- 1Laboratory of Biochemistry, Neurosciences, Natural Resources and Environment, Hassan First University, Faculty of Sciences and Technology, Settat, Morocco
- 2Higher School of Technology of El Kelâa des Sraghna, Cadi Ayyad University, El Kelâa des Sraghna, Morocco
Marine algae are a rich and underexplored source of haloperoxidases, enzymes with wide ranging applications in biocatalysis, pharmaceuticals, and environmental bioremediation due to their ability to catalyze the halogenation of organic compounds. This review focuses on the recent advancements in the purification of haloperoxidases from marine algae, highlighting both traditional and innovative methods. We discussed the limited exploration of green algal haloperoxidases, and the potential for discovering novel enzymes with unique properties. The review examines the advantages and disadvantages of chromatographic techniques, such as ion-exchange, size exclusion, and affinity chromatography, and explores emerging alternatives, including aqueous two-phase systems (ATPS) and microfluidic systems, for improving enzyme yield, purity, and stability. The use of ATPS to address challenges posed by alginate-rich brown algae is emphasized, along with optimization strategies for scaling up purification processes. The growing importance of sustainable and green chemistry approaches to minimize environmental impact while achieving high purification efficiency is also discussed. By analyzing current purification techniques, identifying knowledge gaps, and suggesting future research directions, this review aims to provide meaningful insights into the purification and industrial applications of haloperoxidases, thereby stimulating further exploration and innovation in this field.
1 Introduction
Marine ecosystems host an exceptional variety of life forms, ranging from microorganisms to complex organisms, thriving in diverse habitats (Chandna et al., 2020; Griffin, 2020). This biodiversity is fundamental to sustaining ecosystem stability and functionality (Chandna et al., 2020). Among these organisms, algae are particularly significant as a rich source of bioactive compounds with substantial industrial importance (Tripathi et al., 2024). Of the numerous enzymes derived from these organisms, haloperoxidases have garnered increasing attention due to their unique ability to catalyze the halogenation of organic compounds (Höfler et al., 2019). This catalytic process, which involves the incorporation of halogens such as chlorine, bromine, or iodine into various substrates, plays a critical role in the synthesis of biologically active molecules and environmentally friendly chemical transformations (Franssen, 1994; Höfler et al., 2019). Consequently, haloperoxidases hold immense promise for applications in biocatalysis, pharmaceuticals, and environmental remediation due to their unique catalytic properties, such as regio- and stereoselectivity, as well as their ability to catalyze halogenation reactions (Ayala and Eduardo, 2015; Rebollar-Pérez et al., 2019). In biocatalysis, they can be used to synthesize complex organic compounds; in pharmaceuticals, they facilitate the production of halogenated drugs with enhanced efficacy and bioavailability; and in environmental remediation, they play a role in the degradation of persistent organic pollutants. Despite this potential, haloperoxidases from marine algae remain underutilized, partly due to challenges associated with their extraction and purification (Wever et al., 2018). Conventional purification methods often fall short in achieving the required yield, purity, and stability for industrial applications, highlighting the need for innovative approaches. Furthermore, large-scale utilization of these enzymes has been limited by difficulties in maintaining bioactivity and scalability. Therefore, addressing these knowledge and technical gaps is essential to fully exploit the potential of haloperoxidases and enable their efficient integration into industrial applications. The objective of this review is to provide an in-depth exploration of algal haloperoxidases, focusing on their diversity, purification methodologies, and the associated challenges. Special attention is given to underrepresented groups, such as green algae, and to innovative techniques that could overcome existing limitations. By identifying key knowledge gaps and proposing strategies for optimizing purification, this review aims to facilitate the broader application of haloperoxidases in industrial and biotechnological fields.
2 Overview of haloperoxidases in marine algae
Haloperoxidases are enzymes that catalyze the incorporation of halogen atoms (such as chlorine, bromine, or iodine) into organic compounds using hydrogen peroxide or other peroxides as oxidants (Franssen, 1994; Gérard et al., 2023). This halogenation process is highly selective, facilitating the synthesis of halogenated compounds with significant biological and industrial value (Sharma et al., 2024). Haloperoxidases are typically classified based on the halogen they incorporate and their active site composition, with the main types being chloroperoxidases (CPOs), bromoperoxidases (BPOs), and iodoperoxidases (IPOs), with only BPOs and IPOs are found in marine algae (Butler and Baldwin, 1997; Pacios and Galvez, 2010; Fournier et al., 2014). In marine algae, these enzymes play a critical role in the biosynthesis of halogenated natural products that serve ecological functions such as chemical defense and signaling (Butler and Carter-Franklin, 2004; Baumgartner and Shaun, 2021).
Vanadium-dependent haloperoxidases, including bromoperoxidases (V-BPOs) and iodoperoxidases (V-IPOs), catalyze the oxidation of bromide (Br−) or iodide (I−) using hydrogen peroxide (H2O2), producing halogenated organic compounds and water (Figure 1). These enzymes activate hydrogen peroxide to form a highly reactive intermediate, facilitating regio- and stereoselective halogenation of organic substrates (Aboelnga, 2022; Chen et al., 2022; Zhang and Liu, 2023). V-BPOs, primarily found in red and brown algae, exhibit broad pH stability (4–10) and high thermal resistance, making them valuable for industrial biocatalysis. In contrast, V-IPOs, mainly from brown algae, function within a narrower pH range (5–7) and play key roles in marine iodine metabolism (Tarakhovskaya et al., 2015; Punitha et al., 2018; Ishikawa et al., 2022). In marine ecosystems, halogenation mediated by these enzymes contributes to the production of bioactive compounds involved in chemical defense, microbial inhibition, and ecological interactions. Both V-BPOs and V-IPOs have significant applications in green chemistry, particularly in selective halogenation processes (La Barre et al., 2010; Cabrita et al., 2010).

Figure 1. Catalytic reaction of vanadium haloperoxidase. where X− represents a halide ion (Br−, or I−).
Marine algae, especially brown, red, and green algae, are notable sources of haloperoxidases. Brown algae, such as Laminaria spp., Macrocystis pyrifera, and Ascophyllum nodosum, are rich in vanadium-dependent haloperoxidases (V-HPOs), which are involved in bromination and iodination processes (Küpper and Kroneck, 2014; Tarakhovskaya et al., 2015; Punitha et al., 2018). These enzymes are responsible for the production of brominated and iodinated compounds for defense, tissue repair, and adhesion (La Barre et al., 2010). Red algae, such as Laurencia spp. and Gracilaria spp., produce haloperoxidases that generate halogenated phenols with antimicrobial and antifouling properties, contributing to their ecological success (Weinberger et al., 2007; Thapa et al., 2020; Ishikawa et al., 2022). Green algae, such as Ulva spp. and Codium spp., also harbor haloperoxidases (Rehder, 2014), although they are less extensively studied, and these enzymes catalyze the halogenation of fatty acids and terpenoids as part of the algae’s chemical defense strategies (Carter-Franklin et al., 2003; Weinberger et al., 2007; Thapa et al., 2020).
3 Industrial applications of haloperoxidases
Haloperoxidases have garnered significant attention for their versatility in various industrial applications due to their unique ability to catalyze selective halogenation reactions (Höfler et al., 2019; Sharma et al., 2024). These enzymes are proving invaluable in biocatalysis, pharmaceuticals, environmental remediation, and emerging innovative applications (Littlechild, 1999; García-Zamora et al., 2018; Michail and Isupov, 2014; Höfler et al., 2019; Rebollar-Pérez et al., 2019).
In biocatalysis, haloperoxidases catalyze the selective halogenation of organic compounds using H2O2 as an oxidant, a process visually depicted in their catalytic mechanisms. These enzymes are broadly classified into heme-dependent and vanadium-dependent haloperoxidases. In heme-dependent haloperoxidases, the catalytic cycle begins with the activation of the heme iron to form a highly reactive intermediate known as Compound I, which facilitates the transfer of halide ions (X−) to the substrate, leading to halogenation (Figure 2). In vanadium-dependent haloperoxidases, the resting state involves a vanadate cofactor bound to the active site, which is oxidized by H2O2 to produce a peroxo vanadate species that mediates halogenation (Figure 3). These mechanisms are highly regio- and stereoselective, enabling precise chemical transformations that are challenging to achieve using conventional chemical halogenation. Additionally, the environmentally benign nature of this process, with water as the only byproduct, underscores its sustainability. Recent research by Sharma et al. (2024) highlighted the efficacy of V-HPOs as biocatalysts for selective halogenation, specifically facilitating the synthesis of 1,2,4-oxadiazole from substituted benzamidine hydrochlorides. This compound is a cornerstone in drug discovery, forming part of numerous experimental, investigational, and approved drugs (Boström et al., 2012; Dhameliya et al., 2022). Oxadiazoles, acting as bioisosteres for esters, hydroxamic acids, carboxamides, and carbamates, enhance the metabolic stability of drugs such as ataluren, naldemedine, amenamevir, ozanimod, azilsartan medoxomil, and opicapone, thereby improving their therapeutic efficacy and prolonging their action (Salahuddin et al., 2017; Baykov et al., 2023). Furthermore, studies have shown that V-HPOs exhibit broad substrate specificity and are capable of catalyzing complex reactions, including epoxidation, hydroxylation, and halogenation, which are often challenging to achieve with conventional chemical methods (Bhandari et al., 2023; Gérard et al., 2023). Their diversity and ability to construct greener synthesis routes match green chemistry concepts, decreasing waste and the requirement for hazardous chemicals. V-HPOs, have significant applications in green chemistry due to their ability to catalyze halogenation and oxidation reactions with high selectivity and stability. These enzymes facilitate the biosynthesis of halogenated organic compounds, widely used in pharmaceuticals and as synthetic intermediates in chemical industries. Their enzymatic approach offers an environmentally friendly alternative to using toxic and corrosive reagents like molecular bromine (Wischang and Hartung, 2011). For instance, V-HPOs from A. nodosum have been successfully applied to synthesize brominated pyrroles (Wischang et al., 2011a) and terpenes (Carter-Franklin et al., 2003), as well as optically pure sulfoxides, which are critical intermediates in asymmetric synthesis and pharmaceutical formulations (Ten Brink et al., 2001). Moreover, the immobilization of V-HPOs on supports such as magnetic beads has enabled efficient recycling of these biocatalysts (Wischang et al., 2011b). These applications demonstrate the potential of V-HPOs to drive more sustainable and selective chemical processes.
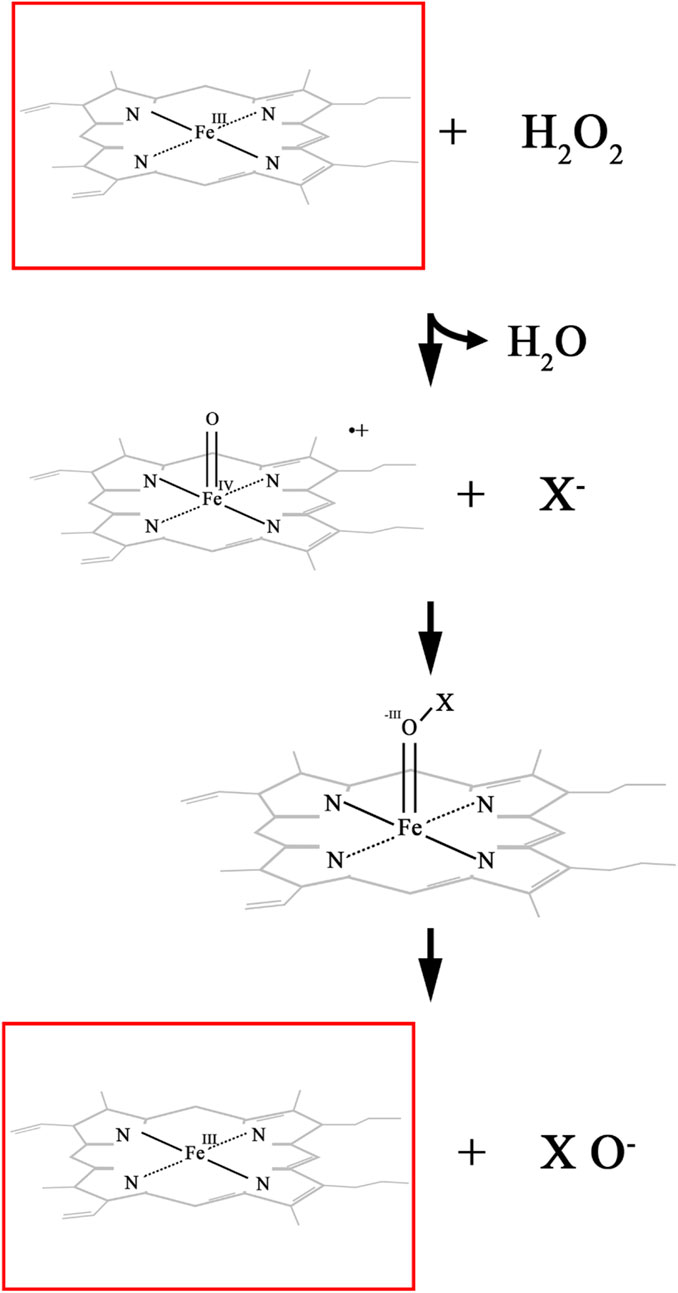
Figure 2. Catalytic mechanism of heme dependent haloperoxidase. where X− represents a halide ion (Br−, or I−).
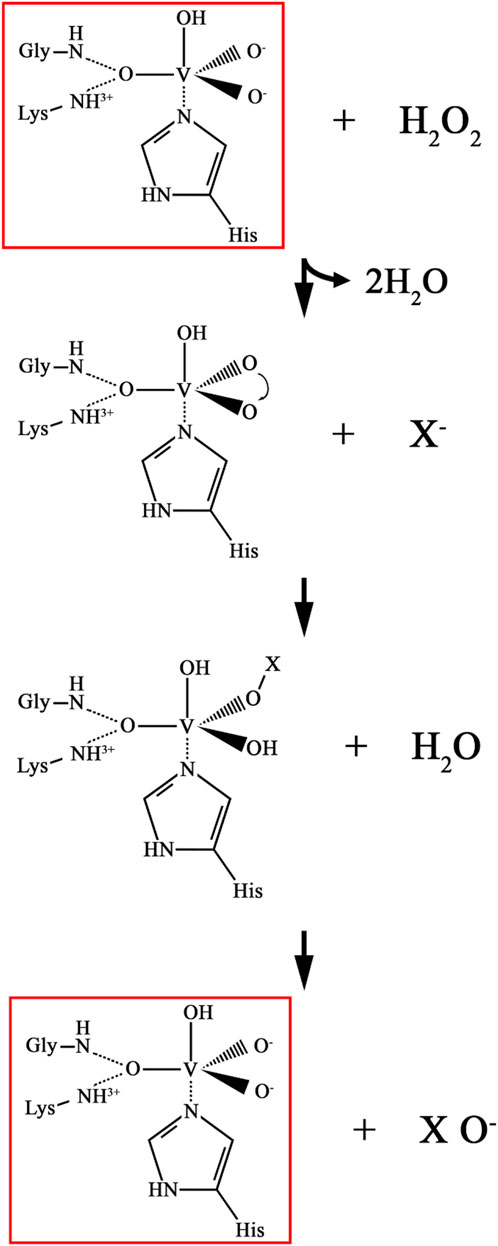
Figure 3. Catalytic mechanism of vanadium dependent haloperoxidase. where X− represents a halide ion (Br−, or I−).
Despite extensive studies on V-HPOs, their application remains poorly exploited, particularly from marine algae. Notably, most research has focused exclusively on bromoperoxidases, neglecting the abundant iodoperoxidases in brown algae such as Laminaria digitata, A. nodosum, and Pelvetia canaliculate (Almeida et al., 2000; Colin et al., 2005; Verhaeghe et al., 2008). These iodoperoxidases hold significant potential for novel applications, yet their properties and industrial relevance remain largely unexplored. Expanding the study of V-HPOs to include these enzymes could open new avenues for biotechnological advancements and further harness the untapped potential of marine algae-derived biocatalysts.
4 Purification techniques of haloperoxidases from algae
Haloperoxidases were purified from algae using various purification techniques. Supplementary Table S1 provides an overview of haloperoxidases from various algal sources, focusing on their purification techniques, prosthetic groups, and optimal activity conditions. Algae are categorized into red, brown, and green types, with red and brown algae being the most extensively studied. Each algal group presents unique characteristics in terms of haloperoxidase stability and adaptability, reflecting their potential for diverse applications. In addition, the purification of haloperoxidases varies across different algal types, with distinct techniques influencing yield and purification fold (Table 1).
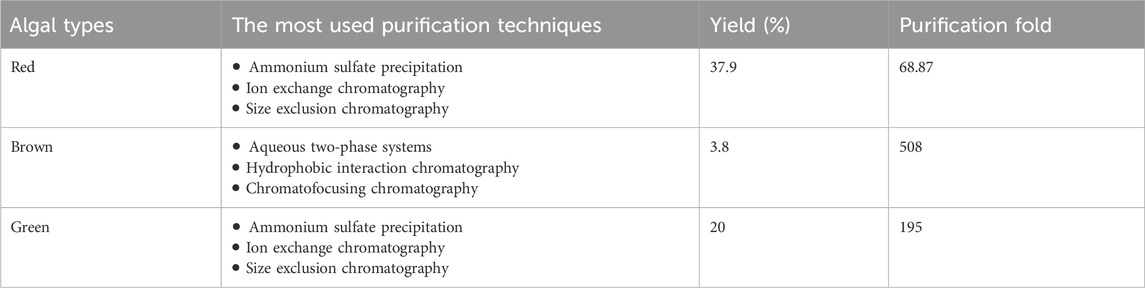
Table 1. The most used purification techniques, yield, and purification fold of haloperoxidases from different algal types.
Red algae exhibit the highest yield (37.9%) but a moderate purification fold (68.87), primarily using ammonium sulfate precipitation, ion-exchange chromatography, and size-exclusion chromatography (Itoh et al., 1985; Sheffield et al., 1992; Kongkiattikajorn and Ruenwongsa, 2006). These algae are prominently represented by species including Corallina spp and Gracilaria spp (Itoh et al., 1985; Sheffield et al., 1992; Rush et al., 1995; Coupe et al., 2007). Vanadium-dependent bromoperoxidases (V-BPOs) exhibit impressive stability, functioning effectively across a wide pH range of 4–10. They are also highly temperature-resistant, with Gracilaria fisheri enzymes remaining stable up to 50°C (Kongkiattikajorn and Pongdam, 2006) and Corallina officinalis enzymes enduring temperatures as high as 80°C (Rush et al., 1995). This makes red algae a valuable source for enzymes suitable for industrial processes requiring robustness under varied conditions (Littlechild and Isupov, 2014).
Brown algae dominate the literature due to their rich diversity, including families like Laminariaceae, Fucaceae, and Phyllariaceae (Wever et al., 2018). Despite having the lowest yield (3.8%), brown algae achieve the highest purification fold (508), primarily using aqueous two-phase systems, hydrophobic interaction chromatography, and chromatofocusing chromatogramphy. These algae primarily produce vanadium-dependent iodoperoxidases (V-IPOs) and bromoperoxidases (V-BPOs), characterized by their moderate pH and temperature stability (pH 5–7, 20°C–60°C) (De Boer et al., 1986; Wever et al., 1985; Almeida et al., 1998; Hara and Sakurai, 1998; Almeida et al., 2000; Almeida et al., 2001; Colin et al., 2003; Verhaeghe et al., 2008; Tarakhovskaya et al., 2015). Brown algae are abundant in alginates, sulfated fucans, and polyphenolic compounds, which pose significant challenges during the extraction and purification of haloperoxidases (Wever et al., 2018). To address these complications, several studies have employed aqueous salt/polymer two-phase systems as an effective purification method (Almeida et al., 1998; Hara and Sakurai, 1998; Almeida et al., 2001; Colin et al., 2003; Verhaeghe et al., 2008; Tarakhovskaya et al., 2015). Additionally, hydrophobic interaction chromatography is frequently utilized, reflecting the complexity of haloperoxidases derived from brown algae (Verhaeghe et al., 2008; Almeida et al., 2001). While less commonly used, chromatofocusing chromatography has proven effective in a study involving Saccorhiza polyschides (Almeida et al., 1998).
Green algae remain underrepresented, with only Cladophora glomerata. This species contains a heme-based haloperoxidase, which is distinct from the vanadium prosthetic groups seen in red and brown algae. Green algae demonstrate an intermediate yield (20%) and purification fold (195), using ammonium sulfate precipitation, ion-exchange chromatography, and size-exclusion chromatography. The enzyme’s activity is optimal at an unusually low pH of 3.1, making it unique among algal haloperoxidases. This enzyme has been successfully purified from C. glomerata using ammonium sulfate precipitation, ion-exchange chromatography and size-exclusion chromatography. The limited exploration of green algae suggests a potential area for further investigation to uncover novel enzymes with unique properties (Verdel et al., 2000).
5 Gaps and future directions in haloperoxidase purification from algae
5.1 Limited exploration of green algal haloperoxidases
Green algae are significantly underrepresented in haloperoxidase studies, with only C. glomerata currently featured in the literature (Verdel et al., 2000). This species stands out for its heme-based haloperoxidase, distinct from the vanadium prosthetic groups typically found in red and brown algae. Its enzymatic activity is optimized at a unique pH of 3.1, which could indicate specialized roles in its native environment. However, the limited exploration of green algae represents a significant gap in the field. Given their ecological diversity and abundance in freshwater and marine ecosystems (Van der Loos et al., 2023; Darmawan et al., 2022), green algae likely harbor novel haloperoxidases with unique properties and applications yet to be uncovered. The challenges in studying green algal haloperoxidases include difficulties in identifying target species due to the immense biodiversity of chlorophyta, the low abundance of haloperoxidases in some species, and the instability of these enzymes during extraction and purification processes. Increasingly, the diverse cellular composition and presence of polysaccharides, secondary metabolites, and rigid cell walls in green algae can complicate enzyme isolation. To address these challenges, future research should adopt innovative methodologies for green algae. Strategies could include optimizing enzyme extraction protocols using specific buffer systems and mild mechanical disruption to preserve enzyme activity. In addition, advanced chromatographic techniques, such as affinity chromatography combined with high-performance liquid chromatography (HPLC), could enhance purification efficiency. Moreover, genomic and transcriptomic analyses could also aid in identifying haloperoxidase-encoding genes, enabling heterologous expression in microbial hosts for scalable enzyme production. Furthermore, comparative studies across diverse green algal genera could provide insights into evolutionary adaptations and enzymatic diversity. From an industrial perspective, green algal haloperoxidases could hold significant promise due to their potential for environmentally friendly applications. Their activity at unique pH ranges, such as that observed in C. glomerata (Verdel et al., 2000), could be advantageous in biocatalytic processes that require extreme conditions. Additionally, these enzymes may be leveraged to produce halogenated compounds with antimicrobial, antifouling, or pharmaceutical properties. Hence, expanding the study of green algal haloperoxidases could fill an important knowledge gap and unlock new avenues for sustainable biotechnological innovations.
5.2 Disadvantages of chromatographic techniques in haloperoxidase purification
While traditional purification methods, including ammonium sulfate precipitation, ion-exchange chromatography, and size exclusion chromatography, are well-established, they dominate the field, leaving room for incorporating innovative approaches. However, affinity chromatography, which exploits specific ligand-enzyme interactions, is a highly effective yet underutilized method for isolating target molecules from complex mixtures due to its exceptional specificity (Acikara et al., 2013; Lecas et al., 2021; Poddar et al., 2021; Kalidas et al., 2023). Similarly, emerging microfluidic systems could offer rapid and high-throughput separation capabilities (Markin et al., 2021; Jain et al., 2024). These systems rely on miniaturized fluid channels to manipulate small volumes of liquids with high precision, allowing efficient and scalable purification (Song et al., 2024; Patil et al., 2024). The key advantages of microfluidic platforms include reduced sample and reagent requirements, enhanced control over flow rates, and minimized exposure to harsh conditions, which are particularly beneficial for preserving the activity of sensitive enzymes like haloperoxidases. Furthermore, the integration of microfluidic devices with advanced detection methods, such as fluorescence or mass spectrometry, can enable real-time monitoring of enzyme activity and purity throughout the purification process (Patil et al., 2023; Hu et al., 2023). Despite their potential, both techniques have not yet been applied to the purification of haloperoxidases from algae, highlighting an opportunity for further exploration in this field. Such advancements could streamline the purification process, enhance yield, and reduce losses associated with enzyme degradation. These chromatographic methods, while effective, present significant challenges, primarily due to the numerous steps involved. Each additional step increases the likelihood of product yield loss (Mattrey et al., 2017; Pyka-Pająk, 2024). Moreover, scaling up these techniques for industrial applications proves both complex and costly (Wever et al., 2018).
5.3 Aqueous two-phase systems: an alternative for purification of haloperoxidases from algae
In brown algae rich in alginates which interfere purification process of haloperoxidases (Wever et al., 2018), a promising alternative involves using a two-phase system consisting of polyethylene glycol (PEG 1550) and potassium carbonate (K2CO3) was developed by Jordan and Vilter (1991) and Vilter (1983). This method effectively addresses the challenge by taking advantage of the unique properties of the two-phase system. The alkaline solution dissolves the alginates in the cell walls, thereby releasing haloperoxidases. Due to their hydrophobic nature, these enzymes preferentially partition into the hydrophobic PEG-rich top layer, while the alginates, retained in the aqueous phase due to the high ionic strength of the solution, settle in the lower phase (Wever et al., 2018). This technique has been successfully applied in studies on several brown algae species, such as Laminaria saccharina, Laminaria hyperborea, and Laminaria ochroleuca (Almeida et al., 2001), as well as S. polyschides (Almeida et al., 2001), and L. digitata (Colin et al., 2003). Moreover, it has been extended to members of the Fucaceae family, including Fucus vesiculosus, Fucus serratus, Fucus edentatus, Fucus distichus, A. nodosum, and Pelvetia canaliculata (Verhaeghe et al., 2008; Tarakhovskaya et al., 2015). However, the effectiveness of this method varies between studies, likely due to differences in enzyme characteristics and alginate composition specific to each species.
The purification of haloperoxidases using aqueous two-phase systems (ATPS) presents additional challenges, as the partitioning of these enzymes depends on their size, charge, and hydrophobicity (Segaran and Chua, 2024). The variability in these haloperoxidase characteristics across algal species further complicates the partitioning process, necessitating careful optimization of the system for each type. Key factors influencing ATPS efficiency include the choice of system components and operational parameters (González-Valdez and Mayolo-Deloisa, 2017; Nontawong et al., 2023). For instance, polymer-salt systems such as PEG-potassium carbonate play a pivotal role in achieving effective phase separation while ensuring compatibility with the enzymes and alginates (Wever et al., 2018). The type of salt used, such as potassium phosphate or potassium citrate, affects ionic strength, which is essential for dissolving alginates and stabilizing haloperoxidases. The molecular weight of PEG is another critical parameter, as it influences the hydrophobicity and partitioning behavior of biomolecules (Wysoczanska and Macedo, 2016). For instance, PEG 1000 effectively purified phycocyanin from Spirulina platensis obtained from Ocean University of China (Zhao et al., 2014), while PEG 4000 was more efficient for S. platensis grown under natural Moroccan conditions (Rahim et al., 2024). Similarly, maintaining an appropriate pH is crucial for preserving enzyme stability and ensuring optimal partitioning (Silvério et al., 2013). Adjusting the phase volume ratio can further enhance the concentration of haloperoxidases in the desired phase while minimizing contamination. Additionally, the inclusion and concentration of neutral salts can refine ionic strength and water activity, contributing to improved separation efficiency. To maximize the yield and purity of haloperoxidases, it is essential to systematically optimize these parameters for each specific algal species. These approaches can improve the reproducibility and efficiency of ATPS, allowing better use of this technique for the enzymatic purification of alginate-rich algae.
Scaling up the purification of haloperoxidases remains a critical challenge, particularly when transitioning from laboratory-scale to industrial-scale processes. While it was described as simple, cost-effective, and efficient approach adaptable for large volumes (Segaran and Chua, 2024; Zhang et al., 2024), several practical challenges must be considered. Cost is a significant factor, as large-scale production requires substantial quantities of PEG and salts, which may not always be economically viable. Reproducibility is another concern, as slight variations in component concentrations, pH, or ionic strength can lead to inconsistencies in phase separation and enzyme recovery. Moreover, enzyme degradation is a potential issue during large-scale processing due to prolonged exposure to the system’s components or suboptimal conditions. Optimization strategies can mitigate these challenges. For instance, incorporating stabilizing agents such as glycerol or trehalose can reduce enzyme degradation during prolonged processing (O’Neill et al., 2017; Dinu et al., 2020; Braham et al., 2021). Additionally, fine-tuning PEG molecular weight and salt type can balance cost-effectiveness with purification efficiency. Studies have shown that the use of ATPS for industrial-scale protein purification, such as for therapeutic enzymes, can achieve high yields when operational parameters are carefully controlled (Singla and Sit, 2023; González-Valdez and Mayolo-Deloisa, 2017).
6 Conclusion
Haloperoxidases from marine algae represent a valuable yet underexplored class of enzymes with substantial potential in industrial biocatalysis, pharmaceutical applications, and environmental remediation. Despite the advancements in the purification of these enzymes, challenges remain, particularly in optimizing yield, purity, and bioactivity while scaling up the process for industrial use. The review highlights the underrepresentation of green algal species in haloperoxidase research, signaling a significant gap in our understanding of their enzymatic properties and applications. Additionally, while traditional chromatographic techniques remain effective, the introduction of novel methods, such as affinity chromatography, ATPS, and microfluidic technologies, offers promising avenues to improve purification efficiency and enzyme stability. Sustainable extraction methods that align with green chemistry principles are gaining attention, reflecting the increasing demand for environmentally friendly practices in enzyme production. Moving forward, developing robust, scalable purification techniques customized to the specific biochemical properties of algal species will be essential for maximizing the industrial potential of haloperoxidases. The growing body of research on these enzymes underscores their promise, but more comprehensive studies are needed to fully unlock their diverse applications. Future work should focus on expanding the range of algal species investigated, optimizing purification systems, and addressing scalability issues to ensure the successful commercialization of marine algal haloperoxidases.
Author contributions
AR: Investigation, Writing–original draft. RB: Writing–original draft. AE: Supervision, Writing–review and editing. AC: Supervision, Writing–original draft.
Funding
The author(s) declare that no financial support was received for the research, authorship, and/or publication of this article.
Conflict of interest
The authors declare that the research was conducted in the absence of any commercial or financial relationships that could be construed as a potential conflict of interest.
Generative AI statement
The authors declare that no Generative AI was used in the creation of this manuscript.
Publisher’s note
All claims expressed in this article are solely those of the authors and do not necessarily represent those of their affiliated organizations, or those of the publisher, the editors and the reviewers. Any product that may be evaluated in this article, or claim that may be made by its manufacturer, is not guaranteed or endorsed by the publisher.
Supplementary material
The Supplementary Material for this article can be found online at: https://www.frontiersin.org/articles/10.3389/fntpr.2025.1537097/full#supplementary-material
References
Aboelnga, M. M. (2022). Mechanistic insights into the chemistry of compound I formation in heme peroxidases: quantum chemical investigations of cytochrome c peroxidase. RSC Adv. 12, 15543–15554. doi:10.1039/D2RA01073A
Acikara, O. B., Çitoğlu, G. S., Özbilgin, S., and Ergene, B. (2013). “Affinity chromatography and importance in drug discovery,” in Column chromatography. Editor D. Martin, and B Martin IntechOpen. 59–97.
Ahern, T. J., Allan, G. G., and Medcalf, D. G. (1980). New bromoperoxidase of marine origin. Partial purification and characterization. Biochim. Biophys. Acta. 616, 329–339. doi:10.1016/0005-2744(80)90150-3
Almeida, M., Filipe, S., Humanes, M., Maia, M. F., Melo, R., Severino, N., et al. (2001). Vanadium haloperoxidases from brown algae of the Laminariaceae family. Phytochem 57, 633–642. doi:10.1016/S0031-9422(01)00094-2
Almeida, M., Humanes, M., Melo, R., Silva, J. A., Da Silva, J. F., Vilter, H., et al. (1998). Saccorhiza polyschides (Phaeophyceae; Phyllariaceae) a new source for vanadium-dependent haloperoxidases. Phytochem 48, 229–239. doi:10.1016/S0031-9422(98)00003-X
Almeida, M. G., Humanes, M., Melo, R., Silva, J. A., Da Silva, J. F., and Wever, R. (2000). Purification and characterisation of vanadium haloperoxidases from the brown alga Pelvetia canaliculata. Phytochem 54, 5–11. doi:10.1016/S0031-9422(99)00602-0
Ayala, M., and Eduardo, T. (2015). “Peroxidases as potential industrial biocatalysts,” in Heme peroxidases. Editors E. Raven,, and B. Dunford (Cambridge, United Kingdom: The Royal Society of Chemistry), 309–333. doi:10.1039/9781782622628-00309
Baumgartner, J. T., and Shaun, M. K. (2021). Investigating the role of vanadium-dependent haloperoxidase enzymology in microbial secondary metabolism and chemical ecology. Msystems 6, 10–1128. doi:10.1128/msystems.00780-21
Baykov, S. V., Shetnev, A. A., Semenov, A. V., Baykova, S. O., and Boyarskiy, V. P. (2023). Room temperature synthesis of bioactive 1, 2, 4-oxadiazoles. Int. J. Mol. Sci. 24, 5406. doi:10.3390/ijms24065406
Bhandari, Y., Sajwan, H., Pandita, P., and Koteswara Rao, V. (2023). Chloroperoxidase applications in chemical synthesis of industrial relevance. Biocatal. Biotransfor. 41, 403–420. doi:10.1080/10242422.2022.2107919
Boström, J., Hogner, A., Llinàs, A., Wellner, E., and Plowright, A. T. (2012). Oxadiazoles in medicinal chemistry. J. Med. Chem. 55, 1817–1830. doi:10.1021/jm2013248
Braham, S. A., Siar, E. H., Arana-Peña, S., Bavandi, H., Carballares, D., Morellon-Sterling, R., et al. (2021). Positive effect of glycerol on the stability of immobilized enzymes: is it a universal fact? Process Biochem. 102, 108–121. doi:10.1016/j.procbio.2020.12.015
Butler, A., and Baldwin, A. H. (1997). “Vanadium bromoperoxidase and functional mimics,” in Metal sites in proteins and models, (structure and bonding). Editors H. A. O. Hill, P. J. Sadler, and A. J. Thomson, 109–132.
Butler, A., and Carter-Franklin, J. N. (2004). The role of vanadium bromoperoxidase in the biosynthesis of halogenated marine natural products. Nat. Prod. Rep. 21, 180–188. doi:10.1039/B302337K
Cabrita, M. T., Vale, C., and Rauter, A. P. (2010). Halogenated compounds from marine algae. Mar. Drugs. 8, 2301–2317. doi:10.3390/md8082301
Carter-Franklin, J. N., Parrish, J. D., Tschirret-Guth, R. A., Little, R. D., and Butler, A. (2003). Vanadium haloperoxidase-catalyzed bromination and cyclization of terpenes. J. Am. Chem. Soc. 125, 3688–3689. doi:10.1021/ja029271v
Chandna, P., Sisodia, R., Kuhad, R. C., and Gaur, N. A. (2020). Analyzing the diversity of microbial communities residing in marine ecosystems. J. Mar. Biotechnol. 1, 637–660. doi:10.1002/9781119143802.ch23
Chen, P. Y. T., Adak, S., Chekan, J. R., Liscombe, D. K., Miyanaga, A., Bernhardt, P., et al. (2022). Structural basis of stereospecific vanadium-dependent haloperoxidase family enzymes in napyradiomycin biosynthesis. Biochem. 61, 1844–1852. doi:10.1021/acs.biochem.2c00338
Colin, C., Leblanc, C., Michel, G., Wagner, E., Leize-Wagner, E., Dorsselaer, A. V., et al. (2005). Vanadium-dependent iodoperoxidases in Laminaria digitata, a novel biochemical function diverging from brown algal bromoperoxidases. J. Biol. Inorg. Chem. 10, 156–166. doi:10.1007/s00775-005-0626-8
Colin, C., Leblanc, C., Wagner, E., Delage, L., Leize-Wagner, E., Van Dorsselaer, A., et al. (2003). The brown algal kelp Laminaria digitata features distinct bromoperoxidase and iodoperoxidase activities. J. Biol. Chem. 278, 23545–23552. doi:10.1074/jbc.M300247200
Coupe, E. E., Smyth, M. G., Fosberry, A. P., Hall, R. M., and Littlechild, J. A. (2007). The dodecameric vanadium-dependent haloperoxidase from the marine algae Corallina officinalis: cloning, expression, and refolding of the recombinant enzyme. Protein Expr. Purif. 52, 265–272. doi:10.1016/j.pep.2006.08.010
Darmawan, M., Zamani, N. P., Irianto, H. E., and Madduppa, H. H. (2022). Diversity and abundance of green seaweed caulerpa (chlorophyta) across Indonesian coastal waters with different nutrient levels: bintan Island, Jepara, and Osi Island. J. ilmu Teknol. Kelaut. Trop. 14, 273–290. doi:10.29244/jitkt.v14i2.37745
De Boer, E., Tromp, M. G. M., Plat, H., Krenn, G. E., and Wever, R. (1986). Vanadium (V) as an essential element for haloperoxidase activity in marine brown algae: purification and characterization of a vanadium (V)-containing bromoperoxidase from Laminaria saccharina. Biochim. Biophys. Acta. 872, 104–115. doi:10.1016/0167-4838(86)90153-6
Dhameliya, T. M., Chudasma, S. J., Patel, T. M., and Dave, B. P. (2022). A review on synthetic account of 1, 2, 4-oxadiazoles as anti-infective agents. Mol. Divers. 26, 2967–2980. doi:10.1007/s11030-021-10375-4
Dinu, M. V., Dinu, I. A., Saxer, S. S., Meier, W., Pieles, U., and Bruns, N. (2020). Stabilizing enzymes within polymersomes by coencapsulation of trehalose. Biomacromolecules 22, 134–145. doi:10.1021/acs.biomac.0c00824
Fournier, J. B., Rebuffet, E., Delage, L., Grijol, R., Meslet-Cladière, L., Rzonca, J., et al. (2014). The vanadium iodoperoxidase from the marine Flavobacteriaceae species Zobellia galactanivorans reveals novel molecular and evolutionary features of halide specificity in the vanadium haloperoxidase enzyme family. Appl. Environ. Microbiol. 80, 7561–7573. doi:10.1128/AEM.02430-14
Franssen, M. C. R. (1994). Haloperoxidases: useful catalysts for halogenation and oxidation reactions. Catal. Today. 22, 441–457. doi:10.1016/0920-5861(94)80117-7
García-Zamora, J. L., León-Aguirre, K., Quiroz-Morales, R., Parra-Saldívar, R., Gómez-Patiño, M. B., Arrieta-Baez, D., et al. (2018). Chloroperoxidase-mediated halogenation of selected pharmaceutical micropollutants. Catalysts 8, 32. doi:10.3390/catal8010032
Gérard, E. F., Mokkawes, T., Johannissen, L. O., Warwicker, J., Spiess, R. R., Blanford, C. F., et al. (2023). How is substrate halogenation triggered by the vanadium haloperoxidase from Curvularia inaequalis? ACS Catal. 13, 8247–8261. doi:10.1021/acscatal.3c00761
González-Valdez, J., and Mayolo-Deloisa, K. (2017). “Practical aspects for the development of ATPS-based processes for protein recovery,” in Aqueous two-phase systems for bioprocess development for the recovery of biological products. Food engineering series. Editors M. Rito-Palomares,, and J. Benavides (Cham: Springer), 35–53.
Griffin, E. (2020). Diversity of marine species. J. Mar. Biol. Oceanogr. 9. doi:10.4172/2324-8661.1000219
Hara, I., and Sakurai, T. (1998). Isolation and characterization of vanadium bromoperoxidase from a marine macroalga, Ecklonia stolonifera. J. Inorg. Biochem. 72, 23–28. doi:10.1016/S0162-0134(98)10055-7
Höfler, G. T., But, A., and Hollmann, F. (2019). Haloperoxidases as catalysts in organic synthesis. Org. Biomol. Chem. 17, 9267–9274. doi:10.1039/C9OB01884K
Hu, S., Wang, B., Luo, Q., Zeng, R., Zhang, J., and Cheng, J. (2023). Advances in droplet-based microfluidic high-throughput screening of engineered strains and enzymes based on ultraviolet, visible, and fluorescent spectroscopy. Fermentation 10, 33. doi:10.3390/fermentation10010033
Ishikawa, T., Washio, K., Kaneko, K., Tang, X. R., Morikawa, M., and Okino, T. (2022). Characterization of vanadium-dependent bromoperoxidases involved in the production of brominated sesquiterpenes by the red alga Laurencia okamurae. Appl. Phycol. 3, 120–131. doi:10.1080/26388081.2022.2081933
Itoh, N., Izumi, Y., and Yamada, H. (1985). Purification of bromoperoxidase from Corallina pilulifera. Biochem. Biophys. Res. Commun. 131, 428–435. doi:10.1016/0006-291X(85)91820-0
Jain, A., Stavrakis, S., and Demello, A. (2024). Droplet-based microfluidics and enzyme evolution. Curr. Opin. Biotechnol. 87, 103097. doi:10.1016/j.copbio.2024.103097
Jordan, P., and Vilter, H. (1991). Extraction of proteins from material rich in anionic mucilages: partition and fractionation of vanadate-dependent bromoperoxidases from the brown algae Laminaria digitata and L. saccharina in aqueous polymer two-phase systems. Biochim. Biophys. Acta. 1073, 98–106. doi:10.1016/0304-4165(91)90188-M
Kalidas, C., and Sangaranarayanan, M. V. (2023). “Affinity chromatography,” In Biophysical chemistry: techniques and applications (Cham: Springer Nature Switzerland), 287–299.
Kongkiattikajorn, J., and Pongdam, S. (2006). Vanadium haloperoxidase from the red alga Gracilaria fisheri. Sci. Asia. 32, 25–30. doi:10.1007/978-3-031-37682-5_14
Kongkiattikajorn, J., and Ruenwongsa, P. (2006). Isolation and characterization of vanadium bromoperoxidases from Thai red alga Gracilaria tenuistipitata. Sci. Asia. 321, 31–35. doi:10.2306/scienceasia1513-1874.2006
Küpper, F. C., and Kroneck, P. M. (2014). “Iodine bioinorganic chemistry: physiology, structures, and mechanisms,” In Iodine chemistry and applications, 555–589. doi:10.1002/9781118909911.ch32
La Barre, S., Potin, P., Leblanc, C., and Delage, L. (2010). The halogenated metabolism of brown algae (Phaeophyta), its biological importance and its environmental significance. Mar. drugs. 8, 988–1010. doi:10.3390/md8040988
Lecas, L., Dugas, V., and Demesmay, C. (2021). Affinity chromatography: a powerful tool in drug discovery for investigating ligand/membrane protein interactions. Sep. Purif. Rev. 50, 315–332. doi:10.1080/15422119.2020.1749852
Littlechild, J. (1999). Haloperoxidases and their role in biotransformation reactions. Curr. Opin. Chem. Biol. 3, 28–34. doi:10.1016/s1367-5931(99)80006-4
Littlechild, J., and Isupov, M. (2014). “Haloperoxidase enzymes as ‘redox catalysts’ important for industrial biocatalysis,” in Recent advances in redox active plant and microbial products. Editors C. Jacob, G. Kirsch, A. Slusarenko, P. Winyard, and T. Burkholz (Dordrecht: Springer), 425–446. doi:10.1007/978-94-017-8953-0_17
Markin, C. J., Mokhtari, D. A., Sunden, F., Appel, M. J., Akiva, E., Longwell, S. A., et al. (2021). Revealing enzyme functional architecture via high-throughput microfluidic enzyme kinetics. Science 373, eabf8761. doi:10.1126/science.abf8761
Mattrey, F. T., Makarov, A. A., Regalado, E. L., Bernardoni, F., Figus, M., Hicks, M. B., et al. (2017). Current challenges and future prospects in chromatographic method development for pharmaceutical research. Trac. Trends Anal. Chem. 95, 36–46. doi:10.1016/j.trac.2017.07.021
Nontawong, B., Bunchoen, P., and Ratanapongleka, k. (2023). Factors affecting the partitioning of peroxidase from aqueous two-phase extraction. Eng. Amp. Technol. Horiz. 40, 400306. doi:10.55003/eth.400306
O’Neill, M. K., Piligian, B. F., Olson, C. D., Woodruff, P. J., and Swarts, B. M. (2017). Tailoring trehalose for biomedical and biotechnological applications. Pure Appl. Chem. 89, 1223–1249. doi:10.1515/pac-2016-1025
Pacios, L. F., and Galvez, O. (2010). Active site, catalytic cycle, and iodination reactions of vanadium iodoperoxidase: a computational study. J. Chem. Theory Comput. 6, 1738–1752. doi:10.1021/ct100041x
Patil, P. D., Gargate, N., Dongarsane, K., Jagtap, H., Phirke, A. N., Tiwari, M. S., et al. (2024). Revolutionizing biocatalysis: a review on innovative design and applications of enzyme-immobilized microfluidic devices. Int. J. Biol. Macromol. 281, 136193. doi:10.1016/j.ijbiomac.2024.136193
Patil, P. D., Salokhe, S., Karvekar, A., Suryavanshi, P., Phirke, A. N., Tiwari, M. S., et al. (2023). Microfluidic based continuous enzyme immobilization: a comprehensive review. Int. J. Biol. Macromol. 253, 127358. doi:10.1016/j.ijbiomac.2023.127358
Poddar, S., Sharmeen, S., and Hage, D. S. (2021). Affinity monolith chromatography: a review of general principles and recent developments. Electrophoresis 42, 2577–2598. doi:10.1002/elps.202100163
Punitha, T., Phang, S. M., Juan, J. C., and Beardall, J. (2018). Environmental control of vanadium haloperoxidases and halocarbon emissions in macroalgae. Mar. Biotechnol. 20, 282–303. doi:10.1007/s10126-018-9820-x
Pyka-Pająk, A. (2024). Special issue on “applications of chromatographic separation techniques in food and chemistry”. Processes 12, 304. doi:10.3390/pr12020304
Rahim, A., Salhi, S., El Khelfaoui, N., Badaoui, B., Essamadi, A., and El Amiri, B. (2024). Effect of C-phycocyanin purified from Spirulina platensis on cooled ram semen quality and in vivo fertility. Theriogenology 215, 234–240. doi:10.1016/j.theriogenology.2023.12.007
Rebollar-Pérez, G., Romero-Guido, C., Baez, A., and Torres, E. (2019). “Halogenases with potential applications for the synthesis of halogenated pharmaceuticals,” in Pharmaceutical biocatalysis (Singapore: Jenny Stanford Publishing), 579–602.
Rehder, D. (2014). Vanadate-dependent peroxidases in macroalgae: function, applications, and environmental impact. Oceanography 2, 2. doi:10.4172/2332-2632.1000121
Rush, C., Willetts, A., Davies, G., Dauter, Z., Watson, H., and Littlechild, J. (1995). Purification, crystallisation and preliminary X-ray analysis of the vanadium-dependent haloperoxidase from Corallina officinalis. FEBS Lett. 359, 244–246. doi:10.1016/0014-5793(95)00055-E
Salahuddin, M. A., Yar, M. S., Mazumder, R., Chakraborthy, G. S., Ahsan, M. J., and Rahman, M. U. (2017). Updates on synthesis and biological activities of 1, 3, 4-oxadiazole: a review. Synth. Commun. 47, 1805–1847. doi:10.1080/00397911.2017.1360911
Segaran, A., and Chua, L. S. (2024). Review of recent applications and modifications of aqueous two-phase system for the separation of biomolecules. Int. J. Biol. Macromol. 276, 133856. doi:10.1016/j.ijbiomac.2024.133856
Sharma, M., Patton, Z. E., Shoemaker, C. R., Bacsa, J., and Biegasiewicz, K. F. (2024). N-Halogenation by vanadium-dependent haloperoxidases enables 1, 2, 4-oxadiazole synthesis. Angew. Chem. 136, e202411387. doi:10.1002/anie.202411387
Sheffield, D. J., Harry, T., Smith, A. J., and Rogers, L. J. (1992). Purification and characterization of the vanadium bromoperoxidase from the macroalga Corallina officinalis. Phytochem 32, 21–26. doi:10.1016/0031-9422(92)80099-Z
Silvério, S. C., Rodríguez, O., Tavares, A. P. M., Teixeira, J. A., and Macedo, E. A. (2013). Laccase recovery with aqueous two-phase systems: enzyme partitioning and stability. J. Mol. Catal. B Enzym. 87, 37–43. doi:10.1016/j.molcatb.2012.10.010
Singla, M., and Sit, N. (2023). Theoretical aspects and applications of aqueous two-phase systems. ChemBioEng. Rev. 10, 65–80. doi:10.1002/cben.202200026
Song, Y., Zhou, Y., Zhang, K., Fan, Z., Zhang, F., and Wei, M. (2024). Microfluidic programmable strategies for channels and flow. Lab. Chip 24, 4483–4513. doi:10.1039/D4LC00423J
Tarakhovskaya, E. R., Bilova, T. E., and Maslov, Y. I. (2015). Hydrogen peroxide content and vanadium-dependent haloperoxidase activity in thalli of six species of Fucales (Phaeophyceae). Phycologia 54, 417–424. doi:10.2216/15-35.1
Ten Brink, H. B., Schoemaker, H. E., and Wever, R. (2001). Sulfoxidation mechanism of vanadium bromoperoxidase from Ascophyllum nodosum: evidence for direct oxygen transfer catalysis. Eur. J. Biochem. 268, 132–138. doi:10.1046/j.1432-1327.2001.01856.x
Thapa, H. R., Lin, Z., Yi, D., Smith, J. E., Schmidt, E. W., and Agarwal, V. (2020). Genetic and biochemical reconstitution of bromoform biosynthesis in Asparagopsis lends insights into seaweed reactive oxygen species enzymology. ACS Chem. Biol. 15 (6), 1662–1670. doi:10.1021/acschembio.0c00299
Tripathi, G., Dubey, P., Ahmad, S., Farooqui, A., and Mishra, V. (2024). Role of algal-derived bioactive compounds in human health. Recent Pat. Biotechnol. 18, 190–209. doi:10.2174/1872208317666230623141740
van der Loos, L. M., De Coninck, L., Zell, R., Lequime, S., Willems, A., De Clerck, O., et al. (2023). Highly divergent CRESS DNA and picorna-like viruses associated with bleached thalli of the green seaweed Ulva. Microbiol. Spectr. 11, e0025523–23. doi:10.1128/spectrum.00255-23
Verdel, E. F., Kline, P. C., Wani, S., and Woods, A. E. (2000). Purification and partial characterization of haloperoxidase from fresh water algae Cladophora glomerata. Comp. Biochem. Physiol. B Biochem. Mol. Biol. 125, 179–187. doi:10.1016/S0305-0491(99)00168-6
Verhaeghe, E., Buisson, D., Zekri, E., Leblanc, C., Potin, P., and Ambroise, Y. (2008). A colorimetric assay for steady-state analyses of iodo-and bromoperoxidase activities. Anal. Biochem. 379, 60–65. doi:10.1016/j.ab.2008.04.041
Vilter, H. (1983). Peroxidases from phaeophyceae IV. Fractionation and location of peroxidase isoenzymes in Ascophyllum nodosum (L.). Bot. Mar. 26, 451–455. doi:10.1515/botm.1983.26.10.451
Weinberger, F., Coquempot, B., Forner, S., Morin, P., Kloareg, B., and Potin, P. (2007). Different regulation of haloperoxidation during agar oligosaccharide-activated defence mechanisms in two related red algae, Gracilaria sp. and Gracilaria chilensis. J. Exp. Bot. 58, 4365–4372. doi:10.1093/jxb/erm303
Wever, R., Krenn, B. E., and Renirie, R. (2018). Marine vanadium-dependent haloperoxidases, their isolation, characterization, and application. Methods. Enzymol. 605, 141–201. doi:10.1016/bs.mie.2018.02.026
Wever, R., Plat, H., and de Boer, E. (1985). Isolation procedure and some properties of the bromoperoxidase from the seaweed Ascophyllum nodosum. Biochim. Biophys. Acta 830, 181–186. doi:10.1016/0167-4838(85)90026-3
Wischang, D., Brücher, O., and Hartung, J. (2011a). Bromoperoxidases and functional enzyme mimics as catalysts for oxidative bromination—a sustainable synthetic approach. Coord. Chem. Rev. 255, 2204–2217. doi:10.1016/j.ccr.2011.04.003
Wischang, D., and Hartung, J. (2011). Parameters for bromination of pyrroles in bromoperoxidase-catalyzed oxidations. Tetrahedron. 67, 4048–4054. doi:10.1016/j.tet.2011.04.010
Wischang, D., Hartung, J., Hahn, T., Ulber, R., Stumpf, T., and Fecher-Trost, C. (2011b). Vanadate (v)-dependent bromoperoxidase immobilized on magnetic beads as reusable catalyst for oxidative bromination. Green. Chem. 13, 102–108. doi:10.1039/C0GC00499E
Wysoczanska, K., and Macedo, E. A. (2016). Effect of molecular weight of polyethylene glycol on the partitioning of DNP-amino acids: PEG (4000, 6000) with sodium citrate at 298.15 K. Fluid Ph. Equilib. 428, 84–91. doi:10.1016/j.fluid.2016.07.009
Zhang, X., Cai, Z., Wang, L., Xie, S., and Zong, W. (2024). Unlocking liquid-liquid separation: exploring the marvels of aqueous two-phase systems. Microchem. J. 200, 110445. doi:10.1016/j.microc.2024.110445
Zhang, X., and Liu, Y. (2023). Direct electrophilic attack of compound I on the indole ring in the peroxygenase mechanism of dehaloperoxidase DHP B in degrading haloindole: electron transfer promotes the reaction. Inorg. Chem. 62, 13230–13240. doi:10.1021/acs.inorgchem.3c01425
Keywords: marine algae, haloperoxidases, purification techniques, aqueous two-phase systems, industrial applications, biocatalysis
Citation: Rahim A, Benjamaa R, Essamadi AK and Chafik A (2025) A critical review on purification methods and applications of marine algal haloperoxidases. Front. Nat. Prod. 4:1537097. doi: 10.3389/fntpr.2025.1537097
Received: 29 November 2024; Accepted: 27 February 2025;
Published: 13 March 2025.
Edited by:
Weaam Ebrahim, Mansoura University, EgyptReviewed by:
Pallavi Sharma, University of Lincoln, United KingdomOmar Al-Dulaimi, London South Bank University, United Kingdom
Copyright © 2025 Rahim, Benjamaa, Essamadi and Chafik. This is an open-access article distributed under the terms of the Creative Commons Attribution License (CC BY). The use, distribution or reproduction in other forums is permitted, provided the original author(s) and the copyright owner(s) are credited and that the original publication in this journal is cited, in accordance with accepted academic practice. No use, distribution or reproduction is permitted which does not comply with these terms.
*Correspondence: Abdellatif Rahim, YS5yYWhpbUB1aHAuYWMubWE=, YWJkZWxsYXRpZi5yYWhpbUB1aHAuYWMubWE=