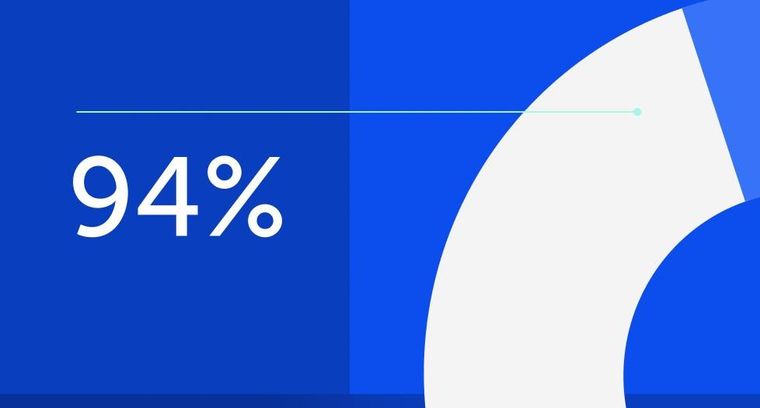
94% of researchers rate our articles as excellent or good
Learn more about the work of our research integrity team to safeguard the quality of each article we publish.
Find out more
REVIEW article
Front. Nanotechnol., 04 April 2025
Sec. Nanodevices
Volume 7 - 2025 | https://doi.org/10.3389/fnano.2025.1545792
Plant disease outbreaks are raising concerns about global food security. Pathogenic evolution and continuous climate changes increase the threat to agriculture and necessitate disease surveillance. To prevent future outbreaks and maintain agricultural sustainability advanced tools are required. Nowadays various types of nanobiosensors such as electrochemical, piezoelectric, thermal, optical, and Fluorescence resonance energy transfer (FRET)-based biosensors are used to predict disease-associated pathogens, toxins, and abiotic stress. Nanobiosensors, provide quick detection of diseases and may protect from future pandemics as they overcome the time dependency of traditional methods and provide real-time monitoring. The incorporation of various nanoparticles with biosensors such as chitosan nanoparticles, silver nanoparticles (AgNPs), gold nanoparticles (AuNPs), multiwalled carbon nanotubes (MWCNTs), and graphene oxide, etc., facilitates the precise detection of various toxins, pesticides, and disease-causing pathogens in plants. Furthermore, the integration of portable devices and artificial intelligence (AI) increases their practical application in agricultural monitoring. Despite their promising aspect, issues with sensor stability, large-scale development, and cost-effectiveness also need to be addressed. Future studies are more concerned with improving durability, multiplex detection ability, and user-friendly field application. To enhance agricultural output, it is necessary to develop an early disease diagnosis approach that is heavily dependent on the ongoing development of cost-effective nanobiosensors. This review focuses on the recent studies of various nanobiosensors development and their operation mechanism for pathogen detection. Additionally, challenges associated with the worldwide acceptance of nano biosensors are also addressed. Overall, nanobiosensors are new-edge technology that enhances plant disease management strategies and risk mitigation in food security.
A nanobiosensor uses nanostructures to detect gases, electric fields, biological agents, chemicals, heat, light, etc. The nanostructure combined with biological recognition elements to provide pathogen, biomolecule, or environmental signal detection with a high degree of sensitivity and specificity. It provides a quick, reversible, and non-destructive assay of target molecules straight from the sample. The nanomaterials used in sensors greatly enhance the system’s sensitivity. The portion of the apparatus in biosensors that binds to the analyte and allows for its precise detection is a biological component such as an enzyme, DNA strand, antibody, or whole cell (Huang et al., 2021). The way biological component, or bioreceptor layer, is attached to the transducer is a major factor in biosensor performance. The primary objective is to enhance the biological component’s stability while forging a strong bond between it and the sensory surface (Zuo et al., 2015; Jiang et al., 2018). From an evolutionary perspective, the classic idea of biosensing, which is innate in many living forms, is employed to defend against hostile situations. As per the IUPAC (International Union of Pure and Applied Chemistry), a biosensor can be described as “a precise biochemical reaction arbitrated by the immune system, isolated enzymes, organelles, or tissues for the detection of chemical compounds through the sensing of optical, thermal, or electrical signals” biosensors are the combination of various components that collectively sense the analyte (Cruz et al., 2014; Zhai et al., 2019). A biosensor is designed to sense minute changes in the biome and well-format interpretable data by the processing of electrical impulses in a readable format (Panchuk et al., 2018; Coroş et al., 2019; Villa et al., 2020). Owing to its number of benefits, such as precise infection detection, food standard evaluation, and other uses, biosensors have started to be utilized for environmental purposes. Biosensors have also been adopted for various therapeutic purposes, such as identifying biomarkers, viruses, tumors, and pollutants to diagnose early symptoms of illnesses (Tsai et al., 2018; Haleem et al., 2021). Yet, their characteristics include low production costs, mobility, fast reaction times, and the capacity to test biomaterials with exactitude and sensitivity even at a small scale. Nanobiosensors have gained increased importance in combating emerging plant diseases, enabling rapid and precise disease detection for agricultural sustainability and global food security (Barry and O’Riordan, 2016; Yousefi et al., 2021). Traditional diagnostic techniques are frequently cumbersome and cannot be implemented in the field. Handheld analyzers, smartphone-integrated systems, and lab-on-a-chip platforms are new-age examples of future diagnostic technologies that provide very accurate real-time pathogen detection on-site. These technologies are based on immunoassays, biosensors, nucleic acid amplification and with the incorporation of AI enhance disease surveillance. Portable diagnostics is becoming an essential tool in precision agriculture due to advancements in multiplex detection and nanotechnology, which are increasing their efficacy, despite issues with sensitivity and regulatory approval (Yadav and Yadav, 2025).
Biosensors, integrated receptor-transducer devices, acquired prominent attention over the last decade for their applications in medication delivery, sustainable agriculture, healthcare diagnosis, and environmental monitoring (Naresh and Lee, 2021). The electronic component of the biosensor finds, logs, and sends information about the physiological state or the presence of various biochemical materials within the ecosystem. These sensors can also detect pH levels, dangerous chemical concentrations, and even minute quantities of certain illnesses. They are available in various forms and sizes. The components of a conventional biosensor include an analyte, electronics, a bioreceptor, a transducer, and a display (Hammond et al., 2016). Biosensors can be classified based on transducer and biorecognition elements, as mentioned in Figure 1.
Figure 1. Represents the classification of biosensors broadly categorized based on biorecognition element and transducer.
To provide long-term, economical, as well as eco-friendly monitoring of plant health and environmental conditions, a sophisticated biosensor must be developed. It promotes sustainable farming methods, improves biocompatibility, and reduces waste. The combination of transition metal oxides coated with conductive polymers (CPs) presents a promising avenue for the innovation of sophisticated biosensors with enhanced capabilities. Several approaches, such as chemical, biological, and electrochemical ones, have been used to synthesize CPs (Ramanavicius and Ramanavicius, 2020).
Optimising CP synthesis has been a major area of study to design a robust and trustworthy biosensor. Several studies highlights the importance of architecture for the selection of the right monomer when constructing a CP layer with precise sensing capabilities. CPs are unlike other materials because of their unique features, namely, their improved electrical conductivity and decreased ionization potential, which are caused by the presence of delocalized π-electrons throughout the polymer chain backbone, and other intriguing features (Vaitkuviene et al., 2013; Naveen et al., 2017; Zamani et al., 2019). The glucose biosensor known as redox enzyme-glucose oxidase often incorporates CPs for the recognition of biological elements in its architecture. According to several studies, GOx sensors may function as a biological catalyst in the production of different CPs, such as polyaniline, polythiophene, and polypyrrole (Krikstolaityte et al., 2014; German et al., 2017). Lately, two-dimensional materials with good metallic conductivity, outstanding semiconducting characteristics, or their combination have been utilized for building well-organized biosensors. Biosensors, wearable electronics, and biofuel cells may all be designed and produced using these special property combinations (Deshmukh et al., 2020). Biosensors play a transformative role in modern agriculture and enhanced their effectiveness with miniaturization, and nanomaterial integration (Gangopadhyay et al., 2024; Ali et al., 2024), the examples of various biosensors and nanomaterial use in the architectures with their role in agriculture are mentioned in Table 1.
Monitoring crop health requires a reliable method for timely detection of plant pathogens for efficient crop management practices, facilitating disease deduction. Various methods are utilized to confirm crop disease such as serological assay, visual examination of symptoms, and DNA-DNA-based pathogen detection. Due to their lower reliability in early recognition and on-field detection advent of nanotools enables the effective development of nanosensors to overcome several obstacles. Significant advancements in the area of nanobiosensesors development include electrochemical biosensors, piezoelectric nanobiosensors, nanomechanical biosensors, optical nanobiosensors, electrochemical nose (eNose), and electronic tongue (e-tongue), nano-barcodes, magnetic nanobiosensors, calorimetric nanobiosensors, etc., (Dar et al., 2020). These all biosensors use various techniques depending on biosensors as piezoelectric biosensors utilize quartz crystal (QZ) and their efficacy can be enhanced by the use of appropriate nanomaterial for instance gold, coated QZ crystals enhance the surface area and provide more antigen-antibody complex. Experimental evidence proved that these biosensors are similarly sensitive to enzyme-linked immunosorbent assay (ELISA) (Choudhary et al., 2010). However, the sensitivity and efficacy of nano biosensors are also dependent on the types of nanostructures they have employed including nanotubes, nanopores, nanoparticles, nanowires, and nanocomposites. Simultaneously, the use of advanced electrochemical techniques provides effective decomposition power with high sensitivity (Huang et al., 2021). In agricultural practices, electrochemical nanobiosensors provide effective detection of pesticides containing hazardous chemicals such as 4-nitrophenol (4-NP) in tomato samples (Trang et al., 2022).
Additionally, bacterial lux-biosensors are used to assess soil toxicity from pesticides and fertilizers, and based on Escherichia coli MG1655 and Vibrio aquamarinus VKPM B-11245 highlighted effectiveness of biosensors in agrochemical toxicity detection. Several types of nanoparticles can be used in electrochemical biosensors as AuNPs, AgNPs, magnetic nanomaterial, and CNTs, which offer various features to the nanosensor for instance, AuNPs reduce the resistance of electron transfer with unique optical properties, and AgNPs provide high reflectivity with enhanced thermal and electric conductivity on the other hand magnetic nanoparticles are composed of magnetic nanomaterials such as cobalt (Co), Iron (Fe) and Nickel (Ni), the distinct chemical property of magnetic nanoparticles shorter the experiment time out of the iron oxide is implemented in the biological analysis. CNTs are a well-known nanostructure for their higher conductivity with significant propensity (Ansari et al., 2020). Electrochemical biosensors may also be employed for the detection of the phytohormones and some modifications in them may enhance the precise real-time evaluation (He et al., 2020).
Nanobiosensors are also applicable for precise detection of heavy metal toxicity, for instance, selenium nanoparticles (SeNPs) based biosensors produced biogenically by the bacteria Stenotrophomonas aidaminiphila. SeNPs are employed to identify heavy metal pollution, particularly in agricultural settings. A face-centered cubic (FCC) crystalline structure with particle sizes ranging from 35 to 40 nm is shown by SeNP characterization, which is supported by methods such as transmission electron microscopy (TEM), X-ray diffraction (XRD), and UV-Visual spectroscopy. Fluorescence intensity measurements are used to assess the sensor’s sensitivity to several heavy metals, including iron (Fe), zinc (Zn), cadmium (Cd), arsenic (As), and mercury (Hg). When these metals are present, the biosensor exhibits a noticeable decrease in fluorescence; the largest inhibitions are seen for arsenic (49%) and mercury (60%) and indicate a higher sensitivity to more hazardous metals (Ahmed et al., 2020). Furthermore, label-free detectors are composed of a significantly sensitive transducer which facilitates the precise detection of numerous DNA–RNA-based biomarkers. An optical biosensor relies on the principle that, at a particular wavelength, it detects the presence of analyte by a color change or can be identified by the photon’s presence. Based on the unique features of optical biosensors they are classified into several types, i.e., optical waveguide-based, surface plasmon resonance-based, photonic crystal-based, optical resonator-based, and optical fiber-based (Huertas et al., 2019; Chen and Wang, 2020). Previous studies proved that immunogens and optical biosensors are a perfect amalgamation for detecting various pathogens (Viter et al., 2017). Since, the advent of nanotechnology, nanotechnology-based detection kits are also available for pathogen detection (Anand and Panigrahi, 2021). Apart from the discussion about biosensors, the eNose, and e-tongue are a unique class of biosensors that mimic a human nose or tongue. In agriculture, they facilitate the detection through fingerprint generation without halting the uniqueness of the sample. They played a vital role in pests, water, and soil analysis (Wesoły et al., 2023). Previous research suggests that eNose with near-infrared (NIR) provides 100% accuracy whereas, e-tongue provides about 90% accuracy based on linear discriminant analysis (LDA) (Sipos et al., 2020). Plant disease detection by eNose relies on the fact that the volatile organic compounds are released by damaged tissues. Through distinct smell print patterns, the development of aroma signature databases—which are sourced from validated clinical samples and processed via sophisticated algorithms allows the accurate diagnosis of certain diseases (Wilson et al., 2004).
Bacterial and fungal diseases are a significant threat to agriculture, which decreases crop quality, quantity, and economic stability. As they spread quickly, it is challenging to control diseases without prior information about the initiation of disease. Bacterial infections deteriorate a plant’s physiology, and morphology leading to decreased output whereas, fungal diseases like rusts and blights damage plants by producing various toxic mycotoxins. Furthermore, climatic change and monoculture practices raise the concern of disease outbreaks. Biocontrol agents, nanotechnology, and integrated crop management are examples of crucial sustainable solutions. To maintain agricultural sustainability efficient disease control techniques, and worldwide efforts are required (Lindsey et al., 2020; Fisher et al., 2020). Eighty percent of plant illnesses are caused by fungal infections, with diseases like downy mildew (Plasmopara) and potato blight (Phytophthora) seriously harming crops (Kumar and Arora, 2020). Plant pathogens such as bacteria, viruses, and fungi, have a devastating effect on agricultural yields and financial stability. For effective management of disease, novel biosensors have been employed such as, based on paper and plastic provide quick, easy, and affordable on-site pathogen detection options (Khater et al., 2017). To gain prior information on illness various biosensors are used to evaluate pathogenic infection in real-time. One such example is, Fiber-optic biosensors which are integrated with surface refractive index modulation and plasmon enhancement and offer extraordinary sensitivity, stability, and specificity in detecting fungal biomarkers with a significant reduction in detection time to 30 min (Chen P. et al., 2024). With sensitive and selective detection of disease-causing pathogens, future diagnosis requires the amalgamation of multiplexing, CRISPR, AI, neural networks, Internet of Things (IoT), and cloud computing (Lorenzo-Villegas et al., 2023).
The electrochemical and fluorescence techniques-based dual mode of biosensor use for sensitive detection of mycotoxins. The technique based on the zeolitic imidazolate framework-8 (ZIF-8) and Fe3O4@AuNPs provides sensitive detection of aflatoxin with an LOD of 0.32 pg/mL (electrochemical) and 0.20 fg/mL (fluorescence) provide about 90% accuracy (Rahmanian et al., 2024). Additionally, these integrated biosensors using Au-Co/Zn ZIF enzymes and gold-DNA nanoclusters in their architecture detect colorimetric dual signals and were used for sugarcane smut detection with a 23.59 aM detection limit (Tang et al., 2024). Adding to this, recent development of carboxymethyl hemicellulose (CM-Hemi) and fluorescent nitrogen-doped carbon dots (CM-Hemi@Ca-N–CDs) hydrogel sensors were developed from sugarcane bagasse provide antifungal and antibacterial qualities against Candida albicans, Staphylococcus aureus, and E. coli. These sensors utilize molecular docking, fluorescence microscopy to validate the hydrogel’s efficacy, demonstrating its microbial interactions and structural stability (Tohamy, 2025). Another example is an electrochemical-based biosensor, which detects a non-enzymatic glucose sensor, that is used for wheat yellow rust (Puccinia striiformis f. sp. tritici) early detection (Hassan et al., 2022). A unique type of biosensors, i.e., chitinase-based biosensors use chitinases, and affinity of chitin to quickly and accurately identify the pathogenic fungus (Lucas-Bautista et al., 2022). Moreover, the development of a label-free electrochemical immunosensors that utilizes an AuNPs-reduced graphene oxide (AuNPs-rGO) nanocomposite, was used for the detection of beet necrotic yellow vein virus (LOD: 150 fg/mL) with no cross-reactivity (Karimzade et al., 2024). Another example of a label-free electrochemical DNA biosensor was developed for early sensitive (LOD: 0.026 nM) detection of Candidatus Liberibacter asiaticus (CLas) (Kazemzadeh-Beneh et al., 2024). Several other examples of biosensors in pathogen detection are listed in Table 2 and the overview of nanobiosensors work in disease detection is represented in Figure 2.
Figure 2. Represents the complete process of the nanobiosensors in disease detection: when the various (A) types of analytes such as enzymes, proteins, antibodies, and nucleic acid, etc., produce signals after interacting with nanocomposites used in (B) nanobiosensors architecture they transmit through the various (C) transducers (thermal, electrical, optical, piezoelectric, acoustic and, calorimetric) and these signals will (D) amplify and be processed further for monitoring.
Nanotechnology enables significantly advanced plant pathogen detection and contributes to the identification of fungal, nematode, and bacterial infections. However, there are relatively few reports of plant viral disease diagnosis (Boonham et al., 2008; Yao et al., 2009; Singh et al., 2022). Lin’s research on nano-based biosensors has significantly enhanced the detection sensitivity of lettuce mosaic virus, cowpea mosaic virus, and TMV compared to conventional ELISA techniques (Chartuprayoon et al., 2013; Lin et al., 2014). Cucumber mosaic virus (CMV) was detected via indirect ELISA, which involves three steps: fixing the virus antigen, treating a particular antibody, and incubating the antibody-labeled secondary antibody. A mercury electrode was used to monitor the process, which produced a sensitivity that was four times greater than the typical spectrophotometric ELISA. Other viruses, such as the TMV, and turnip mosaic virus (TuMV), were also shown to have this elevated sensitivity. Self-assembled monolayers containing gold electrodes were utilized for immunosensors, namely, for the diagnosis of the plum pox virus (PPV) (Jarocka et al., 2011).
Afterward, in 2013, Jarocka et al. diagnosed the presence of prunus necrotic ringspot virus (PNRSV) using the same method, and the findings driven to the conclusion that the similarity of biosensors with ELISA (Jarocka et al., 2013). Tsuda et al. used an optical immunosensor, lateral flow immunoassay (LFIA), for TMV detection. Subsequently, this technique was used for the diagnosis of several additional viruses, such as citrus tristeza virus (CTV), and various strains of potato virus (Tsuda et al., 1992; Danks and Barker, 2000; Salomone et al., 2004; Drygin et al., 2012) with 2 ng/mL reported sensitivity. Elevated sensitivity and the capacity to identify numerous viruses in a single experiment were demonstrated by research (Charlermroj et al., 2013) that used specific antibodies for viruses such as melon yellow spot virus (MYSV), watermelon silver mottle virus (WSMoV), and chilli vein-banding mottle virus (CVbMV). However, their acceptance was constrained by the assays’ intricacy and the requirement for fluorescence readers. Additionally, reports have highlighted label-free SPR-based biosensors enable orchid virus detection such as odontoglossum ringspot virus (ORSV) and cymbidium mosaic virus (CymMV), as well as TMV, CMV, and lettuce mosaic virus. Furthermore, the special optical characteristics in FRET, QDs have been extensively employed in biosensors (Boltovets et al., 2002; Torrance et al., 2006; Skottrup et al., 2007a; Skottrup et al., 2007b; Algar and Krull, 2008; Frasco and Chaniotakis, 2009; Lin et al., 2014). Reports also claim that the treatment of Fe2O3, SiO2, and ZnO nanoparticles was studied on plants that show growth enhancement (Rastogi et al., 2017; Vazquez-Hernandez et al., 2019; Cai et al., 2019; Farooq et al., 2021). NiONP foliar spray and soil soaking resulted in more leaves and higher fresh and dry weights in virus-infected cucumber plants (Derbalah and Elsharkawy, 2019). Similarly, tobacco plants infected with the turnip mosaic virus showed higher fresh and dry weights after receiving a 50 mg/L foliar spray of TiO2 and FeO3. In contrast to untreated plants, a 200 mg/L treatment did not show discernible effects (Hao et al., 2018). TMV and PVY inoculation, tomato plants treated with AgNPs showed a substantial increase in polyphenol oxidase and antioxidant enzyme POD activity (Noha et al., 2018; Hao et al., 2019).
However, it is important to investigate the best dosages, plant growth phases, and particular NP kinds that provide the most advantages, and affect the interactions between viruses and vectors, taking into account possible dosage and stage dependencies. A multidisciplinary strategy with careful planning and the development of nano-based antiviral techniques are necessary for the control of phytoviruses in a sustainable manner.
Biosensor applications in agriculture and medical science are expanding quickly. The bioconversion should be properly monitored to optimize and maintain. Biosensors are essential because they monitor products, biomass, enzymes, antibodies, or by-products, which allows them to indirectly detect process parameters. Biosensors are perfect for precisely regulating fermentation and assuring consistent outcomes because of their great selectivity, low cost, ease of automation, and straightforward instrumentation. Furthermore, ion exchange retrieval uses biosensors to identify alterations in biological composition. For example, previous reports on isoelectric liquor supernatant containing glutamate and its ion exchange retrieval have been conducted using glutamate biosensors. Numerous crucial factors are engaged in the complex process of fermentation, most of which are challenging to assess in real-time. Critical metabolites must be monitored online so they can optimize and speed up the detection of biological process regulation. Because of their ease of use and speedy reaction, biosensors have garnered considerable interest in online fermentation process monitoring (Yan et al., 2014).
Advancements in molecular imaging DNA sequencing technology and plant biology, biosensors research have made great strides. Traditional mass spectrometry was accurate, but it was deficient in vital information on the dynamics and localization of transporters, receptors, and enzyme substrates. These days, biosensors make it simple and efficient to access this data. Dynamically responsive sensors can detect activities such as metabolite conversion or signal initiation, which are necessary to quantify dynamic processes under physiological settings. Additionally, missing components for analyte transport, control, or metabolism can be found using biosensors. The efflux of phloem loading-sucrose from the mesophyll is performed by a transport step carried out by the sucrose sensing FRET, which is in charge of protein identification. When starving yeast cells are exposed to glucose, sugar transporters that can work right away are identified using fluorimeter-based tests using sugar sensing FRET sensors (Bermejo et al., 2011). Similar experiments pinpoint the genes that influence the pH of the cytosol or vacuoles in yeast (Brett et al., 2011). The application of biosensors in genetic screening provides the availability of imaging methods with enough throughput (Jones et al., 2013). Within the field of metabolic engineering, the necessity to generate factories of microbial cells for chemical synthesis is becoming more challenging due to environmental concerns and the unsustainable nature of goods generated from petroleum. Metabolic engineering is seen by researchers as a high-potential forward-looking technology that facilitates a sustainable bioeconomy (Woolston et al., 2013). Additionally, a significant portion of fuels, commodity chemicals, and medicines are made through the use of beneficial microbes derived from renewable feedstocks, replacing the requirement of plant extraction or petroleum refining. To choose the individuals possessing the required phenotype, effective screening techniques are also necessary due to the great potential for variety production. The prior techniques used spectroscopy-based enzymatic assay analytics, but their throughput was constrained. To get around this problem, engineered genetically encoded biosensors offer cellular metabolite monitoring in vivo. These biosensors are potential for high-throughput screening and selection processes that use cell survival and FACS (fluorescence-activated cell sorting), respectively. A ligand-binding peptide containing FRET sensors is made up of two donor and acceptor fluorophores. The peptide had a conformational shift that resulted in a FRET change when a prominent ligand was bound (Peroza et al., 2015). Sensors based on FRET were restricted in that they could only offer information on the quantity of the targeted metabolites and were not able to alter downstream signaling, demonstrating excellent orthogonality, temporal resolution, and simplicity of construction (Bermejo et al., 2011).
Biosensors that glow are known as fluorescent-based biosensors; these are the imaging tools used in medication development and cancer research. They have made it possible to gain an understanding of how enzymes are regulated at the cellular level. FRET biosensors that are genetically encoded and based on GFP are essential. Fluorescent biosensors are tiny scaffolds that have one or more fluorescent probes attached to them via a receptor (chemically, enzymatically, or genetically). By recognizing a particular analyte, the receptor transduces a fluorescent or electroluminescence signal that is easily identified and quantified (Wang et al., 2009). Applications of biosensing in biodefense: the military may employ biosensors in the event of a biological assault. The primary goal of these biosensors is to quickly and accurately detect organisms known as biowarfare agents (BWAs), which include viruses, poisons, and bacteria (both vegetative and spore-forming). The use of molecular techniques for the development of biosensors that can recognize chemical markers linked to biological warfare agents has received substantial attention. Particularly, gene-specific detection capabilities provided by nucleic acid-based sensing devices eliminate the requirement for amplification stages to get the necessary sensitivity. Nucleic acid-based sensors are more sensitive than conventional antibody-based detection techniques because of their inherent gene-based specificity (Mehrotra, 2016).
The subject of biosensor research and development is now accessible to interdisciplinary collaboration because of advancements in nanotechnology. By controlling shape and size, NMs such as metal- and oxide-based, CNTs, QDs, NRs, and dendrimers may be explored for a variety of features that might lead to enhanced biosensor performance and increased detection power. The operation of nanobiosensors is based on the same fundamental principles as their traditional, but the employment of nanoscale components in their architecture made the difference. The dimensionality and extraordinary transdisciplinary nature provide the advantage over conventional micro and macro counterparts. In the field of nanotechnology, nanosensors are essential for (a) analysis of nanoscopic particles in the environment, (b) diagnosis of biochemicals in medical diagnosis and cellular organelles, (c) monitoring physical and chemical changes in regions that are hard-to-reach regions, and (d) measurement of extremely-low concentrations of potentially toxic and harmful substances (Abdel-Karim et al., 2020).
Biosensors become more potent instruments in agricultural biotechnology in recent years with the ability to precis identification of biomolecules, which is essential for continuously increasing the need of sustainable agriculture the assessment of plant development, stress responses, and disease resistance is necessary. The assessment required to evaluate gene regulation of plants in response to particular stress as soon as possible. The development of fluorescent nanosensors, which are used for the in situ detection of plant miRNAs, one of the essential components of gene regulation, is a breakthrough in the area of new-age agriculture management practices. Chen and colleagues developed nanosensors using the MB-AuNP complex, it made up of nanoparticles (AuNPs) with molecular beacons (MBs) to detect miR156, an important regulator of plant growth. The MB-AuNP complex provides high stability against DNase I, this feature facilitates to ensure the specific selectivity of target miRNA detection. This study highlights real-time miRNA monitoring and gives a critical insight into how sensors can be used in plant biotechnology to understand gene regulation in response to particular stimuli (Chen L. et al., 2024). Similarly, Ultra-sensitive detection of sugarcane smut is made possible by a unique biofuel cell-based nano biosensor that uses RCA, a DNA nano-grid array, and Mn-doped ZIF-67. It improves signal amplification, electron transport, and enzyme loading, leading to a 16.7-fold increase in sensitivity and a detection limit of 34.5 aM. In precision agriculture, the self-powered, smartphone-assisted platform provides a portable and effective way to monitor plant diseases in real-time (Fu et al., 2025).
A recent development in dual-modal biosensing devices provides sensitive detection of sugarcane smut with great sensitivity and accuracy for early detection with a detection range of 0.0001–10,000 pM and a detection limit of 56.76 aM. It amplifies detecting signals by combining 3D DNA walker technology and uses gold-vanadium metal-organic frameworks (Au-V-MOF). The double-stranded DNA structures absorb a dye and improve signal detection, this sensor particularly uses catalytic capabilities of DNAzymes and a hybridization chain reaction (Che et al., 2025). Another example of a portable dual-modal detection tool is given by Song et al., 2025, particularly for sugarcane pokkah boeng disease for precise and early identification a detection limit of 6.1 aM and a detection range of 0.0001–10,000 pM. This sensor is made up of a cross-N DNA framework with Exo III exonuclease-assisted signal amplification and uses gold nanomenzyme (Mn3O4@Au), and molybdenum disulfide (MoS2) with graphdiyne (GDY) (MoS2@GDY) in its architecture. As these sensors are based on colorimetric and electrochemical detection, prevent false positives (Song et al., 2025).
To improve small-scale disease localization, a recent study suggests a novel methodology for more precise and effective detection of pathogens. Optimization of model architectures and the use of dynamic representation modules and multi-feature scale operation-like strategies, accuracy is increased by 94.8% with a decrease in memory up to 90% (Chen et al., 2025). With the development of deep learning models, agricultural disease diagnosis become feasible, for instance, YOLOv8, provides critical insight into how AI and mobile technologies may be used to develop to gain sustainability in agriculture with real-time monitoring (Nwaneto et al., 2025). To provide early and precise detection, a new mobile bio-platform was reported with advanced technologies to detect sugarcane smut in real-time assessment with a sensitive detection limit of 23.59 aM and a detection range of 0.0001–10,000 pM. It utilizes gold-cobalt/zinc zeolite imidazolate framework enzymes, and gold-DNA nanoclusters and provides valuable insights for future plant disease management and real-world implementation of nanobiosensors (Tang et al., 2024). Figure 3 demonstrates how nanobiosensors are used for real-time monitoring of precise agriculture practices. Another example of one such nanobiosensors is micro-sized gold interdigitated electrodes, a label-free DNA-based impedimetric sensor is used for identifying the soil-borne pathogen Ralstonia solanacearum, and a DNA probe with non-faradaic electrochemical impedance spectroscopy to provide very sensitive detection with a limit as low as 0.1 ng/µL (Patel et al., 2023).
Figure 3. Schematic representation of real-time plant monitoring at an (a) early growth stage by a (b) nanosensor. The (c) system detects various analytes interacting with nanoparticles, which are then transduced by the various type of (d) sensor transducers. Signals are generated, that are integrated with ((e)/(f)) smart AI detection and smartphone-based real-time monitoring, enabling precise and data-driven farming with real-time (g) detection of biotic and abiotic stress.
The role of nanomaterials has been studied extensively in improving biosensing based on how they are classified. For example, any sensors that use metallic NPs to improve biochemical signals are considered NPs-based biosensors. In a similar vein, biosensors utilizing nanotubes for charge transport and carrier are referred to as nanotube-based biosensors, whereas, biosensors based on CNTs improve the specificity and efficiency of reactions. Similarly, QDs are used as contrast agents in QD-based sensors to enhance optical response. Utilizing CNTs for biosensors, the most studied class of nanomaterial in biosensors for diagnostics are CNTs, which are fascinating one-dimensional nanotubes. CNTs have hollow cylinder-like structures that can be single-, double-, or multi-walled CNTs, which are made up of various layers of concentric graphite topped by fullerene hemispheres. Additionally, their distinct architectures, these materials exhibit exceptional mechanical and electrical characteristics, chemical stability and high thermal conductivity, little surface fouling, low over-voltage, and a high aspect surface-to-volume ratio (Sireesha et al., 2018; Simon et al., 2019). These nanostructures’ enhanced surface-to-volume ratio and their unique electron transport characteristics mean that even small surface perturbations—like those brought on by macromolecule binding have a significant impact on their electrical conductance. Biosensors based on nanorods are frequently employed as straightforward electrochemical modifiers that offer a very focused procedure. Typically, gold, graphene, manganese, zinc, iron oxide, or their amalgamation are used to manufacture them. They are frequently used in the detection of nucleic acids or simple biological indicators like hydrogen peroxide and glucose. A novel CDs/Au NR An assembly-based FRET sensor with a linear detection range of 0–155 μM and a detection limit of 0.05 μM was developed by Liu et al. to detect lead ions (Liu et al., 2019). The use of AuNPs-based assays for the diagnosis of Botrytis grey mould (caused by B. fabae or B. cinerea) in legumes. The technique uses biotinylated capture probes and portable SPCEs to identify and measure microorganisms. It can identify a single spore in plant samples and has ten times the sensitivity of quantitative PCR (Sambasivam et al., 2024).
The sensitivity, specificity, and mobility of biosensors for plant disease detection have greatly increased recently; yet, issues with field application, environmental stability, and cost-effectiveness still exist. To improve real-time monitoring and sustainable disease control in agriculture, future advancements should concentrate on combining AI-driven data analysis, multiplex detection, scalable, and environmental friendly manufacturing.
Nanoscale sensors and probes have the potential for improving plant disease diagnosis and evaluation, but face challenges like toxicity, environmental impact, data exchange, and sensor stability. Safety testing is crucial for agricultural nanosensors. The latest generation of nanosensors is expected to measure in real-time and have improved wireless connection. They should be resilient and able to tolerate abiotic factors in the agricultural field (Li et al., 2020). Crop plant diseases brought on by phytopathogenic bacteria generate enormous losses and seriously jeopardize the security of the world’s food supply.
The prompt diagnosis of plant diseases by effective diagnostic technologies is essential for ensuring the sustainability of agriculture and the world’s food supply. While molecular tests based on antibodies and nucleic acids are well-established and standardized for the diagnosis of plant diseases, the evaluation procedures are intricate and time-consuming. Applications of nano-inspired biosensors in human health, environmental sciences, quality assurance, and other fields have improved human life quality. Numerous plant pathogens, including bacteria, fungi, phytoplasmas, nematodes, viroid, and viruses, as well as abiotic stressors, phytohormones, and mi RNAs, have been identified through the development of nano-inspired biosensors. Notably, biosensors have been altered and tailored, or “nano-tuned,” utilizing a variety of nanomaterials’ properties to get around the drawbacks of traditional techniques and achieve previously unheard levels of performance (sensing ultra-trace amounts) for measurements both in vivo and in vitro, resulting in the creation of “Next Gen Nano-inspired Biosensors.” Customizable nanomaterials or nanocomposites are increasingly being combined with conventional biosensors and biosensing technologies, such as plasmonic nanosensors, surface-enhanced raman scattering fluorescence, chemiluminescence, and sophisticated electrochemical assays. Now that the unexplored gap in the area has been identified, it is being utilized to create plant biosensors with nanotechnology inspiration (Sharma and Dhadly, 2023). Existing methods for genetic transformation and plant breeding have been enhanced by nano-based devices. Additionally, it has been noted that agricultural techniques based on engineered nanomaterials (ENMs) can adapt to changing climatic circumstances based on ENMs and the environment (Mittal et al., 2020). Nutrient distribution that is specifically targeted is aided by ENMs coated with molecular recognition components like antibodies or aptamers. This promotes the sustainable use of fertilizers and minimizes negative environmental effects while increasing crop output. These ENMs improve agricultural practices and address problems with both traditional and modern agricultural practices of agrochemicals and their effective application and management by improving targeted delivery. By enhancing the photosynthetic efficiency of crops and preventing the impact of abiotic stresses on photosynthesis rate, chlorophyll quantity, light absorption efficiency, scavenging reactive oxygen species, and supporting an efficient electron transport system, ENM-based foliar treatment increases crop productivity (Lowry et al., 2019). It has occasionally been discovered that foods with high photosynthetic rates have less nutritional value. Consequently, the high nutrition content in meals is maintained by spraying iron, zinc, and silica micronutrients with ENMs. Additionally, altering the surface chemistry of ENMs makes them cling more to leaves, which boosts the effectiveness of cuticle and mesophyll cells’ absorption of nutrients. Furthermore, according to Giraldo et al. (2019), these ENMs have been utilized in the designing of sensing devices that assess crop health with great spatial and temporal resolution.
Nanoparticles can enhance detection systems in food safety, environmental monitoring, medical diagnostics, and contaminant detection. However, they face challenges such as high cost and product sensitivity, toxicity, and difficulty in large-scale fabrication. Additionally, the stability and sensitivity of the raw materials are crucial. Nanosensors also face challenges in power consumption, durability, biocompatibility, and interaction with current systems. To address these issues, careful planning, safety precautions, and suitable materials are needed. Ethical and privacy issues may arise, necessitating standard operating procedures and laws to control data collection. Despite these challenges, nanomaterials have potential applications in various fields.
Maintaining sustainability in agriculture practices and environmental monitoring necessitates the introduction of new techniques that ease the early detection of agricultural issues. The use of nanosensors in agricultural practices requires sensitivity and specificity over traditional methods. The conventional techniques are time-consuming, restrict the sample limit, and require handling experts. Nanobiosensors are an economic, continuous monitoring approach and can also be a successful alternative to chemical-based pesticides, which can enhance the unsustainability of agriculture. However, a more extensive study is needed on the application of nanosensors in agroecology. Nowadays nanosensor techniques are extensively used in environmental evaluation and offer a viable herbicide identification and environmental monitoring method when paired with cutting-edge degradation research (Zamora-Sequeira et al., 2019; Singh et al., 2021). Nanobiosensors provide a sensitive and portable platform for the real-time monitoring of pollutants such as pesticides, heavy metals, toxins, and various pathogens. Nanobiosensors such as enzymatic nanobiosensors are cost-effective approaches and provide rapid analysis in the picomolar range without the involvement of trained staff that is required in conventional techniques. The incorporation of nano biosensors into the agriculture sector provides a wide range of benefits (Verma, 2017; Verma and Rani, 2021).
Some classes of nanobiosensors use mathematical modeling to interpret vital processes and generate digital analogs. This special class of nano biosensors is renowned as plant nanobionics that convey plant natural processes information (Butnariu and Butu, 2019). Moreover, nanobiosensors easier to quickly analysis of biotic and abiotic stress on crops. The high-throughput screening of various classes of plant hormones is possible by fluorescent-based nanosensors that use specific receptors, illuminate in the dark, and facilitate to understanding of hormonal signaling pathways (Chesterfield et al., 2020). The objective of realizing high-throughput, low-cost, multiplexed operations for clinical diagnostics using microfluidic-based lab-on-a-chip (LOC) devices has yet been unexplored, despite the engineering of several biosensors in the last few decades. To do this, more sophisticated and reasonably priced nano biosensors must be developed through well-coordinated interdisciplinary research including engineers, biologists, scientists, and medical professionals. There is a need to focus on most alluring characteristics of nanostructured materials, such as dimension, quantum size, and surface effects, which should be the main focus of investigation. It is also an imperative aspect to find new nanomaterials with improved qualities for biosensing applications. Nanotechnology-based biosensors ought to be included in compact microfluidic devices that possess electronics, on-chip controllers, sample handling, and analysis capabilities. Devices that are straightforward, affordable, eco-friendly, disposable, and efficient diagnostic instruments would be produced as a precise implication of this integration. Shortly Automation, integration, and downsizing should be the goals of nanobiosensor technology. The commercialization of these goods may be facilitated by incorporating cutting-edge technology elements like AI, cloud computing, data analysis, deep learning (DL), cyber-physical systems, and the IoT (Malekzad et al., 2017; Yüce and Kurt, 2017; Bhattarai and Hameed, 2020).
AC: Conceptualization, Resources, Writing – original draft. DT: Writing – review and editing. RR: Supervision, Writing – review and editing.
The author(s) declare that no financial support was received for the research and/or publication of this article.
The authors declare that the research was conducted in the absence of any commercial or financial relationships that could be construed as a potential conflict of interest.
The author(s) declare that no Generative AI was used in the creation of this manuscript.
All claims expressed in this article are solely those of the authors and do not necessarily represent those of their affiliated organizations, or those of the publisher, the editors and the reviewers. Any product that may be evaluated in this article, or claim that may be made by its manufacturer, is not guaranteed or endorsed by the publisher.
Abdel-Karim, R., Reda, Y., and Abdel-Fattah, A. (2020). Review—nanostructured materials-based nanosensors. J. Electrochem. Soc. 167 (3), 037554. doi:10.1149/1945-7111/ab67aa
Ahmed, F., Dwivedi, S., Shaalan, N. M., Kumar, S., Arshi, N., Alshoaibi, A., et al. (2020). Development of selenium nanoparticle based agriculture sensor for heavy metal toxicity detection. Agriculture 10 (12), 610. doi:10.3390/agriculture10120610
Algar, W. R., and Krull, U. J. (2008). Quantum dots as donors in fluorescence resonance energy transfer for the bioanalysis of nucleic acids, proteins, and other biological molecules. Anal. Bioanal. Chem. 391, 1609–1618. doi:10.1007/s00216-007-1703-3
Ali, K., Nishtar, Z., Sabir, R. M., and Safdar, M. (2024). “Biosensors for environmental monitoring in the smart agriculture sector,” in Agriculture and aquaculture applications of biosensors and bioelectronics (Hershey, PA: IGI Global), 135–154.
Anand, K., and Panigrahi, B. (2021). “Green synthesized nanoparticles: a way to produce novel nano-biosensor for agricultural application,” in Nanotechnology in sustainable agriculture (Boca Raton, FL: CRC Press, Taylor & Francis), 175–190.
Ananias Reis, D., Caon, N. B., Giuliana, V., Alexandre Luis, P., Rosane Nora, C., João Victor, N., et al. (2025). Electrochemical biosensor based on gherkin (Cucumis anguria) peroxidase immobilized on chitosan-coated iron oxide nanoparticles for phenolic compounds detection. Electroanalysis, 202400251. doi:10.1002/elan.202400251
Ansari, M. H., Lavhale, S., Kalunke, R. M., Srivastava, P. L., Pandit, V., Gade, S., et al. (2020). Recent advances in plant nanobionics and nanobiosensors for toxicology applications. Curr. Nanosci. 16 (1), 27–41. doi:10.2174/1573413715666190409101305
Arvand, M., and Mirroshandel, A. A. (2019). An efficient fluorescence resonance energy transfer system from quantum dots to graphene oxide nano sheets: application in a photoluminescence aptasensing probe for the sensitive detection of diazinon. Food Chem. 280, 115–122. doi:10.1016/j.foodchem.2018.12.069
Asefpour Vakilian, K. (2024). Detecting abiotic stresses in rice plants using a smart optical biosensor based on gold nanoparticles. Iran. J. Biosyst. Eng. 55 (1), 51–69. doi:10.22059/ijbse.2024.380124.665559
Barry, S., and O’Riordan, A. (2016). Electrochemical nanosensors: advances and applications. Rep. Electrochem., 1–14. doi:10.2147/rie.s80550
Bermejo, C., Ewald, J. C., Lanquar, V., Jones, A. M., and Frommer, W. B. (2011). In vivo biochemistry: quantifying ion and metabolite levels in individual cells or cultures of yeast. Biochem. J. 438 (1), 1–10. doi:10.1042/bj20110428
Bhattarai, P., and Hameed, S. (2020). Basics of biosensors and nanobiosensors. Nanobiosensors Des. Appl., 1–22. doi:10.1002/9783527345137.ch1
Boltovets, P. M., Boyko, V. R., Kostikov, I. Y., Dyachenko, N. S., Snopok, B. A., and Shirshov, Y. M. (2002). Simple method for plant virus detection: effect of antibody immobilization technique. J. Virological Methods 105 (1), 141–146. doi:10.1016/s0166-0934(02)00098-8
Boonham, N., Glover, R., Tomlinson, J., and Mumford, R. (2008). Exploiting generic platform technologies for the detection and identification of plant pathogens. Sustain. Dis. Manag. a Eur. context, 355–363. doi:10.1007/978-1-4020-8780-6_15
Borse, V., and Srivastava, R. (2019). Process parameter optimization for lateral flow immunosensing. Mater. Sci. Energy Technol. 2 (3), 434–441. doi:10.1016/j.mset.2019.04.003
Brett, C. L., Kallay, L., Hua, Z., Green, R., Chyou, A., Zhang, Y., et al. (2011). Genome-wide analysis reveals the vacuolar pH-stat of Saccharomyces cerevisiae. PloS one 6 (3), e17619. doi:10.1371/journal.pone.0017619
Butnariu, M., and Butu, A. (2019). Plant nanobionics: application of nanobiosensors in plant biology. Plant Nanobionics Volume 2, Approaches Nanoparticles, Biosynth. Toxic., 337–376. doi:10.1007/978-3-030-16379-2_12
Cai, L., Liu, C., Fan, G., Liu, C., and Sun, X. (2019). Preventing viral disease by ZnONPs through directly deactivating TMV and activating plant immunity in Nicotiana benthamiana. Environ. Sci. Nano 6 (12), 3653–3669. doi:10.1039/c9en00850k
Camacho, M. J., Albuquerque, D. C., Inácio, M. L., Martins, V. C., Mota, M., Freitas, P. P., et al. (2024). FTA-LAMP based biosensor for a rapid in-field detection of Globodera pallida—the pale potato cyst nematode. Front. Bioeng. Biotechnol. 12, 1337879. doi:10.3389/fbioe.2024.1337879
Caratelli, V., Fegatelli, G., Moscone, D., and Arduini, F. (2022). A paper-based electrochemical device for the detection of pesticides in aerosol phase inspired by nature: a flower-like origami biosensor for precision agriculture. Biosens. Bioelectron. 205, 114119. doi:10.1016/j.bios.2022.114119
Charlermroj, R., Himananto, O., Seepiban, C., Kumpoosiri, M., Warin, N., Oplatowska, M., et al. (2013). Multiplex detection of plant pathogens using a microsphere immunoassay technology. PloS one 8 (4), e62344. doi:10.1371/journal.pone.0062344
Chartuprayoon, N., Rheem, Y., Ng, J. C., Nam, J., Chen, W., and Myung, N. V. (2013). Polypyrrole nanoribbon based chemiresistive immunosensors for viral plant pathogen detection. Anal. Methods 5 (14), 3497–3502. doi:10.1039/c3ay40371h
Che, R., Tang, D., Fu, B., Yan, F., Yan, M., Wu, Y., et al. (2025). Dual-modal improved biosensing platform for sugarcane smut pathogen based on biological enzyme-Mg2+ DNAzyme coupled with DNA transporter cascading hybridization chain reaction. Int. J. Biol. Macromol. 286, 138403. doi:10.1016/j.ijbiomac.2024.138403
Chen, C., and Wang, J. (2020). Optical biosensors: an exhaustive and comprehensive review. Analyst 145 (5), 1605–1628. doi:10.1039/c9an01998g
Chen, D., Lin, F., Lu, C., Zhuang, J., Su, H., Zhang, D., et al. (2025). YOLOv8-MDN-Tiny: a lightweight model for multi-scale disease detection of postharvest golden passion fruit. Postharvest Biol. Technol. 219, 113281. doi:10.1016/j.postharvbio.2024.113281
Chen, L., Hu, G., Cui, B., Gao, J., Gu, X., Cui, H., et al. (2024a). Development of a stable and efficient fluorescence nanosensor for in situ detection of MicroRNA in plant. Sensors Actuators B Chem. 404, 135244. doi:10.1016/j.snb.2023.135244
Chen, P., Wu, H., Zhao, Y., Zhong, L., Zhang, Y., Zhan, X., et al. (2024b). Quantitative assessment of fungal biomarkers in clinical samples via an interface-modulated optical fiber biosensor. Adv. Mater. 36 (21), 2312985. doi:10.1002/adma.202312985
Chesterfield, R. J., Whitfield, J. H., Pouvreau, B., Cao, D., Alexandrov, K., Beveridge, C. A., et al. (2020). Rational design of novel fluorescent enzyme biosensors for direct detection of strigolactones. ACS Synth. Biol. 9 (8), 2107–2118. doi:10.1021/acssynbio.0c00192
Choudhary, M. K., Agrawal, R., Kumar, R., Singh, P., Gupta, B. R. K., and Singh, K. P. (2010). Detection of cadmium-resistant rhizobacteria using piezoelectric nanobiosensor. Int. J. Nanosci. 9 (05), 461–469. doi:10.1142/s0219581x10007034
Coroş, M., Pruneanu, S., and Stefan-van Staden, R. I. (2019). Recent progress in the graphene-based electrochemical sensors and biosensors. J. Electrochem. Soc. 167 (3), 037528. doi:10.1149/2.0282003JES
Cruz, A. F. D., Norena, N., Kaushik, A., and Bhansali, S. (2014). A low-cost miniaturized potentiostat for point-of-care diagnosis. Biosens. Bioelectron. 62, 249–254. doi:10.1016/j.bios.2014.06.053
Danks, C., and Barker, I. (2000). On-site detection of plant pathogens using lateral-flow devices. Eppo Bull. 30 (3-4), 421–426. doi:10.1111/j.1365-2338.2000.tb00922.x
Dar, F. A., Qazi, G., and Pirzadah, T. B. (2020). Nano-biosensors: NextGen diagnostic tools in agriculture. Nanobiotechnology Agric. approach towards Sustain., 129–144. doi:10.1007/978-3-030-39978-8_7
Das, S., Agarwal, D. K., Mandal, B., Rao, V. R., and Kundu, T. (2021). Detection of the chilli leaf curl virus using an attenuated total reflection-mediated localized surface-plasmon-resonance-based optical platform. ACS omega 6 (27), 17413–17423. doi:10.1021/acsomega.1c01702
Derbalah, A. S. H., and Elsharkawy, M. M. (2019). A new strategy to control Cucumber mosaic virus using fabricated NiO-nanostructures. J. Biotechnol. 306, 134–141. doi:10.1016/j.jbiotec.2019.10.003
Deshmukh, K., Kovářík, T., and Pasha, S. K. (2020). State of the art recent progress in two dimensional MXenes based gas sensors and biosensors: a comprehensive review. Coord. Chem. Rev. 424, 213514. doi:10.1016/j.ccr.2020.213514
Drygin, Y. F., Blintsov, A. N., Grigorenko, V. G., Andreeva, I. P., Osipov, A. P., Varitzev, Y. A., et al. (2012). Highly sensitive field test lateral flow immunodiagnostics of PVX infection. Appl. Microbiol. Biotechnol. 93, 179–189. doi:10.1007/s00253-011-3522-x
Fallatah, A., Kuperus, N., Almomtan, M., and Padalkar, S. (2022). Sensitive biosensor based on shape-controlled ZnO nanostructures grown on flexible porous substrate for pesticide detection. Sensors 22 (9), 3522. doi:10.3390/s22093522
Farooq, T., Adeel, M., He, Z., Umar, M., Shakoor, N., da Silva, W., et al. (2021). Nanotechnology and plant viruses: an emerging disease management approach for resistant pathogens. ACS Nano 15 (4), 6030–6037. doi:10.1021/acsnano.0c10910
Fisher, M. C., Gurr, S. J., Cuomo, C. A., Blehert, D. S., Jin, H., Stukenbrock, E. H., et al. (2020). Threats posed by the fungal kingdom to humans, wildlife, and agriculture. MBio 11 (3), e00449-20–e01128. doi:10.1128/mbio.00449-20
Frasco, M. F., and Chaniotakis, N. (2009). Semiconductor quantum dots in chemical sensors and biosensors. Sensors 9 (9), 7266–7286. doi:10.3390/s90907266
Fu, B., Che, R., Yan, F., Wu, Y., Luo, H., Yan, J., et al. (2025). Sensitivity-enhanced self-powered biosensing platform for detection of sugarcane smut using Mn-doped ZIF-67, RCA-DNA nano-grid array and capacitor. Biosens. Bioelectron. 273, 117182. doi:10.1016/j.bios.2025.117182
Gangopadhyay, M., Chakravarti, S., Sanyamat, S., and Dewanjee, S. (2024). “Biosensors in agriculture: pioneering the future of precision farming and sustainability,” in Biosensors: developments, challenges and perspectives (Singapore: Springer Nature Singapore), 259–301.
German, N., Popov, A., Ramanaviciene, A., and Ramanavicius, A. (2017). Evaluation of enzymatic formation of polyaniline nanoparticles. Polymer 115, 211–216. doi:10.1016/j.polymer.2017.03.028
Giraldo, J. P., Wu, H., Newkirk, G. M., and Kruss, S. (2019). Nanobiotechnology approaches for engineering smart plant sensors. Nat. Nanotechnol. 14 (6), 541–553. doi:10.1038/s41565-019-0470-6
Haleem, A., Javaid, M., Singh, R. P., Suman, R., and Rab, S. (2021). Biosensors applications in medical field: a brief review. Sensors Int. 2, 100100. doi:10.1016/j.sintl.2021.100100
Hammond, J. L., Formisano, N., Estrela, P., Carrara, S., and Tkac, J. (2016). Electrochemical biosensors and nanobiosensors. Essays Biochem. 60 (1), 69–80. doi:10.1042/ebc20150008
Hao, Y., Fang, P., Ma, C., White, J. C., Xiang, Z., Wang, H., et al. (2019). Engineered nanomaterials inhibit Podosphaera pannosa infection on rose leaves by regulating phytohormones. Environ. Res. 170, 1–6. doi:10.1016/j.envres.2018.12.008
Hao, Y., Yuan, W., Ma, C., White, J. C., Zhang, Z., Adeel, M., et al. (2018). Engineered nanomaterials suppress Turnip mosaic virus infection in tobacco (Nicotiana benthamiana). Environ. Sci. Nano 5 (7), 1685–1693. doi:10.1039/c8en00014j
Hassan, M. H., Omar, A. M., Daskalakis, E., Mohamed, A. A., Boyd, L. A., Blanford, C., et al. (2022). Multi-layer biosensor for pre-symptomatic detection of Puccinia strifformis, the causal agent of yellow rust. Biosensors 12 (10), 829. doi:10.3390/bios12100829
He, K. C., Wang, H. R., Yang, H., Sun, L. J., Liu, W., and Bao, N. (2020). Digitalized pencil trace modified electrodes for real time evaluation of salicylic acid in detached Arabidopsis thaliana leaves during regeneration. Anal. Chim. Acta 1120, 59–66. doi:10.1016/j.aca.2020.04.051
Huang, X., Zhu, Y., and Kianfar, E. (2021). Nano biosensors: properties, applications and electrochemical techniques. J. Mater. Res. Technol. 12, 1649–1672. doi:10.1016/j.jmrt.2021.03.048
Huertas, C. S., Calvo-Lozano, O., Mitchell, A., and Lechuga, L. M. (2019). Advanced evanescent-wave optical biosensors for the detection of nucleic acids: an analytic perspective. Front. Chem. 7, 724. doi:10.3389/fchem.2019.00724
Jablonski, M., Poghossian, A., Severins, R., Keusgen, M., Wege, C., and Schöning, M. J. (2021). Capacitive field-effect biosensor studying adsorption of tobacco mosaic virus particles. Micromachines 12 (1), 57. doi:10.3390/mi12010057
Jarocka, U., Wąsowicz, M., Radecka, H., Malinowski, T., Michalczuk, L., and Radecki, J. (2011). Impedimetric immunosensor for detection of plum pox virus in plant extracts. Electroanalysis 23 (9), 2197–2204. doi:10.1002/elan.201100152
Jarocka, U., Radecka, H., Malinowski, T., Michalczuk, L., and Radecki, J. (2013). Detection of Prunus Necrotic Ringspot Virus in plant extracts with impedimetric immunosensor based on glassy carbon electrode. Electroanalysis, 25(2), 433–438. doi:10.1002/elan.201200470
Jiang, P., Wang, Y., Zhao, L., Ji, C., Chen, D., and Nie, L. (2018). Applications of gold nanoparticles in non-optical biosensors. Nanomaterials 8 (12), 977. doi:10.3390/nano8120977
Jones, A. M., Grossmann, G., Danielson, J. Å., Sosso, D., Chen, L. Q., Ho, C. H., et al. (2013). In vivo biochemistry: applications for small molecule biosensors in plant biology. Curr. Opin. Plant Biol. 16 (3), 389–395. doi:10.1016/j.pbi.2013.02.010
Karimzade, M., Kazemzadeh-Beneh, H., Heidari, N., Boroumand, M. R., Norouzi, P., Safarnejad, M. R., et al. (2024). The rGO@AuNPs modified label-free electrochemical immunosensor to sensitive detection of CP-BNYVV protein of Rhizomania disease agent in sugar beet. Plant Methods 20 (1), 181. doi:10.1186/s13007-024-01307-y
Kazemzadeh-Beneh, H., Safarnejad, M. R., Norouzi, P., Samsampour, D., Alavi, S. M., and Shaterreza, D. (2024). Development of label-free electrochemical OMP-DNA probe biosensor as a highly sensitive system to detect of citrus huanglongbing. Sci. Rep. 14 (1), 12183. doi:10.1038/s41598-024-63112-w
Khater, M., De La Escosura-Muñiz, A., and Merkoçi, A. (2017). Biosensors for plant pathogen detection. Biosens. Bioelectron. 93, 72–86. doi:10.1016/j.bios.2016.09.091
Krikstolaityte, V., Kuliesius, J., Ramanaviciene, A., Mikoliunaite, L., Kausaite-Minkstimiene, A., Oztekin, Y., et al. (2014). Enzymatic polymerization of polythiophene by immobilized glucose oxidase. Polymer 55 (7), 1613–1620. doi:10.1016/j.polymer.2014.02.003
Kumar, V., and Arora, K. (2020). Trends in nano-inspired biosensors for plants. Mater. Sci. Energy Technol. 3, 255–273. doi:10.1016/j.mset.2019.10.004
Kundu, M., Bhardwaj, H., Pandey, M. K., Krishnan, P., Kotnala, R. K., and Sumana, G. (2019a). Development of electrochemical biosensor based on CNT–Fe3O4 nanocomposite to determine formaldehyde adulteration in orange juice. J. Food Sci. Technol. 56, 1829–1840. doi:10.1007/s13197-019-03635-7
Kundu, M., Prasad, S., Krishnan, P., and Gajjala, S. (2019b). A novel electrochemical biosensor based on hematite (α-Fe2O3) flowerlike nanostructures for sensitive determination of formaldehyde adulteration in fruit juices. Food Bioprocess Technol. 12, 1659–1671. doi:10.1007/s11947-019-02318-7
Li, W., Zhang, X., Shi, Y., Hu, X., Wang, X., Liang, N., et al. (2023). A dual-modal biosensor coupling cooperative catalysis strategy for sensitive detection of AFB1 in agri-products. Food Chem. 426, 136553. doi:10.1016/j.foodchem.2023.136553
Li, Z., Yu, T., Paul, R., Fan, J., Yang, Y., and Wei, Q. (2020). Agricultural nanodiagnostics for plant diseases: recent advances and challenges. Nanoscale Adv. 2 (8), 3083–3094. doi:10.1039/c9na00724e
Lima, A. S., Prieto, K. R., Santos, C. S., Valerio, H. P., Garcia-Ochoa, E. Y., Huerta-Robles, A., et al. (2018). In-vivo electrochemical monitoring of H2O2 production induced by root-inoculated endophytic bacteria in Agave tequilana leaves. Biosens. Bioelectron. 99, 108–114. doi:10.1016/j.bios.2017.07.039
Lin, H. Y., Huang, C. H., Lu, S. H., Kuo, I. T., and Chau, L. K. (2014). Direct detection of orchid viruses using nanorod-based fiber optic particle plasmon resonance immunosensor. Biosens. Bioelectron. 51, 371–378. doi:10.1016/j.bios.2013.08.009
Lindsey, A. P. J., Murugan, S., and Renitta, R. E. (2020). Microbial disease management in agriculture: current status and future prospects. Biocatal. Agric. Biotechnol. 23, 101468. doi:10.1016/j.bcab.2019.101468
Liu, G., Feng, D. Q., Qian, Y., Wang, W., and Zhu, J. J. (2019). Construction of FRET biosensor for off-on detection of lead ions based on carbon dots and gold nanorods. Talanta 201, 90–95. doi:10.1016/j.talanta.2019.03.101
Liu, X., Cheng, H., Zhao, Y., Wang, Y., and Li, F. (2022). Portable electrochemical biosensor based on laser-induced graphene and MnO2 switch-bridged DNA signal amplification for sensitive detection of pesticide. Biosens. Bioelectron. 199, 113906. doi:10.1016/j.bios.2021.113906
Liu, Z., Wang, Z., Ma, L., Guo, L., Yang, H., Liu, Y., et al. (2025). Programmable target-driven MNAzyme and DNA tetrahedron framework synergistically constructed label-free electrochemical sensing platform for TMV RNA detection. Sensors Actuators B Chem. 428, 137256. doi:10.1016/j.snb.2025.137256
Lorenzo-Villegas, D. L., Gohil, N. V., Lamo, P., Gurajala, S., Bagiu, I. C., Vulcanescu, D. D., et al. (2023). Innovative biosensing approaches for swift identification of candida species, intrusive pathogenic organisms. Life 13 (10), 2099. doi:10.3390/life13102099
Lowry, G. V., Avellan, A., and Gilbertson, L. M. (2019). Opportunities and challenges for nanotechnology in the agri-tech revolution. Nat. Nanotechnol. 14 (6), 517–522. doi:10.1038/s41565-019-0461-7
Lucas-Bautista, J. A., Bautista-Baños, S., Ventura-Aguilar, R. I., and Hernández-López, M. (2022). Chitinases in plants and possible use as biomarkers for the design of biosensors in the detection of phytopathogenic fungi. Rev. Mex. ciencias agrícolas 13 (4), 701–713. doi:10.29312/remexca.v13i4.2717
Luna-Moreno, D., Sánchez-Álvarez, A., Islas-Flores, I., Canto-Canche, B., Carrillo-Pech, M., Villarreal-Chiu, J. F., et al. (2019). Early detection of the fungal banana black sigatoka pathogen Pseudocercospora fijiensis by an SPR immunosensor method. Sensors 19 (3), 465. doi:10.3390/s19030465
Malekzad, H., Sahandi Zangabad, P., Mirshekari, H., Karimi, M., and Hamblin, M. R. (2017). Noble metal nanoparticles in biosensors: recent studies and applications. Nanotechnol. Rev. 6 (3), 301–329. doi:10.1515/ntrev-2016-0014
Mehrotra, P. (2016). Biosensors and their applications–A review. J. oral Biol. craniofacial Res. 6 (2), 153–159. doi:10.1016/j.jobcr.2015.12.002
Mittal, D., Kaur, G., Singh, P., Yadav, K., and Ali, S. A. (2020). Nanoparticle-based sustainable agriculture and food science: recent advances and future outlook. Front. Nanotechnol. 2, 579954. doi:10.3389/fnano.2020.579954
Molinari, F. N., Marelli, M., Berretti, E., Serrecchia, S., Coppola, R. E., De Cesare, F., et al. (2025). Cutting-edge sensor design: MIP nanoparticle-functionalized nanofibers for gas-phase detection of limonene in predictive agriculture. Polymers 17 (3), 326. doi:10.3390/polym17030326
Naresh, V., and Lee, N. (2021). A review on biosensors and recent development of nanostructured materials-enabled biosensors. Sensors 21 (4), 1109. doi:10.3390/s21041109
Naveen, M. H., Gurudatt, N. G., and Shim, Y. B. (2017). Applications of conducting polymer composites to electrochemical sensors: a review. Appl. Mater. today 9, 419–433. doi:10.1016/j.apmt.2017.09.001
Nikitin, M., Deych, K., Grevtseva, I., Girsova, N., Kuznetsova, M., Pridannikov, M., et al. (2018). Preserved microarrays for simultaneous detection and identification of six fungal potato pathogens with the use of real-time PCR in matrix format. Biosensors 8 (4), 129. doi:10.3390/bios8040129
Noha, K., Bondok, A. M., and El-Dougdoug, K. A. (2018). Evaluation of silver nanoparticles as antiviral agent against ToMV and PVY in tomato plants. Sciences 8 (01), 100–111.
Nwaneto, C. B., Yinka-Banjo, C., and Ugot, O. (2025). An object detection solution for early detection of taro leaf blight disease in the West African sub-region. Frankl. Open 10, 100197. doi:10.1016/j.fraope.2024.100197
Panchuk, V., Yaroshenko, I., Legin, A., Semenov, V., and Kirsanov, D. (2018). Application of chemometric methods to XRF-data–A tutorial review. Anal. Chim. Acta 1040, 19–32. doi:10.1016/j.aca.2018.05.023
Parihar, A., Choudhary, N. K., Sharma, P., and Khan, R. (2023). MXene-based aptasensor for the detection of aflatoxin in food and agricultural products. Environ. Pollut. 316, 120695. doi:10.1016/j.envpol.2022.120695
Patel, R., Vinchurkar, M., Shaikh, A. M., Patkar, R., Adami, A., Giacomozzi, F., et al. (2023). Part I: non-faradaic electrochemical impedance-based DNA biosensor for detecting phytopathogen–Ralstonia solanacearum. Bioelectrochemistry 150, 108370. doi:10.1016/j.bioelechem.2023.108370
Peroza, E. A., Ewald, J. C., Parakkal, G., Skotheim, J. M., and Zamboni, N. (2015). A genetically encoded Förster resonance energy transfer sensor for monitoring in vivo trehalose-6-phosphate dynamics. Anal. Biochem. 474, 1–7. doi:10.1016/j.ab.2014.12.019
Rahmanian, H., Malekkiani, M., Dadmehr, M., Es' haghi, Z., and Moshirian-Farahi, S. S. (2024). A biosensor integrating the electrochemical and fluorescence strategies for detection of aflatoxin B1 based on a dual-functionalized platform. Anal. Chim. Acta 1323, 343085. doi:10.1016/j.aca.2024.343085
Ramanavicius, S., and Ramanavicius, A. (2020). Conducting polymers in the design of biosensors and biofuel cells. Polymers 13 (1), 49. doi:10.3390/polym13010049
Rastogi, A., Zivcak, M., Sytar, O., Kalaji, H. M., He, X., Mbarki, S., et al. (2017). Impact of metal and metal oxide nanoparticles on plant: a critical review. Front. Chem. 5, 78. doi:10.3389/fchem.2017.00078
Rong, Y., Li, H., Ouyang, Q., Ali, S., and Chen, Q. (2020). Rapid and sensitive detection of diazinon in food based on the FRET between rare-earth doped upconversion nanoparticles and graphene oxide. Spectrochimica Acta Part A Mol. Biomol. Spectrosc. 239, 118500. doi:10.1016/j.saa.2020.118500
Salomone, A., Mongelli, M., Roggero, P., and Boscia, D. (2004). Reliability of detection of Citrus tristeza virus by an immunochromatographic lateral flow assay in comparison with ELISA. J. Plant Pathology, 43–48.
Sambasivam, P., Bilkiss, M., Soda, N., Bar, I., Shiddiky, M. J., and Ford, R. (2024). A rapid electrochemical biosensor diagnostic for Botrytis ssp. causing Botrytis gray mold of temperate legumes. ACS Agric. Sci. and Technol. 4 (11), 1184–1193. doi:10.1021/acsagscitech.4c00136
Sharma, D., Ngasainao, M. R., and Kumari, P. (2024). Development of enzyme-based biosensor for residual detection of organophosphate pesticides using functionalized nanocellulose-modified silver nanoparticles. Microchem. J. 207, 111856. doi:10.1016/j.microc.2024.111856
Sharma, S., and Dhadly, D. K. (2023). Nano-inspired biosensors and plant diseases: recent advances and challenges. Nanoparticles Plant-Microbe Interact., 135–162. doi:10.1016/b978-0-323-90619-7.00002-3
Simon, J., Flahaut, E., and Golzio, M. (2019). Overview of carbon nanotubes for biomedical applications. Materials 12 (4), 624. doi:10.3390/ma12040624
Singh, H., Sharma, A., Bhardwaj, S. K., Arya, S. K., Bhardwaj, N., and Khatri, M. (2021). Recent advances in the applications of nano-agrochemicals for sustainable agricultural development. Environ. Sci. Process. and Impacts 23 (2), 213–239. doi:10.1039/d0em00404a
Singh, R., Kuddus, M., Singh, P. K., and Choden, D. (2022). Nanotechnology for nanophytopathogens: from detection to the management of plant viruses. BioMed Res. Int. 2022 (1), 8688584. doi:10.1155/2022/8688584
Sipos, L., Végh, R., Bodor, Z., Zaukuu, J. L. Z., Hitka, G., Bázár, G., et al. (2020). Classification of bee pollen and prediction of sensory and colorimetric attributes—a sensometric fusion approach by e-Nose, e-Tongue and NIR. Sensors 20 (23), 6768. doi:10.3390/s20236768
Sireesha, M., Jagadeesh Babu, V., Kranthi Kiran, A. S., and Ramakrishna, S. (2018). A review on carbon nanotubes in biosensor devices and their applications in medicine. Nanocomposites 4 (2), 36–57. doi:10.1080/20550324.2018.1478765
Skottrup, P., Hearty, S., Frøkiær, H., Leonard, P., Hejgaard, J., O’Kennedy, R., et al. (2007a). Detection of fungal spores using a generic surface plasmon resonance immunoassay. Biosens. Bioelectron. 22 (11), 2724–2729. doi:10.1016/j.bios.2006.11.017
Skottrup, P., Nicolaisen, M., and Justesen, A. F. (2007b). Rapid determination of Phytophthora infestans sporangia using a surface plasmon resonance immunosensor. J. Microbiol. methods 68 (3), 507–515. doi:10.1016/j.mimet.2006.10.011
Sok, V., and Fragoso, A. (2019). Amperometric biosensor for glyphosate based on the inhibition of tyrosinase conjugated to carbon nano-onions in a chitosan matrix on a screen-printed electrode. Microchim. Acta 186, 569–578. doi:10.1007/s00604-019-3672-6
Song, Y., Wang, Z., Wu, Q., Su, J., Liao, J., Zhang, X., et al. (2025). A dual-mode strategy for early detection of sugarcane pokkah boeng disease pathogen: a portable sensing device based on Cross-N DNA framework and MoS2@ GDY. Biosens. Bioelectron. 267, 116874. doi:10.1016/j.bios.2024.116874
Sun, K., Liu, Y., Tang, Q., Ma, B., Zhang, B., Fu, X., et al. (2025). CADLAB: a contamination-free, argonaute-enhanced, dual detection of plant viruses via lateral flow assay biosensors system. Sensors Actuators B Chem. 426, 137045. doi:10.1016/j.snb.2024.137045
Tak, M., Gupta, V., and Tomar, M. (2014). Flower-like ZnO nanostructure based electrochemical DNA biosensor for bacterial meningitis detection. Biosens. Bioelectron. 59, 200–207. doi:10.1016/j.bios.2014.03.036
Talari, F. F., Bozorg, A., Faridbod, F., and Vossoughi, M. (2021). A novel sensitive aptamer-based nanosensor using rGQDs and MWCNTs for rapid detection of diazinon pesticide. J. Environ. Chem. Eng. 9 (1), 104878. doi:10.1016/j.jece.2020.104878
Tang, D., Wang, Z., Che, R., Liao, J., Yan, M., Zhang, X., et al. (2024). Design of a portable bioassay system based on highly efficent nanozyme and Au-DNA nanocluster: toward current/colorimetric dual-signal detection of sugarcane smut. Chem. Eng. J. 500, 156912. doi:10.1016/j.cej.2024.156912
Tang, Y., Huang, X., Wang, X., Wang, C., Tao, H., and Wu, Y. (2022). G-quadruplex DNAzyme as peroxidase mimetic in a colorimetric biosensor for ultrasensitive and selective detection of trace tetracyclines in foods. Food Chem. 366, 130560. doi:10.1016/j.foodchem.2021.130560
Tohamy, H. A. S. (2025). Carboxymethyl hemicellulose hydrogel as a fluorescent biosensor for bacterial and fungal detection with DFT and molecular docking studies. Sci. Rep. 15 (1), 741. doi:10.1038/s41598-024-83157-1
Torrance, L., Ziegler, A., Pittman, H., Paterson, M., Toth, R., and Eggleston, I. (2006). Oriented immobilisation of engineered single-chain antibodies to develop biosensors for virus detection. J. virological methods 134 (1-2), 164–170. doi:10.1016/j.jviromet.2005.12.012
Trang, N. L. N., Nga, D. T. N., Hoang, V. T., Ngo, X. D., Nhung, P. T., and Le, A. T. (2022). Bio-AgNPs-based electrochemical nanosensors for the sensitive determination of 4-nitrophenol in tomato samples: the roles of natural plant extracts in physicochemical parameters and sensing performance. RSC Adv. 12 (10), 6007–6017. doi:10.1039/d1ra09202b
Tsai, T. T., Huang, T. H., Chen, C. A., Ho, N. Y. J., Chou, Y. J., and Chen, C. F. (2018). Development a stacking pad design for enhancing the sensitivity of lateral flow immunoassay. Sci. Rep. 8 (1), 17319. doi:10.1038/s41598-018-35694-9
Tsuda, S., Kameya-Iwaki, M., Hanada, K., Kouda, Y., Hikata, Y., and Tomaru, K. (1992). A novel detection and identification technique for plant viruses: rapid immunofilter paper assay (RIPA). Plant Dis. 76, 466. doi:10.1094/pd-76-0466
Vaitkuviene, A., Kaseta, V., Voronovic, J., Ramanauskaite, G., Biziuleviciene, G., Ramanaviciene, A., et al. (2013). Evaluation of cytotoxicity of polypyrrole nanoparticles synthesized by oxidative polymerization. J. Hazard. Mater. 250, 167–174. doi:10.1016/j.jhazmat.2013.01.038
Vatankhah, A., Reezi, S., Izadi, Z., Ghasemi-Varnamkhasti, M., and Motamedi, A. (2022). Development of an ultrasensitive electrochemical biosensor for detection of Agrobacterium tumefaciens in Rosa hybrida L. Measurement 187, 110320. doi:10.1016/j.measurement.2021.110320
Vazquez-Hernandez, C., Feregrino-Perez, A. A., Perez-Ramirez, I., Ocampo-Velazquez, R. V., Rico-García, E., Torres-Pacheco, I., et al. (2019). Controlled elicitation increases steviol glycosides (SGs) content and gene expression-associated to biosynthesis of SGs in Stevia rebaudiana B. cv. Morita II. Industrial Crops Prod. 139, 111479. doi:10.1016/j.indcrop.2019.111479
Veltman, B., Harpaz, D., Melamed, S., Tietel, Z., Tsror, L., and Eltzov, E. (2022). Whole-cell bacterial biosensor for volatile detection from Pectobacterium-infected potatoes enables early identification of potato tuber soft rot disease. Talanta 247, 123545. doi:10.1016/j.talanta.2022.123545
Verma, M. L. (2017). Nanobiotechnology advances in enzymatic biosensors for the agri-food industry. Environ. Chem. Lett. 15 (4), 555–560. doi:10.1007/s10311-017-0640-4
Verma, M. L., and Rani, V. (2021). Biosensors for toxic metals, polychlorinated biphenyls, biological oxygen demand, endocrine disruptors, hormones, dioxin, phenolic and organophosphorus compounds: a review. Environ. Chem. Lett. 19 (2), 1657–1666. doi:10.1007/s10311-020-01116-4
Villa, J. E., Afonso, M. A., Dos Santos, D. P., Mercadal, P. A., Coronado, E. A., and Poppi, R. J. (2020). Colloidal gold clusters formation and chemometrics for direct SERS determination of bioanalytes in complex media. Spectrochimica Acta Part A Mol. Biomol. Spectrosc. 224, 117380. doi:10.1016/j.saa.2019.117380
Viter, R., Tereshchenko, A., Smyntyna, V., Ogorodniichuk, J., Starodub, N., Yakimova, R., et al. (2017). Toward development of optical biosensors based on photoluminescence of TiO2 nanoparticles for the detection of Salmonella. Sensors Actuators B Chem. 252, 95–102. doi:10.1016/j.snb.2017.05.139
Wang, H., Nakata, E., and Hamachi, I. (2009). Recent progress in strategies for the creation of protein-based fluorescent biosensors. ChemBioChem 10 (16), 2560–2577. doi:10.1002/cbic.200900249
Wang, P., Li, H., Hassan, M. M., Guo, Z., Zhang, Z. Z., and Chen, Q. (2019). Fabricating an acetylcholinesterase modulated UCNPs-Cu2+ fluorescence biosensor for ultrasensitive detection of organophosphorus pesticides-diazinon in food. J. Agric. Food Chem. 67 (14), 4071–4079. doi:10.1021/acs.jafc.8b07201
Wesoły, M., Przewodowski, W., and Ciosek-Skibińska, P. (2023). Electronic noses and electronic tongues for the agricultural purposes. TrAC Trends Anal. Chem. 164, 117082. doi:10.1016/j.trac.2023.117082
Wilson, A. D., Lester, D. G., and Oberle, C. S. (2004). Development of conductive polymer analysis for the rapid detection and identification of phytopathogenic microbes. Phytopathology 94 (5), 419–431. doi:10.1094/phyto.2004.94.5.419
Woolston, B. M., Edgar, S., and Stephanopoulos, G. (2013). Metabolic engineering: past and future. Annu. Rev. Chem. Biomol. Eng. 4 (1), 259–288. doi:10.1146/annurev-chembioeng-061312-103312
Yadav, A., and Yadav, K. (2025). Portable solutions for plant pathogen diagnostics: development, usage, and future potential. Front. Microbiol. 16, 1516723. doi:10.3389/fmicb.2025.1516723
Yan, C., Dong, F., Chun-Yuan, B., Si-Rong, Z., and Jian-Guo, S. (2014). Recent progress of commercially available biosensors in China and their applications in fermentation processes. J. Northeast Agric. Univ. Engl. Ed. 21 (4), 73–85. doi:10.1016/s1006-8104(15)30023-4
Yao, K. S., Li, S. J., Tzeng, K. C., Cheng, T. C., Chang, C. Y., Chiu, C. Y., et al. (2009). Fluorescence silica nanoprobe as a biomarker for rapid detection of plant pathogens. Adv. Mater. Res. 79, 513–516. doi:10.4028/www.scientific.net/amr.79-82.513
Yeh, C. H., Chang, Y. H., Chang, T. C., Lin, H. P., and Lin, Y. C. (2010). Electro-microchip DNA-biosensor for bacteria detection. Analyst 135 (10), 2717–2722. doi:10.1039/c0an00186d
Yousefi, S. R., Alshamsi, H. A., Amiri, O., and Salavati-Niasari, M. (2021). Synthesis, characterization and application of Co/Co3O4 nanocomposites as an effective photocatalyst for discoloration of organic dye contaminants in wastewater and antibacterial properties. J. Mol. Liq. 337, 116405. doi:10.1016/j.molliq.2021.116405
Yüce, M., and Kurt, H. (2017). How to make nanobiosensors: surface modification and characterisation of nanomaterials for biosensing applications. RSC Adv. 7 (78), 49386–49403. doi:10.1039/c7ra10479k
Zamani, F. G., Moulahoum, H., Ak, M., Demirkol, D. O., and Timur, S. (2019). Current trends in the development of conducting polymers-based biosensors. TrAC Trends Anal. Chem. 118, 264–276. doi:10.1016/j.trac.2019.05.031
Zamora-Sequeira, R., Alvarado-Hidalgo, F., Robles-Chaves, D., Sáenz-Arce, G., Avendano-Soto, E. D., Sánchez-Kopper, A., et al. (2019). Electrochemical characterization of mancozeb degradation for wastewater treatment using a sensor based on poly (3, 4-ethylenedioxythiophene)(PEDOT) modified with carbon nanotubes and gold nanoparticles. Polymers 11 (9), 1449. doi:10.3390/polym11091449
Zhai, Z., Leng, B., Yang, N., Yang, B., Liu, L., Huang, N., et al. (2019). Rational construction of 3D-networked carbon nanowalls/diamond supporting CuO architecture for high-performance electrochemical biosensors. Small 15 (48), 1901527. doi:10.1002/smll.201901527
Zhang, Z., Ou, X., Ma, L., Li, C., Yang, Z., and Duan, J. (2024). A double methylene blue labeled single-stranded DNA and hairpin DNA coupling biosensor for the detection of Fusarium oxysporum f. sp. cubense race 4. Bioelectrochemistry 156, 108612. doi:10.1016/j.bioelechem.2023.108612
Zheng, L., Cai, G., Qi, W., Wang, S., Wang, M., and Lin, J. (2019). Optical biosensor for rapid detection of Salmonella typhimurium based on porous gold@ platinum nanocatalysts and a 3D fluidic chip. ACS sensors 5 (1), 65–72. doi:10.1021/acssensors.9b01472
Zheng, Q., Huang, W., Cui, X., Dong, Y., Shi, Y., Ma, H., et al. (2018). Identification of wheat yellow rust using optimal three-band spectral indices in different growth stages. Sensors 19 (1), 35. doi:10.3390/s19010035
Zhu, W., Li, L., Zhou, Z., Yang, X., Hao, N., Guo, Y., et al. (2020). A colorimetric biosensor for simultaneous ochratoxin A and aflatoxins B1 detection in agricultural products. Food Chem. 319, 126544. doi:10.1016/j.foodchem.2020.126544
Keywords: biosensors, nanobiosensors, pathogen detection, environmental monitoring, food security, real-time monitoring
Citation: Chaturvedi A, Tripathi D and Ranjan R (2025) Nano-enabled biosensors in early detection of plant diseases. Front. Nanotechnol. 7:1545792. doi: 10.3389/fnano.2025.1545792
Received: 15 December 2024; Accepted: 11 March 2025;
Published: 04 April 2025.
Edited by:
Stefano Stassi, Polytechnic University of Turin, ItalyReviewed by:
Abdellatif Ait Lahcen, Old Dominion University, United StatesCopyright © 2025 Chaturvedi, Tripathi and Ranjan. This is an open-access article distributed under the terms of the Creative Commons Attribution License (CC BY). The use, distribution or reproduction in other forums is permitted, provided the original author(s) and the copyright owner(s) are credited and that the original publication in this journal is cited, in accordance with accepted academic practice. No use, distribution or reproduction is permitted which does not comply with these terms.
*Correspondence: Rajiv Ranjan, cmFqaXZyYW5qYW5idEBnbWFpbC5jb20=
†These authors have contributed equally to this work
Disclaimer: All claims expressed in this article are solely those of the authors and do not necessarily represent those of their affiliated organizations, or those of the publisher, the editors and the reviewers. Any product that may be evaluated in this article or claim that may be made by its manufacturer is not guaranteed or endorsed by the publisher.
Research integrity at Frontiers
Learn more about the work of our research integrity team to safeguard the quality of each article we publish.