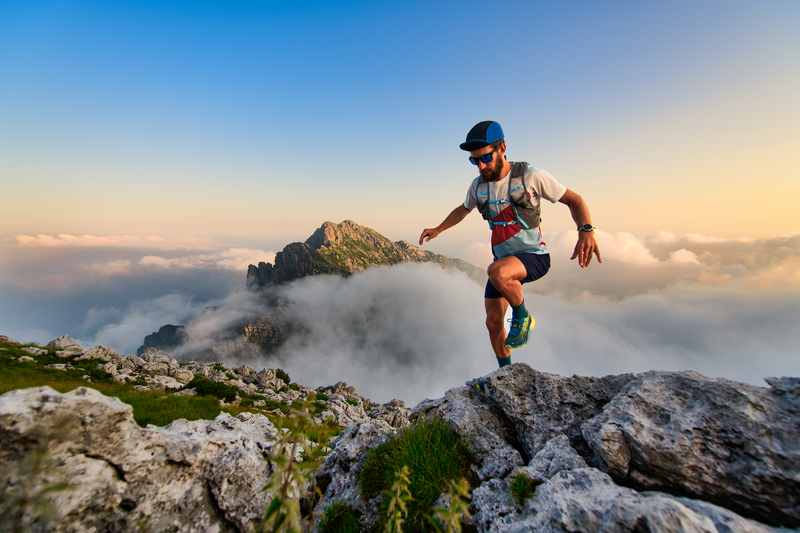
95% of researchers rate our articles as excellent or good
Learn more about the work of our research integrity team to safeguard the quality of each article we publish.
Find out more
REVIEW article
Front. Nanotechnol. , 18 March 2025
Sec. Biomedical Nanotechnology
Volume 7 - 2025 | https://doi.org/10.3389/fnano.2025.1564188
Despite significant advancements in pharmaceutical sciences, conventional drug delivery system remains limited by issues like poor permeability, toxicity, suboptimal efficacy, and inadequate targeting. These challenges pose substantial barrier to effective treatment for complex conditions like cancer, heart problems, chronic pain management, etc. Mesoporous silica nanoparticle (MSN), with their remarkable structural tunability and multifunctionality, have emerged as a transformative solution in the realm of drug delivery system. This review delves into the state-of-the-art synthesis methods of MSNs including physical, chemical, top down and bottom-up approaches with particular attention to the widely used Sol-Gel process. We also explore innovative drug loading strategies and controlled release mechanisms, underscoring how factors such as pore size, particle shape, and surface charge influence therapeutic outcomes. Furthermore, we highlight the burgeoning applications of MSNs across multiple domains, ranging from anticancer therapy and gene delivery to emerging fields such as precision agriculture and environmental remediation. Recent studies demonstrate the versatility of MSNs in addressing both biomedical and ecological challenges, making them an indispensable tool in modern science. By synthesizing Collectively, this review aims to provide a comprehensive resource for researchers and practitioners, fostering continued innovation in the design and application of MSN-based nanotechnology.
Mesoporous silica nanoparticles (MSNs) have garnered significant attention from academia and the pharmaceutical industry in the last 20 years as potential drug delivery systems (DDS) (Trzeciak et al., 2021a). Polymeric based nanoparticles (Kankala et al., 2017), dendrimers (Kankala et al., 2018), and lipid based (Gong et al., 2015) have resulted in treatment process of many diseases including infectious disorders. Both organic and inorganic type nanoparticles have been studied thoroughly for its application in medicine (Alhariri et al., 2013). Iron oxide and quantum dots are being marketed. Carbon dots, silica nanoparticles, layered double hydroxide nanoparticles, other metal oxide nanoparticles like gold or silver are also used in therapeutic activities (Deshpande et al., 2013; Puri et al., 2009). Cornell dots combining radioactive iodide and organic colors are approved for phase one trials (Gad et al., 2016), Because of its highly biocompatible nature, variable surface functionalization properties, and flexible structure and composition, mesoporous silica nanoparticles (MSNs) are a prime example of nanotechnology used in biomedicine (Xu et al., 2023). For many years, researchers have faced great difficulty when it comes to drug nano-formulations. It is still very difficult to design safe, biocompatible devices that deliver exact medication concentrations to specific areas via in vivo barriers. Using intelligent drug delivery systems (DDS) is a viable approach to solving this problem. In this effort, mesoporous silica shows promise as a material. Mesoporous silica nanoparticles (MSNs) have been around since the early 1990s and have been used in a wide range of scientific fields and businesses, including molecular biology, electrochemistry, heterogeneous catalysis, and analytical chemistry. The first time these nanostructured materials were used in pharmacy was in 2001, when MCM-41 silica was used for the first time in ibuprofen release methods by Vallet-Regi et al. (2001). This ground-breaking study stimulated ongoing investigation into the potential of mesoporous particles as drug delivery system carriers. Interestingly, silica has uses in food additives and cosmetics and is FDA-approved as “Generally Recognized as Safe” (GRAS) (Huang R. et al., 2020). In a positive step, the US FDA approved small silica nanoparticles as imaging agents for a human clinical study (Phillips et al., 2014a). Scientists are encouraged by this discovery that MSNs might be used as drug-delivery vehicles in clinical settings. Additionally, research using simulated bodily fluids has shown that mesoporous silica nanoparticles degrade in three stages after delivery; this characteristic is helpful for the kinetics of drug release (Lin et al., 2012). Mesoporous silica nanoparticles are silica compounds at the nanoscale that have porous properties, as the name implies. MSNs have pores of a diameter of 2–50 nm, according to the International Union of Pure and Applied Chemistry (IUPAC) classification (Isa et al., 2019). Nanomaterials also referred to as nanomedicine, are used to deliver medications and may have sizes as small as hundreds of nanometers (Pillai et al., 2019). The Mobil Oil Research Group found and named it M41S (Kresge et al., 1992). It was by chance that this was discovered. M41S exhibited large pore volumes, variable pore sizes, thin amorphous walls, high surface area, and low Bronsted acidity (Kresge et al., 2004; Habeche et al., 2020). The three main members of M41S are MCM-41, MCM-48, and MCM-50. MCM-48 features cubic mesostructured gyroidic phase Ia3d, MCM-41 has a hexagonal pore structure, and MCM-50 has a lamellar geometry consisting of silicate or porous aluminosilicate layers divided by surfactant layers (Rahikkala et al., 2018).
Due to its complex manufacturing and thermal instability, MCM-48 and MCM-50 are the least studied (Habeche et al., 2020), as shown in Figure 1. Various kinds of MSNs have been discovered, such as hollow MSNs, amorphous family (SBA-n) from Fudan University (FDU), folded sheet mesoporous material-16 (FSM-16) (Santa Barbara Pharmaceuticals 2021), and others (Chew et al., 2010). This section looks into both new and current methods for creating MSNs. Because of their superior physicochemical stability, biocompatibility, one-step functionalization, large surface area and pore volume, easy production, and customizable particle size and shape, MSNs are often used as drug delivery agents as mentioned in Table 1 (Niculescu, 2020).
Figure 1. Representation of various mesoporous silica nanoparticles (MSNs) with distinct structural classifications (He et al., 2018).
Table 1. Summary of the advantages and limitations of mesoporous silica nanoparticles (MSNs) in biomedical applications (Jyothirmayi et al., 2024).
For the synthesis of MSN multiple adjustments can be done like changes in pH, Surfactant and silica source. Hollow silica Nanoparticles (HSN) sub class of mesoporous silica nanoparticles are widely used for increasing drug loading and pore volume., can be prepared by various techniques like soft templating method (single vessel templating, vesicle templating and micro emulsion templating method), hard templating method and polymerlatexes-templating method, stober method, sol gel method and many more (Jyothirmayi et al., 2024).
There are four significant nanoparticle kinds synthesis methods: physical, chemical, and bottom-up and top-down. Physical synthesis methods include procedures such as inert gas condensation, severe plastic deformation, ultrasonic shot peeling, high-energy ball milling, grinding, and pyrolysis. These procedures are routinely used to create metallic nanoparticles (Maribel et al., 2009). Chemical techniques depend on chemical reduction processes (Rodríguez-Sánchez et al., 2000), electrochemical operations (Sharma et al., 2009) or phytochemical reduction (Raspolli Galletti et al., 2013). Examples include microemulsion, chemical coprecipitation, chemical vapor condensation, and pulse electrodeposition. The most common chemical synthesis is Sol-gel.
Bottom-up approaches begin at the atomic level and continue with the synthesis of molecules. In the top-down technique, the resultant nanoparticles have a higher beginning size. To obtain the required size, mechanical methods or acid additions are used to decrease particle size (Mallick et al., 2004).
The Chemical approaches for nanoparticle production are toxic and dangerous substances, endangering biological systems and the environment (Shankar et al., 2005). In response to these issues, green nanotechnology has evolved as an essential subfield of nanotechnology. Green nanotechnology focuses on safe and eco-friendly techniques for nanomaterial manufacturing, offering an alternative to traditional physical and chemical synthesis processes. Green nanotechnology uses microorganisms such as fungi, algae, and bacteria, as well as plant extracts, including biomolecules like proteins, enzymes, amino acids, and vitamins. These natural sources play an essential role in bio reduction, bioleaching, and nanoparticle production (Van Emden et al., 1988). Green nanotechnology is gaining popularity because it eliminates toxic chemical reagents, resulting in more effective and cost-efficient product synthesis (Raspolli Galletti et al., 2013).
The Sol-Gel process allows for the production of nanoparticles in a variety of sizes to meet specific requirements. The Sol-Gel approach relies on condensation hydrolysis reactions using organic silicone precursors like TEOS or TMOS. During this process, the precursors are hydrolyzed under acidic conditions to produce silanol (Si-OH). These silanol groups then condense to create siloxane (Si-O-Si) bonds, which ultimately contribute to gel formation. Several templates, including CTAB, are employed to cause mesoporosity. Following gel formation, drying and calcination are used to remove solvent and surfactant molecules, resulting in the development of mesoporous silica. The hydrolysis and condensation processes produce a new phase known as the “Sol”, which is subsequently converted into the “Gel” phase by particle condensation (Kinnari et al., 2011; Brinker and Scherer, 1990).
The general reactions of TEOS that lead to the formation of silica particles in the sol-gel process can be written as given below.
The Stober technique is a remarkable version of the Sol-Gel technology with unique characteristics (Kurdyukov et al., 2018). This approach is very effective in producing monodispersed silicon nanoparticles ranging in size from 50 to 2000 nm. It does this by using ammonia as a catalyst, alcohol as a reaction media, and TEOS (Stöber et al., 1968) as a precursor. MSNPs synthesized using the Stober technique have an organized structure with consistent mesopores of fewer than 100 nm. Additionally, they have specified surface areas that range from 600 to 1,000 m2/g and pore volumes spanning from 0.6 to 1.0 mL/g (Wang et al., 2014).
Initially, Grun et al. pioneered the creation of sub micrometer-scaled MCM-41 particles utilizing a modified Stober technique (Grün et al., 1997). These particles were then further refined, culminating in 100 nm particles using a dilute surfactant solution (Cai et al., 2001). To create MSNPs smaller than 50 nm, procedures such as dialysis or a dual surfactant approach have been used, as mentioned in (Suzuki et al., 2004).
In an ideal situation, MCM-41 100 nm particles are synthesized by adding TEOS to a hot basic aqueous solution of CTAB (pH 11). Nanoparticles are produced using base-catalyzed Sol-Gel Condensation and hexagon-shaped micelles (Coti et al., 2009; Wu SH. et al., 2011).
In recent days, silica bases are extensively utilized carriers for medicinal substances, with the amorphous form being the most widespread because of its lesser harmful effects than crystalline silica (Buyuktimkin and Wurster, 2015; McCarthy et al., 2016; Bharti et al., 2015; Jeelani et al., 2020; Miura et al., 2010; Carvalho et al., 2020). The properties of silica structure may be improved by regulating its pores and creating organized mesopore silica particles (Jeelani et al., 2020; Chircov et al., 2020), which has a high surface area (>700 m2/g) and pore volume (over 1 cm2/g), resulting in regulated release (Zid et al., 2020). MSNs have two surfaces: an inner and outer membrane. This outer membrane may help to improve the efficiency of targeted distribution (Natarajan and Selvaraj, 2014; Le et al., 2019). The most frequent MSNs are HMS (hollow mesoporous silica), MSU (Michigan State University), and MCF (meso cellular form), among others. Now, MSNP loading techniques are classified into two types such as solvent-based methods (wet method) and solvent-free methods, as illustrated in Figure 2 (Seljak et al., 2020; Li et al., 2019). Adsorption, solvent evaporation, incipient wetness impregnation, diffusion-supported loading, one-pot drug loading, co-spraying, and covalent grafting are some examples of solvent-based approaches. Physical mixing, melting, co-milling, and microwave irradiation are all solvent-free processes. The most common methods are solvent evaporation, adsorption, incipient wetness impregnation, melt method, and co-miling, in which the desired drug is adsorbed onto the surface of the MSNP under favorable conditions that promote drug carrier interaction such as van der Waals, hydrogen bonding, and so on (Andersson et al., 2004). Drug loading varies based on surface area and affinity to silica substrate (Singh et al., 2011; Lehto et al., 2014). A comprehensive overview of drug loading methods utilizing mesoporous silica particles (MSPs), categorized by drug type, Biopharmaceutics Classification System (BCS) class are listed in Table 2.
Figure 2. Illustration of various drug loading techniques categorized into solvent-based and solvent-free methods.
Table 2. Comprehensive overview of drug loading methods utilizing mesoporous silica particles (MSPs), categorized by drug type, Biopharmaceutics Classification System (BCS) class, and literature references (Maleki et al., 2017).
Such method is used to Increase the drug release kinetics through MSN.
Mesoporous silica nanoparticle (MSN) are submerged in a concentrated drug solution, which causes drug molecules to adhere to their surfaces. These drug-laden MSN are then isolated from the solution using filtering or centrifugation before being dried to eliminate any leftover solvent remnants (Xie et al., 2016)., Because of its low operating temperature, the adsorption technique is frequently employed for both hydrophilic (Brás et al., 2014) and hydrophobic medicines (Li and Zhang, 2006), as well as thermally sensitive compounds. However, with poorly soluble medications, getting a high drug solution concentration is problematic (Xie et al., 2016), as an increase in the concentration of drug solution results in mesopores obstructions and a reduction in loading surface area (Zheng et al., 2019). Another barrier is time consumption, which is compounded by uncertain medication loading levels and high drug waste (Šoltys et al., 2019). Nonetheless, solvent selection significantly impacts the drug loading process; the solvent with the most significant active pharmaceutical ingredient (API) solubility may not be optimum. For example, valsartan loaded better in dichloromethane than in methanol despite the latter’s more significant concentration. Similarly, aprepitant showed thrice greater loading in chloroform than in methanol (Charnay et al., 2004). Charnay et al. found that using polar solvents such as dimethyl sulfoxide and dimethyl formamide resulted in poor ibuprofen loading into MCM-41 material (Mellaerts et al., 2008).
For the synthesis of catalysts (Campanati et al., 2003; Van Speybroeck et al., 2009) and drug loading into mesoporous particles (Trzeciak et al., 2020; Mellaerts et al., 2008; Wang et al., 2015a), this approach works well. This process involves adding a concentrated drug solution, dropwise, to the silica, so that the drug’s volume matches the pore volume. The drug-diffusing wet powder is then dried for 24 h, vacuum-pressed for 48 h at 40°C (Wang et al., 2015a), and excess drug coating is removed by washing with a solvent. Washing to get rid of extra medication is a crucial step that has a significant impact.
Washing MCM-41 decreased the quantity of encapsulated ibuprofen from 1,350 to 500 mg/g, according to Charnay et al. (Mellaerts et al., 2008). This process’s primary benefits are the accurate measurement of the drug load (Wang et al., 2015a) and the complete use of a sizable MSN pore capacity. High concentrated drug solution and repeated impregnation are utilised to achieve a high degree of drug loading (5%–40% w/w) (Šoltys et al., 2019).
It is also a frequently employed procedure that includes solvent evaporation, which modifies the physical state, localization, and rate of release from the carrier, and adsorption, which removes the solvent via filtering and results in drug loss (Wang et al., 2015a; Abd-elbary et al., 2014). To create drug-loaded MSN, the silica is now dissolved in a volatile organic solution such as ethanol or dichloromethane and dried using a rotary evaporator (Ambrogi et al., 2012b) or heating (Maleki et al., 2017; He et al., 2017; Deraz, 2017). Mellaerts et al. used solvent evaporation and incipient wetness impregnation techniques to load itraconazole and ibuprofen onto SBA-15 Silica (Trzeciak et al., 2020).
Potrezebowski et al.'s DiSupLo methodology provides an easy-to-use method for packing medications inside mesoporous silica nanoparticle (MSN) (Liu et al., 2012). A predetermined amount of MSN and a pre-homogenized drug combination are added to an open weighing vessel, which is then in a sealed vessel and left to sit at room temperature for 3 hours with ethanol within. Using NMR, the length of the drug-loading process is tracked every 30 min. Eventually, the drug-loaded sample’s ethanol is thermally extracted. This method’s adaptability is proven by loading nonsteroidal anti-inflammatory medicines (NSAIDs) such as ibuprofen, flurbiprofen, and ketoprofen into MCM-41 pores. Multiple investigations have shown good medication integration utilizing a 1:3 weight ratio of API (active pharmaceutical ingredient) to MCM-41, with filling factors of 33% and 50%, respectively. When a 1:1 ratio is used, a part of the API is located within the pore with a filling factor of roughly 60%, while the remaining stays on the exterior wall of MCM-41 (Liu et al., 2012). When the API/MSN weight ratio is less than the maximum filling factor, the DiSupLo technique allows for high drug loading and full API encapsulation. While most research focuses on single-drug loading and seldom investigates complexity, binary, or ternary systems (Rosenholm et al., 2009; Ahern et al., 2012), this approach allows for the simultaneous loading of two or more components with the appropriate drug composition (Liu et al., 2012).
In addition to MSN medication loading, it is often employed in food and chromatography (Bouledjouidja et al., 2016; Kompella and Koushik, 2001; Pasquali and Bettini, 2008). Supercritical fluids are utilized here because they have various benefits over organic solvents, such as liquid-like density, gas-like viscosity, low interfacial tension, and so on, which give them an edge as an impregnating agent (Bush et al., 2007). The supercritical phase allows for easier transfer of solubilized substances to the solid matrix, resulting in faster and more uniform impregnation than in the liquid phase. CO2 is a popular solvent because of its moderate critical condition (31.2°C, 7.4 MPa) in the SCF process. It is also inert, non-flammable, and non-toxic. (Kompella and Koushik, 2001; Wang LH. et al., 2013). The SCF approach offers an alternate method of loading onto silica that reduces processing time to 2 h (Hillerström et al., 2014). Stan et al. demonstrated that a relatively modest concentration of ibuprofen in nonpolar liquid CO2 was sufficient to produce the maximal loading of MCM-41 (Gurikov and Smirnova, 2018). Despite the benefits of SCF, multiple investigations have shown that many medications have low SCCO2 solubility (Kerkhofs et al., 2015; Nuchuchua et al., 2017).
Drug encapsulation may be accomplished by including pharmaceuticals into the production phase of mesoporous silica nanoparticle (MSN) (Dementeva et al., 2018). MSN is typically produced using a sol-gel technique using inert surfactant micelles as templating agents, which are then removed using extraction or calcination procedures (Andersson et al., 2004). After MSN synthesis, drug loading is carried out utilizing recognized procedures (Li et al., 2019; Maleki et al., 2017; Hillerström et al., 2014). However, these traditional procedures are often multistep and time-consuming, resulting in limited drug absorption and burst release, presenting issues for many formulations (Dementeva and Rudoy, 2016). The combination of MSN production and medication loading solves these challenges. Dementia et al. suggested employing micelles of myramistin (an antibacterial drug) as templates in the sol-gel fabrication of mesoporous silica carriers (Dementeva et al., 2016). This approach produces a high drug content (>1 g/g of SiO2) and is pH sensitive, with potential applications to additional amphiphilic medicines such as anticancer and anti-inflammatory compounds (Dementeva et al., 2018; Davis et al., 2011; Zhou et al., 2013; Wan et al., 2016). Wan et al. successfully loaded two medicines, hydrophilic heparin, and hydrophobic ibuprofen, onto mesoporous silica using a one-pot synthesis technique. This approach, which uses Evaporation Induced Self Assembly (EISA) for in situ drug loading, has various benefits, including a considerable decrease in delivery system setup time from 74 to 10 h (Wang N. et al., 2019).
Covalent grafting has emerged as a well-established method for loading medicines onto mesoporous silica nanoparticle (MSN) (Ahern et al., 2012; Fasiku et al., 2019; Giret et al., 2015). The functional groups on the outside of MSN walls allow for covalent interaction with medications, making it a more viable choice than adsorption with increased accuracy. This method provides more control, limiting undesired medication release before reaching the target location. Covalently connecting medications involves using common bonds such as amide, disulfide, ester, thiol, etc. The following drug release from these systems occurs by hydrolysis, enzymatic degradation, exchange processes, or redox reactions (Wong and Choi, 2015). This releasing process, known as linker cleavage, is well-addressed in the literature (Ding and Li, 2017; Mortera et al., 2009a). Notably, cysteine, sulfasalazine (a prodrug), and paclitaxel all exhibit this mechanism (Popat et al., 2012; Rosenholm et al., 2010; Uejo et al., 2013). Rosenholm et al. demonstrated the flexibility and effectiveness of this approach by covalently bonding methotrexate (MTX) to poly (ethyleneimine)-functionalized MSN (Yuan et al., 2013).
These offer a high degree of medication loading and take less time. Furthermore, since the API/MSN ratio directly affects the drug concentration in the mesopore, it is simpler to forecast.
The drug loading technique entails heating a drug-mesoporous silica combination over the active pharmaceutical ingredient’s (API) melting point (Kinnari et al., 2011; Waters et al., 2013). Potrzebowski et al. found that the thermal solvent-free melt approach is more successful in incorporating ibuprofen into MCM-41 than the incipient wetness method. The filling factor, calculated as the weight-to-weight ratio of ibuprofen to MCM-41, was about 60%. However, this strategy is only appropriate for thermally stable medications, since some research indicates a higher relationship between molten viscosity (Hampsey et al., 2004).
A controlled heating procedure, in which the drug’s temperature is managed during loading via a feedback mechanism, is an alternate method for drug loading into silica particles. This approach efficiently stops medication deterioration (Waters et al., 2013). Waters et al., along with collaborators, used this approach to load fenofibrate into a variety of silica structures, including SBA-15 (Skorupska et al., 2014).
Microwave irradiation (300 MHz–300 GHz) is widely used for energy generation through heat production. When polar and charged molecules interact with microwaves, they align with the oscillating field, leading to rapid agitation, energy dissipation, and internal heating (Sun et al., 2016; Gupta and Eugene, 2011; Peng et al., 2012). Unlike conventional heating, which transfers heat from the surface inward, microwaves enable uniform internal heating, reducing reaction times, enhancing yields, and minimizing by-products (Jian et al., 2019; Guo et al., 2020; Schanche, 2003; Kappe and Dallinger, 2009; Kappe, 2008).
This technology has been applied across diverse fields, including food processing (Sansano et al., 2018), water treatment (Wei et al., 2020), and chemical synthesis (Mirzaei and Neri, 2016). In synthesis, microwaves have facilitated the production of various materials, including metal oxides (Mirzaei and Neri, 2016), nanomaterials (Tsuji, 2017), MOFs (Khan and Jhung, 2015), polymers (Ebner et al., 2011), and graphene-based compounds (Li et al., 2017). While reviews exist on microwave-assisted synthesis of porous materials, a comprehensive study on silica materials synthesized via this method remains lacking (Cao et al., 2009; Park et al., 2004; Tompsett et al., 2006; Nithya et al., 2019). This review aims to fill that gap, focusing on mesoporous and non-porous silica synthesized through microwave techniques and their applications.
Microwave-assisted synthesis has revolutionized the fabrication of mesoporous silica nanoparticles (MSNs) by offering unparalleled efficiency, precision, and sustainability. Unlike conventional thermal methods, which rely on surface-to-bulk heat transfer and often result in localized overheating and prolonged reaction times, microwave irradiation induces volumetric heating, ensuring rapid nucleation, uniform temperature distribution, and superior structural integrity. This technique drastically reduces synthesis duration—from hours to mere minutes—while enabling precise control over pore architecture, surface area, and particle morphology, thereby enhancing MSN functionality for targeted applications. Furthermore, its inherent ability to minimize by-product formation and eliminate excessive purification steps positions it as a highly efficient and environmentally sustainable alternative to traditional hydrothermal synthesis. The method has been extensively employed to engineer highly ordered mesoporous structures, such as SBA-15, SBA-16, and MCM-41, which play pivotal roles in catalysis, drug delivery, adsorption, and environmental remediation. By allowing precise modulation of irradiation power, surfactant concentration, and reaction kinetics, microwave-assisted synthesis elevates the potential of MSNs, offering a scalable, eco-conscious, and next-generation approach for advanced material development (Díaz de Greñu et al., 2020).
Milling is a flexible process for creating submicrometric particles (Willart and Descamps, 2008) and solid amorphization (Trzeciak et al., 2021b). Research has shown its efficacy, notably with organic molecules such as benzoic acid and 4-fluorobenzoic acid, which may be integrated into MCM-41 pores by planetary ball milling (Malfait et al., 2019). The loading process for guest compounds is determined by the substance-to-silica ratio, with maximum loading capabilities of more than 50% recorded at a 1:1 ratio. Furthermore, this approach permits guest molecules to be spread throughout many zones of MCM-41 (Malfait et al., 2019). Notably, Hedous et al. show that co-milling SBA-15 with ibuprofen increases drug loading into the matrix, with a filling degree of 40% by weight (Fu et al., 2010). Milling is a feasible alternative to solvent-based processes, providing simplicity, decreased time consumption, and scalability for industrial applications.
Drug absorption is common during mesoporous silica nanoparticle (MSNP) release, as shown in Figure 3. However, owing to limited control over mesopores, the delivery rate is of sustained type, reliant on drug dissolution. Aqueous-soluble medicines have less favorable release dynamics than hydrophobic pharmaceuticals since lower dissolution allows for better control over release kinetics as mentioned in Table 3. In Vitro, investigations have shown that interactions between particles and phospholipids (cell membranes) during endocytosis might improve the delivery of encapsulated hydrophobic medicines (Lu et al., 2007). To ensure regulated release, polymers are absorbed or covalently attached to particle surfaces (Fu et al., 2003). In closed conditions, polymer chains block apertures, preventing medication release. However, they may inflate or coil in response to particular stimuli, exposing pores and enabling medication administration. Another method for regulated release is to create chemical bonds across pores (Mal et al., 2003). Alternatively, bulky groups (such as Au) may be added to pore openings to serve as gatekeepers (Vivero-Escoto et al., 2010a). These bulky groups interact non-covalently, resulting in nanomachines such as nano valves and snap-tops (Coti et al., 2009). Various stimuli may be used to provide effective control, including chemical stimuli in which particles react to external chemical additions or internal changes (e.g., pH, enzymes) (Coti et al., 2009; Vivero-Escoto et al., 2010a; Manzano et al., 2009). Electrical stimuli via external electrodes or internal reducing conditions provides another avenue for regulation (Ambrogio et al., 2010; Mortera et al., 2009b), or light as a power source for release mechanisms (Mal et al., 2003; Angelos et al., 2007).
Figure 3. The schematic representation of mesoporous channels in nanoparticles facilitates enhanced drug diffusion and release kinetics.
Table 3. Drug release profiles for different carriers, highlighting variations in release rates and sustained drug delivery kinetics (Bush et al., 2007).
Matsumura established the idea of passive targeting in 1986, which is based on tumour tissues’ leaky, discontinuous microvasculature and poor lymphatic drainage, resulting in increased permeability and retention (EPR) as shown in Figure 4C. Tumours exhibit fast angiogenesis to support proliferation (Matsumura and Maeda, 1986), resulting in vascular openings that allow nanocarriers to penetrate and accumulate. However, in order to accumulate efficiently, mesoporous silica nanoparticles (MSNs) must avoid fast renal clearance and the reticuloendothelial system. This method has been widely used to improve tumour targeting using silica nanocarriers (Zhang et al., 2016). Functionalized MSNs, for example, that included magnetic nanoparticles in mesoporous shells and surface changes, were able to successfully reach tumour locations in mice via passive targeting. These MSNs have also been used to produce multimodal imaging probes for MRI applications (Lee et al., 2011).
Figure 4. Schematic representation of nanoparticle (NP)-based drug delivery strategies. (A) Various types of nanoparticles, including liposomes, micelles, mesoporous silica nanoparticles (MSNs), dendrimers, and gold nanoparticles, serve as versatile carriers for therapeutic agents. (B) Functionalization with ligands such as antibodies, hyaluronic acid, peptides, and folic acid enhances targeting specificity. (C) Passive targeting exploits the enhanced permeability and retention (EPR) effect, wherein nanoparticles accumulate preferentially in tumour tissues due to defective vasculature and impaired lymphatic drainage. (D) Active targeting employs ligand-functionalized nanoparticles that bind to specific receptors (e.g., GPCRs) on cancer cells, facilitating cellular internalization and endosomal uptake, thereby improving therapeutic efficacy (Created with BioRender.com).
Nanoparticle size significantly affects MSN circulation, distribution, tissue accumulation, and cellular uptake. The optimal size for biological use is 10–300 nm, with 100–200 nm particles achieving the highest tumour accumulation via the EPR effect (Petros and DeSimone, 2010; Maeda et al., 2000). Particles of 80 nm show longer residence times, while those larger than 400 nm have poor tumour penetration. Spherical MSNs (80–360 nm) tend to accumulate in the liver and spleen (He Q. et al., 2011). Cellular uptake studies show that 50 nm particles provide the best internalization, though smaller particles may agglomerate and hinder endocytosis (Chithrani et al., 2006).
Nanoparticle shape also influences passive targeting. Spherical MSNs are preferred due to easier fabrication and faster internalization (Gao et al., 2005). Studies show that larger aspect ratios (ARs) enhance cellular uptake and accumulation, with rod-shaped MSNs (AR 2.1–2.5) showing improved tissue penetration (Trewyn et al., 2008). Shorter rods are cleared quickly from the liver, while longer rods accumulate in the spleen. Determining the ideal MSN shape remains challenging due to mixed findings (Huang et al., 2010a; Meng et al., 2011a; Shao et al., 2017).
MSN surface properties affect cell internalization, biodistribution, and circulation (Nel et al., 2009). Negatively charged silanol groups can bind to RBCs, causing hemolysis and reduced targeting, but surface functionalization can mitigate this (Slowing et al., 2009; Teng et al., 2014). PEGylation (with 10,000–20,000 g/mol PEG) reduces protein adsorption and hemolysis (Ke et al., 2017; Cauda et al., 2010; He Q. et al., 2010) while enhancing internalization, though repeated injections may trigger faster clearance due to anti-PEG antibodies. Zwitterionic copolymers and lipid coatings also improve circulation stability (Rosenbrand et al., 2018; Ishida et al., 2003).
Surface charge impacts cell uptake, with positively charged nanocarriers showing better internalization than neutral or negative ones (Yuan et al., 2012). Functionalization with contrast agents (e.g., indocyanine green) or NH2 and PO3 groups enhances drug loading, solubility, and imaging performance (Chaudhary et al., 2019). NH2-modified MSNs have shown improved solubility for lymphoma drugs and enhanced drug delivery under hypoxic conditions in prostate cancer cells (Meka et al., 2018).
Active targeting takes use of the unique interactions between cancer cells and drug-loaded MSNs, demanding surface functionalization with specialized ligands. These ligands, which range from macromolecules and antibodies to nucleic acids, vitamins, sugars, and peptides, allow MSNs to precisely attach to cancer cells by taking advantage of ligand affinity for overexpressed receptors on tumour surfaces (Peer et al., 2007). Active targeting techniques are divided into three categories: tumour cell targeting, vascular targeting, and subcellular organelle targeting, with each providing site-specific delivery to improve treatment effectiveness. Figure 4D describes MSNPs are functionalized with particular ligands that bind to cancer cell-overexpressed receptors (e.g., GPCRs). After binding, MSNPs are internalized into cells via receptor-mediated endocytosis, delivering their payload to intracellular compartments such as endosomes. This dual targeting technique improves the delivery efficiency and therapeutic potential of MSNPs in cancer treatments.
The biodistribution of silica nanoparticles (SNPs) is primarily governed by their physicochemical properties such as size, shape, charge, porosity, and surface functionalization, all of which affect toxicity (Wei et al., 2018; Jasinski et al., 2018). Understanding these parameters is essential for developing successful SNP-based therapeutics. Smaller SNPs (<6 nm) are cleared renally with minimal toxicity, while larger particles (>90 nm) may still appear in urine due to unknown mechanisms (Huang et al., 2011; Lu et al., 2010; Lu et al., 2012). Hepatobiliary clearance is influenced by particle size and surface modifications like PEGylation, which prolongs circulation time, while unshielded cationic particles clear rapidly through the liver (Souris et al., 2010). Aspect ratio also plays a role, with elongated particles exhibiting slower renal clearance than spherical ones, and smaller aspect ratios increasing hepatobiliary clearance rates (Huang et al., 2011; Liu et al., 2016).
The formation of a protein corona mitigates toxicity by increasing surface area and facilitating clearance (Croissant et al., 2018). Dissolution kinetics vary with silica condensation, network connectivity, and surface functionalization, with interrupted networks degrading faster in basic conditions (Möller and Bein, 2019). Ultimately, SNP clearance via renal or hepatobiliary pathways is closely tied to their degradation rate and physicochemical traits.
Preclinical safety evaluation of SNPs necessitates consideration of dose, administration route, and animal models (choice, gender, age) etc. SNPs initially interact with endothelial cells, necessitating toxicity studies in these cells due to their critical role in vascular transport (Cao et al., 2021). Toxicity pathways include mitochondrial ROS generation, MAPK and NF-κB upregulation, and inflammasome activation as shown in Table 4.
Table 4. The summarizes SNP toxicity studies, detailing administration routes, dosages, mechanisms, and adverse effects.
The amount and kind of medicine that may be loaded, as well as the drug’s rate of dissolution, are determined by the pore’s size and shape (Bush et al., 2007). Therefore, in order to avoid the medication releasing too soon, the proper pore size must be employed (Varga et al., 2015; Wang et al., 2015b). The MSN’s meso-scaled channels aid in the storage of non-crystalline medications (Khushalani et al., 1995). They must be investigated in order to regulate the pore size, kind, concentration of templates, and chain length (Maleki et al., 2017; Jana et al., 2004). The pore size rises from 1.6 nm to 4.2 nm with an increase in chain length from C8 to C22 in tetra ammonium salt, according to research by Jana et al. (Widenmeyer and Anwander, 2002).
Additional research has demonstrated that it is feasible to modify the pore size by up to 4.1 nm, which can change the surfactant chain length (Zhang et al., 2008; Xiong et al., 2015). In addition to these variables, pore size is further influenced by catalyst concentration, silica precursor content, and experimental conditions (time and temperature) (Argyo et al., 2014). Mesopores typically have surface areas of 1,000 m2/g, pore volumes of 1–2 cm2/g, and pore diameters of less than 15 nm (NŽK, 2015; Wu et al., 2013).
The size, shape, and surface charge of particles are important in determining in vitro and in vivo drug delivery as MSN with less than 1 µm diameter are much preferred due to their ability for faster mass transport and excellent dispersibility (Khushalani et al., 1995; Jana et al., 2004; Chiang et al., 2011). The pharmacokinetics of MSN are affected by changes in surface charge (Huang et al., 2010b). The cellular interaction, distribution, and elimination are all controlled by the particle size (Varache et al., 2015). The size of the particles can be changed with changes in pH (Yu et al., 2012), reaction temperature (Yokoi et al., 2010), stirring rate (Yamada et al., 2013), type of silica precursor used (Ma et al., 2013), and additives (He et al., 2009) as listed in Table 5. Chiang et al. and Wu et al. showed an increase in the hydrolysis rate and polymerization of silica precursor with increased reaction temperature, leading to larger particle size (Chiang et al., 2011; Yokoi et al., 2010; Wu and Yamauchi, 2012; Ma et al., 2012; Lv et al., 2016). Lv et al. (Sarkar et al., 2016), who worked on TEA, described the effects of base concentration (TEA), reaction temperature, and stirring rates on the size of the particles. It was seen that an increase in temperature (55°C) led to an increase in particle size from 21 nm to 38nm, while the particle size decreased from 51nm to 41 nm when base concentration was increased (0.18g TEA). Now, changing the stirring rates from 100 to 700rpm drastically reduced the particle size from 110nm to 38 nm. However, interestingly, upon further increasing the stirring rate from 700rpm to 1000rpm, there was no further change in size. TEM images given below show the effect of stirring rate, base concentration, and reaction temperature on particle size.
Figure 5 presents diverse applications of mesoporous silica nanoparticles (MSNs) across various fields.
Figure 5. MSNs have diverse applications across multiple fields, contributing to advancements in technology and science.
Lesser density porous carrier such as porous silicon dioxide (Sylysia), polypropylene foam powder (Accurel), porous calcium silicate (Florite), magnesium aluminium metasilicate (Neuslin) and porous ceramic with open or closed pore structure that provides large surface area and can be used for improving the dissolution and bioavailability of poorly soluble drugs like meloxicam, aspirin and indomethacin.
Randy Mellaerts et al. proved that ordered mesoporous silica (OMS) is a promising carrier for achieving enhanced oral bioavailability of drugs with poor aqueous solubility. it presented the effects of spherical MSNs as an oral availability of the model drug TEL and examined their cellular uptake and cytotoxicity (Lu et al., 2010).
The delivery of cisplatin can be controlled, allowing for sustained and targeted delivery. The pore structure of MSNPs enables tunable release kinetics, which can be tailored to match the desired therapeutic profile, thus providing a sustained release of potentially toxic Cisplatin molecules along with a decrease in nonspecific release due to enzymatic hydrolysis. The MSNPs can be loaded with multiple drugs, allowing for combination therapy to target different paths involved in cancer progression. Numerous studies of in vivo toxicity, intake distribution, and clearance were done in animal models (He QJ. et al., 2011; Meng et al., 2011b). Where MSNPs of 100 nm (MC-41 type) gave good biocompatibility in mice for a longer term (He QJ. et al., 2011). MSNPs also deliver Anti-cancer drugs into human xenografts in mice and decrease tumour growth (He QJ. et al., 2011). In a study, modified MSNP using PEI-PEG coating delivered DOX on a KB-31 Xenografted tumour model (Lercher et al., 2006). Table 6 provides an overview of MSNs formulations used in Cancer.
Because MSNPs are a flexible carrier, medications with different characters may be loaded. They have a strong propensity to accumulate within the tumour site because of the enhanced EPR effect (Lercher et al., 2006). Targeting and choosing overexpressed receptors in tumour areas and ligands for those receptors that are conjugated onto the MSN surface allows the medicine to be optimised to reach the precise place. Folic acid (FA) is one such ligand that raises folate levels. In order to provide 5-aminolevulinic acid for photodynamic therapy against B16F 10 skin cancer, Ma et al. (Kleitz et al., 2003) conjugated folic onto the surface of the HMSNs. Hyaluronic acid, or HA, is another well-researched ligand for CD44 receptors. Zhang et al. (Jammaer et al., 2009) reported that DOX-MSN had both receptor-mediated and enzyme-responsive properties.
HA-MSN was synthesised by Gary Bobo et al. (Vialpando et al., 2011) for photodynamic treatment against a colon cancer cell line. The investigation, which used HCT-116 as a model, demonstrated how HA-MSN’s targeting of the CD44 receptor boosted its efficiency over ordinary MSN.
The biosensor in biosensing is composed of an embedded receptor or indicator and a functionalized MSN matrix (Gao et al., 2020). The idea underlying sensing is to identify variations in the optical or electrical signals of different analytes, which are distinguished by their sensitivity to variations in optical detection techniques as RAMAN and UV spectroscopy. This makes it possible to identify several biological targets, including bacteria, viruses, carbohydrates, amino acids, proteins, and glutathione (Gao et al., 2020; Qiu et al., 2017; Huang F. et al., 2020; Guo et al., 2021; Zhu et al., 2021; Tuna et al., 2022; Singh RK. et al., 2017). The MSN matrix increases the receptor’s or indicator’s physiological stability, which raises sensitivity and detection rates. Furthermore, MSN’s structured mesoporous silica structure allows for the creation of particular reaction chambers or promotes interfacial interactions (Gao et al., 2020). Research reports that aptamer-gated aminated MSNs were used for bacterial quantification (Zhu et al., 2021).
NP-based contrast agents have gained importance in the realm of bioimaging, which uses bioimaging for diagnostic reasons (Liu et al., 2023; Lu et al., 2022; Al-Hetty et al., 2023). It is possible to overcome stability and low water solubility difficulties with MSN by adding contrast ants. Targeting ligands applied to the surface of MSN increase the concentration of contrast compounds at specific places (Yu et al., 2018; Sun B. et al., 2021). In magnetic resonance imaging (MRI), Fe, Mn, and Gd-based nanoprobes perform better in contrast because of the porosity nature of MSNPs, which enables water molecules to penetrate the matrix (Yu et al., 2018). MSN enhances photo-bleach resistance and boosts quantum yields for fluorescence imaging (FL) by preventing dye self-aggregation or self-quenching (Sun B. et al., 2021). For PET imaging, radioisotopes like Cu and F must be used to mark molecular probes. When these radioisotopes are added, the MSN matrix lengthens their half-life (Shaffer et al., 2016; Rojas et al., 2015; Chen et al., 2015).
Similar to this, the MSN matrix protects contrast agents in photoacoustic imaging (PA), such as polydopamine (PDA) NP (Cai et al., 2019), Cy754 (Liu Z. et al., 2015), and ICG (Ferrauto et al., 2019) to provide the necessary PA signals. Moreover, multimodal imaging (Grzelak et al., 2022; Huang et al., 2019; Zou et al., 2021), computed tomography (CT) imaging (Chen et al., 2021; Yuan et al., 2021), and ultrasound imaging (Ho et al., 2020; Kempen et al., 2015) also make substantial use of MSNPs.
MSNPs can also be used to transport DNA, RNA, and Nucleic acids in the case of gene therapy as they enable these nucleic acids to bind to the outer surface along with drugs entrapped in the pores. An example of such a method is surface grafting (G2) PAMAM dendrimers (Radu et al., 2004).
MSNPs have also been identified for the use of theranostics applications because of its known biocompatibility (Vivero-Escoto et al., 2010b; Slowing et al., 2008; Chen et al., 2011b; Chen et al., 2011c; Bulatović et al., 2014; Kaluđerović et al., 2010a; García-Peñas et al., 2012; Kaluđerović et al., 2010b; Pérez-Quintanilla et al., 2009; Ceballos-Torres et al., 2014) dual drug targeting. Through functionalization [inorganic structures like Fe3O4 magnetic nanoparticles (Zhu et al., 2010), Gold nanocrystal (Chen et al., 2010), Quantum dots (Kim et al., 2006), etc. Or chemically grafting function groups like PEG (Lu et al., 2010), fluorescent molecules (He QJ. et al., 2010), etc. Of MSN surface, biomedical imaging diagnostic, and therapy for lesion sites for cancer can be produced (Wu H. et al., 2011).
Due of its connections to several neurological conditions, cancers, respiratory disorders, and irregularities related to hormones and reproduction, chemical pesticide usage has sparked worries about public health. The need to produce ecologically friendly pesticides has increased as a result of this problem. Food products that have been stored are mostly stagnant due to the actions of fungus, insects, and rodents under different storage conditions. Crop losses resulting from diseases and insects can range from 5% to 10% in temperate zones and from 50% to 100% in tropical areas, according to estimates (Torney et al., 2007). Honeycomb mesoporous silica nanoparticles (MSNs) with 3-nm pores were created for the first time by Torney, Francois, et al. These MSNs carry genetic material and medications into isolated plant cells and whole leaves by acting as nanocarriers.
Genes and chemical inducers are packed inside the MSNs, and gold nanoparticles cover the ends to stop molecular leaching. Developments in MSNs, such pore enlargement and multi-functionalization, may enable the delivery of drugs, nucleotides, and proteins to specific destinations in plant biotechnology. Pre-treating maize seeds with silica nanoparticles increases the production of organic compounds in the maize seed wall, hence improving resistance to fungal attacks (Suriyaprabha et al., 2012). Additionally, compared to alternative silica-based sources, pre-treated seeds exhibit higher germination rates and improved absorption capacity, resulting in increased nutritional content (Siddiqui and Al-Whaibi, 2014). The use of silica nanoparticles was found to enhance mean germination durations, seed germination indices, and germination rates by Siddiqui and Al-Whaibi (Shi et al., 2013). When 8 g/L of silica nanoparticles were used, germination rates rose by 22.16%. Plants frequently struggle to survive in stressful environments, including those with rising salinities. In order to live, a gene encoding a particular protein that protects against salt has to be introduced.
Silica nanoparticles aid in better oil recovery by reducing the leakage of brine, heavy metals, and radioactive substances into water. In the case of a leak, the impacted water is channeled underground, causing water flooding, which aids oil recovery. However, the usefulness of nanoparticles in high salinity water, where oil extraction takes place, may be hindered, requiring changes to their functioning to assist the extraction process. Experimental experiments have established the efficiency of H+ protection offered to silica nanoparticles in saltwater, with the addition of HCl acting as a shielding mechanism (Youssif et al., 2018). Surface-treated nanoparticles have reversible adsorption properties, enabling them to travel through oil-rich rocks. Additionally, the application of viscosity enhancers eliminates transportation problems. The behavior of silica nanoparticles in sandstone, dolomite, and limestone was investigated (Rognmo et al., 2018). For example, commercial silica nanoparticles of 20 nm in size were employed as the reservoir in sandstone cores. The optimal oil recovery was obtained at a silica nanoparticle concentration of 0.1 weight % (Yousefvand and Jafari, 2015). Silica nanoparticles generate a consistent CO2 foam from oil and stay stable throughout displacement, resulting in greater oil recovery and a lower carbon footprint owing to CO2 (Khan et al., 2014). Incorporating nanoparticles into polymers has also showed potential for alleviating floods and increasing oil recovery. Nano-silicates, for example, may boost the recovery factor by up to 10% owing to the viscosity of the injected fluid (Qu et al., 2006). These discoveries illustrate nanotechnology’s great potential to improve oil recovery techniques.
The contamination of water with heavy metal ions poses serious health and environmental risks, necessitating efficient removal strategies. Industries such as food processing, leather, and textile dyeing release toxic metal ions beyond permissible limits, highlighting the need for cost-effective adsorbents. Mesoporous silica has gained prominence for removing heavy metal ions like chromium (Cr), cadmium (Cd), arsenic (As), lead (Pb), mercury (Hg), copper (Cu), sodium (Na), and cobalt (Co.), as well as organic pollutants from industrial effluents (Jadhav et al., 2016; Savage and Diallo, 2005).
Various techniques, including electrochemical treatment, photodegradation, and chemical coagulation, have been explored for metal ion removal (Ibrahim et al., 2010). Among them, MSN-based adsorption stands out for its efficiency, ease of recovery, and reusability (Jadhav et al., 2018; Jadhav et al., 2019). Research interest in MSN applications for heavy metal removal has significantly increased over the past decade (Karbassian and Ghrib, 2018).
A key advancement is the functionalization of MCM-41-type mesoporous silica with organic groups and polymers, enhancing adsorption efficiency for chromium ions. This review compiles recent MSN-based metal ion removal strategies, summarizing newly synthesized materials and their adsorption capacities. The insights provided will aid future research and development in MSN-based water purification.
Addressing climate change requires more than atmospheric CO2 capture; effective stabilization demands advanced techniques like carbon capture and storage (CCS) and carbon capture and utilization (CCU) (D'Alessandro et al., 2010; Aresta et al., 2014). CCS mitigates emissions from industrial sources by capturing and storing CO2 under high pressure (Tomé and Marrucho, 2016; Tapia et al., 2018). Despite its industrial adoption, high costs and technical challenges limit its commercial viability (Bui et al., 2018). The U.S. leads in CCS deployment (7–8.4 MtCO2/year), followed by China (0.4–2 MtCO2) and Europe (0.7–1 MtCO2) (Wee, 2013; Koh et al., 2019). CCS employs chemical, geological, marine, and terrestrial sequestration but does not convert CO2 into usable products, risking its eventual release. Adsorbent efficiency depends on combustion stages, with meso/macroporous materials suited for pre-combustion and microporous sorbents for post-combustion (Singh G. et al., 2017; Luo et al., 2012).
Conversely, CCU offers a sustainable alternative by converting CO2 into fuels and chemicals, reducing greenhouse gas impact (Kurisingal et al., 2019a; Kurisingal et al., 2019b; Ghiat and Al-Ansari, 2021). More efficient than CCS long-term (Porosoff et al., 2016), CCU repurposes CO2 into hydrocarbons, methanol, and formic acid, minimizing fossil fuel reliance (Wang X. et al., 2019; Calvinho et al., 2018; Branco et al., 2016; Donphai et al., 2016; Wang WH. et al., 2015; Han et al., 2019; Fujiwara et al., 2015; Owen et al., 2016). Conversion methods include hydrogenation, cycloaddition, photochemical reduction, oxidative dehydrogenation, and electrochemical reduction (Dai et al., 2016; Ding et al., 2020; Jeyalakshmi et al., 2013; Kiatphuengporn et al., 2016). Despite CO2’s stability requiring high energy input, industries use ∼3,600 million tons annually. Future policies, like carbon taxation, could enhance CO2 utilization (Parvanian et al., 2022), necessitating novel conversion technologies.
Heterogeneous catalysts such as metal–organic frameworks (MOFs), transition-metal complexes, porous organic polymers, covalent organic frameworks (COFs), zeolites, porous carbon, and mesoporous silica nanoparticles (MSNs) have shown superior efficiency in CO2 utilization (Mohan et al., 2020a; Mohan et al., 2020b; Gui et al., 2020; Kurisingal et al., 2020; Roy et al., 2019). MSNs, with tunable silanol groups, high surface areas (∼2,370 m2 g-1), and large pore volumes (∼1.4 cm3 g-1), enable enhanced metal dispersion, crucial for catalysis (Mohan et al., 2021a; Mohan et al., 2021b; Cashin et al., 2018).
Clinical trials involving the potential use of Mesoporous silica are being done from 2007. As of now clinical trials have showed safety and good tolerability of silica in humans. MSNs oral delivery was established in 2014 for the purpose of enhancing pharmacokinetics profile of poor water-soluble drugs (Qu et al., 2006).
Silica nanoparticles with lipid hybrid formulation was loaded with Ibuprofen (Lipo-ceramic IBU). Randomised, double blind, single dose oral study of 20 mg Ibuprofen was practiced on 16 healthy male volunteers., and the bioavailability of lipo-ceramic-IBU was found to be 1.95 times greater than commercial nurofen tablet (Tan et al., 2014).
Similarly in another clinical trial conducted in 2016, 12 subjects were selected and resulted in an increased bioavailability of ordered MSNs oral dose compared to Lipanthyl commercially (Bukara et al., 2016).
Due to positive clinical outcomes of silica based nanocarriers the safety, pharmacokinetics and metabolic profile of Cornell dots or C dots (Hybrid core shell silica nanoparticles) of diameter 6 nm functionalized with 124I targeting peptide cyclo- (Arg-Gly-Tyr) and cy5 (124I-cRGDY-PEG-C dots) was first conducted in humans using PET imaging and clinical tracer in 2014 at clinical trials (NCT01266096) (Phillips et al., 2014b). Good safety and reproducible PK signatures of C dots with whole body clearance half time of 13–21 h s was witnessed after iv injection which is shorter than 111In labelled liposomes and 131I labelled humanised monoclonal antibody A33. These results led to the use of Nanoparticles in human cancer (Phillips et al., 2014b).
In another study, 8 nm shell silica nanoparticles were encapsulated with cy 5.5 and connected with cRGDY on the surface, (cRGDY-PEG-cy 5.5- Nanoparticles)., nodes detection of head and neck melanoma at phase one/2 clinical trials (Zanoni et al., 2021) and phase two trials are expected to finish in 2024 (NCT 02106598).
Another two clinical trials were also conducted on C dots. In 2018, the 89Zr-DFo-cRGDY-PEG-Cy5-C dot traces were used in both surgical and non-surgical patients for PET-CT imaging of malignant brain tumour in phase one clinical trials (NCT03465618). In 2023, distribution and removal profiles of these particles were characterized.
In 2019, 64cu-NOTA-PSMA-PEG-Cy 5.5-C′ dot tracer was used in another human phase one clinical trial for prostate cancer by PET and MRI imaging (NCT04167969) (Xu et al., 2023).
The rapid advancements in nanotechnology, particularly in the development of mesoporous silica nanoparticles (MSNs), have demonstrated their significant potential in therapeutic and diagnostic applications. The recent clinical approvals of lipid-nanoparticle-based mRNA vaccines have reinforced the promise of nanomaterials in medicine, yet the clinical translation of nanoparticles, including silica-based platforms, remains largely limited. Despite preclinical evidence supporting their efficacy, only a handful of MSN formulations have progressed to human trials. Notably, plasmonic resonance therapy using ferromagnetic core-shell silica nanoparticles has exhibited superior outcomes in coronary atherosclerosis treatment compared to conventional interventions (NCT01270139) (Kharlamov et al., 2017). Furthermore, AuroLase® therapy utilizing gold-shell silica-core nanoparticles has achieved remarkable tumour ablation efficacy in prostate and head and neck cancers (NCT04240639, NCT02680535, NCT04656678, NCT00848042) with minimal systemic toxicity (Rastinehad et al., 2019). Similarly, Cornell dots, a class of ultra-small silica nanoparticles, have demonstrated their safety and efficacy in cancer imaging and sentinel lymph node detection in clinical settings (NCT01266096, NCT02106598) (Phillips et al., 2014c; Zanoni et al., 2021). These pioneering studies underscore the clinical viability of MSNs, yet their widespread adoption necessitates further advancements in overcoming biological barriers and addressing toxicity concerns.
The primary impediments to the clinical translation of MSNs involve their biocompatibility, biodistribution, and potential toxicity. The physicochemical properties, including size, surface functionalization, porosity, and degradation kinetics, critically influence their pharmacokinetic behaviour and toxicity profiles. Reports of silica nanoparticle-induced pathologic lesions, coagulation disturbances, and inflammatory responses in vital organs such as the liver, heart, lungs, and spleen highlight the necessity of optimizing these parameters to mitigate adverse effects. Strategies such as surface functionalization with polyethylene glycol (PEG) and folic acid have demonstrated promise in minimizing systemic toxicity by reducing off-target accumulation. Additionally, the enhanced biodegradability of mesoporous silica compared to its nonporous counterparts suggests a favourable safety profile, yet inconsistencies in in vivo toxicity studies necessitate standardized evaluation methodologies. The divergent findings on MSN toxicity stem from variations in nanoparticle physicochemical properties, dosing regimens, and administration routes, underscoring the need for more comprehensive toxicity assessments that closely mimic clinical conditions (Hargrove et al., 2020; Di Pasqua et al., 2013).
Although MSNs hold immense promise for targeted drug delivery, the transition from preclinical models to clinical applications remains a formidable challenge. The majority of existing studies are confined to in vitro investigations, which do not fully recapitulate the complexities of in vivo biological environments. Limited tissue penetration and the lack of robust data on MSN clearance mechanisms further complicate their clinical translation. Addressing these gaps requires extensive in vivo studies to elucidate the biodistribution, accumulation, and elimination pathways of MSNs, ensuring their long-term safety and efficacy. Moreover, the development of more sophisticated MSN-based drug delivery systems, integrating stimuli-responsive and targeted therapeutic strategies, represents a crucial avenue for enhancing clinical applicability.
In conclusion, mesoporous silica nanoparticles offer a transformative approach to cancer therapy and drug delivery, yet significant challenges remain their clinical adoption. This review underscores the recent advancements in MSN functionalization, stimuli-triggered drug release, and targeted delivery strategies. While the preclinical landscape is promising, prospective investigations focusing on biocompatibility, pharmacokinetics, and toxicity mitigation are imperative to facilitate the transition of MSN-based nanocarriers from laboratory research to clinical practice. Future efforts should prioritize refining MSN designs to enhance their therapeutic efficacy while ensuring safety, ultimately paving the way for their integration into mainstream medical applications.
The limitations of conventional therapies underscore an urgent need for next-generation treatment strategies with superior efficacy and precision. Mesoporous silica nanoparticles (MSNs) have emerged as a groundbreaking platform for drug delivery, offering tunable physicochemical properties—such as pore architecture, morphology, and surface functionalization—that enable precise control over drug loading and release. This review comprehensively examines the advancements in MSN-based drug carriers, highlighting their potential across diverse biomedical applications, including targeted cancer therapy, gene delivery, theranostics, and environmental remediation. The intricate relationship between MSN design and therapeutic performance underscores the necessity of tailored nanostructures for specific medical applications.
Despite significant progress in optimizing MSNs for controlled drug delivery, their clinical translation remains a formidable challenge. While extensive in vitro studies have demonstrated promising results, the transition to in vivo applications is hindered by unresolved issues such as tissue penetration, systemic distribution, and long-term biocompatibility. Current research predominantly relies on in vitro models that fail to accurately replicate the complexities of the human physiological environment. Moreover, a critical gap in the existing literature is the limited discussion on the biodistribution, metabolism, and clearance pathways of MSNs, raising concerns about their potential accumulation and toxicity in biological systems. Addressing these uncertainties necessitates extensive preclinical in vivo studies to elucidate MSN processing, retention, and elimination within living organisms.
Future research should prioritize refining MSN synthesis, enhancing biocompatibility, and establishing scalable, reproducible manufacturing techniques to facilitate clinical adoption. Additionally, interdisciplinary collaborations integrating cutting-edge nanotechnology, advanced imaging modalities, and pharmacokinetic modeling will be pivotal in overcoming translational barriers. By addressing these challenges, MSNs hold the potential to revolutionize drug delivery, optimize therapeutic efficacy, minimize adverse effects, and advance the era of precision medicine.
RF: Writing–original draft, Writing–review and editing. PK: Writing–review and editing. KK: Supervision, Writing–review and editing.
The author(s) declare that no financial support was received for the research, authorship, and/or publication of this article. This research received no specific grant from any funding agency in the public, commercial, or not-for-profit sectors.
I would like to express my deep gratitude to my guide, Kalpana, for her valuable guidance and support throughout this research. Her expertise and encouragement have been instrumental in the completion of this manuscript.
The authors declare that the research was conducted in the absence of any commercial or financial relationships that could be construed as a potential conflict of interest.
The authors declare that no Generative AI was used in the creation of this manuscript.
All claims expressed in this article are solely those of the authors and do not necessarily represent those of their affiliated organizations, or those of the publisher, the editors and the reviewers. Any product that may be evaluated in this article, or claim that may be made by its manufacturer, is not guaranteed or endorsed by the publisher.
Abd-elbary, A., El Nabarawi, M. A., Hassen, D. H., and Taha, A. A. (2014). Inclusion and characterization of ketoprofen into different mesoporous silica nanoparticles using three loading methods. Int. J. Pharm. Pharm. Sci. 6, 183–191.
Ahern, R. J., Crean, A. M., and Ryan, K. B. (2012). The influence of supercritical carbon dioxide (SC-CO2) processing conditions on drug loading and physicochemical properties. Int. J. Pharm. 439, 92–99. doi:10.1016/j.ijpharm.2012.09.047
Alhariri, M., Azghani, A., and Omri, A. (2013). Liposomal antibiotics for the treatment of infectious diseases. Expert Opin. Drug Deliv. 10 (10), 1515–1532. doi:10.1517/17425247.2013.822860
Al-Hetty, HRAK, Jalil, A. T., Al-Tamimi, J. H. Z., Shakier, H. G., Kandeel, M., Saleh, M. M., et al. (2023). Engineering and surface modification of carbon quantum dots for cancer bioimaging. Inorg. Chem. Commun. 149, 110433. doi:10.1016/j.inoche.2023.110433
Ambrogi, V., Marmottini, F., and Pagano, C. (2013). Amorphous carbamazepine stabilization by the mesoporous silicate SBA-15. Micropor Mesopor Mater 177, 1–7. doi:10.1016/j.micromeso.2013.04.008
Ambrogi, V., Perioli, L., Marmottini, F., Accorsi, O., Pagano, C., Ricci, M., et al. (2008). Role of mesoporous silicates on carbamazepine dissolution rate enhancement. Micropor Mesopor Mater 113, 445–452. doi:10.1016/j.micromeso.2007.12.003
Ambrogi, V., Perioli, L., Marmottini, F., Giovagnoli, S., Esposito, M., and Rossi, C. (2007). Improvement of dissolution rate of piroxicam by inclusion into MCM-41 mesoporous silicate. Eur. J. Pharm. Sci. 32, 216–222. doi:10.1016/j.ejps.2007.07.005
Ambrogi, V., Perioli, L., Pagano, C., Latterini, L., Marmottini, F., Ricci, M., et al. (2012a). MCM-41 for furosemide dissolution improvement. Micropor Mesopor Mater 147, 343–349. doi:10.1016/j.micromeso.2011.07.007
Ambrogi, V., Perioli, L., Pagano, C., Marmottini, F., Moretti, M., Mizzi, F., et al. (2010). Econazole nitrate-loaded MCM-41 for an antifungal topical powder formulation. J. Pharm. Sci. 99, 4738–4745. doi:10.1002/jps.22183
Ambrogi, V., Perioli, L., Pagano, C., Marmottini, F., Ricci, M., Sagnella, A., et al. (2012b). Use of SBA-15 for furosemide oral delivery enhancement. Eur. J. Pharm. Sci. 46, 43–48. doi:10.1016/j.ejps.2012.02.004
Ambrogio, M. W., Pecorelli, T. A., Patel, K., Khashab, N. M., Trabolsi, A., Khatib, H. A., et al. (2010). Snap-top nanocarriers. Org. Lett. 12, 3304–3307. doi:10.1021/ol101286a
Andersson, J., Rosenholm, J., Areva, S., and Lindén, M. (2004). Influences of material characteristics on ibuprofen drug loading and release profiles from ordered micro- and mesoporous silica matrices. Chem. Mat. 16, 4160–4167. doi:10.1021/cm0401490
Andrade, G. F., Soares, D. C. F., Almeida, R. K. S., and Sousa, E. M. B. (2012). Mesoporous silica SBA-16 functionalized with alkoxysilane groups: preparation, characterization, and release profile study. J. Nanomater 2012, 1–10. doi:10.1155/2012/816496
Angelos, S., Choi, E., Vogtle, F., De Cola, L., and Zink, J. I. (2007). Photo-Driven expulsion of molecules from mesostructured silica nanoparticles. J. Phys. Chem. C 111, 6589–6592. doi:10.1021/jp070721l
Aresta, M., Dibenedetto, A., and Angelini, A. (2014). Catalysis for the valorization of exhaust carbon: from CO2 to chemicals, materials, and fuels. Technological use of CO2. Chem. Rev. 114, 1709–1742. doi:10.1021/cr4002758
Argyo, C., Weiss, V., Bräuchle, C., and Bein, T. (2014). Multifunctional mesoporous silica nanoparticles as a universal platform for drug delivery. Chem. Mater 26, 435–451. doi:10.1021/cm402592t
Baeza, A., Guisasola, E., Ruiz-Hernández, E., and Vallet-Regí, M. (2012). Magnetically triggered multidrug release by hybrid mesoporous silica nanoparticles. Chem. Mater 24 (3), 517–524. doi:10.1021/cm203000u
Bernardos, A., Aznar, E., Marcos, M. D., Martínez-Máñez, R., Sancenón, F., Soto, J., et al. (2009). Enzyme-responsive controlled release using mesoporous silica supports capped with lactose. Angew. Chem. Int. Ed. Engl. 48 (32), 5884–5887. doi:10.1002/anie.200900880
Bernardos, A., Mondragon, L., Aznar, E., Marcos, M. D., Martínez-Mañez, R., Sancenon, F., et al. (2010). Enzyme-responsive intracellular controlled release using nanometric silica mesoporous supports capped with “saccharides.”. ACS Nano 4 (11), 6353–6368. doi:10.1021/nn101499d
Bharti, C., Nagaich, U., Pal, A. K., and Gulati, N. (2015). Mesoporous silica nanoparticles in target drug delivery system: a review. Int. J. Pharm. Investig. 5, 124–133. doi:10.4103/2230-973x.160844
Bouledjouidja, A., Masmoudi, Y., Van Speybroeck, M., Schueller, L., and Badens, E. (2016). Impregnation of fenofibrate on mesoporous silica using supercritical carbon dioxide. Int. J. Pharm. 499, 1–9. doi:10.1016/j.ijpharm.2015.12.049
Branco, J. B., Ferreira, A. C., Gonçalves, A. P., Soares, C. O., and Gasche, T. A. (2016). Synthesis of methanol using copper–f block element bimetallic oxides as catalysts and greenhouse gases (CO2, CH4) as feedstock. J. Catal. 341, 24–32. doi:10.1016/j.jcat.2016.06.007
Brás, A. R., Fonseca, I. M., Dionísio, M., Schönhals, A., Affouard, F., and Correia, N. T. (2014). Influence of nanoscale confinement on the molecular mobility of ibuprofen. J. Phys. Chem. C 118, 13857–13868. doi:10.1021/jp500630m
Brinker, C. J., Carnes, E. C., Ashley, C. E., and Willman, C. L. (2017). Porous nanoparticle-supported lipid bilayers (protocells) for targeted delivery and methods of using same. U. S. Pat. US20150272885A1.
Brinker, C. J., and Scherer, G. W. (1990). Sol-gel science: the physics and Chemistry of sol-gel processing. San Diego: Academic Press.
Bui, M., Adjiman, C. S., Bardow, A., Anthony, E. J., Boston, A., Brown, S., et al. (2018). Carbon capture and storage (CCS): the way forward. Energy Environ. Sci. 11, 1062–1176. doi:10.1039/c7ee02342a
Bukara, K., Schueller, L., Rosier, J., Martens, M. A., Daems, T., Verheyden, L., et al. (2016). Ordered mesoporous silica to enhance the bioavailability of poorly water-soluble drugs: proof of concept in man. Eur. J. Pharm. Biopharm. 108, 220–225. doi:10.1016/j.ejpb.2016.08.020
Bulatović, M. Z., Maksimović-Ivanić, D., Bensing, C., Gómez-Ruiz, S., Steinborn, D., Schmidt, H., et al. (2014). Organotin(IV)-Loaded mesoporous silica as a biocompatible strategy in cancer treatment. Angew. Chem. Int. Ed. Engl. 53, 5982–5987. doi:10.1002/anie.201400763
Bush, J. R., Akgerman, A., and Hall, K. R. (2007). Synthesis of controlled release device with supercritical CO2 and co-solvent. J. Supercrit. Fluids 41, 311–316. doi:10.1016/j.supflu.2006.09.008
Buyuktimkin, T., and Wurster, D. E. (2015). The influence of the adsorption of metoclopramide on the surface ionization of fumed silica. Int. J. Pharm. 478, 164–171. doi:10.1016/j.ijpharm.2014.11.028
Cai, C., Li, X., Wang, Y., Liu, M., Shi, X., Xia, J., et al. (2019). Polydopamine-coated gold core/hollow mesoporous silica shell particles as a nanoplatform for multimode imaging and photothermal therapy of tumors. Chem. Eng. J. 362, 842–850. doi:10.1016/j.cej.2019.01.072
Cai, Q., Luo, Z. S., Pang, W. Q., Fan, Y. W., Chen, X. H., and Cui, F. Z. (2001). Dilute solution routes to various controllable morphologies of MCM-41 silica with a basic medium. Chem. Mater 13, 258–263. doi:10.1021/cm990661z
Calvinho, K. U. D., Laursen, A. B., Yap, K. M. K., Goetjen, T. A., Hwang, S., Murali, N., et al. (2018). Selective CO2 reduction to C3 and C4 oxyhydrocarbons on nickel phosphides at overpotentials as low as 10 mV. Energy Environ. Sci. 11, 2550–2559. doi:10.1039/c8ee00936h
Campanati, M., Fornasari, G., and Vaccari, A. (2003). Fundamentals in the preparation of heterogeneous catalysts. Catal. Today 77, 299–314. doi:10.1016/s0920-5861(02)00375-9
Cao, Y., Li, S., and Chen, J. (2021). Modeling better in vitro models for the prediction of nanoparticle toxicity: a review. Toxicol. Mech. Methods 31 (1), 1–17. doi:10.1080/15376516.2020.1828521
Cao, Y., Wei, H., and Xia, Z. (2009). Advances in microwave-assisted synthesis of ordered mesoporous materials. Trans. Nonferrous Met. Soc. China 19, s656–s664. doi:10.1016/s1003-6326(10)60127-6
Carvalho, G. C., Sábio, R. M., de Cássia Ribeiro, T., Monteiro, A. S., Pereira, D. V., Ribeiro, S. J. L., et al. (2020). Highlights in mesoporous silica nanoparticles as a multifunctional controlled drug delivery nanoplatform for infectious diseases treatment. Pharm. Res. 37, 191. doi:10.1007/s11095-020-02917-6
Cashin, V. B., Eldridge, D. S., Yu, A., and Zhao, D. (2018). Surface functionalization and manipulation of mesoporous silica adsorbents for improved removal of pollutants: a review. Environ. Sci. Water Res. Technol. 4, 110–128. doi:10.1039/c7ew00322f
Cauda, V., Argyo, C., and Bein, T. (2010). Impact of different PEGylation patterns on the long-term bio-stability of colloidal mesoporous silica nanoparticles. J. Mater Chem. 20 (39), 8693–8699. doi:10.1039/c0jm01390k
Ceballos-Torres, J., Virag, P., Cenariu, M., Prashar, S., Fajardo, M., Fischer-Fodor, E., et al. (2014). Anti-cancer applications of titanocene-functionalised nanostructured systems: an insight into cell death mechanisms. Chem. Eur. J. 20, 10811–10828. doi:10.1002/chem.201400300
Charnay, C., Bégu, S., Tourné-Péteilh, C., Nicole, L., Lerner, D. A., and Devoisselle, J. M. (2004). Inclusion of ibuprofen in mesoporous templated silica: drug loading and release property. Eur. J. Pharm. Biopharm. 57, 533–540. doi:10.1016/j.ejpb.2003.12.007
Chaudhary, Z., Khan, G. M., Abeer, M. M., Pujara, N., Tse, B. W. C., McGuckin, M. A., et al. (2019). Efficient photoacoustic imaging using indocyanine green (ICG) loaded functionalized mesoporous silica nanoparticles. Biomater. Sci. 7 (12), 5002–5015. doi:10.1039/c9bm00822e
Chen, B., Wang, Z., Quan, G., Peng, X., Pan, X., Wang, R., et al. (2012). In vitro and in vivo evaluation of ordered mesoporous silica as a novel adsorbent in liquisolid formulation. Int. J. Nanomed 7, 199–209. doi:10.2147/IJN.S26763
Chen, F., Hong, H., Goel, S., Graves, S. A., Orbay, H., Ehlerding, E. B., et al. (2015). In vivo tumor vasculature targeting of CuS@MSN based theranostic nanomedicine. ACS Nano 9, 3926–3934. doi:10.1021/nn507241v
Chen, J., Liu, M., Chen, C., Gong, H., and Gao, C. (2011a). Synthesis and characterization of silica nanoparticles with well-defined thermoresponsive PNIPAM via a combination of RAFT and click chemistry. ACS Appl. Mater Interfaces 3 (8), 3215–3223. doi:10.1021/am2007189
Chen, L., Di, J., Cao, C., Zhao, Y., Ma, Y., Luo, J. Y., et al. (2011b). A pH-driven DNA nanoswitch for responsive controlled release. Chem. Commun. 47, 2850–2852. doi:10.1039/c0cc04765a
Chen, L., Wen, Y., Su, B., Di, J., Song, Y., and Jiang, L. (2011c). Programmable DNA switch for bioresponsive controlled release. J. Mater Chem. 21, 13811–13816. doi:10.1039/c1jm12203g
Chen, L., Xu, J., Wang, Y., and Huang, R. (2021). Ultra-small MoS2 nanodots-incorporated mesoporous silica nanospheres for pH-sensitive drug delivery and CT imaging. J. Mater Sci. Technol. 63, 91–96. doi:10.1016/j.jmst.2020.03.019
Chen, Y., Chen, H. R., Guo, L. M., He, Q. J., Chen, F., Zhou, J., et al. (2010). Hollow/rattle-type mesoporous nanostructures by a structural difference-based selective etching strategy. ACS Nano 4, 529–539. doi:10.1021/nn901398j
Cheng, Y. J., Luo, G. F., Zhu, J. Y., Xu, X. D., Zeng, X., Cheng, D. B., et al. (2015). Enzyme-induced and tumor-targeted drug delivery system based on multifunctional mesoporous silica nanoparticles. ACS Appl. Mater Interfaces 7 (17), 9078–9087. doi:10.1021/acsami.5b00752
Chew, T. L., Ahmad, A. L., and Bhatia, S. (2010). Ordered mesoporous silica (OMS) as an adsorbent and membrane for separation of carbon dioxide (CO2). Adv. Colloid Interface Sci. 153 (1–2), 43–57. doi:10.1016/j.cis.2009.12.001
Chiang, Y. D., Lian, H. Y., Leo, S. Y., Wang, S. G., Yamauchi, Y., and Wu, K. C. W. (2011). Controlling particle size and structural properties of mesoporous silica nanoparticles using the Taguchi method. J. Phys. Chem. C 115, 13158–13165. doi:10.1021/jp201017e
Chircov, C., Spoiala, A., Paun, C., Craciun, L., Ficai, D., Ficai, A., et al. (2020). Mesoporous silica platforms with potential applications in release and adsorption of active agents. Molecules 25, 3814. doi:10.3390/molecules25173814
Chithrani, B. D., Ghazani, A. A., and Chan, W. C. W. (2006). Determining the size and shape dependence of gold nanoparticle uptake into mammalian cells. Nano Lett. 6 (4), 662–668. doi:10.1021/nl052396o
Chou, C. C., Chen, W., Hung, Y., and Mou, C. Y. (2017). Molecular elucidation of biological response to mesoporous silica nanoparticles in vitro and in vivo. ACS Appl. Mater Interfaces 9 (27), 22235–22251. doi:10.1021/acsami.7b05359
Coti, K. K., Belowich, M. E., Liong, M., Ambrogio, M. W., Lau, Y. A., Khatib, H. A., et al. (2009). Mechanised nanoparticles for drug delivery. Nanoscale 1, 16–39. doi:10.1039/b9nr00162j
Croissant, J. G., and Brinker, C. J. (2018). “Biodegradable silica-based nanoparticles: dissolution kinetics and selective bond cleavage,”. The enzymes. Editor F. Tamanoi (Academic Press), 43, 181–214. doi:10.1016/bs.enz.2018.07.008
Dai, W., Zhang, Y., Tan, Y., Luo, X., and Tu, X. (2016). Reusable and efficient polymer nanoparticles grafted with hydroxyl-functionalized phosphonium-based ionic liquid catalyst for cycloaddition of CO2 with epoxides. Appl. Catal. A 514, 43–50. doi:10.1016/j.apcata.2016.01.004
D'Alessandro, D. M., Smit, B., and Long, J. R. (2010). Carbon dioxide capture: prospects for new materials. Angew. Chem. Int. Ed. 49, 6058–6082. doi:10.1002/anie.201000431
Davis, B., Richens, J., and O’Shea, P. (2011). Label-free critical micelle concentration determination of bacterial quorum sensing molecules. Biophys. J. 101, 245–254. doi:10.1016/j.bpj.2011.05.033
Dementeva, O. V., Roumyantseva, T. B., and Rudoy, V. M. (2016). The first example of silica nanoshell synthesis on vesicles of a cationic glycerolipid—potential antitumor drug. Colloid J. 78, 281–284. doi:10.1134/s1061933x16020034
Dementeva, O. V., and Rudoy, V. M. (2016). One-pot synthesis and loading of mesoporous SiO2 nanocontainers using micellar drugs as a template. RSC Adv. 6, 36207–36210. doi:10.1039/c6ra03602c
Dementeva, O. V., Rudoy, V. M., and Zhigletsova, S. K. (2018). One-pot synthesis and loading of silica nanocontainers using surface-active drugs as templating agents. Int. J. Drug Dev. Res. 10, C1–C002.
Deraz, N. (2017). The comparative jurisprudence of catalysts preparation methods: I. Precipitation and impregnation methods. J. Ind. Environ. Chem. 2, 19–21.
Deshpande, P., Biswas, S., and Torchilin, V. P. (2013). Current trends in the use of liposomes for tumor targeting. Nanomedicine (Lond). 8 (9), 1509–1528. doi:10.2217/nnm.13.118
Díaz de Greñu, B., Reyes, R., Costero, A. M., Ros-Lis, J. V., Amorós, P., and Ros-Lis, J. V. (2020). Recent progress of microwave-assisted synthesis of silica materials. Nanomater. (Basel) 10, 1092. doi:10.3390/nano10061092
Ding, C., and Li, Z. (2017). A review of drug release mechanisms from nanocarrier systems. Mater Sci. Eng. C 76, 1440–1453. doi:10.1016/j.msec.2017.03.130
Ding, H., Mal, A., and Wang, C. (2020). Tailored covalent organic frameworks by post-synthetic modification. Mater Chem. Front. 4, 113–127. doi:10.1039/c9qm00555b
Di Pasqua, A. J., Yuan, H., Chung, Y., Kim, J. K., Huckle, J. E., Li, C., et al. (2013). Neutron-activatable holmium-containing mesoporous silica nanoparticles as a potential radionuclide therapeutic agent for ovarian cancer. J. Nucl. Med. 54 (1), 111–116. doi:10.2967/jnumed.112.106609
Doadrio, J. C., Sousa, E. M. B., Izquierdo-Barba, I., Doadrio, A. L., Perez-Pariente, J., and Vallet-Regí, M. (2006). Functionalization of mesoporous materials with long alkyl chains as a strategy for controlling drug delivery pattern. J. Mater Chem. 16, 462–466. doi:10.1039/b510101h
Donphai, W., Piriyawate, N., Witoon, T., Jantaratana, P., Varabuntoonvit, V., and Chareonpanich, M. (2016). Effect of magnetic field on CO2 conversion over Cu-ZnO/ZrO2 catalyst in hydrogenation reaction. J. CO2 Util. 16, 204–211. doi:10.1016/j.jcou.2016.07.007
Ebner, C., Bodner, T., Stelzer, F., and Wiesbrock, F. (2011). One decade of microwave-assisted polymerizations: quo vadis? Macromol. Rapid Commun. 32, 254–288. doi:10.1002/marc.201000539
Eren, Z. S., Tunçer, S., Gezer, G., Yildirim, L. T., Banerjee, S., and Yilmaz, A. (2016). Improved solubility of celecoxib by inclusion in SBA-15 mesoporous silica: drug loading in different solvents and release. Micropor Mesopor Mater 235, 211–223. doi:10.1016/j.micromeso.2016.08.014
Fasiku, V., Amuhaya, E. K., Rajab, K. M., and Omolo, C. A. (2019). “Nano/microparticles encapsulation via covalent drug conjugation,” in Nano- and micro-encapsulation—techniques and applications. Editor N. Abu-Thabit (London: IntechOpen), 230–251.
Ferrauto, G., Carniato, F., Di Gregorio, E., Botta, M., and Tei, L. (2019). Photoacoustic ratiometric assessment of mitoxantrone release from theranostic ICG-conjugated mesoporous silica nanoparticles. Nanoscale 11, 18031–18036. doi:10.1039/c9nr06524e
Fu, Q., Rao, G. V., Ista, L. K., Wu, Y., Andrzejewski, B. P., Sklar, L. A., et al. (2003). Control of molecular transport through stimuli-responsive ordered mesoporous materials. Adv. Mater 15, 1262–1266. doi:10.1002/adma.200305165
Fu, T., Guo, L., Kang, L., Wang, T., and Li, J. (2010). Template occluded SBA-15: an effective dissolution enhancer for poorly water-soluble drug. Appl. Surf. Sci. 256, 6963–6968. doi:10.1016/j.apsusc.2010.05.007
Fujiwara, M., Satake, T., Shiokawa, K., and Sakurai, H. (2015). CO2 hydrogenation for C2+ hydrocarbon synthesis over composite catalyst using surface-modified HB zeolite. Appl. Catal. B 179, 37–43. doi:10.1016/j.apcatb.2015.05.004
Fuller, E. G., Sun, H., Dhavalikar, R. D., Unni, M., Scheutz, G. M., Sumerlin, B. S., et al. (2019). Externally triggered heat and drug release from magnetically controlled nanocarriers. ACS Appl. Polym. Mater 1 (2), 211–220. doi:10.1021/acsapm.8b00100
Gad, A., Kydd, J., Piel, B., and Rai, P. (2016). Targeting cancer using polymeric nanoparticle-mediated combination chemotherapy. Int. J. Nanomed Nanosurg 2. doi:10.16966/2470-3206.116
Gao, H. J., Shi, W. D., and Freund, L. B. (2005). Mechanics of receptor-mediated endocytosis. Proc. Natl. Acad. Sci. U. S. A. 102, 9469–9474. doi:10.1073/pnas.0503879102
Gao, M., Zeng, J., Liang, K., Zhao, D., and Kong, B. (2020). Mesoporous silica materials: interfacial assembly of mesoporous silica-based optical heterostructures for sensing applications (adv. Funct. Mater. 9/2020). Adv. Funct. Mater 30, 1906950. doi:10.1002/adfm.202070057
Gao, Q., Xu, Y., Wu, D., Sun, Y., and Li, X. (2009). pH-responsive drug release from polymer-coated mesoporous silica spheres. J. Phys. Chem. C 113 (29), 12753–12758. doi:10.1021/jp9043978
García-Peñas, A., Gómez-Ruiz, S., Pérez-Quintanilla, D., Paschke, R., Sierra, I., Prashar, S., et al. (2012). Study of the cytotoxicity and particle action in human cancer cells of titanocene-functionalized materials with potential application against tumors. J. Inorg. Biochem. 106, 100–110. doi:10.1016/j.jinorgbio.2011.09.033
Ghiat, I., and Al-Ansari, T. (2021). A review of carbon capture and utilisation as a CO2 abatement opportunity within the EWF nexus. J. CO2 Util. 45, 101432. doi:10.1016/j.jcou.2020.101432
Giret, S., Wong Chi Man, M., and Carcel, C. (2015). Mesoporous-silica-functionalized nanoparticles for drug delivery. Chem. Eur. J. 21, 13850–13865. doi:10.1002/chem.201500578
Gong, T., Xie, J., Liao, J., Zhang, T., Lin, S., and Lin, Y. (2015). Nanomaterials and bone regeneration. Bone Res. 3 (15029), 15029. doi:10.1038/boneres.2015.29
Grün, M., Lauer, I., and Unger, K. K. (1997). The synthesis of micrometer- and submicrometer-size spheres of ordered mesoporous oxide MCM-41. Adv. Mat. 9, 254–257. doi:10.1002/adma.19970090317
Grzelak, J., Gázquez, J., Grayston, A., Teles, M., Herranz, F., Roher, N., et al. (2022). Magnetic mesoporous silica nanorods loaded with ceria and functionalized with fluorophores for multimodal imaging. ACS Appl. Nano Mater 5, 2113–2125. doi:10.1021/acsanm.1c03837
Gui, B., Lin, G., Ding, H., Gao, C., Mal, A., and Wang, C. (2020). Three-dimensional covalent organic frameworks: from topology design to applications. Acc. Chem. Res. 53, 2225–2234. doi:10.1021/acs.accounts.0c00357
Guisasola, E., Baeza, A., Talelli, M., Arcos, D., Moros, M., de la Fuente, J. M., et al. (2015). Magnetic-responsive release controlled by hot spot effect. Langmuir 31 (46), 12777–12782. doi:10.1021/acs.langmuir.5b03470
Gunaydin, S., and Yılmaz, A. (2015). Improvement of solubility of celecoxib by inclusion in MCM-41 mesoporous silica: drug loading and release. Turk J. Chem. 39, 317–333. doi:10.3906/kim-1409-56
Guo, J., Chen, S., Tian, S., Liu, K., and Ma, X. (2021). A sensitive and quantitative prognosis of C-reactive protein at picogram level using mesoporous silica encapsulated core-shell up-conversion nanoparticle-based lateral flow strip assay. Talanta 230, 122335. doi:10.1016/j.talanta.2021.122335
Guo, Y., Jian, X., Zhang, L., Mu, C., Yin, L., Xie, J., et al. (2020). Plasma-induced FeSiAl@Al2O3@SiO2 core–shell structure for exceptional microwave absorption and anti-oxidation at high temperature. Chem. Eng. J. 384, 123371. doi:10.1016/j.cej.2019.123371
Guo, Z., Liu, X. M., Ma, L., Li, J., Zhang, H., Gao, Y. P., et al. (2013). Effects of particle morphology, pore size and surface coating of mesoporous silica on Naproxen dissolution rate enhancement. Colloids Surf. B 101, 228–235. doi:10.1016/j.colsurfb.2012.06.026
Gupta, M., and Eugene, W. W. L. (2011). “Microwaves—theory,” in Microwaves and metals (Singapore: John Wiley and Sons (Asia) Pte Ltd.), 25–41.
Gurikov, P., and Smirnova, I. (2018). Amorphization of drugs by adsorptive precipitation from supercritical solutions: a review. J. Supercrit. Fluids 132, 105–125. doi:10.1016/j.supflu.2017.03.005
Habeche, F., Hachemaoui, M., Mokhtar, A., Chikh, K., Benali, F., Mekki, A., et al. (2020). Recent advances on the preparation and catalytic applications of metal complexes supported-mesoporous silica MCM-41. J. Inorg. Organomet. Polym. Mater 30, 3064–3083.
Hampsey, J. E., Castro, C. M., McCaughey, B., Wang, D., Mitchell, B., and Lu, Y. (2004). Preparation of micrometer-to sub-micrometer-sized nanostructured silica particles using high-energy ball milling. J. Am. Ceram. Soc. 87, 1280–1286. doi:10.1111/j.1151-2916.2004.tb07723.x
Han, N., Wang, Y., Deng, J., Zhou, J., Wu, Y., Yang, H., et al. (2019). Self-templated synthesis of hierarchical mesoporous SnO2 nanosheets for selective CO2 reduction. J. Mater Chem. A 7, 1267–1272. doi:10.1039/c8ta10959a
Hargrove, D., Liang, B., Kashfi-Sadabad, R., Joshi, G. N., Gonzalez-Fajardo, L., Glass, S., et al. (2020). Tumor-mesoporous silica nanoparticle interactions following intraperitoneal delivery for targeting peritoneal metastasis. J. Control Release 328, 846–858. doi:10.1016/j.jconrel.2020.11.003
He, H. X., Gan, Q., and Feng, C. G. (2018). An ion-imprinted silica gel polymer prepared by surface imprinting technique combined with aqueous solution polymerization for selective adsorption of Ni(II) from aqueous solution. Chin. J. Polym. Sci. 36 (4), 462–471. doi:10.1007/s10118-018-2063-5
He, Q., Cui, X., Cui, F., Guo, L., and Shi, J. (2009). Size-controlled synthesis of monodispersed mesoporous silica nanospheres under a neutral condition. Micropor Mesopor Mater 117, 609–616. doi:10.1016/j.micromeso.2008.08.004
He, Q., Zhang, J., Shi, J., Zhu, Z., Zhang, L., Bu, W., et al. (2010a). The effect of PEGylation of mesoporous silica nanoparticles on nonspecific binding of serum proteins and cellular responses. Biomaterials 31 (6), 1085–1092. doi:10.1016/j.biomaterials.2009.10.046
He, Q., Zhang, Z., Gao, F., Li, Y., and Shi, J. (2011a). In vivo biodistribution and urinary excretion of mesoporous silica nanoparticles: effects of particle size and PEGylation. Small 7 (2), 271–280. doi:10.1002/smll.201001459
He, Q. J., Zhang, J. M., Shi, J. L., Zhu, Z. Y., Zhang, L. X., Bu, W. B., et al. (2010b). The effect of PEGylation of mesoporous silica nanoparticles on nonspecific binding of serum proteins and cellular responses. Biomaterials 31, 1085–1092. doi:10.1016/j.biomaterials.2009.10.046
He, Q. J., Zhang, Z. W., Gao, F., Li, Y. P., and Shi, J. L. (2011b). In vivo biodistribution and urinary excretion of mesoporous silica nanoparticles: effects of particle size and PEGylation. Small 7, 271–280. doi:10.1002/smll.201001459
He, Y., Liang, S., Long, M., and Xu, H. (2017). Mesoporous silica nanoparticles as potential carriers for enhanced drug solubility of paclitaxel. Mater Sci. Eng. C 78, 12–17. doi:10.1016/j.msec.2017.04.049
Heikkilä, T., Salonen, J., Tuura, J., Kumar, N., Salmi, T., Murzin, D. Y., et al. (2007). Evaluation of mesoporous TCPSi, MCM-41, SBA-15, and TUD-1 materials as API carriers for oral drug delivery. Drug Deliv. 14, 337–347. doi:10.1080/10717540601098823
Hillerström, A., Andersson, M., Samuelsson, J., and van Stam, J. (2014). Solvent strategies for loading and release in mesoporous silica. Colloid Interface Sci. Commun. 3, 5–8. doi:10.1016/j.colcom.2015.01.001
Hillerström, A., van Stam, J., and Andersson, M. (2009). Ibuprofen loading into mesostructured silica using liquid carbon dioxide as a solvent. Green Chem. 11, 662–667. doi:10.1039/b821281c
Ho, Y. J., Wu, C. H., Jin, Q. f., Lin, C. Y., Chiang, P. H., Wu, N., et al. (2020). Superhydrophobic drug-loaded mesoporous silica nanoparticles capped with β-cyclodextrin for ultrasound image-guided combined antivascular and chemo-sonodynamic therapy. Biomaterials 232, 119723. doi:10.1016/j.biomaterials.2019.119723
Horcajada, P., Rámila, A., Pérez-Pariente, J., and Vallet-Regí, M. (2004). Influence of pore size of MCM-41 matrices on drug delivery rate. Micropor Mesopor Mater 68, 105–109. doi:10.1016/j.micromeso.2003.12.012
Hozayen, W. G., Mahmoud, A. M., Desouky, E. M., El-Nahass, E. S., Soliman, H. A., and Farghali, A. A. (2019). Cardiac and pulmonary toxicity of mesoporous silica nanoparticles is associated with excessive ROS production and redox imbalance in Wistar rats. Biomed. Pharmacother. 109, 2527–2538. doi:10.1016/j.biopha.2018.11.093
Hu, Y., Wang, J., Zhi, Z., Jiang, T., and Wang, S. (2011). Facile synthesis of 3D cubic mesoporous silica microspheres with a controllable pore size and their application for improved delivery of a water-insoluble drug. J. Colloid Interface Sci. 363, 410–417. doi:10.1016/j.jcis.2011.07.022
Hu, Y., Zhi, Z., Zhao, Q., Wu, C., Zhao, P., Jiang, H., et al. (2012). 3D cubic mesoporous silica microsphere as a carrier for poorly soluble drug carvedilol. Micropor Mesopor Mater 147, 94–101. doi:10.1016/j.micromeso.2011.06.001
Huang, C., Zhang, Z., Guo, Q., Zhang, L., Fan, F., Qin, Y., et al. (2019). A dual-model imaging theragnostic system based on mesoporous silica nanoparticles for enhanced cancer phototherapy. Adv. Healthc. Mater 8, 1900840. doi:10.1002/adhm.201900840
Huang, F., Guo, R., Xue, L., Cai, G., Wang, S., Li, Y., et al. (2020b). An acid-responsive microfluidic Salmonella biosensor using curcumin as a signal reporter and ZnO-capped mesoporous silica nanoparticles for signal amplification. Sens. Actuators B Chem. 312, 127958. doi:10.1016/j.snb.2020.127958
Huang, R., Shen, Y. W., Guan, Y. Y., Jiang, Y. X., Wu, Y., Rahman, K., et al. (2020a). Mesoporous silica nanoparticles: facile surface functionalization and versatile biomedical applications in oncology. Acta Biomater. 116, 1–15. doi:10.1016/j.actbio.2020.09.009
Huang, X., Li, L., Liu, T., Hao, N., Liu, H., Chen, D., et al. (2011). The shape effect of mesoporous silica nanoparticles on biodistribution, clearance, and biocompatibility in vivo. ACS Nano 5 (7), 5390–5399. doi:10.1021/nn200365a
Huang, X., Teng, X., Chen, D., Tang, F., and He, J. (2010a). The effect of the shape of mesoporous silica nanoparticles on cellular uptake and cell function. Biomaterials 31 (3), 438–448. doi:10.1016/j.biomaterials.2009.09.060
Huang, X., Teng, X., Chen, D., Tang, F., and He, J. (2010b). The effect of the shape of mesoporous silica nanoparticles on cellular uptake and cell function. Biomaterials 31, 438–448. doi:10.1016/j.biomaterials.2009.09.060
Ibrahim, I. A. M., Zikry, A. A. F., and Sharaf, M. A. (2010). Preparation of spherical silica nanoparticles: stöber silica. J. Am. Sci. 6, 985–989.
Inoue, M., Sakamoto, K., Suzuki, A., Nakai, S., Ando, A., Shiraki, Y., et al. (2021). Size and surface modification of silica nanoparticles affect the severity of lung toxicity by modulating endosomal ROS generation in macrophages. Part Fibre Toxicol. 18 (1), 21–20. doi:10.1186/s12989-021-00415-0
Isa, M., Ahmad, E. D., and Abdul, R. M. B. (2019). Optimization of synthesis parameters of mesoporous silica nanoparticles based on ionic liquid by experimental design and its application as a drug delivery agent. J. Nanomater 2019 (8), 1–8. doi:10.1155/2019/4982054
Ishida, T., Masuda, K., Ichikawa, T., Ichihara, M., Irimura, K., and Kiwada, H. (2003). Accelerated clearance of a second injection of PEGylated liposomes in mice. Int. J. Pharm. 255 (1–2), 167–174. doi:10.1016/s0378-5173(03)00085-1
Jadhav, S. A., Brunella, V., Miletto, I., Berlier, G., and Scalarone, D. (2016). Synthesis of poly (N-isopropylacrylamide) by distillation precipitation polymerization and quantitative grafting on mesoporous silica. J. Appl. Polym. Sci. 133, 44181. doi:10.1002/app.44181
Jadhav, S. A., Garud, H. B., Patil, A. H., Patil, G. D., Patil, C. R., Dongale, T. D., et al. (2019). Recent advancements in silica nanoparticles-based technologies for removal of dyes from water. Colloid Interface Sci. Commun. 30, 100181. doi:10.1016/j.colcom.2019.100181
Jadhav, S. A., Nistico, R., Magnacca, G., and Scalarone, D. (2018). Packed hybrid silica nanoparticles as sorbents with thermo-switchable surface chemistry and pore size for fast extraction of environmental pollutants. RSC Adv. 8, 1246–1254. doi:10.1039/c7ra11869d
Jambhrunkar, S., Qu, Z., Popat, A., Karmakar, S., Xu, C., and Yu, C. (2014). Modulating in vitro release and solubility of griseofulvin using functionalized mesoporous silica nanoparticles. J. Colloid Interface Sci. 434, 218–225. doi:10.1016/j.jcis.2014.08.019
Jammaer, J., Aerts, A., D’Haen, J., Seo, J. W., and Martens, J. A. (2009). Convenient synthesis of ordered mesoporous silica at room temperature and quasi-neutral pH. J. Mater Chem. 19, 8290–8293. doi:10.1039/b915273c
Jana, S. K., Mochizuki, A., and Namba, S. (2004). Progress in pore-size control of mesoporous MCM-41 molecular sieve using surfactant having different alkyl chain lengths and various organic auxiliary chemicals. Catal. Surv. Asia 8, 1–13. doi:10.1023/b:cats.0000015110.85694.d9
Jasinski, D. L., Li, H., and Guo, P. (2018). The effect of size and shape of RNA nanoparticles on biodistribution. Mol. Ther. 26 (3), 784–792. doi:10.1016/j.ymthe.2017.12.018
Jeelani, P. G., Mulay, P., Venkat, R., and Ramalingam, C. (2020). Multifaceted application of silica nanoparticles: a review. Silicon 12, 1337–1354. doi:10.1007/s12633-019-00229-y
Jeyalakshmi, V., Rajalakshmi, K., Mahalakshmy, R., Krishnamurthy, K. R., and Viswanathan, B. (2013). Application of photocatalysis for mitigation of carbon dioxide. Res. Chem. Intermed. 39, 2565–2602. doi:10.1007/s11164-012-0783-7
Jia, L., Shen, J., Li, Z., Zhang, D., Zhang, Q., Duan, C., et al. (2012). Successfully tailoring the pore size of mesoporous silica nanoparticles: exploitation of delivery systems for poorly water-soluble drugs. Int. J. Pharm. 439, 81–91. doi:10.1016/j.ijpharm.2012.10.011
Jian, X., Tian, W., Li, J., Deng, L., Zhou, Z., Zhang, L., et al. (2019). High-temperature oxidation-resistant ZrN0.4B0.6/SiC nanohybrid for enhanced microwave absorption. ACS Appl. Mater Interfaces 11, 15869–15880. doi:10.1021/acsami.8b22448
Jiang, Y., Liu, S., Zhang, Y., Li, H., He, H., Dai, J., et al. (2017). Magnetic mesoporous nanospheres anchored with LyP-1 as an efficient pancreatic cancer probe. Biomaterials 115, 9–18. doi:10.1016/j.biomaterials.2016.11.006
Jyothirmayi, P., Anusha, A., Sushma, V., Sirisha, V., Sravani, K., Nagalakshmi, G., et al. (2024). Mesoporous silica nanoparticles as promising nano carriers—an overview. Eur. J. Pharm. Med. Res. 11 (1), 121–129.
Kajani, A. A., Rafiee, L., Javanmard, S. H., Dana, N., and Jandaghian, S. (2023). Carbon dot incorporated mesoporous silica nanoparticles for targeted cancer therapy and fluorescence imaging. RSC Adv. 13 (14), 9491–9500. doi:10.1039/d3ra00768e
Kaluđerović, G. N., Pérez-Quintanilla, D., Sierra, I., Prashar, S., del Hierro, I., Žižak, Ž., et al. (2010b). Silica-supported organotin(IV) complexes as antitumor agents: synthesis, characterization, and biological activity. J. Mater Chem. 20, 806–814. doi:10.1039/b919269g
Kaluđerović, G. N., Pérez-Quintanilla, D., Žižak, Ž., Juranić, Z. D., and Gómez-Ruiz, S. (2010a). Improvement of cytotoxicity of titanocene-functionalized mesoporous materials by the increase of the titanium content. Dalton Trans. 39, 2597–2608. doi:10.1039/b920051g
Kankala, R. K., Zhang, Y. S., Wang, S. B., Lee, C. H., and Chen, A. Z. (2017). Supercritical fluid technology: an emphasis on drug delivery and related biomedical applications. Adv. Healthc. Mater 6, 1700433. doi:10.1002/adhm.201700433
Kankala, R. K., Zhu, K., Sun, X. N., Liu, C. G., Wang, S. B., and Chen, A. Z. (2018). Cardiac tissue engineering on the nanoscale. ACS Biomater. Sci. Eng. 4, 800–818. doi:10.1021/acsbiomaterials.7b00913
Kappe, C. O. (2008). Microwave dielectric heating in synthetic organic chemistry. Chem. Soc. Rev. 37, 1127–1139. doi:10.1039/b803001b
Kappe, C. O., and Dallinger, D. (2009). Controlled microwave heating in modern organic synthesis: highlights from the 2004–2008 literature. Mol. Divers 13, 71–193. doi:10.1007/s11030-009-9138-8
Karbassian, F. (2018). “Porous silicon,” in Porosity—process technologies and applications. Editor T. H. Ghrib (London: Intech Open).
Ke, P. C., Lin, S., Parak, W. J., Davis, T. P., and Caruso, F. (2017). A decade of the protein corona. ACS Nano 11 (12), 11773–11776. doi:10.1021/acsnano.7b08008
Kempen, P. J., Greasley, S., Parker, K. A., Campbell, J. C., Chang, H. Y., Jones, J. R., et al. (2015). Theranostic mesoporous silica nanoparticles biodegrade after pro-survival drug delivery and ultrasound/magnetic resonance imaging of stem cells. Theranostics 5, 631–642. doi:10.7150/thno.11389
Kerkhofs, S., Saïdi, F., Vandervoort, N., Van den Mooter, G., Martineau, C., Taulelle, F., et al. (2015). Silica capsules enclosing P123 triblock copolymer micelles for flurbiprofen storage and release. J. Mater Chem. B 3, 3054–3061. doi:10.1039/c5tb00058k
Khan, I., Farhan, M., Singh, P., and Thiagarajan, P. (2014). Nanotechnology for environmental remediation. Res. J. Pharm. Biol. Chem. Sci. 5 (3), 1916–1927.
Khan, N. A., and Jhung, S. H. (2015). Synthesis of metal-organic frameworks (MOFs) with microwave or ultrasound: rapid reaction, phase-selectivity, and size reduction. Coord. Chem. Rev. 285, 11–23. doi:10.1016/j.ccr.2014.10.008
Kharlamov, A. N., Feinstein, J. A., Cramer, J. A., Boothroyd, J. A., Shishkina, E. V., and Shur, V. (2017). Plasmonic photothermal therapy of atherosclerosis with nanoparticles: long-term outcomes and safety in NANOM-FIM trial. Future Cardiol. 13 (4), 345–363. doi:10.2217/fca-2017-0009
Khushalani, D., Kuperman, A., Ozin, G. A., Tanaka, K., Coombs, N., Olken, M. M., et al. (1995). Metamorphic materials: restructuring siliceous mesoporous materials. Adv. Mat. 7, 842–846. doi:10.1002/adma.19950071005
Kiatphuengporn, S., Jantaratana, P., Limtrakul, J., and Chareonpanich, M. (2016). Magnetic field-enhanced catalytic CO2 hydrogenation and selective conversion to light hydrocarbons over Fe/MCM-41 catalysts. Chem. Eng. J. 306, 866–875. doi:10.1016/j.cej.2016.08.029
Kim, J., Lee, J. E., Lee, J., Yu, J. H., Kim, B. C., An, K., et al. (2006). Magnetic fluorescent delivery vehicle using uniform mesoporous silica spheres embedded with monodisperse magnetic and semiconductor nanocrystals. J. Am. Chem. Soc. 128, 688–689. doi:10.1021/ja0565875
Kinnari, P., Makila, E., Heikkila, T., Salonen, J., Hirvonen, J., and Santos, H. A. (2011). Comparison of mesoporous silicon and non-ordered mesoporous silica materials as drug carriers for itraconazole. Int. J. Pharm. 414, 148–156. doi:10.1016/j.ijpharm.2011.05.021
Kleitz, F., Liu, D., Gopinathan, M. A., Park, I. S., Solovyov, L. A., Shmakov, A. N., et al. (2003). Large cage face-centered-cubic Fm3m mesoporous silica: synthesis and structure. J. Phys. Chem. B 107, 14296–14300. doi:10.1021/jp036136b
Koh, M., Ju, B., and Seo, W. (2019). A review on public understanding of carbon dioxide capture and storage (CCS) in South Korea. Energy Procedia 159, 315–320. doi:10.1016/j.egypro.2019.01.009
Kompella, U. B., and Koushik, K. (2001). Preparation of drug delivery systems using supercritical fluid technology. Crit. Rev. Ther. Drug Carr. Syst. 18, 28–199. doi:10.1615/critrevtherdrugcarriersyst.v18.i2.20
Kresge, C. T., Leonowicz, M. E., Roth, W. J., Vartuli, J. C., and Beck, J. S. (1992). Ordered mesoporous molecular sieves synthesized by a liquid-crystal template mechanism. Nature 359 (6397), 710–712. doi:10.1038/359710a0
Kresge, C. T., Vartuli, J. C., Roth, W. J., and Leonowicz, M. E. (2004). “The discovery of ExxonMobil’s M41S family of mesoporous molecular sieves,” in Studies in surface science and catalysis. Editor O. Terasaki (Amsterdam: Elsevier), 148, 53–72. doi:10.1016/s0167-2991(04)80193-9
Kumar, D., Chirravuri, S. V. S., and Shastri, N. R. (2014). Impact of surface area of silica particles on dissolution rate and oral bioavailability of poorly water-soluble drugs: a case study with aceclofenac. Int. J. Pharm. 461, 459–468. doi:10.1016/j.ijpharm.2013.12.017
Kurdyukov, D. A., Eurov, D. A., Kirilenko, D. A., Sokolov, V. V., and Golubev, V. G. (2018). Tailoring the size and microporosity of Stöber silica particles. Microporous Mesoporous Mat. 258, 205–210. doi:10.1016/j.micromeso.2017.09.017
Kurisingal, J. F., Rachuri, Y., Gu, Y., Choe, Y., and Park, D. W. (2020). Multi-variate metal-organic framework as an efficient catalyst for the cycloaddition of CO2 and epoxides in a gas-liquid-solid reactor. Chem. Eng. J. 386, 121700. doi:10.1016/j.cej.2019.05.061
Kurisingal, J. F., Rachuri, Y., Gu, Y., Kim, G. H., and Park, D. W. (2019a). Binary metal-organic frameworks: catalysts for the efficient solvent-free CO2 fixation reaction via cyclic carbonate synthesis. Appl. Catal. A 571, 1–11. doi:10.1016/j.apcata.2018.11.035
Kurisingal, J. F., Rachuri, Y., Palakkal, A. S., Pillai, R. S., Gu, Y., Choe, Y., et al. (2019b). Water-tolerant DUT-series metal-organic frameworks: a theoretical-experimental study for the chemical fixation of CO2 and catalytic transfer hydrogenation of ethyl levulinate to γ-valerolactone. ACS Appl. Mater Interfaces 11, 41458–41471. doi:10.1021/acsami.9b16834
Lamson, N. G., Berger, A., Fein, K. C., and Whitehead, K. A. (2020). Anionic nanoparticles enable the oral delivery of proteins by enhancing intestinal permeability. Nat. Biomed. Eng. 4 (1), 84–96. doi:10.1038/s41551-019-0465-5
Le, T. T., Elyafi, A. K. E., Mohammed, A. R., and Al-Khattawi, A. (2019). Delivery of poorly soluble drugs via mesoporous silica: impact of drug overloading on release and thermal profiles. Pharmaceutics 11, 269. doi:10.3390/pharmaceutics11060269
Lee, C. H., Cheng, S. H., Huang, I. P., Souris, J. S., Yang, C. S., Mou, C. Y., et al. (2010). Intracellular pH-responsive mesoporous silica nanoparticles for the controlled release of anticancer chemotherapeutics. Angew. Chem. 122 (44), 8390–8395. doi:10.1002/ange.201002639
Lee, J. E., Lee, N., Kim, T., Kim, J., and Hyeon, T. (2011). Multifunctional mesoporous silica nanocomposite nanoparticles for theranostic applications. Acc. Chem. Res. 44 (10), 893–902. doi:10.1021/ar2000259
Lee, K. I., Su, C. C., Fang, K. M., Wu, C. C., Wu, C. T., and Chen, Y. W. (2020). Ultrafine silicon dioxide nanoparticles cause lung epithelial cells apoptosis via oxidative stress-activated PI3K/Akt-mediated mitochondria- and endoplasmic reticulum stress-dependent signaling pathways. Sci. Rep. 10 (1), 9928. doi:10.1038/s41598-020-66644-z
Lehto, V. P., and Riikonen, J. (2014). “Drug loading and characterization of porous silicon materials,” in Porous silicon for biomedical applications. Editor H. A. Santos (Cambridge: Woodhead Publishing), 337–355.
Lercher, J. A., Kaliaguine, S., and Gobin, O. C. (2006). SBA-16 materials synthesis, diffusion and sorption properties. Munich: Munich, Germany: Technical University of.
Li, J., Zheng, L., Cai, H., Sun, W., Shen, M., Zhang, G., et al. (2013a). Polyethyleneimine-mediated synthesis of folic acid-targeted iron oxide nanoparticles for in vivo tumor MR imaging. Biomaterials 34 (33), 8382–8392. doi:10.1016/j.biomaterials.2013.07.070
Li, J. S., Huang, H., Zhou, Y. J., Zhang, C. Y., and Li, Z. T. (2017). Research progress of graphene-based microwave absorbing materials in the last decade. J. Mater Res. 32, 1213–1230. doi:10.1557/jmr.2017.80
Li, N., Yu, Z., Pan, W., Han, Y., Zhang, T., and Tang, B. (2013b). A near-infrared light-triggered nanocarrier with reversible DNA valves for intracellular controlled release. Adv. Funct. Mater 23 (18), 2255–2262. doi:10.1002/adfm.201202564
Li, W. P., Liao, P. Y., Su, C. H., and Yeh, C. S. (2014). Formation of oligonucleotide-gated silica shell-coated Fe3O4-Au core–shell nanotrisoctahedra for magnetically targeted and near-infrared light-responsive theranostic platform. J. Am. Chem. Soc. 136 (28), 10062–10075. doi:10.1021/ja504118q
Li, X. Q., and Zhang, W. X. (2006). Iron nanoparticles: the core−shell structure and unique properties for Ni(II) sequestration. Langmuir. 22 (10), 4638–4642. doi:10.1021/la060057k
Li, Z., Zhang, Y. T., and Feng, N. P. (2019). Mesoporous silica nanoparticles: synthesis, classification, drug loading, pharmacokinetics, biocompatibility, and application in drug delivery. Expert Opin. Drug Deliv. 16, 219–237. doi:10.1080/17425247.2019.1575806
Limnell, T., Santos, H. A., Mäkilä, E., Heikkilä, T., Salonen, J., Murzin, D. Y., et al. (2011). Drug delivery formulations of ordered and nonordered mesoporous silica: comparison of three drug loading methods. J. Pharm. Sci. 100, 3294–3306. doi:10.1002/jps.22577
Lin, C. H., Cheng, S. H., Liao, W. N., Wei, P. R., Sung, P. J., Weng, C. F., et al. (2012). Mesoporous silica nanoparticles for the improved anticancer efficacy of cisplatin. Int. J. Pharm. 429 (1–2), 138–147. doi:10.1016/j.ijpharm.2012.03.026
Liu, J., Bu, W., Pan, L., and Shi, J. (2013). NIR-triggered anticancer drug delivery by upconverting nanoparticles with integrated azobenzene-modified mesoporous silica. Angew. Chem. Int. Ed. Engl. 52 (16), 4375–4379. doi:10.1002/anie.201300183
Liu, J., Liang, H., Li, M., Luo, Z., Zhang, J., Guo, X., et al. (2018). Tumor acidity activating multifunctional nanoplatform for NIR-mediated multiple enhanced photodynamic and photothermal tumor therapy. Biomaterials 157, 107–124. doi:10.1016/j.biomaterials.2017.12.003
Liu, J., Liu, Y., Bu, W., Bu, J., Sun, Y., Du, J., et al. (2014). Ultrasensitive nanosensors based on upconversion nanoparticles for selective hypoxia imaging in vivo upon near-infrared excitation. J. Am. Chem. Soc. 136 (27), 9701–9709. doi:10.1021/ja5042989
Liu, J., Luo, Z., Zhang, J., Luo, T., Zhou, J., Zhao, X., et al. (2016). Hollow mesoporous silica nanoparticles facilitated drug delivery via cascade pH stimuli in tumor microenvironment for tumor therapy. Biomaterials 83, 51–65. doi:10.1016/j.biomaterials.2016.01.008
Liu, Q., Cheng, H. B., Ma, R., Yu, M., Huang, Y., Li, L., et al. (2023). A DNA-based nanodevice for near-infrared light-controlled drug release and bioimaging. Nano Today 48, 101747. doi:10.1016/j.nantod.2022.101747
Liu, Q., Zhang, J., Sun, W., Xie, Q. R., Xia, W., and Gu, H. (2012). Delivering hydrophilic and hydrophobic chemotherapeutics simultaneously by magnetic mesoporous silica nanoparticles to inhibit cancer cells. Int. J. Nanomed 7, 999–1013. doi:10.2147/ijn.s28088
Liu, R., Zhang, Y., Zhao, X., Agarwal, A., Mueller, L. J., and Feng, P. (2010). pH-responsive nanogated ensemble based on gold-capped mesoporous silica through an acid-labile acetal linker. J. Am. Chem. Soc. 132 (5), 1500–1501. doi:10.1021/ja907838s
Liu, Y., Liu, Y., Bu, W., Cheng, C., Zuo, C., Xiao, Q., et al. (2015a). Hypoxia induced by upconversion-based photodynamic therapy: towards highly effective synergistic bioreductive therapy in tumors. Angew. Chem. Int. Ed. Engl. 54 (28), 8105–8109. doi:10.1002/anie.201500478
Liu, Y., Wei, H., Tang, J., Yuan, J., Wu, M., Yao, C., et al. (2020b). Dysfunction of pulmonary epithelial tight junction induced by silicon dioxide nanoparticles via the ROS/ERK pathway and protein degradation. Chemosphere 255, 126954. doi:10.1016/j.chemosphere.2020.126954
Liu, Y. Q., Xue, S. M., Zhang, P., Xu, L. N., Wang, D. P., Li, G., et al. (2020a). Silica nanoparticles disturb ion channels and transmembrane potentials of cardiomyocytes and induce lethal arrhythmias in mice. Int. J. Nanomedicine 15, 7397–7413. doi:10.2147/ijn.s261692
Liu, Z., Rong, P., Yu, L., Zhang, X., Yang, C., Guo, F., et al. (2015b). Dual-modality noninvasive mapping of sentinel lymph node by photoacoustic and near-infrared fluorescent imaging using dye-loaded mesoporous silica nanoparticles. Mol. Pharm. 12, 3119–3128. doi:10.1021/mp500698b
Lu, J., Li, Z., Zink, J. I., and Tamanoi, F. (2012). In vivo tumor suppression efficacy of mesoporous silica nanoparticles-based drug-delivery system: enhanced efficacy by folate modification. Nanomed Nanotechnol. Biol. Med. 8 (2), 212–220. doi:10.1016/j.nano.2011.06.002
Lu, J., Liong, M., Li, Z., Zink, J. I., and Tamanoi, F. (2010). Biocompatibility, biodistribution, and drug-delivery efficiency of mesoporous silica nanoparticles for cancer therapy in animals. Small 6 (16), 1794–1805. doi:10.1002/smll.201000538
Lu, J., Liong, M., Zink, J. I., and Tamanoi, F. (2007). Mesoporous silica nanoparticles as a delivery system for hydrophobic anticancer drugs. Small 3, 1341–1346. doi:10.1002/smll.200700005
Lu, L., Yang, M., Kim, Y., Zhang, T., Kwon, N., Li, H., et al. (2022). An unconventional nano-AIEgen originating from a natural plant polyphenol for multicolor bioimaging. Cell Rep. Phys. Sci. 3, 100745. doi:10.1016/j.xcrp.2022.100745
Luo, Y., Li, B., Wang, W., Wu, K., and Tan, B. (2012). Hypercrosslinked aromatic heterocyclic microporous polymers: a new class of highly selective CO2 capturing materials. Adv. Mat. 24, 5703–5707. doi:10.1002/adma.201202447
Lv, X., Zhang, L., Xing, F., and Lin, H. (2016). Controlled synthesis of monodispersed mesoporous silica nanoparticles: particle size tuning and formation mechanism investigation. Micropor Mesopor Mater 225, 238–244. doi:10.1016/j.micromeso.2015.12.024
Ma, K., Sai, H., and Wiesner, U. (2012). Ultrasmall sub-10 nm near-infrared fluorescent mesoporous silica nanoparticles. J. Am. Chem. Soc. 134, 13180–13183. doi:10.1021/ja3049783
Ma, K., Werner-Zwanziger, U., Zwanziger, J., and Wiesner, U. (2013). Controlling growth of ultrasmall sub-10 nm fluorescent mesoporous silica nanoparticles. Chem. Mater 25, 677–691. doi:10.1021/cm303242h
Ma, R., Qi, Y., Zhao, X., Li, X., Sun, X., Niu, P., et al. (2020). Amorphous silica nanoparticles accelerated atherosclerotic lesion progression in ApoE−/− mice through endoplasmic reticulum stress-mediated CD36 up-regulation in macrophage. Part Fibre Toxicol. 17 (1), 50. doi:10.1186/s12989-020-00380-0
Ma, X., Teh, C., Zhang, Q., Borah, P., Choong, C., Korzh, V., et al. (2014). Redox-responsive mesoporous silica nanoparticles: a physiologically sensitive co-delivery vehicle for siRNA and doxorubicin. Antioxid. Redox Signal 21 (5), 707–722. doi:10.1089/ars.2012.5076
Maeda, H., Wu, J., Sawa, T., Matsumura, Y., and Hori, K. (2000). Tumor vascular permeability and the EPR effect in macromolecular therapeutics: a review. J. Control Release 65 (1–2), 271–284. doi:10.1016/s0168-3659(99)00248-5
Mal, N. K., Fujiwara, M., Tanaka, Y., Taguchi, T., and Matsukata, M. (2003). Photo-switched storage and release of guest molecules in the pore void of coumarin-modified MCM-41. Chem. Mater 15, 3385–3394. doi:10.1021/cm0343296
Maleki, A., and Hamidi, M. (2016). Dissolution enhancement of a model poorly water-soluble drug, atorvastatin, with ordered mesoporous silica: comparison of MSF with SBA-15 as drug carriers. Expert Opin. Drug Deliv. 13, 171–181. doi:10.1517/17425247.2015.1111335
Maleki, A., Kettiger, H., Schoubben, A., Rosenholm, J. M., Ambrogi, V., and Hamidi, M. (2017). Mesoporous silica materials: from physicochemical properties to enhanced dissolution of poorly water-soluble drugs. J. Control Release 262, 329–347. doi:10.1016/j.jconrel.2017.07.047
Malfait, B., Correia, N. T., Mussi, A., Paccou, L., Guinet, Y., and Hédoux, A. (2019). Solid-state loading of organic molecular materials within mesoporous silica matrix: application to ibuprofen. Micropor Mesopor Mater 277, 203–207. doi:10.1016/j.micromeso.2018.10.022
Mallick, K., Witcomb, M. J., and Scurrell, M. S. (2004). Polymer stabilized silver nanoparticles: a photochemical synthesis route. J. Mater Sci. 39 (14), 4459–4463. doi:10.1023/b:jmsc.0000034138.80116.50
Manzano, M., Colilla, M., and Vallet-Regi, M. (2009). Drug delivery from ordered mesoporous matrices. Expert Opin. Drug Deliv. 6, 1383–1400. doi:10.1517/17425240903304024
Maribel, G., Guzmán, J. D., and Godet, S. (2009). Synthesis of silver nanoparticles by chemical reduction method and their antibacterial activity. Int. J. Chem. Biomol. Eng. 2, 104.
Martínez-Carmona, M., Lozano, D., Colilla, M., and Vallet-Regí, M. (2018). Lectin-conjugated pH-responsive mesoporous silica nanoparticles for targeted bone cancer treatment. Acta Biomater. 65, 393–404. doi:10.1016/j.actbio.2017.11.007
Matsumura, Y., and Maeda, H. (1986). A new concept for macromolecular therapeutics in cancer chemotherapy: mechanism of tumoritropic accumulation of proteins and the antitumor agent smancs. Cancer Res. 46 (12 Pt 1), 6387–6392.
McCarthy, C. A., Ahern, R. J., Dontireddy, R., Ryan, K. B., and Crean, A. M. (2016). Mesoporous silica formulation strategies for drug dissolution enhancement: a review. Expert Opin. Drug Deliv. 13, 93–108. doi:10.1517/17425247.2016.1100165
Meka, A. K., Jenkins, L. J., Dàvalos-Salas, M., Pujara, N., Wong, K. Y., Kumeria, T., et al. (2018). Enhanced solubility, permeability and anticancer activity of vorinostat using tailored mesoporous silica nanoparticles. Pharmaceutics 10 (4), 283. doi:10.3390/pharmaceutics10040283
Mellaerts, R., Jammaer, J. A. G., Van Speybroeck, M., Chen, H., Van Humbeeck, J., Augustijns, P., et al. (2008). Physical state of poorly water-soluble therapeutic molecules loaded into SBA-15 ordered mesoporous silica carriers: a case study with itraconazole and ibuprofen. Langmuir 24, 8651–8659. doi:10.1021/la801161g
Meng, H., Xue, M., Xia, T., Ji, Z., Tarn, D., Zink, J. I., et al. (2011b). Use of size and a copolymer design feature to improve the biodistribution and the enhanced permeability and retention effect of doxorubicin-loaded mesoporous silica nanoparticles in a murine xenograft tumor model. ACS Nano 5, 4131–4144. doi:10.1021/nn200809t
Meng, H., Yang, S., Li, Z., Xia, T., Chen, J., Ji, Z., et al. (2011a). Aspect ratio determines the quantity of mesoporous silica nanoparticle uptake by a small GTPase-dependent macropinocytosis mechanism. ACS Nano 5 (6), 4434–4447. doi:10.1021/nn103344k
Mirzaei, A., and Neri, G. (2016). Microwave-assisted synthesis of metal oxide nanostructures for gas sensing application: a review. Sens. Actuators B Chem. 237, 749–775. doi:10.1016/j.snb.2016.06.114
Miura, H., Kanebako, M., Shirai, H., Nakao, H., Inagi, T., and Terada, K. (2010). Enhancement of dissolution rate and oral absorption of a poorly water-soluble drug, K-832, by adsorption onto porous silica using supercritical carbon dioxide. Eur. J. Pharm. Biopharm. 76, 215–221. doi:10.1016/j.ejpb.2010.06.016
Mohan, A., Peter, J., Rout, L., Thomas, A. M., Nagappan, S., Parambadath, S., et al. (2021a). Facile synthesis of silver nanoparticles stabilized dual-responsive silica nanohybrid: a highly active switchable catalyst for oxidation of alcohols in aqueous medium. Colloids Surf. A 611, 125846. doi:10.1016/j.colsurfa.2020.125846
Mohan, A., Peter, J., Rout, L., Thomas, A. M., Nagappan, S., Zhang, W. J., et al. (2021b). In situ thermosensitive hybrid mesoporous silica: preparation and the catalytic activities for carbonyl compound reduction. Dalton Trans. 50, 11730–11741. doi:10.1039/d1dt00323b
Mohan, A., Rout, L., Thomas, A. M., Nagappan, S., Parambadath, S., Park, S. S., et al. (2020b). Silver nanoparticles impregnated pH-responsive nanohybrid system for the catalytic reduction of dyes. Microporous Mesoporous Mat. 303, 110260. doi:10.1016/j.micromeso.2020.110260
Mohan, A., Rout, L., Thomas, A. M., Peter, J., Nagappan, S., Parambadath, S., et al. (2020a). Palladium nanoparticles-anchored dual-responsive SBA-15-PNIPAM/PMAA nanoreactor: a novel heterogeneous catalyst for a green Suzuki-Miyaura cross-coupling reaction. RSC Adv. 10, 28193–28204. doi:10.1039/d0ra05786j
Möller, K., and Bein, T. (2019). Degradable drug carriers: vanishing mesoporous silica nanoparticles. Chem. Mater 31 (12), 4364–4378. doi:10.1021/acs.chemmater.9b00221
Mortera, R., Vivero-Escoto, J., Slowing, I. I., Garrone, E., Onida, B., and Lin, V. S. Y. (2009a). Cell-induced intracellular controlled release of membrane-impermeable cysteine from a mesoporous silica nanoparticle-based drug delivery system. Chem. Commun. 45, 3219–3221. doi:10.1039/b900559e
Mortera, R., Vivero-Escoto, J., Slowing, I. I., Garrone, E., Onida, B., and Lin, V. S. Y. (2009b). Cell-induced intracellular controlled release of membrane-impermeable cysteine from a mesoporous silica nanoparticle-based drug delivery system. Chem. Commun., 3219–3221. doi:10.1039/b900559e
Muhammad, F., Guo, M., Qi, W., Sun, F., Wang, A., Guo, Y., et al. (2011). pH-triggered controlled drug release from mesoporous silica nanoparticles via intracelluar dissolution of ZnO nanolids. J. Am. Chem. Soc. 133 (23), 8778–8781. doi:10.1021/ja200328s
Natarajan, S. K., and Selvaraj, S. (2014). Mesoporous silica nanoparticles: importance of surface modifications and its role in drug delivery. RSC Adv. 4, 14328–14334. doi:10.1039/c4ra00781f
Nel, A. E., Madler, L., Velegol, D., Xia, T., Hoek, E. M. V., Somasundaran, P., et al. (2009). Understanding biophysicochemical interactions at the nano-bio interface. Nat. Mater 8 (7), 543–557. doi:10.1038/nmat2442
Nel, A. E., Zink, J. I., and Meng, H. (2016). Lipid bilayer coated mesoporous silica nanoparticles with a high loading capacity for one or more anticancer agents. U. S. Pat. US20160008283A1.
Niculescu, V. C. (2020). Mesoporous silica nanoparticles for bio-applications. Front. Mater 7, 36. doi:10.3389/fmats.2020.00036
Nithya, T., Kavitha, P., Karthik, P., Anpo, M., and Neppolian, B. (2019). “The microwave-assisted synthesis of silica-based materials and their photocatalysis,” in Chemistry of silica and zeolite-based materials (Amsterdam: Elsevier), 73–88.
Nuchuchua, O., Nejadnik, M. R., Goulooze, S. C., Lješković, N. J., Every, H. A., and Jiskoot, W. (2017). Characterization of drug delivery particles produced by supercritical carbon dioxide technologies. J. Supercrit. Fluids 128, 244–262. doi:10.1016/j.supflu.2017.06.002
Nžk, D. J. O. (2015). Large pore mesoporous silica nanomaterials for application in delivery of biomolecules. Nanoscale 7, 2199–2209. doi:10.1039/c4nr06114d
Owen, R. E., Plucinski, P., Mattia, D., Torrente-Murciano, L., Ting, V. P., and Jones, M. D. (2016). Effect of support of Co-Na-Mo catalysts on the direct conversion of CO2 to hydrocarbons. J. CO2 Util. 16, 97–103. doi:10.1016/j.jcou.2016.06.009
Paris, J. L., Cabañas, M. V., Manzano, M., and Vallet-Regí, M. (2015). Polymer-grafted mesoporous silica nanoparticles as ultrasound-responsive drug carriers. ACS Nano 9 (11), 11023–11033. doi:10.1021/acsnano.5b04378
Park, S. E., Chang, J. S., Hwang, Y. K., Kim, D. S., Jhung, S. H., and Hwang, J. S. (2004). Supramolecular interactions and morphology control in microwave synthesis of nanoporous materials. Catal. Surv. Asia 8, 91–110. doi:10.1023/b:cats.0000026990.25778.a8
Parvanian, A. M., Sadeghi, N., Rafiee, A., Shearer, C. J., and Jafarian, M. (2022). Application of porous materials for CO2 reutilization: a review. Energies 15 (1), 63–65. doi:10.3390/en15010063
Parveen, A., Rizvi, S. H., Sushma, , Mahdi, F., Ahmad, I., Singh, P. P., et al. (2017). Intranasal exposure to silica nanoparticles induces alterations in pro-inflammatory environment of rat brain. Toxicol. Ind. Health 33 (2), 119–132. doi:10.1177/0748233715602985
Pasquali, I., and Bettini, R. (2008). Are pharmaceutics really going supercritical? Int. J. Pharm. 364, 176–187. doi:10.1016/j.ijpharm.2008.05.014
Peer, D., Karp, J. M., Hong, S., Farokhzad, O. C., Margalit, R., and Langer, R. (2007). Nanocarriers as an emerging platform for cancer therapy. Nat. Nanotechnol. 2 (12), 751–760. doi:10.1038/nnano.2007.387
Peng, Z., Hwang, J. Y., and Andriese, M. (2012). Magnetic loss in microwave heating. Appl. Phys. Express 5, 027304. doi:10.1143/apex.5.027304
Pérez-Quintanilla, D., Gómez-Ruiz, S., Žižak, Ž., Sierra, I., Prashar, S., del Hierro, I., et al. (2009). A new generation of anticancer drugs: mesoporous materials modified with titanocene complexes. Chem. Eur. J. 15, 5588–5597. doi:10.1002/chem.200900151
Petros, R. A., and DeSimone, J. M. (2010). Strategies in the design of nanoparticles for therapeutic applications. Nat. Rev. Drug Discov. 9 (8), 615–627. doi:10.1038/nrd2591
Phillips, E., Peñate-Medina, O., Zanzonico, P., Carvajal, R., Mohan, P., Ye, Y., et al. (2014a). Clinical translation of an ultrasmall inorganic optical-PET imaging nanoparticle probe. Sci. Transl. Med. 6 (260), 260ra149. doi:10.1126/scitranslmed.3009524
Phillips, E., Penate-Medina, O., Zanzonico, P. B., Carvajal, R. D., Mohan, P., Ye, Y., et al. (2014b). Clinical translation of an ultrasmall inorganic optical-PET imaging nanoparticle probe. Sci. Transl. Med. 6, 260ra149. doi:10.1126/scitranslmed.3009524
Phillips, E., Penate-Medina, O., Zanzonico, P. B., Carvajal, R. D., Mohan, P., Ye, Y., et al. (2014c). Clinical translation of an ultrasmall inorganic optical-PET imaging nanoparticle probe. Sci. Transl. Med. 6 (260), 260ra149. doi:10.1126/scitranslmed.3009524
Pillai, G. (2019). “Nanotechnology toward treating cancer: a comprehensive review,” in Applications of targeted nano drugs and delivery systems. Editors S. S. Mohapatra, S. Ranjan, N. Dasgupta, R. K. Mishra, and S. Thomas (Amsterdam: Elsevier), 221–256.
Popat, A., Ross, B. P., Liu, J., Jambhrunkar, S., Kleitz, F., and Qiao, S. (2012). Enzyme-responsive controlled release of covalently bound prodrug from functional mesoporous silica nanospheres. Angew. Chem. Int. Ed. Engl. 51, 12486–12489. doi:10.1002/anie.201206416
Porosoff, M. D., Yan, B., and Chen, J. G. (2016). Catalytic reduction of CO2 by H2 for synthesis of CO, methanol, and hydrocarbons: challenges and opportunities. Energy Environ. Sci. 9, 62–73. doi:10.1039/c5ee02657a
Puri, A., Loomis, K., Smith, B., Lee, J. H., Yavlovich, A., Heldman, E., et al. (2009). Lipid-based nanoparticles as pharmaceutical drug carriers: from concepts to clinic. Crit. Rev. Ther. Drug Carr. Syst. 26 (6), 523–580. doi:10.1615/critrevtherdrugcarriersyst.v26.i6.10
Qiu, Z., Shu, J., and Tang, D. (2017). Bioresponsive release system for visual fluorescence detection of carcinoembryonic antigen from mesoporous silica nanocontainers mediated optical color on quantum dot-enzyme-impregnated paper. Anal. Chem. 89, 5152–5160. doi:10.1021/acs.analchem.7b00989
Qu, F., Zhu, G., Huang, S., Li, S., Sun, J., Zhang, D., et al. (2006). Controlled release of captopril by regulating the pore size and morphology of ordered mesoporous silica. Micropor Mesopor Mater 92, 1–9. doi:10.1016/j.micromeso.2005.12.004
Radu, D. R., Lai, C. Y., Jeftinija, K., Rowe, E. W., Jeftinija, S., and Lin, V. S. Y. (2004). A polyamidoamine dendrimer-capped mesoporous silica nanosphere-based gene transfection reagent. J. Am. Chem. Soc. 126, 13216–13217. doi:10.1021/ja046275m
Rahikkala, A., Pereira, S. A. P., Figueiredo, P., Passos, M. L. C., Araújo, ARTS, Saraiva, M. L., et al. (2018). Mesoporous silica nanoparticles for targeted and stimuli-responsive delivery of chemotherapeutics: a review. Adv. Biosyst. 2 (1), 1700185. doi:10.1002/adbi.201800020
Raspolli Galletti, A. M., Antonetti, C., Marracci, M., Piccinelli, F., and Tellini, B. (2013). Novel microwave-synthesis of Cu nanoparticles in the absence of any stabilizing agent and their antibacterial and antistatic applications. Appl. Surf. Sci. 280, 610–618. doi:10.1016/j.apsusc.2013.05.035
Rastinehad, A. R., Anastos, H., Wajswol, E., Winoker, J. S., Sfakianos, J. P., Doppalapudi, S. K., et al. (2019). Gold nanoshell-localized photothermal ablation of prostate tumors in a clinical pilot device study. Proc. Natl. Acad. Sci. U. S. A. 116 (37), 18590–18596. doi:10.1073/pnas.1906929116
Reichenbach, H. G., Schwertmann, V., and Cornell, R. M. (1992). Iron oxides in the laboratory—preparation and characterization. Z Pflanzenernähr Bodenkd 155 (3), 254.
Rodríguez-Sánchez, L., Blanco, M. C., and López-Quintela, M. A. (2000). Electrochemical synthesis of silver nanoparticles. J. Phys. Chem. B 104 (41), 9683–9688. doi:10.1021/jp001761r
Rognmo, A. U., Heldal, S., and Fernø, M. A. (2018). Silica nanoparticles to stabilize CO2-foam for improved CO2 utilization: enhanced CO2 storage and oil recovery from mature oil reservoirs. Fuel 216, 621–626. doi:10.1016/j.fuel.2017.11.144
Rojas, S., Gispert, J. D., Menchón, C., Baldoví, H. G., Buaki-Sogo, M., Rocha, M., et al. (2015). Novel methodology for labeling mesoporous silica nanoparticles using the 18F isotope and their in vivo biodistribution by positron emission tomography. J. Nanopart Res. 17, 131. doi:10.1007/s11051-015-2938-0
Rosenbrand, R., Barata, D., Sutthavas, P., Mohren, R., Cillero-Pastor, B., Habibovic, P., et al. (2018). Lipid surface modifications increase mesoporous silica nanoparticle labeling properties in mesenchymal stem cells. Int. J. Nanomed 13, 7711–7725. doi:10.2147/ijn.s182428
Rosenholm, J. M., Peuhu, E., Bate-Eya, L. T., Eriksson, J. E., Sahlgren, C., and Lindén, M. (2010). Cancer-cell-specific induction of apoptosis using mesoporous silica nanoparticles as drug-delivery vectors. Small 6, 1234–1241. doi:10.1002/smll.200902355
Rosenholm, J. M., Peuhu, E., Eriksson, J. E., Sahlgren, C., and Lindén, M. (2009). Targeted intracellular delivery of hydrophobic agents using mesoporous hybrid silica nanoparticles as carrier systems. Nano Lett. 9, 3308–3311. doi:10.1021/nl901589y
Roy, L., Al-Afyouni, M. H., Derosha, D. E., Mondal, B., Dimucci, I. M., Lancaster, K. M., et al. (2019). Reduction of CO2 by a masked two-coordinate cobalt(I) complex and characterization of a proposed oxodicobalt(II) intermediate. Chem. Sci. 10, 918–929. doi:10.1039/c8sc02599a
Sansano, M., De los Reyes, R., Andrés, A., and Heredia, A. (2018). Effect of microwave frying on acrylamide generation, mass transfer, color, and texture in French fries. Food Bioprocess Technol. 11, 1934–1939. doi:10.1007/s11947-018-2144-z
Sarkar, A., Ghosh, S., Chowdhury, S., Pandey, B., and Sil, P. C. (2016). Targeted delivery of quercetin loaded mesoporous silica nanoparticles to the breast cancer cells. Biochim. Biophys. Acta 1860, 2065–2075. doi:10.1016/j.bbagen.2016.07.001
Savage, N., and Diallo, M. S. (2005). Nanomaterials and water purification: opportunities and challenges. J. Nanopart Res. 7, 331–342. doi:10.1007/s11051-005-7523-5
Schanche, J. S. (2003). Microwave synthesis solutions from personal chemistry. Mol. Divers 7, 291–298. doi:10.1023/b:modi.0000006866.38392.f7
Seljak, K. B., Kocbek, P., and Gašperlin, M. (2020). Mesoporous silica nanoparticles as delivery carriers: an overview of drug loading techniques. J. Drug Deliv. Sci. Technol. 59, 101906. doi:10.1016/j.jddst.2020.101906
Serda, R. E., Meraz, I. M., Gu, J., Xia, X., Shen, H., Sun, T., et al. (2015). Mesoporous silicon particles for the presentation of tumour antigens and adjuvant for anti-cancer immunity. U. S. Pat. US8926994B2.
Shaffer, T. M., Harmsen, S., Khwaja, E., Kircher, M. F., Drain, C. M., and Grimm, J. (2016). Stable radiolabeling of sulfur-functionalized silica nanoparticles with copper-64. Nano Lett. 16, 5601–5604. doi:10.1021/acs.nanolett.6b02161
Shankar, S. S., Rai, A., Ahmad, A., and Sastry, M. (2005). Controlling the optical properties of lemongrass extract synthesized gold nanotriangles and potential application in infrared-absorbing optical coatings. Chem. Mat. 17 (3), 566–572. doi:10.1021/cm048292g
Shao, D., Lu, M. M., Zhao, Y. W., Zhang, F., Tan, Y. F., Zheng, X., et al. (2017). The shape effect of magnetic mesoporous silica nanoparticles on endocytosis, biocompatibility, and biodistribution. Acta Biomater. 49, 531–540. doi:10.1016/j.actbio.2016.11.007
Sharma, V. K., Yngard, R. A., and Lin, Y. (2009). Silver nanoparticles: green synthesis and their antimicrobial activities. Adv. Colloid Interf. Sci. 145 (1–2), 83–96. doi:10.1016/j.cis.2008.09.002
Shen, S. C., Ng, W. K., Chia, L., Dong, Y. C., and Tan, R. B. H. (2010). Stabilized amorphous state of ibuprofen by co-spray drying with mesoporous SBA-15 to enhance dissolution properties. J. Pharm. Sci. 99, 1997–2007. doi:10.1002/jps.21967
Shen, S. C., Ng, W. K., Chia, L., Hu, J., and Tan, R. B. H. (2011). Physical state and dissolution of ibuprofen formulated by co-spray drying with mesoporous silica: effect of pore and particle size. Int. J. Pharm. 410, 188–195. doi:10.1016/j.ijpharm.2011.03.018
Shi, S., Wang, W., Liu, L., Wu, S., Wei, Y., and Li, W. (2013). Effect of chitosan/nano-silica coating on the physicochemical characteristics of longan fruit under ambient temperature. J. Food Eng. 118 (1), 125–131. doi:10.1016/j.jfoodeng.2013.03.029
Siddiqui, M. H., and Al-Whaibi, M. H. (2014). Role of nano-SiO2 in germination of tomato (Lycopersicum esculentum Mill.). Saudi J. Biol. Sci. 21 (1), 13–17. doi:10.1016/j.sjbs.2013.04.005
Singh, A., Worku, Z. A., and Van den Mooter, G. (2011). Oral formulation strategies to improve solubility of poorly water-soluble drugs. Expert Opin. Drug Deliv. 8, 1361–1378. doi:10.1517/17425247.2011.606808
Singh, G., Lakhi, K. S., Kim, I. Y., Kim, S., Srivastava, P., Naidu, R., et al. (2017b). Highly efficient method for the synthesis of activated mesoporous biocarbons with extremely high surface area for high-pressure CO2 adsorption. ACS Appl. Mater Interfaces 9, 29782–29793. doi:10.1021/acsami.7b08797
Singh, R. K., Patel, K. D., Leong, K. W., and Kim, H. W. (2017a). Progress in nanotheranostics based on mesoporous silica nanomaterial platforms. ACS Appl. Mater Interfaces 9, 10309–10337. doi:10.1021/acsami.6b16505
Skorupska, E., Jeziorna, A., Paluch, P., and Potrzebowski, M. J. (2014). Ibuprofen in mesopores of mobil crystalline material 41 (MCM-41): a deeper understanding. Mol. Pharm. 11, 1512–1519. doi:10.1021/mp400670f
Slowing, I. I., Vivero-Escoto, J. L., Wu, C. W., and Lin, V. S. Y. (2008). Mesoporous silica nanoparticles as controlled release drug delivery and gene transfection carriers☆. Adv. Drug Deliv. Rev. 60, 1278–1288. doi:10.1016/j.addr.2008.03.012
Slowing, I. I., Wu, C. W., Vivero-Escoto, J. L., and Lin, V. S. Y. (2009). Mesoporous silica nanoparticles for reducing hemolytic activity towards mammalian red blood cells. Small 5 (1), 57–62. doi:10.1002/smll.200800926
Šoltys, M., Kovačík, P., Dammer, O., Beránek, J., and Štěpánek, F. (2019). Effect of solvent selection on drug loading and amorphisation in mesoporous silica particles. Int. J. Pharm. 555, 19–27. doi:10.1016/j.ijpharm.2018.10.075
Song, S. W., Hidajat, K., and Kawi, S. (2005). Functionalized SBA-15 materials as carriers for controlled drug delivery: influence of surface properties on matrix-drug interactions. Langmuir 21, 9568–9575. doi:10.1021/la051167e
Souris, J. S., Lee, C. H., Cheng, S. H., Chen, C. T., Yang, C. S., Ho, J. A. A., et al. (2010). Surface charge-mediated rapid hepatobiliary excretion of mesoporous silica nanoparticles. Biomaterials 31 (21), 5564–5574. doi:10.1016/j.biomaterials.2010.03.048
Stöber, W., Fink, A., and Bohn, E. (1968). Controlled growth of monodisperse silica spheres in the micron size range. J. Colloid Interface Sci. 26, 62–69. doi:10.1016/0021-9797(68)90272-5
Sun, B., Zhen, X., and Jiang, X. (2021b). Development of mesoporous silica-based nanoprobes for optical bioimaging applications. Biomater. Sci. 9, 3603–3620. doi:10.1039/d1bm00204j
Sun, J., Wang, W., and Yue, Q. (2016). Review on microwave-matter interaction fundamentals and efficient microwave-associated heating strategies. Mater. (Basel) 9, 231. doi:10.3390/ma9040231
Sun, M., Zhang, J., Liang, S., Du, Z., Liu, J., Sun, Z., et al. (2021a). Metabolomic characteristics of hepatotoxicity in rats induced by silica nanoparticles. Ecotoxicol. Environ. Saf. 208, 111496. doi:10.1016/j.ecoenv.2020.111496
Sun, Q., You, Q., Wang, J., Liu, L., Wang, Y., Song, Y., et al. (2018). Theranostic nanoplatform: triple-modal imaging-guided synergistic cancer therapy based on liposome-conjugated mesoporous silica nanoparticles. ACS Appl. Mater Interfaces 10 (2), 1963–1975. doi:10.1021/acsami.7b13651
Suriyaprabha, R., Karunakaran, G., Yuvakkumar, R., Prabu, P., Rajendran, V., and Kannan, N. (2012). Growth and physiological responses of maize (Zea mays L.) to porous silica nanoparticles in soil. J. Nanopart Res. 14 (12), 1294. doi:10.1007/s11051-012-1294-6
Suzuki, K., Ikari, K., and Imai, H. (2004). Synthesis of silica nanoparticles having a well-ordered mesostructure using a double surfactant system. J. Am. Chem. Soc. 126, 462–463. doi:10.1021/ja038250d
Tan, A., Eskandar, N. G., Rao, S., and Prestidge, C. A. (2014). First-in-man bioavailability and tolerability studies of a silica–lipid hybrid (Lipoceramic) formulation: a Phase I study with ibuprofen. Drug Deliv. Transl. Res. 4, 212–221. doi:10.1007/s13346-013-0172-9
Tapia, J. F. D., Lee, J. Y., Ooi, R. E. H., Foo, D. C. Y., and Tan, R. R. (2018). A review of optimization and decision-making models for the planning of CO2 capture, utilization, and storage (CCUS) systems. Sustain Prod. Consum. 13, 1–15. doi:10.1016/j.spc.2017.10.001
Tassinari, R., Martinelli, A., Valeri, M., and Maranghi, F. (2021). Amorphous silica nanoparticles induced spleen and liver toxicity after acute intravenous exposure in male and female rats. Toxicol. Ind. Health 37 (6), 328–335. doi:10.1177/07482337211010579
Teng, Z., Wang, S., Su, X., Chen, G., Liu, Y., Luo, Z., et al. (2014). Facile synthesis of yolk-shell structured inorganic-organic hybrid spheres with ordered radial mesochannels. Adv. Mat. 26 (22), 3741–3747. doi:10.1002/adma.201400136
Thomas, M. J. K., Slipper, I., Walunj, A., Jain, A., Favretto, M. E., Kallinteri, P., et al. (2010). Inclusion of poorly soluble drugs in highly ordered mesoporous silica nanoparticles. Int. J. Pharm. 387, 272–277. doi:10.1016/j.ijpharm.2009.12.023
Tomé, L. C., and Marrucho, I. M. (2016). Ionic liquid-based materials: a platform to design engineered CO2 separation membranes. Chem. Soc. Rev. 45, 2785–2824. doi:10.1039/c5cs00510h
Tompsett, G. A., Conner, W. C., and Yngvesson, K. S. (2006). Microwave synthesis of nanoporous materials. ChemPhysChem 7, 296–319. doi:10.1002/cphc.200500449
Torney, F., Trewyn, B. G., Lin, V. S. Y., and Wang, K. (2007). Mesoporous silica nanoparticles deliver DNA and chemicals into plants. Nat. Nanotechnol. 2 (5), 295–300. doi:10.1038/nnano.2007.108
Tourné-Péteilh, C., Brunel, D., Bégu, S., Chiche, B., Fajula, F., Lerner, D. A., et al. (2003). Synthesis and characterisation of ibuprofen-anchored MCM-41 silica and silica gel. New J. Chem. 27, 1415–1418. doi:10.1039/b307046h
Traitel, T., Cohen, Y., and Kost, J. (2000). Characterization of glucose-sensitive insulin release systems in simulated in vivo conditions. Biomaterials 21 (16), 1679–1687. doi:10.1016/s0142-9612(00)00050-8
Trewyn, B. G., Nieweg, J. A., Zhao, Y., and Lin, V. S. Y. (2008). Biocompatible mesoporous silica nanoparticles with different morphologies for animal cell membrane penetration. Chem. Eng. J. 137 (1), 23–29. doi:10.1016/j.cej.2007.09.045
Trzeciak, K., Chotera-Ouda, A., Bak-Sypien, I. I., and Potrzebowski, M. J. (2021a). Mesoporous silica particles as drug delivery systems—the state of the art in loading methods and the recent progress in analytical techniques for monitoring these processes. Pharmaceutics 13 (950), 950. doi:10.3390/pharmaceutics13070950
Trzeciak, K., Kaźmierski, S., Druzbicki, K., and Potrzebowski, M. J. (2021b). Mapping of guest localization in mesoporous silica particles by solid-state NMR and ab initio modeling: new insights into benzoic acid and p-fluorobenzoic acid embedded in MCM-41 via ball milling. J. Phys. Chem. C 125, 10096–10109. doi:10.1021/acs.jpcc.1c01675
Trzeciak, K., Kaźmierski, S., Wielgus, E., and Potrzebowski, M. J. (2020). DiSupLo—new extremely easy and efficient method for loading of active pharmaceutical ingredients into the pores of MCM-41 mesoporous silica particles. Micropor Mesopor Mater 308, 110506. doi:10.1016/j.micromeso.2020.110506
Tsuji, M. (2017). Microwave-assisted synthesis of metallic nanomaterials in liquid phase. ChemistrySelect 2, 805–819. doi:10.1002/slct.201700011
Tuna, B. G., Durdabak, D. B., Ercan, M. K., Dogan, S., Kavruk, M., Dursun, A. D., et al. (2022). Detection of viruses by probe-gated silica nanoparticles directly from swab samples. Talanta 246, 123429. doi:10.1016/j.talanta.2022.123429
Uejo, F., Limwikrant, W., Moribe, K., and Yamamoto, K. (2013). Dissolution improvement of fenofibrate by melting inclusion in mesoporous silica. Asian J. Pharm. Sci. 8, 329–335. doi:10.1016/j.ajps.2013.11.001
Vadia, N., and Rajput, S. (2012). Study on formulation variables of methotrexate-loaded mesoporous MCM-41 nanoparticles for dissolution enhancement. Eur. J. Pharm. Sci. 45, 8–18. doi:10.1016/j.ejps.2011.10.016
Vallet-Regi, M., Ramila, A., del Real, R. P., and Perez-Pariente, J. (2001). A new property of MCM-41: drug delivery system. Chem. Mat. 13 (2), 308–311. doi:10.1021/cm0011559
Van Emden, H. F., Ball, S. L., and Rao, M. R. (1988). “Pest, disease and weed problems in pea, lentil, faba bean and chickpea,” in World crops: cool season food legumes (Dordrecht: Springer), 519–534.
van Rijt, S. H., Bolukbas, D. A., Argyo, C., Datz, S., Lindner, M., Eickelberg, O., et al. (2015). Protease-mediated release of chemotherapeutics from mesoporous silica nanoparticles to ex vivo human and mouse lung tumors. ACS Nano 9 (3), 2377–2389. doi:10.1021/nn5070343
Van Speybroeck, M., Barillaro, V., Thi, T. D., Mellaerts, R., Martens, J., Van Humbeeck, J., et al. (2009). Ordered mesoporous silica material SBA-15: a broad-spectrum formulation platform for poorly soluble drugs. J. Pharm. Sci. 98, 2648–2658. doi:10.1002/jps.21638
Van Speybroeck, M., Mellaerts, R., Thi, T. D., Martens, J. A., Van Humbeeck, J., Annaert, P., et al. (2011). Preventing release in the acidic environment of the stomach via occlusion in ordered mesoporous silica enhances the absorption of poorly soluble weakly acidic drugs. J. Pharm. Sci. 100, 4864–4876. doi:10.1002/jps.22703
Varache, M., Bezverkhyy, I., Saviot, L., Bouyer, F., Baras, F., and Bouyer, F. (2015). Optimization of MCM-41 type silica nanoparticles for biological applications: control of size and absence of aggregation and cell cytotoxicity. J. Non-Cryst Solids 408, 87–97. doi:10.1016/j.jnoncrysol.2014.10.020
Varga, N., Benkő, M., Sebők, D., Bohus, G., Janovák, L., and Dékány, I. (2015). Mesoporous silica core–shell composite functionalized with polyelectrolytes for drug delivery. Micropor Mesopor Mater 213 (C), 134–141. doi:10.1016/j.micromeso.2015.02.008
Vialpando, M., Aerts, A., Persoons, J., Martens, J., and Van Den Mooter, G. (2011). Evaluation of ordered mesoporous silica as a carrier for poorly soluble drugs: influence of pressure on the structure and drug release. J. Pharm. Sci. 100, 3411–3420. doi:10.1002/jps.22535
Vivero-Escoto, J. L., Slowing, I. I., Trewyn, B. G., and Lin, V. S. Y. (2010a). Mesoporous silica nanoparticles for intracellular controlled drug delivery. Small 6, 1952–1967. doi:10.1002/smll.200901789
Vivero-Escoto, J. L., Slowing, I. I., Trewyn, B. G., and Lin, V. S. Y. (2010b). Mesoporous silica nanoparticles for intracellular controlled drug delivery. Small 6, 1952–1967. doi:10.1002/smll.200901789
Vivero-Escoto, J. L., Slowing, I. I., Wu, C. W., and Lin, V. S. Y. (2009). Photoinduced intracellular controlled release drug delivery in human cells by gold-capped mesoporous silica nanosphere. J. Am. Chem. Soc. 131 (10), 3462–3463. doi:10.1021/ja900025f
von, M. G., Ren, Y., Park, J. H., Min, D. H., Kotamraju, V. R., Jayakumar, J., et al. (2008). In vivo tumor cell targeting with “click” nanoparticles. Bioconjug Chem. 19 (8), 1570–1578. doi:10.1021/bc800077y
Wan, M. M., Li, Y. Y., Yang, T., Zhang, T., Sun, X. D., and Zhu, J. H. (2016). In situ loading of drugs into mesoporous silica SBA-15. Chem. Eur. J. 22, 6294–6301. doi:10.1002/chem.201504532
Wang, D. P., Wang, Z. J., Zhao, R., Lin, C. X., Sun, Q. Y., Yan, C. P., et al. (2020). Silica nanomaterials induce organ injuries by Ca2+-ROS-initiated disruption of the endothelial barrier and triggering intravascular coagulation. Part Fibre Toxicol. 17 (1), 12. doi:10.1186/s12989-020-00340-8
Wang, F., Li, Z., Liu, D., and Wang, G. (2014). Synthesis of magnetic mesoporous silica composites via a modified Stöber approach. J. Porous Mater 21, 513–519. doi:10.1007/s10934-014-9798-3
Wang, G., Dong, J., Yuan, T., Zhang, J., Wang, L., and Wang, H. (2016b). Visible light and pH-responsive polymer-coated mesoporous silica nanohybrids for controlled release. Macromol. Biosci. 16 (7), 990–994. doi:10.1002/mabi.201600008
Wang, L. H., Chen, X., Xu, H., Zhang, L. L., Hu, J., Zhang, M. J., et al. (2013b). A novel strategy to design sustained-release poorly water-soluble drug mesoporous silica microparticles based on supercritical fluid technique. Int. J. Pharm. 454, 135–142. doi:10.1016/j.ijpharm.2013.07.027
Wang, N., Cheng, X., Li, N., Wang, H., and Chen, H. (2019a). Nanocarriers and their loading strategies. Adv. Healthc. Mater 8, e1801002–e1801012. doi:10.1002/adhm.201801002
Wang, W. H., Himeda, Y., Muckerman, J. T., Manbeck, G. F., and Fujita, E. (2015d). CO2 hydrogenation to formate and methanol as an alternative to photo- and electrochemical CO2 reduction. Chem. Rev. 115, 12936–12973. doi:10.1021/acs.chemrev.5b00197
Wang, X., Wang, Z., Zhuang, T. T., Dinh, C. T., Li, J., Nam, D. H., et al. (2019b). Efficient upgrading of CO to C3 fuel using asymmetric C-C coupling active sites. Nat. Commun. 10 (1), 5186. doi:10.1038/s41467-019-13190-6
Wang, Y., Han, N., Zhao, Q., Bai, L., Li, J., Jiang, T., et al. (2015c). Redox-responsive mesoporous silica as carriers for controlled drug delivery: a comparative study based on silica and PEG gatekeepers. Eur. J. Pharm. Sci. 72, 12–20. doi:10.1016/j.ejps.2015.02.008
Wang, Y., Sun, Y., Wang, J., Yang, Y., Li, Y., Yuan, Y., et al. (2016a). Charge-reversal APTES-modified mesoporous silica nanoparticles with high drug loading and release controllability. ACS Appl. Mater Interfaces 8, 17166–17175. doi:10.1021/acsami.6b05370
Wang, Y., Zhao, Q., Han, N., Bai, L., Li, J., Liu, J., et al. (2015a). Mesoporous silica nanoparticles in drug delivery and biomedical applications. Nanomedicine (NBM). 11, 313–327. doi:10.1016/j.nano.2014.09.014
Wang, Y., Zhao, Q., Han, N., Bai, L., Li, J., Liu, J., et al. (2015b). Mesoporous silica nanoparticles in drug delivery and biomedical applications. Nanomedicine. 11, 313–327. doi:10.1016/j.nano.2014.09.014
Wang, Y., Zhao, Q., Hu, Y., Sun, L., Bai, L., Jiang, T., et al. (2013a). Ordered nanoporous silica as carriers for improved delivery of water-insoluble drugs: a comparative study between three-dimensional and two-dimensional macroporous silica. Int. J. Nanomed 8, 4015–4031. doi:10.2147/ijn.s52605
Waters, L. J., Hussain, T., Parkes, G., Hanrahan, J. P., and Tobin, J. M. (2013). Inclusion of fenofibrate in a series of mesoporous silicas using microwave irradiation. Eur. J. Pharm. Biopharm. 85, 936–941. doi:10.1016/j.ejpb.2013.08.002
Wee, J. H. (2013). A review on carbon dioxide capture and storage technology using coal fly ash. Appl. Energy 106, 143–151. doi:10.1016/j.apenergy.2013.01.062
Wei, R., Wang, P., Zhang, G., Wang, N., and Zheng, T. (2020). Microwave-responsive catalysts for wastewater treatment: a review. Chem. Eng. J. 382, 122781. doi:10.1016/j.cej.2019.122781
Wei, Y., Quan, L., Zhou, C., and Zhan, Q. (2018). Factors relating to the biodistribution and clearance of nanoparticles and their effects on in vivo application. Nanomedicine. 13 (12), 1495–1512. doi:10.2217/nnm-2018-0040
Widenmeyer, M., and Anwander, R. (2002). Pore size control of highly ordered mesoporous silica MCM-48. Chem. Mat. 14, 1827–1831. doi:10.1021/cm011273b
Willart, J. F., and Descamps, M. (2008). Solid-state amorphization of pharmaceuticals. Mol. Pharm. 5, 905–920. doi:10.1021/mp800092t
Wong, P. T., and Choi, S. K. (2015). Mechanisms of drug release in nanotherapeutic delivery systems. Chem. Rev. 115, 3388–3432. doi:10.1021/cr5004634
Wu, C., Zhao, Z., Zhao, Y., Hao, Y., Liu, Y., and Liu, C. (2014). Preparation of a push–pull osmotic pump of felodipine solubilized by mesoporous silica nanoparticles with a core–shell structure. Int. J. Pharm. 475, 298–305. doi:10.1016/j.ijpharm.2014.08.033
Wu, H., Zhang, S., Zhang, J., Liu, G., Shi, J., Zhang, L., et al. (2011b). A hollow-core, magnetic, and mesoporous double-shell nanostructure: in situ decomposition/reduction synthesis, bioimaging, and drug-delivery properties. Adv. Funct. Mater 21, 1850–1862. doi:10.1002/adfm.201002337
Wu, K. C. W., and Yamauchi, Y. (2012). Controlling physical features of mesoporous silica nanoparticles (MSNs) for emerging applications. J. Mater Chem. 22, 1251–1256. doi:10.1039/c1jm13811a
Wu, S. H., Hung, Y., and Mou, C. Y. (2011a). Mesoporous silica nanoparticles as nanocarriers. Chem. Commun. 47, 9972–9985. doi:10.1039/c1cc11760b
Wu, S. H., Mou, C. Y., and Lin, H. P. (2013). Synthesis of mesoporous silica nanoparticles. Chem. Soc. Rev. 42, 3862–3875. doi:10.1039/c3cs35405a
Xie, X., Li, F., Zhang, H., Lu, Y., Lian, S., Lin, H., et al. (2016). EpCAM aptamer-functionalized mesoporous silica nanoparticles for efficient colon cancer cell-targeted drug delivery. Eur. J. Pharm. Sci. 83, 28–35. doi:10.1016/j.ejps.2015.12.014
Xiong, L., Du, X., Shi, B., Bi, J., Kleitz, F., and Qiao, S. Z. (2015). Tunable stellate mesoporous silica nanoparticles for intracellular drug delivery. J. Mater Chem. B 3, 1712–1721. doi:10.1039/c4tb01601g
Xu, B., Li, S., Shi, R., and Liu, H. (2023). Multifunctional mesoporous silica nanoparticles for biomedical applications. Signal Transduct. Target Ther. 8 (435), 435. doi:10.1038/s41392-023-01654-7
Yamada, H., Urata, C., Ujiie, H., Yamauchi, Y., and Kuroda, K. (2013). Preparation of aqueous colloidal mesostructured and mesoporous silica nanoparticles with controlled particle size in a very wide range from 20 nm to 700 nm. Nanoscale 5, 6145–6153. doi:10.1039/c3nr00334e
Yang, G., Sun, X., Liu, J., Feng, L., and Liu, Z. (2016). Light-responsive, singlet oxygen-triggered on-demand drug release from photosensitizer-doped mesoporous silica nanorods for cancer combination therapy. Adv. Funct. Mater 26 (26), 4722–4732. doi:10.1002/adfm.201600722
Yang, L., Wang, J., Yang, S., Lu, Q., Li, P., and Li, N. (2019). Rod-shape MSN@MoS2 nanoplatform for FL/MSOT/CT imaging-guided photothermal and photodynamic therapy. Theranostics 9 (14), 3992–4005. doi:10.7150/thno.32715
Yang, X., Feng, L., Zhang, Y., Hu, H., Shi, Y., Liang, S., et al. (2018). Co-exposure of silica nanoparticles and methylmercury induced cardiac toxicity in vitro and in vivo. Sci. Total Environ. 631–632, 811–821. doi:10.1016/j.scitotenv.2018.03.107
Yao, X., Niu, X., Ma, K., Huang, P., Grothe, J., Kaskel, S., et al. (2017). Graphene quantum dots-capped magnetic mesoporous silica nanoparticles as a multifunctional platform for controlled drug delivery, magnetic hyperthermia, and photothermal therapy. Small 13 (2), 1602225. doi:10.1002/smll.201602225
Yokoi, T., Karouji, T., Ohta, S., Kondo, J. N., and Tatsumi, T. (2010). Synthesis of mesoporous silica nanospheres promoted by basic amino acids and their catalytic application. Chem. Mat. 22, 3900–3908. doi:10.1021/cm9037846
Yousefvand, H., and Jafari, A. (2015). Enhanced oil recovery using polymer/nanosilica. Procedia Mater Sci. 11, 565–570. doi:10.1016/j.mspro.2015.11.068
Youssif, M. I., El-Maghraby, R. M., Saleh, S. M., and Elgibaly, A. (2018). Silica nanofluid flooding for enhanced oil recovery in sandstone rocks. Egypt J. Pet. 27 (1), 105–110. doi:10.1016/j.ejpe.2017.01.006
Yu, L., Lin, H., Lu, X., and Chen, Y. (2018). Multifunctional mesoporous silica nanoprobes: material chemistry–based fabrication and bio-imaging functionality. Adv. Ther. 1, 1800078. doi:10.1002/adtp.201800078
Yu, M., Zhou, L., Zhang, J., Yuan, P., Thorn, P., Gu, W., et al. (2012). A simple approach to prepare monodisperse mesoporous silica nanospheres with adjustable sizes. J. Colloid Interface Sci. 376, 67–75. doi:10.1016/j.jcis.2012.03.014
Yu, Y., Zhu, T., Li, Y., Jing, L., Yang, M., Li, Y., et al. (2019). Repeated intravenous administration of silica nanoparticles induces pulmonary inflammation and collagen accumulation via JAK2/STAT3 and TGF-β/Smad3 pathways in vivo. Int. J. Nanomedicine 14, 7237–7247. doi:10.2147/ijn.s209458
Yuan, L., Chen, W., Hu, J., Zhang, J. Z., and Yang, D. (2013). Mechanistic study of the covalent loading of paclitaxel via disulfide linkers for controlled drug release. Langmuir 29, 734–743. doi:10.1021/la304324r
Yuan, Y., Zhou, R., Li, T., Qu, S., Bai, H., Liang, J., et al. (2021). Enriched Au nanoclusters with mesoporous silica nanoparticles for improved fluorescence/computed tomography dual-modal imaging. Cell Prolif. 54, e13008. doi:10.1111/cpr.13008
Yuan, Y. Y., Mao, C. Q., Du, X. J., Du, J. Z., Wang, F., and Wang, J. (2012). Surface charge switchable nanoparticles based on zwitterionic polymer for enhanced drug delivery to tumor. Adv. Mater 24 (40), 5476–5480. doi:10.1002/adma.201202296
Zanoni, D. K., Stambuk, H. E., Madajewski, B., Montero, P. H., Matsuura, D., Busam, K. J., et al. (2021). Use of ultrasmall core-shell fluorescent silica nanoparticles for image-guided sentinel lymph node biopsy in head and neck melanoma: a nonrandomized clinical trial. JAMA Netw. Open 4, e211936. doi:10.1001/jamanetworkopen.2021.1936
Zhang, L., Qiao, S., Jin, Y., Yang, H., Budihartono, S., Stahr, F., et al. (2008). Fabrication and size-selective bioseparation of magnetic silica nanospheres with highly ordered periodic mesostructure. Adv. Funct. Mater 18, 3203–3212. doi:10.1002/adfm.200800363
Zhang, Q., Liu, F., Nguyen, K. T., Ma, X., Wang, X., Xing, B., et al. (2012). Multifunctional mesoporous silica nanoparticles for cancer-targeted and controlled drug delivery. Adv. Funct. Mater 22 (24), 5144–5156. doi:10.1002/adfm.201201316
Zhang, Y., Hou, Z., Ge, Y., Deng, K., Liu, B., Li, X., et al. (2015). DNA-hybrid-gated photothermal mesoporous silica nanoparticles for NIR-responsive and aptamer-targeted drug delivery. ACS Appl. Mater Interfaces 7 (37), 20696–20706. doi:10.1021/acsami.5b05522
Zhang, Y., Lou, J., Williams, G. R., Ye, Y., Ren, D., Shi, A., et al. (2022). Cu2+-chelating mesoporous silica nanoparticles for synergistic chemotherapy/chemodynamic therapy. Pharmaceutics 14 (6), 1200. doi:10.3390/pharmaceutics14061200
Zhang, Y., Yue, Q., Zagho, M. M., Zhang, J., Elzatahry, A. A., Jiang, Y., et al. (2019). Core–shell magnetic mesoporous silica microspheres with large mesopores for enzyme immobilization in biocatalysis. ACS Appl. Mater Interfaces 11 (10), 10356–10363. doi:10.1021/acsami.8b18721
Zhang, Y., Zhi, Z., Jiang, T., Zhang, J., Wang, Z., and Wang, S. (2010). Spherical mesoporous silica nanoparticles for loading and release of the poorly water-soluble drug telmisartan. J. Control Release 145, 257–263. doi:10.1016/j.jconrel.2010.04.029
Zhang, Y. N., Poon, W., Tavares, A. J., McGilvray, I. D., and Chan, W. C. W. (2016). Nanoparticle-liver interactions: cellular uptake and hepatobiliary elimination. J. Control Release 240, 332–348. doi:10.1016/j.jconrel.2016.01.020
Zhao, Q., Wang, C., Liu, Y., Wang, J., Gao, Y., Zhang, X., et al. (2014). PEGylated mesoporous silica as a redox-responsive drug delivery system for loading thiol-containing drugs. Int. J. Pharm. 477 (1–2), 613–622. doi:10.1016/j.ijpharm.2014.10.056
Zheng, F. F., Zhang, P. H., Xi, Y., Chen, J. J., Li, L. L., and Zhu, J. J. (2015). Aptamer/graphene quantum dots nanocomposite capped fluorescent mesoporous silica nanoparticles for intracellular drug delivery and real-time monitoring of drug release. Anal. Chem. 87 (23), 11739–11745. doi:10.1021/acs.analchem.5b03131
Zheng, X., Feng, S., Wang, X., Shi, Z., Mao, Y., Zhao, Q., et al. (2019). MSNCs and MgO-MSNCs as drug delivery systems to control the adsorption kinetics and release rate of indometacin. Asian J. Pharm. Sci. 14, 275–286. doi:10.1016/j.ajps.2018.08.004
Zhou, C., Weir, M. D., Zhang, K., Deng, D., Cheng, L., and Xu, H. H. K. (2013). Synthesis of new antibacterial quaternary ammonium monomer for incorporation into CaP nanocomposite. Dent. Mater 29, 859–870. doi:10.1016/j.dental.2013.05.005
Zhu, A., Jiao, T., Ali, S., Xu, Y., Ouyang, Q., and Chen, Q. (2021). SERS sensors based on aptamer-gated mesoporous silica nanoparticles for quantitative detection of Staphylococcus aureus with signal molecular release. Anal. Chem. 93, 9788–9796. doi:10.1021/acs.analchem.1c01280
Zhu, Y. F., Ikoma, T., Hanagata, N., and Kaskel, S. (2010). Rattle-type Fe3O4@SiO2 hollow mesoporous spheres as carriers for hydrophobic anticancer drugs. Small 6, 471–478. doi:10.1002/smll.200901403
Zid, L., Zelenak, V., Almasi, M., Zelenakova, A., Szucsova, J., Bednarcik, J., et al. (2020). Mesoporous silica as a drug delivery system for naproxen: influence of surface functionalization. Molecules 25, 4722. doi:10.3390/molecules25204722
Zou, R., Gao, Y., Zhang, Y., Jiao, J., Wong, K. L., and Wang, J. (2021). 68Ga-labeled magnetic-NIR persistent luminescent hybrid mesoporous nanoparticles for multimodal imaging-guided chemotherapy and photodynamic therapy. ACS Appl. Mater Interfaces 13, 9667–9680. doi:10.1021/acsami.0c21623
Zou, Z., He, D., Cai, L., He, X., Wang, K., Yang, X., et al. (2016). Alizarin complexone functionalized mesoporous silica nanoparticles: a smart system integrating glucose-responsive double-drugs release and real-time monitoring capabilities. ACS Appl. Mater Interfaces 8 (13), 8358–8366. doi:10.1021/acsami.5b12576
Keywords: MSN (mesoporous silica nanoparticle), HMS (hollow mesoporous silica), NP (nanoparticles), sol gel, drug delivery
Citation: Fatima R, Katiyar P and Kushwaha K (2025) Recent advances in mesoporous silica nanoparticle: synthesis, drug loading, release mechanisms, and diverse applications. Front. Nanotechnol. 7:1564188. doi: 10.3389/fnano.2025.1564188
Received: 21 January 2025; Accepted: 27 February 2025;
Published: 18 March 2025.
Edited by:
Maqusood Ahamed, King Saud University, Saudi ArabiaReviewed by:
Parviz Vahedi, Maragheh University of Medical Sciences, IranCopyright © 2025 Fatima, Katiyar and Kushwaha. This is an open-access article distributed under the terms of the Creative Commons Attribution License (CC BY). The use, distribution or reproduction in other forums is permitted, provided the original author(s) and the copyright owner(s) are credited and that the original publication in this journal is cited, in accordance with accepted academic practice. No use, distribution or reproduction is permitted which does not comply with these terms.
*Correspondence: Kalpana Kushwaha, a2FscGFuYWt1c2h3YWhhQGNzam11LmFjLmlu
Disclaimer: All claims expressed in this article are solely those of the authors and do not necessarily represent those of their affiliated organizations, or those of the publisher, the editors and the reviewers. Any product that may be evaluated in this article or claim that may be made by its manufacturer is not guaranteed or endorsed by the publisher.
Research integrity at Frontiers
Learn more about the work of our research integrity team to safeguard the quality of each article we publish.