cell Mol. Immunol. 13 (3), 316–327. doi:10.1038/cmi.2015.104
Kamada, N., Hisamatsu, T., and Okamoto, S. (2008). Unique CD14+ intestinal macrophages contribute to the pathogenesis of Crohn disease via IL-23/IFN-γ axis. J. Clin. Investigation 118, 2269–2280. doi:10.1172/JCI34610
Kapellos, T. S., Bonaguro, L., Gemünd, I., Reusch, N., Saglam, A., Hinkley, E. R., et al. (2019). Human monocyte subsets and phenotypes in major chronic inflammatory diseases. Front. Immunol. 10, 2035. doi:10.3389/fimmu.2019.02035
Kaplan, D. E., Serper, M., John, B. V., Tessiatore, K. M., Lerer, R., Mehta, R., et al. (2021). Effects of metformin exposure on survival in a large national cohort of patients with diabetes and cirrhosis. Clin. Gastroenterology Hepatology 19 (10), 2148–2160. e14. doi:10.1016/j.cgh.2020.08.026
Karlmark, K. R., Weiskirchen, R., Zimmermann, H. W., Gassler, N., Ginhoux, F., Weber, C., et al. (2009). Hepatic recruitment of the inflammatory Gr1+monocyte subset upon liver injury promotes hepatic fibrosis. Hepatology 50 (1), 261–274. doi:10.1002/hep.22950
Karlsen, T. H., Sheron, N., Zelber-Sagi, S., Carrieri, P., Dusheiko, G., Bugianesi, E., et al. (2022). The EASL-lancet liver commission: Protecting the next generation of Europeans against liver disease complications and premature mortality. Lancet 399 (10319), 61–116. doi:10.1016/s0140-6736(21)01701-3
Kawamura, S., Onai, N., Miya, F., Sato, T., Tsunoda, T., Kurabayashi, K., et al. (2017). Identification of a human clonogenic progenitor with strict monocyte differentiation potential: A counterpart of mouse cMoPs. Immunity 46 (5), 835–848. doi:10.1016/j.immuni.2017.04.019
Kazankov, K., Barrera, F., Møller, H. J., Rosso, C., Bugianesi, E., David, E., et al. (2016). The macrophage activation marker sCD163 is associated with morphological disease stages in patients with non-alcoholic fatty liver disease. Liver Int. 36 (10), 1549–1557. doi:10.1111/liv.13150
Kazankov, K., Jørgensen, S. M. D., Thomsen, K. L., Møller, H. J., Vilstrup, H., George, J., et al. (2019). The role of macrophages in nonalcoholic fatty liver disease and nonalcoholic steatohepatitis. Nat. Rev. Gastroenterol. Hepatol. 16 (3), 145–159. doi:10.1038/s41575-018-0082-x
Kedarisetty, C. K., Anand, L., Bhardwaj, A., Bhadoria, A. S., Kumar, G., Vyas, A. K., et al. (2015). Combination of granulocyte colony-stimulating factor and erythropoietin improves outcomes of patients with decompensated cirrhosis. Gastroenterology 148 (7), 1362–1370. e7. doi:10.1053/j.gastro.2015.02.054
Kisseleva, T., and Brenner, D. (2021). Molecular and cellular mechanisms of liver fibrosis and its regression. Nat. Rev. Gastroenterol. Hepatol. 18 (3), 151–166. doi:10.1038/s41575-020-00372-7
Kolios, G. (2006). Role of Kupffer cells in the pathogenesis of liver disease. Wjg 12 (46), 7413. doi:10.3748/wjg.v12.i46.7413
Korf, H., Boesch, M., Meelberghs, L., and van der Merwe, S. (2019). Macrophages as key players during adipose tissue-liver crosstalk in nonalcoholic fatty liver disease. Semin. Liver Dis. 39 (03), 291–300. doi:10.1055/s-0039-1687851
Korf, H., du Plessis, J., van Pelt, J., De Groote, S., Cassiman, D., Verbeke, L., et al. (2019). Inhibition of glutamine synthetase in monocytes from patients with acute-on-chronic liver failure resuscitates their antibacterial and inflammatory capacity. Gut 68 (10), 1872–1883. doi:10.1136/gutjnl-2018-316888
Koyama, Y., and Brenner, D. A. (2017). Liver inflammation and fibrosis. J. Clin. Investigation 127 (1), 55–64. doi:10.1172/jci88881
Krenkel, O., Puengel, T., Govaere, O., Abdallah, A. T., Mossanen, J. C., Kohlhepp, M., et al. (2018). Therapeutic inhibition of inflammatory monocyte recruitment reduces steatohepatitis and liver fibrosis. Hepatology 67 (4), 1270–1283. doi:10.1002/hep.29544
Krenkel, O., and Tacke, F. (2017). Liver macrophages in tissue homeostasis and disease. Nat. Rev. Immunol. 17 (5), 306–321. doi:10.1038/nri.2017.11
Kubes, P., and Jenne, C. (2018). Immune responses in the liver. Annu. Rev. Immunol. 36 (1), 247–277. doi:10.1146/annurev-immunol-051116-052415
Laleman, W., Claria, J., Van der Merwe, S., Moreau, R., and Trebicka, J. (2018). Systemic inflammation and acute-on-chronic liver failure: Too much, not enough. Can. J. Gastroenterology Hepatology 2018. undefined-undefined. doi:10.1155/2018/1027152
Lebossé, F., Gudd, C., Tunc, E., Singanayagam, A., Nathwani, R., Triantafyllou, E., et al. (2019). CD8+ T cells from patients with cirrhosis display a phenotype that may contribute to cirrhosis-associated immune dysfunction. EBioMedicine 49, 258–268. doi:10.1016/j.ebiom.2019.10.011
Lee, J., Breton, G., Oliveira, T. Y. K., Zhou, Y. J., Aljoufi, A., Puhr, S., et al. (2015). Restricted dendritic cell and monocyte progenitors in human cord blood and bone marrow. J. Exp. Med. 212 (3), 385–399. doi:10.1084/jem.20141442
Lee, Y. A., Wallace, M. C., and Friedman, S. L. (2015). Pathobiology of liver fibrosis: A translational success story. Gut 64 (5), 830–841. doi:10.1136/gutjnl-2014-306842
Lefere, S., and Tacke, F. (2019). Macrophages in obesity and non-alcoholic fatty liver disease: Crosstalk with metabolism. JHEP Rep. 1 (1), 30–43. doi:10.1016/j.jhepr.2019.02.004
Lesińska, M., Hartleb, M., Gutkowski, K., and Nowakowska-Duława, E. (2014). Procalcitonin and macrophage inflammatory protein-1 beta (MIP-1β) in serum and peritoneal fluid of patients with decompensated cirrhosis and spontaneous bacterial peritonitis. Adv. Med. Sci. 59 (1), 52–56. doi:10.1016/j.advms.2013.07.006
Li, H., Zheng, H.-W., Chen, H., Xing, Z.-Z., You, H., Cong, M., et al. (2012). Hepatitis B virus particles preferably induce Kupffer cells to produce TGF-β1 over pro-inflammatory cytokines. Dig. Liver Dis. 44 (4), 328–333. doi:10.1016/j.dld.2011.11.005
Li, L., Wei, W., Li, Z., Chen, H., Li, Y., Jiang, W., et al. (2018). The spleen promotes the secretion of CCL2 and supports an M1 dominant phenotype in hepatic macrophages during liver fibrosis. Cell Physiol. Biochem. 51 (2), 557–574. doi:10.1159/000495276
Li, P., He, K., Li, J., Liu, Z., and Gong, J. (2017). The role of Kupffer cells in hepatic diseases. Mol. Immunol. 85, 222–229. doi:10.1016/j.molimm.2017.02.018
Liang, S., Zhong, Z., Kim, S. Y., Uchiyama, R., Roh, Y. S., Matsushita, H., et al. (2019). Murine macrophage autophagy protects against alcohol-induced liver injury by degrading interferon regulatory factor 1 (IRF1) and removing damaged mitochondria. J. Biol. Chem. 294 (33), 12359–12369. doi:10.1074/jbc.ra119.007409
Liaskou, E., Zimmermann, H. W., Li, K. K., Oo, Y. H., Suresh, S., Stamataki, Z., et al. (2013). Monocyte subsets in human liver disease show distinct phenotypic and functional characteristics. Hepatology 57 (1), 385–398. doi:10.1002/hep.26016
Lin, C.-Y., Tsai, I.-F., Ho, Y.-P., Huang, C.-T., Lin, Y.-C., Lin, C.-J., et al. (2007). Endotoxemia contributes to the immune paralysis in patients with cirrhosis. J. Hepatology 46 (5), 816–826. doi:10.1016/j.jhep.2006.12.018
Liu, J., Yu, Q., Wu, W., Huang, X., Broering, R., Werner, M., et al. (2018). TLR2 stimulation strengthens intrahepatic myeloid-derived cell-mediated T cell tolerance through inducing kupffer cell expansion and IL-10 production. J. I. 200 (7), 2341–2351. doi:10.4049/jimmunol.1700540
MacParland, S. A., Liu, J. C., Ma, X.-Z., Innes, B. T., Bartczak, A. M., Gage, B. K., et al. (2018). Single cell RNA sequencing of human liver reveals distinct intrahepatic macrophage populations. Nat. Commun. 9 (1), 4383. doi:10.1038/s41467-018-06318-7
Mandorfer, M., Kozbial, K., Schwabl, P., Chromy, D., Semmler, G., Stättermayer, A. F., et al. (2020). Changes in hepatic venous pressure gradient predict hepatic decompensation in patients who achieved sustained virologic response to interferon‐free therapy. Hepatology 71 (3), 1023–1036. doi:10.1002/hep.30885
Mandrekar, P., and Szabo, G. (2009). Signalling pathways in alcohol-induced liver inflammation. J. Hepatology 50 (6), 1258–1266. doi:10.1016/j.jhep.2009.03.007
Marcellin, P., Gane, E., Buti, M., Afdhal, N., Sievert, W., Jacobson, I. M., et al. (2013). Regression of cirrhosis during treatment with tenofovir disoproxil fumarate for chronic Hepatitis B: A 5-year open-label follow-up study. Lancet 381 (9865), 468–475. doi:10.1016/s0140-6736(12)61425-1
Marcellin, P., and Kutala, B. K. (2018). Liver diseases: A major, neglected global public health problem requiring urgent actions and large-scale screening. Liver Int. 38, 2–6. doi:10.1111/liv.13682
Mazagova, M., Wang, L., Anfora, A. T., Wissmueller, M., Lesley, S. A., Miyamoto, Y., et al. (2015). Commensal microbiota is hepatoprotective and prevents liver fibrosis in mice. FASEB J. 29 (3), 1043–1055. doi:10.1096/fj.14-259515
Miyata, T., and Nagy, L. E. (2020). Programmed cell death in alcohol-associated liver disease. Clin. Mol. Hepatol. 26 (4), 618–625. doi:10.3350/cmh.2020.0142
Mokdad, A. A., Lopez, A. D., Shahraz, S., Lozano, R., Mokdad, A. H., Stanaway, J., et al. (2014). Liver cirrhosis mortality in 187 countries between 1980 and 2010: A systematic analysis. BMC Med. 12 (1), 145. doi:10.1186/s12916-014-0145-y
Moreau, R., Jalan, R., Gines, P., Pavesi, M., Angeli, P., Cordoba, J., et al. (2013). Acute-on-Chronic liver failure is a distinct syndrome that develops in patients with acute decompensation of cirrhosis. Gastroenterology 144 (7), 1426–1437. e9. doi:10.1053/j.gastro.2013.02.042
Mosmann, T. R., Cherwinski, H., Bond, M. W., Giedlin, M. A., and Coffman, R. L. (1986). Two types of murine helper T cell clone. I. Definition according to profiles of lymphokine activities and secreted proteins. J. Immunol. 136 (7), 2348–2357.
Mossanen, J. C., Krenkel, O., Ergen, C., Govaere, O., Liepelt, A., Puengel, T., et al. (2016). Chemokine (C-C motif) receptor 2-positive monocytes aggravate the early phase of acetaminophen-induced acute liver injury. Hepatology 64 (5), 1667–1682. doi:10.1002/hep.28682
Mouries, J., Brescia, P., Silvestri, A., Spadoni, I., Sorribas, M., Wiest, R., et al. (2019). Microbiota-driven gut vascular barrier disruption is a prerequisite for non-alcoholic steatohepatitis development. J. Hepatology 71 (6), 1216–1228. doi:10.1016/j.jhep.2019.08.005
Mridha, A. R., Wree, A., Robertson, A. A. B., Yeh, M. M., Johnson, C. D., Van Rooyen, D. M., et al. (2017). NLRP3 inflammasome blockade reduces liver inflammation and fibrosis in experimental NASH in mice. J. Hepatology 66 (5), 1037–1046. doi:10.1016/j.jhep.2017.01.022
Murray, P. J., Allen, J. E., Biswas, S. K., Fisher, E. A., Gilroy, D. W., Goerdt, S., et al. (2014). Macrophage activation and polarization: Nomenclature and experimental guidelines. Immunity 41 (1), 14–20. doi:10.1016/j.immuni.2014.06.008
Nathan, C. F., Murray, H. W., Wiebe, M. E., and Rubin, B. Y. (1983). Identification of interferon-gamma as the lymphokine that activates human macrophage oxidative metabolism and antimicrobial activity. J. Exp. Med. 158 (3), 670–689. doi:10.1084/jem.158.3.670
Netea, M. G., Joosten, L. A., Latz, E., Mills, K. H., Natoli, G., Stunnenberg, H. G., et al. (2016). Trained immunity: A program of innate immune memory in health and disease. Science 352 (6284), aaf1098. doi:10.1126/science.aaf1098
Newsome, P. N., Fox, R., King, A. L., Barton, D., Than, N.-N., Moore, J., et al. (2018). Granulocyte colony-stimulating factor and autologous cd133-positive stem-cell therapy in liver cirrhosis (REALISTIC): An open-label, randomised, controlled phase 2 trial. Lancet Gastroenterology Hepatology 3 (1), 25–36. doi:10.1016/s2468-1253(17)30326-6
Nguyen-Lefebvre, A. T., Ajith, A., Portik-Dobos, V., Horuzsko, D. D., Arbab, A. S., Dzutsev, A., et al. (2018). The innate immune receptor TREM-1 promotes liver injury and fibrosis. J. Clin. Investigation 128 (11), 4870–4883. doi:10.1172/jci98156
Nielsen, M. C., Hvidbjerg Gantzel, R., Clària, J., Trebicka, J., Møller, H. J., and Grønbæk, H. (2020). Macrophage activation markers, CD163 and CD206, in acute-on-chronic liver failure. Cells 9 (5), 1175. doi:10.3390/cells9051175
Nieto, J. C., Perea, L., Soriano, G., Zamora, C., Cantó, E., Medina, A., et al. (2018). Ascitic fluid regulates the local innate immune response of patients with cirrhosis. J. Leukoc. Biol. 104 (4), 833–841. doi:10.1002/jlb.3a0218-072r
Nieto, J. C., Sanchez, E., Romero, C., Roman, E., Poca, M., Guarner, C., et al. (2015). Impaired innate immune response of leukocytes from ascitic fluid of patients with spontaneous bacterial peritonitis. J. Leukoc. Biol. 98 (5), 819–825. doi:10.1189/jlb.3ab0315-106r
O'Brien, A. J., Fullerton, J. N., Massey, K. A., Auld, G., Sewell, G., James, S., et al. (2014). Immunosuppression in acutely decompensated cirrhosis is mediated by prostaglandin E2. Nat. Med. 20 (5), 518–523. doi:10.1038/nm.3516
Olsson, A., Venkatasubramanian, M., Chaudhri, V. K., Aronow, B. J., Salomonis, N., Singh, H., et al. (2016). Single-cell analysis of mixed-lineage states leading to a binary cell fate choice. Nature 537 (7622), 698–702. doi:10.1038/nature19348
Onorato, L., Monari, C., Capuano, S., and Grimaldi, P. (2022). Prevalence and therapeutic management of infections by multi-drug-resistant organisms (MDROs) in patients with liver cirrhosis: A narrative review. Antibiot. (Basel) 11 (2). doi:10.3390/antibiotics11020232
Ostrand-Rosenberg, S., Beury, D. W., Parker, K. H., and Horn, L. A. (2020). Survival of the fittest: How myeloid-derived suppressor cells survive in the inhospitable tumor microenvironment. Cancer Immunol. Immunother. 69 (2), 215–221. doi:10.1007/s00262-019-02388-8
Pal, S., Dey, D., Chakraborty, B. C., Nandi, M., Khatun, M., Banerjee, S., et al. (2022). Diverse facets of MDSC in different phases of chronic HBV infection: Impact on HBV-specific T-cell response and homing. Hepatology. 10.1002/hep.32331
Passlick, B., Flieger, D., and Ziegler-Heitbrock, H. (1989). Identification and characterization of a novel monocyte subpopulation in human peripheral blood. Blood 74 (7), 2527–2534. doi:10.1182/blood.v74.7.2527.bloodjournal7472527
Patel, A. A., Zhang, Y., Fullerton, J. N., Boelen, L., Rongvaux, A., Maini, A. A., et al. (2017). The fate and lifespan of human monocyte subsets in steady state and systemic inflammation. J. Exp. Med. 214 (7), 1913–1923. doi:10.1084/jem.20170355
Perugorria, M. J., Esparza-Baquer, A., Oakley, F., Labiano, I., Korosec, A., Jais, A., et al. (2019). Non-parenchymal TREM-2 protects the liver from immune-mediated hepatocellular damage. Gut 68 (3), 533–546. doi:10.1136/gutjnl-2017-314107
Piano, S., Singh, V., Caraceni, P., Maiwall, R., Alessandria, C., Fernandez, J., et al. (2019). Epidemiology and effects of bacterial infections in patients with cirrhosis worldwide. Gastroenterology 156 (5), 1368–e10. doi:10.1053/j.gastro.2018.12.005
Planas, R., Montoliu, S., Ballesté, B., Rivera, M., Miquel, M., Masnou, H., et al. (2006). Natural history of patients hospitalized for management of cirrhotic ascites. Clin. Gastroenterology Hepatology 4 (11), 1385–1394. doi:10.1016/j.cgh.2006.08.007
Ponziani, F. R., Zocco, M. A., Cerrito, L., Gasbarrini, A., and Pompili, M. (2018). Bacterial translocation in patients with liver cirrhosis: Physiology, clinical consequences, and practical implications. Expert Rev. Gastroenterology Hepatology 12 (7), 641–656. doi:10.1080/17474124.2018.1481747
Prickett, T. C. R., Mckenzie, J. L., and Hart, D. N. J. (1988). Characterization of interstitial dendritic cells in human liver. Transplantation 46 (5), 754–761. doi:10.1097/00007890-198811000-00024
Quintin, J., Saeed, S., Martens, J. H. A., Giamarellos-Bourboulis, E. J., Ifrim, D. C., Logie, C., et al. (2012). Candida albicans infection affords protection against reinfection via functional reprogramming of monocytes. Cell Host Microbe 12 (2), 223–232. doi:10.1016/j.chom.2012.06.006
Rainer, F., Horvath, A., Sandahl, T. D., Leber, B., Schmerboeck, B., Blesl, A., et al. (2018). Soluble CD163 and soluble mannose receptor predict survival and decompensation in patients with liver cirrhosis, and correlate with gut permeability and bacterial translocation. Aliment. Pharmacol. Ther. 47 (5), 657–664. doi:10.1111/apt.14474
Ramachandran, P., Pellicoro, A., Vernon, M. A., Boulter, L., Aucott, R. L., Ali, A., et al. (2012). Differential Ly-6C expression identifies the recruited macrophage phenotype, which orchestrates the regression of murine liver fibrosis. Proc. Natl. Acad. Sci. U. S. A. 109 (46), E3186–E3195. doi:10.1073/pnas.1119964109
Ramachandran, P., Dobie, R., Wilson-Kanamori, J. R., Dora, E. F., Henderson, B. E. P., Luu, N. T., et al. (2019). Resolving the fibrotic niche of human liver cirrhosis at single-cell level. Nature 575 (7783), 512–518. doi:10.1038/s41586-019-1631-3
Ramachandran, P., Matchett, K. P., Dobie, R., Wilson-Kanamori, J. R., and Henderson, N. C. (2020). Single-cell technologies in hepatology: New insights into liver biology and disease pathogenesis. Nat. Rev. Gastroenterol. Hepatol. 17 (8), 457–472. doi:10.1038/s41575-020-0304-x
Rao, R. (2009). Endotoxemia and gut barrier dysfunction in alcoholic liver disease. Hepatology 50 (2), 638–644. doi:10.1002/hep.23009
Remmerie, A., Martens, L., and Scott, C. L. (2020). Macrophage subsets in obesity, aligning the liver and adipose tissue. Front. Endocrinol. 11, 259. doi:10.3389/fendo.2020.00259
Remmerie, A., Martens, L., Thoné, T., Castoldi, A., Seurinck, R., Pavie, B., et al. (2020). Osteopontin expression identifies a subset of recruited macrophages distinct from kupffer cells in the fatty liver. Immunity 53 (3), 641–657. e14. doi:10.1016/j.immuni.2020.08.004
Resheq, Y. J., Li, K.-K., Ward, S. T., Wilhelm, A., Garg, A., Curbishley, S. M., et al. (2015). Contact-dependent depletion of hydrogen peroxide by catalase is a novel mechanism of myeloid-derived suppressor cell induction operating in human hepatic stellate cells. J. I. 194 (6), 2578–2586. doi:10.4049/jimmunol.1401046
Rode, A., Nicoll, A., Møller, H. J., Lim, L., Angus, P. W., Kronborg, I., et al. (2013). Hepatic macrophage activation predicts clinical decompensation in chronic liver disease. Gut 62 (8), 1231–1232. doi:10.1136/gutjnl-2012-304135
Rothlin, C. V., Ghosh, S., Zuniga, E. I., Oldstone, M. B. A., and Lemke, G. (2007). TAM receptors are pleiotropic inhibitors of the innate immune response. Cell 131 (6), 1124–1136. doi:10.1016/j.cell.2007.10.034
Ruiz-Alcaraz, A. J., Carmona-Martínez, V., Tristán-Manzano, M., Machado-Linde, F., Sánchez-Ferrer, M. L., García-Peñarrubia, P., et al. (2018). Characterization of human peritoneal monocyte/macrophage subsets in homeostasis: Phenotype, GATA6, phagocytic/oxidative activities and cytokines expression. Sci. Rep. 8 (1), 12794. doi:10.1038/s41598-018-30787-x
Ruiz-Alcaraz, A. J., Tapia-Abellán, A., Fernández-Fernández, M. D., Tristán-Manzano, M., Hernández-Caselles, T., Sánchez-Velasco, E., et al. (2016). A novel CD14high CD16high subset of peritoneal macrophages from cirrhotic patients is associated to an increased response to LPS. Mol. Immunol. 72, 28–36. doi:10.1016/j.molimm.2016.02.012
Ruiz‐Alcaraz, A. J., Martínez-Banaclocha, H., Marín-Sánchez, P., and Carmona-Martínez, V. (2020). Isolation of functional mature peritoneal macrophages from healthy humans. Immunol. Cell Biol. 98 (2), 114–126. doi:10.1111/imcb.12305
Runyon, B. A., and Aasld, (2013). Introduction to the revised American Association for the Study of Liver Diseases Practice Guideline management of adult patients with ascites due to cirrhosis 2012. Hepatology 57 (4), 1651–1653. doi:10.1002/hep.26359
Saha, B., Tornai, D., Kodys, K., Adejumo, A., Lowe, P., McClain, C., et al. (2019). Biomarkers of macrophage activation and immune danger signals predict clinical outcomes in alcoholic hepatitis. Hepatology 70 (4), 1134–1149. doi:10.1002/hep.30617
Sanchez-Tapias, J., Thomas, H. C., and Sherlock, S. (1977). Lymphocyte populations in liver biopsy specimens from patients with chronic liver disease. Gut 18 (6), 472–475. doi:10.1136/gut.18.6.472
Sandler, N. G., Koh, C., Roque, A., Eccleston, J. L., Siegel, R. B., Demino, M., et al. (2011). Host response to translocated microbial products predicts outcomes of patients with HBV or HCV infection. Gastroenterology 141 (4), 12201230 e1–12303. doi:10.1053/j.gastro.2011.06.063
Sarin, S. K., Pande, A., and Schnabl, B. (2019). Microbiome as a therapeutic target in alcohol-related liver disease. J. Hepatology 70 (2), 260–272. doi:10.1016/j.jhep.2018.10.019
Sasaki, R., Devhare, P. B., Steele, R., Ray, R., and Ray, R. B. (2017). Hepatitis C virus-induced CCL5 secretion from macrophages activates hepatic stellate cells. Hepatology 66 (3), 746–757. doi:10.1002/hep.29170
Satoh, T., Nakagawa, K., Sugihara, F., Kuwahara, R., Ashihara, M., Yamane, F., et al. (2017). Identification of an atypical monocyte and committed progenitor involved in fibrosis. Nature 541 (7635), 96–101. doi:10.1038/nature20611
Scott, N. A., and Mann, E. R. (2020). Regulation of mononuclear phagocyte function by the microbiota at mucosal sites. Immunology 159 (1), 26–38. doi:10.1111/imm.13155
Seki, E., De Minicis, S., Österreicher, C. H., Kluwe, J., Osawa, Y., Brenner, D. A., et al. (2007). TLR4 enhances TGF-β signaling and hepatic fibrosis. Nat. Med. 13 (11), 1324–1332. doi:10.1038/nm1663
Selimoglu-Buet, D., Wagner-Ballon, O., Saada, V., Bardet, V., Itzykson, R., Bencheikh, L., et al. (2015). Characteristic repartition of monocyte subsets as a diagnostic signature of chronic myelomonocytic leukemia. Blood 125 (23), 3618–3626. doi:10.1182/blood-2015-01-620781
Serbina, N. V., and Pamer, E. G. (2006). Monocyte emigration from bone marrow during bacterial infection requires signals mediated by chemokine receptor CCR2. Nat. Immunol. 7 (3), 311–317. doi:10.1038/ni1309
Shah, N., Montes de Oca, M., Jover-Cobos, M., Tanamoto, K.-i., Muroi, M., Sugiyama, K.-i., et al. (2013). Role of toll-like receptor 4 in mediating multiorgan dysfunction in mice with acetaminophen induced acute liver failure. Liver Transpl. 19 (7), 751–761. doi:10.1002/lt.23655
Sherlock, S. (1977). Immunological disturbance in diseases of liver and thyroid. Proc. R. Soc. Med. 70, 851–855. doi:10.1177/003591577707001207
Shetty, S., Lalor, P. F., and Adams, D. H. (2018). Liver sinusoidal endothelial cells - Gatekeepers of hepatic immunity. Nat. Rev. Gastroenterol. Hepatol. 15 (9), 555–567. doi:10.1038/s41575-018-0020-y
Sierro, F., Evrard, M., Rizzetto, S., Melino, M., Mitchell, A. J., Florido, M., et al. (2017). A liver capsular network of monocyte-derived macrophages restricts hepatic dissemination of intraperitoneal bacteria by neutrophil recruitment. Immunity 47 (2), 374–388. doi:10.1016/j.immuni.2017.07.018
Singanayagam, A., and Triantafyllou, E. (2021). Macrophages in chronic liver failure: Diversity, plasticity and therapeutic targeting. Front. Immunol. 12, 661182. doi:10.3389/fimmu.2021.661182
Smith, P. D., Smythies, L. E., Shen, R., Greenwell-Wild, T., Gliozzi, M., and Wahl, S. M. (2011). Intestinal macrophages and response to microbial encroachment. Mucosal Immunol. 4 (1), 31–42. doi:10.1038/mi.2010.66
Smythies, L. E., Sellers, M., Clements, R. H., Mosteller-Barnum, M., Meng, G., Benjamin, W. H., et al. (2005). Human intestinal macrophages display profound inflammatory anergy despite avid phagocytic and bacteriocidal activity. J. Clin. Invest. 115 (1), 66–75. doi:10.1172/jci200519229
Song, Q., Hawkins, G. A., Wudel, L., Chou, P. C., Forbes, E., Pullikuth, A. K., et al. (2019). Dissecting intratumoral myeloid cell plasticity by single cell RNA‐seq. Cancer Med. 8 (6), 3072–3085. doi:10.1002/cam4.2113
Staufer, K., Dengler, M., Huber, H., Marculescu, R., Stauber, R., Lackner, C., et al. (2017). The non-invasive serum biomarker soluble Axl accurately detects advanced liver fibrosis and cirrhosis. Cell Death Dis. 8 (10), e3135. doi:10.1038/cddis.2017.554
Stengel, S., Quickert, S., Lutz, P., Ibidapo-Obe, O., Steube, A., Köse-Vogel, N., et al. (2020). Peritoneal level of CD206 associates with mortality and an inflammatory macrophage phenotype in patients with decompensated cirrhosis and spontaneous bacterial peritonitis. Gastroenterology 158 (6), 1745–1761. doi:10.1053/j.gastro.2020.01.029
Strauss, O., Dunbar, P. R., Bartlett, A., and Phillips, A. (2015). The immunophenotype of antigen presenting cells of the mononuclear phagocyte system in normal human liver - a systematic review. J. Hepatology 62 (2), 458–468. doi:10.1016/j.jhep.2014.10.006
Su, T., Yang, Y., Lai, S., Jeong, J., Jung, Y., McConnell, M., et al. (2021). Single-cell transcriptomics reveals zone-specific alterations of liver sinusoidal endothelial cells in cirrhosis. Cell. Mol. Gastroenterology Hepatology 11 (4), 1139–1161. doi:10.1016/j.jcmgh.2020.12.007
Sugimoto, C., Hasegawa, A., Saito, Y., Fukuyo, Y., Chiu, K. B., Cai, Y., et al. (2015). Differentiation kinetics of blood monocytes and dendritic cells in macaques: Insights to understanding human myeloid cell development. J. I. 195 (4), 1774–1781. doi:10.4049/jimmunol.1500522
Sunderkötter, C., Nikolic, T., Dillon, M. J., van Rooijen, N., Stehling, M., Drevets, D. A., et al. (2004). Subpopulations of mouse blood monocytes differ in maturation stage and inflammatory response. J. Immunol. 172 (7), 4410–4417. doi:10.4049/jimmunol.172.7.4410
Sutti, S., Bruzzì, S., Heymann, F., Liepelt, A., Krenkel, O., Toscani, A., et al. (2019). CX3CR1 mediates the development of monocyte-derived dendritic cells during hepatic inflammation. Cells 8 (9), 1099. doi:10.3390/cells8091099
Suzuki, Y., Shirai, M., Asada, K., Yasui, H., Karayama, M., Hozumi, H., et al. (2018). Macrophage mannose receptor, CD206, predict prognosis in patients with pulmonary tuberculosis. Sci. Rep. 8 (1), 13129. doi:10.1038/s41598-018-31565-5
Tacke, F., Ginhoux, F., Jakubzick, C., van Rooijen, N., Merad, M., and Randolph, G. J. (2006). Immature monocytes acquire antigens from other cells in the bone marrow and present them to T cells after maturing in the periphery. J. Exp. Med. 203 (3), 583–597. doi:10.1084/jem.20052119
Tak, T., Drylewicz, J., Conemans, L., de Boer, R. J., Koenderman, L., Borghans, J. A. M., et al. (2017). Circulatory and maturation kinetics of human monocyte subsets in vivo. Blood 130 (12), 1474–1477. doi:10.1182/blood-2017-03-771261
Tak, T., van Groenendael, R., Pickkers, P., and Koenderman, L. (2017). Monocyte subsets are differentially lost from the circulation during acute inflammation induced by human experimental endotoxemia. J. Innate Immun. 9 (5), 464–474. doi:10.1159/000475665
Tamoutounour, S., Henri, S., Lelouard, H., de Bovis, B., de Haar, C., van der Woude, C. J., et al. (2012). CD64 distinguishes macrophages from dendritic cells in the gut and reveals the Th1-inducing role of mesenteric lymph node macrophages during colitis. Eur. J. Immunol. 42 (12), 3150–3166. doi:10.1002/eji.201242847
Tan-Garcia, A., Wai, L.-E., Zheng, D., Ceccarello, E., Jo, J., Banu, N., et al. (2017). Intrahepatic CD206+ macrophages contribute to inflammation in advanced viral-related liver disease. J. Hepatology 67 (3), 490–500. doi:10.1016/j.jhep.2017.04.023
Tandon, P., and Garcia-Tsao, G. (2008). Bacterial infections, sepsis, and multiorgan failure in cirrhosis. Semin. Liver Dis. 28 (1), 26–42. doi:10.1055/s-2008-1040319
Tapia-Abellán, A., Martínez-Esparza, M., Ruiz-Alcaraz, A. J., and Hernández-Caselles, T. (2012). The peritoneal macrophage inflammatory profile in cirrhosis depends on the alcoholic or hepatitis C viral etiology and is related to ERK phosphorylation. BMC Immunol. 13 (1), 42. doi:10.1186/1471-2172-13-42
Thaler, B., Hohensinner, P. J., Krychtiuk, K. A., Matzneller, P., Koller, L., Brekalo, M., et al. (2016). Differential in vivo activation of monocyte subsets during low-grade inflammation through experimental endotoxemia in humans. Sci. Rep. 6, 30162. doi:10.1038/srep30162
Tian, Y., Kuo, C.-f., Akbari, O., and Ou, J.-h. J. (2016). Maternal-Derived Hepatitis B Virus e Antigen Alters Macrophage Function in Offspring to Drive Viral Persistence after Vertical Transmission. Immunity 44 (5), 1204–1214. doi:10.1016/j.immuni.2016.04.008
Tomita, K., Freeman, B. L., Bronk, S. F., LeBrasseur, N. K., White, T. A., Hirsova, P., et al. (2016). CXCL10-Mediates macrophage, but not other innate immune cells-associated inflammation in murine nonalcoholic steatohepatitis. Sci. Rep. 6, 28786. doi:10.1038/srep28786
Trebicka, J., Fernandez, J., Papp, M., Caraceni, P., Laleman, W., Gambino, C., et al. (2021). PREDICT identifies precipitating events associated with the clinical course of acutely decompensated cirrhosis. J. Hepatol. 74 (5), 1097–1108. doi:10.1016/j.jhep.2020.11.019
Triantafyllou, E., Pop, O. T., Possamai, L. A., Wilhelm, A., Liaskou, E., Singanayagam, A., et al. (2018). MerTK expressing hepatic macrophages promote the resolution of inflammation in acute liver failure. Gut 67 (2), 333–347. doi:10.1136/gutjnl-2016-313615
Triantos, C., Kalafateli, M., Assimakopoulos, S. F., Karaivazoglou, K., Mantaka, A., Aggeletopoulou, I., et al. (2022). Endotoxin translocation and gut barrier dysfunction are related to variceal bleeding in patients with liver cirrhosis. Front. Med. 9, 836306. doi:10.3389/fmed.2022.836306
Tsochatzis, E. A., Bosch, J., and Burroughs, A. K. (2014). Liver cirrhosis. Lancet 383 (9930), 1749–1761. doi:10.1016/s0140-6736(14)60121-5
Tsuchida, T., and Friedman, S. L. (2017). Mechanisms of hepatic stellate cell activation. Nat. Rev. Gastroenterol. Hepatol. 14 (7), 397–411. doi:10.1038/nrgastro.2017.38
Tutusaus, A., de Gregorio, E., Cucarull, B., Cristóbal, H., Aresté, C., Graupera, I., et al. (2020). A functional role of GAS6/TAM in nonalcoholic steatohepatitis progression implicates AXL as therapeutic target. Cell. Mol. Gastroenterology Hepatology 9 (3), 349–368. doi:10.1016/j.jcmgh.2019.10.010
Van der Merwe, S., Chokshi, S., Bernsmeier, C., and Albillos, A. (2021). The multifactorial mechanisms of bacterial infection in decompensated cirrhosis. J. Hepatology 75, S82–S100. doi:10.1016/j.jhep.2020.11.029
Varol, C., Landsman, L., Fogg, D. K., Greenshtein, L., Gildor, B., Margalit, R., et al. (2007). Monocytes give rise to mucosal, but not splenic, conventional dendritic cells. J. Exp. Med. 204 (1), 171–180. doi:10.1084/jem.20061011
Veglia, F., Sanseviero, E., and Gabrilovich, D. I. (2021). Myeloid-derived suppressor cells in the era of increasing myeloid cell diversity. Nat. Rev. Immunol. 21 (8), 485–498. doi:10.1038/s41577-020-00490-y
Verma, N., Kaur, A., Sharma, R., Bhalla, A., Sharma, N., De, A., et al. (2018). Outcomes after multiple courses of granulocyte colony‐stimulating factor and growth hormone in decompensated cirrhosis: A randomized trial. Hepatology 68 (4), 1559–1573. doi:10.1002/hep.29763
Villani, A. C., Satija, R., Reynolds, G., Sarkizova, S., Shekhar, K., Fletcher, J., et al. (2017). Single-cell RNA-seq reveals new types of human blood dendritic cells, monocytes, and progenitors. Science 356 (6335), eaah4573. doi:10.1126/science.aah4573
Waidmann, O., Brunner, F., Herrmann, E., Zeuzem, S., Piiper, A., and Kronenberger, B. (2013). Macrophage activation is a prognostic parameter for variceal bleeding and overall survival in patients with liver cirrhosis. J. Hepatology 58 (5), 956–961. doi:10.1016/j.jhep.2013.01.005
Wang, J., Yu, Y., Yang, Y., Wu, S. S., Zhu, H. H., Liu, Y. N., et al. (2015). Expression of serum sCD163 in patients with liver diseases and inflammatory disorders. Int. J. Clin. Exp. Pathol. 8 (7), 8419–8425.
Wang, K. Y., Huang, R. Y., Tong, X. Z., Zhang, K. N., Liu, Y. W., Zeng, F., et al. (2019). Molecular and clinical characterization of TMEM71 expression at the transcriptional level in glioma. CNS Neurosci. Ther. 25 (9), 965–975. doi:10.1111/cns.13137
Wang, S., Chen, Z., Hu, C., Qian, F., Cheng, Y., Wu, M., et al. (2013). Hepatitis B virus surface antigen selectively inhibits TLR2 ligand-induced IL-12 production in monocytes/macrophages by interfering with JNK activation. J. I. 190 (10), 5142–5151. doi:10.4049/jimmunol.1201625
Wanless, I. R., Nakashima, E., and Sherman, M. (2000). Regression of human cirrhosis. Archives Pathology Laboratory Med. 124 (11), 1599–1607. doi:10.5858/2000-124-1599-rohc
Wanless, I. R. (2020). The role of vascular injury and congestion in the pathogenesis of cirrhosis: The congestive escalator and the parenchymal extinction sequence. Curr. Hepatol. Rep. 19 (1), 40–53. doi:10.1007/s11901-020-00508-y
Wasmuth, H. E., Kunz, D., Yagmur, E., Timmer-Stranghöner, A., Vidacek, D., Siewert, E., et al. (2005). Patients with acute on chronic liver failure display 'sepsis-like' immune paralysis. J. Hepatology 42 (2), 195–201. doi:10.1016/j.jhep.2004.10.019
Wen, Y., Lambrecht, J., Ju, C., and Tacke, F. (2021). Hepatic macrophages in liver homeostasis and diseases-diversity, plasticity and therapeutic opportunities. Cell Mol. Immunol. 18 (1), 45–56. doi:10.1038/s41423-020-00558-8
Weston, C. J., Zimmermann, H. W., and Adams, D. H. (2019). The role of myeloid-derived cells in the progression of liver disease. Front. Immunol. 10, 893. doi:10.3389/fimmu.2019.00893
Wiest, R., Albillos, A., Trauner, M., Bajaj, J. S., and Jalan, R. (2017). Targeting the gut-liver axis in liver disease. J. Hepatology 67 (5), 1084–1103. doi:10.1016/j.jhep.2017.05.007
Wiest, R., Lawson, M., and Geuking, M. (2014). Pathological bacterial translocation in liver cirrhosis. J. Hepatology 60 (1), 197–209. doi:10.1016/j.jhep.2013.07.044
Williams, J. A., and Ding, W.-X. (2020). Role of autophagy in alcohol and drug-induced liver injury. Food Chem. Toxicol. 136, 111075. doi:10.1016/j.fct.2019.111075
Wu, J., Meng, Z., Jiang, M., Zhang, E., Trippler, M., Broering, R., et al. (2010). Toll-like receptor-induced innate immune responses in non-parenchymal liver cells are cell type-specific. Immunology 129 (3), 363–374. doi:10.1111/j.1365-2567.2009.03179.x
Wu, R., Nakatsu, G., Zhang, X., and Yu, J. (2016). Pathophysiological mechanisms and therapeutic potentials of macrophages in non-alcoholic steatohepatitis. Expert Opin. Ther. Targets 20 (5), 615–626. doi:10.1517/14728222.2016.1125883
Xing, T., Li, L., Cao, H., and Huang, J. (2007). Altered immune function of monocytes in different stages of patients with acute on chronic liver failure. Clin. Exp. Immunol. 147 (1), 184–188. doi:10.1111/j.1365-2249.2006.03259.x
Xiong, X., Kuang, H., Ansari, S., Liu, T., Gong, J., Wang, S., et al. (2019). Landscape of intercellular crosstalk in healthy and NASH liver revealed by single-cell secretome gene analysis. Mol. Cell 75 (3), 644–660. doi:10.1016/j.molcel.2019.07.028
Yan, A. W., E. Fouts, D., Brandl, J., Stärkel, P., Torralba, M., Schott, E., et al. (2011). Enteric dysbiosis associated with a mouse model of alcoholic liver disease. Hepatology 53 (1), 96–105. doi:10.1002/hep.24018
Yan, K., and Garcia-Tsao, G. (2016). Novel prevention strategies for bacterial infections in cirrhosis. Expert Opin. Pharmacother. 17 (5), 689–701. doi:10.1517/14656566.2016.1145663
Yona, S., Kim, K.-W., Wolf, Y., Mildner, A., Varol, D., Breker, M., et al. (2013). Fate mapping reveals origins and dynamics of monocytes and tissue macrophages under homeostasis. Immunity 38 (1), 79–91. doi:10.1016/j.immuni.2012.12.001
Yongxiang, W., Zongfang, L. i., Guowei, L. i., Zongzheng, J. i., Xi, C., and Tao, W. u. (2002). Effects of splenomegaly and splenic macrophage activity in hypersplenism due to cirrhosis. Am. J. Med. 113 (5), 428–431. doi:10.1016/s0002-9343(02)01210-x
Younossi, Z. M., Golabi, P., and Henry, L. (2019). A comprehensive review of patient-reported outcomes in patients with chronic liver diseases. J. Clin. Gastroenterology 53 (5), 331–341. doi:10.1097/mcg.0000000000001179
Yrlid, U., Jenkins, C. D., and MacPherson, G. G. (2006). Relationships between distinct blood monocyte subsets and migrating intestinal lymph dendritic cells in vivo under steady-state conditions. J. Immunol. 176 (7), 4155–4162. doi:10.4049/jimmunol.176.7.4155
Zapater, P., Francés, R., González-Navajas, J. M., de la Hoz, M. A., Moreu, R., Pascual, S., et al. (2008). Serum and ascitic fluid bacterial dna: A new independent prognostic factor in noninfected patients with cirrhosis. Hepatology 48 (6), 1924–1931. doi:10.1002/hep.22564
Zapater, P., González-Navajas, J. M., Such, J., and Francés, R. (2015). Immunomodulating effects of antibiotics used in the prophylaxis of bacterial infections in advanced cirrhosis. Wjg 21 (41), 11493–11501. doi:10.3748/wjg.v21.i41.11493
Zhang, X., Han, J., Man, K., Li, X., Du, J., Chu, E. S. H., et al. (2016). CXC chemokine receptor 3 promotes steatohepatitis in mice through mediating inflammatory cytokines, macrophages and autophagy. J. Hepatology 64 (1), 160–170. doi:10.1016/j.jhep.2015.09.005
Ziegler-Heitbrock, L., and Hofer, T. P. (2013). Toward a refined definition of monocyte subsets. Front. Immunol. 4, 23. doi:10.3389/fimmu.2013.00023
Zigmond, E., Samia-Grinberg, S., Pasmanik-Chor, M., Brazowski, E., Shibolet, O., Halpern, Z., et al. (2014). Infiltrating monocyte-derived macrophages and resident kupffer cells display different ontogeny and functions in acute liver injury. J. I. 193 (1), 344–353. doi:10.4049/jimmunol.1400574
Zimmermann, H. W., Bruns, T., Weston, C. J., Curbishley, S. M., Liaskou, E., Li, K.-K., et al. (2016). Bidirectional transendothelial migration of monocytes across hepatic sinusoidal endothelium shapes monocyte differentiation and regulates the balance between immunity and tolerance in liver. Hepatology 63 (1), 233–246. doi:10.1002/hep.28285
Zimmermann, H. W., Seidler, S., Nattermann, J., Gassler, N., Hellerbrand, C., Zernecke, A., et al. (2010). Functional contribution of elevated circulating and hepatic non-classical CD14+CD16+ monocytes to inflammation and human liver fibrosis. PLoS One 5 (6), e11049. doi:10.1371/journal.pone.0011049
Keywords: cirrhosis, monocytes, macrophages, liver injury, inflammation, ACLF, immuneparesis, immunotherapy
Citation: Geng A, Flint E and Bernsmeier C (2022) Plasticity of monocytes and macrophages in cirrhosis of the liver. Front. Netw. Physiol. 2:937739. doi: 10.3389/fnetp.2022.937739
Received: 06 May 2022; Accepted: 27 June 2022;
Published: 15 July 2022.
Edited by:
Alireza Mani, University College London, United KingdomReviewed by:
Khalil Hajiasgharzadeh, Tabriz University of Medical Sciences, IranAntonios Chatzigeorgiou, National and Kapodistrian University of Athens, Greece
Copyright © 2022 Geng, Flint and Bernsmeier. This is an open-access article distributed under the terms of the Creative Commons Attribution License (CC BY). The use, distribution or reproduction in other forums is permitted, provided the original author(s) and the copyright owner(s) are credited and that the original publication in this journal is cited, in accordance with accepted academic practice. No use, distribution or reproduction is permitted which does not comply with these terms.
*Correspondence: Christine Bernsmeier, Yy5iZXJuc21laWVyQHVuaWJhcy5jaA==