- 1Department of Allied and Public Health, School of Health, Sport and Bioscience, University of East London, London, United Kingdom
- 2Department of Research and Innovation, Medway NHS Foundation Trust, Gillingham, United Kingdom
- 3Division of Sustainable Development, College of Science and Engineering, Hamad Bin Khalifa University, Qatar Foundation, Doha, Qatar
- 4Department of Chemistry and Biochemistry, University of Arizona, Tucson, AZ, United States
- 5Department of Chemistry, Virginia Tech University, Blacksburg, VA, United States
- 6Department of Comparative Biomedical Science, College of Veterinary Medicine, Mississippi State University, Stoneville, MS, United States
- 7Department of Chemistry, Faculty of Science, University of Ibadan, Ibadan, Nigeria
Waterborne microbial contamination poses significant threats to public health and environmental sustainability. Traditional water treatment methods, while effective to a certain extent, are often limited in their ability to completely eradicate microbial pathogens and mitigate emerging challenges such as disinfection by-products and microbial resistance. In recent years, nanoparticles have emerged as promising candidates for microbial control in water treatment due to their unique physicochemical properties and antimicrobial efficacy. This review provides a comprehensive examination of the use of nanoparticles for microbial control in water treatment, focusing on their antimicrobial mechanisms, applications, and ecological implications. The review discusses the types of nanoparticles commonly used in water treatment, including silver nanoparticles, copper nanoparticles, titanium dioxide nanoparticles, and carbon-based nanoparticles, and examines their antimicrobial mechanisms, such as cell membrane damage, reactive oxygen species generation, and interference with microbial metabolic processes. Furthermore, the review explores the applications of nanoparticles in the disinfection of drinking water, wastewater treatment, water purification in remote areas, and biofilm control. Additionally, the ecological implications of nanoparticle-based water treatment, including nanoparticle release into the environment, environmental persistence, toxicity to non-target organisms, and regulatory challenges, are critically evaluated. Finally, future perspectives and challenges in nanoparticle-based water treatment, such as enhanced nanoparticle synthesis and stability, development of sustainable treatment technologies, integration with conventional methods, and addressing knowledge gaps, are discussed. Overall, this review provides valuable insights into the potential of nanoparticles as innovative tools for addressing microbial contamination in water treatment while highlighting the need for further research and sustainable practices to ensure their safe and effective implementation.
1 Introduction
Efficient wastewater treatment, crucial for meeting both effluent and drinking water quality standards, hinges significantly on the efficacy of the disinfection stage. Traditional disinfection methods, such as chlorination and ultraviolet radiation, while widely utilized due to their effectiveness, present notable disadvantages (Rikta, 2019). Chlorination, for instance, can lead to the formation of harmful disinfection by-products (DBPs) like trihalomethanes and haloacetic acids, which are linked to an increased risk of carcinogenicity, mutagenicity, cytotoxicity, teratogenicity, and other health issues (Srivastav et al., 2020). Moreover, physical methods like ultraviolet radiation require substantial energy inputs and have limited efficacy against some resistant microbial strains, such as tetracycline-resistant Escherichia coli (Huang et al., 2013, Huang et al., 2016). These drawbacks underscore the need for exploring alternative disinfection technologies that can overcome these limitations while ensuring safe water.
Over the past decade, significant technological advancements have been made in water treatment processes, among which the use of nanomaterials for wastewater disinfection has emerged as a particularly notable innovation (Zhao et al., 2023). Nanoparticles are minuscule particles that exhibit unique physical and chemical properties due to their small size (<100 nm) and large surface-to-volume ratio (Salem et al., 2022). These properties include enhanced reactivity, strength, and electrical characteristics, which make them highly versatile for various applications (Khan S. A. et al., 2022). In the context of wastewater treatment, nanoparticles such as silver (nAg), fullerenes, and carbon nanotubes (CNT) have garnered attention for their potent antimicrobial properties (Epelle et al., 2022). These materials operate through diverse mechanisms, including the photocatalytic production of reactive oxygen species, disruption of microbial cell envelopes, and interference with essential biological processes like energy transduction and DNA synthesis (Li et al., 2008; Rikta, 2019). Such actions render them effective against a wide array of microbial pathogens commonly found in wastewater (Mahendra et al., 2009). The incorporation of nanoparticles into water treatment systems can significantly improve disinfection efficiency by overcoming the limitations of traditional methods, such as the formation of harmful byproducts in chlorination or the high energy requirements of ultraviolet radiation (Rikta, 2019; Epelle et al., 2022).
Nanoparticles combat microbial contaminants through a variety of sophisticated mechanisms, each tailored to the unique properties of the nanomaterial involved. For instance, titanium dioxide (TiO2) nanoparticles leverage their photocatalytic properties under UV light to generate reactive oxygen species (ROS), such as hydroxyl radicals and peroxide, which are lethal to microorganisms (Mahendra et al., 2009). This action disrupts vital cellular components, leading to the inactivation of bacteria and viruses. Similarly, silver nanoparticles (AgNPs) exert their antimicrobial effects by releasing Ag+ ions, which interfere with the function of cellular proteins and DNA, ultimately leading to cell death (Vasiliev et al., 2023). Additionally, carbon nanotubes (CNT) employ a physical mechanism by compromising the integrity of the bacterial cell envelope through direct contact, causing structural damage that results in cell leakage and death (Mahendra et al., 2009; Asaftei et al., 2023). These diverse mechanisms highlight the versatility of nanoparticles in targeting and inactivating a wide array of pathogens, making them highly effective as disinfectants in water treatment processes.
Nanotechnology offers several compelling advantages over traditional disinfection methods in wastewater treatment. Nanoparticles like TiO2 and silver demonstrate high efficacy against a broad spectrum of pathogens, including those resistant to conventional treatments, and do so with lower energy consumption and without the need for hazardous chemicals (Chakhtouna et al., 2021). These attributes underscore the transformative potential of nanotechnology in ensuring safer, more sustainable water treatment solutions. Given these significant advantages, this narrative review paper aims to exhaustively examine the recent advancements in nanoparticle technology and assess their feasibility for practical, large-scale applications shortly. By exploring current research and development, the paper seeks to bridge the gap between laboratory efficacy and real-world implementation, providing a comprehensive analysis of how nanotechnology can revolutionize water treatment practices and contribute to global health and sustainability goals.
2 Method
A comprehensive literature search was conducted to identify relevant studies on the use of nanoparticles for microbial control in water treatment. Data extraction was performed to gather relevant information from literature. Key information extracted from each article included nanoparticle type, synthesis method, physicochemical properties, antimicrobial mechanisms, effectiveness in water treatment, and ecological implications. Data were organized and synthesized to provide a comprehensive overview of the current state of knowledge regarding the use of nanoparticles for microbial control in water treatment. The extracted data were analyzed to identify trends, patterns, and gaps in the literature. Common themes and findings from reviewed literature were synthesized to provide insights into the efficacy, mechanisms, and challenges associated with nanoparticle-based water treatment. The implications of the findings for water treatment practice and recommendations for future research were discussed based on the analysis of the data.
3 Types of nanoparticles for microbial control
Antimicrobial resistance is emerging as a significant public health threat globally, underscoring the critical need for innovative solutions to combat this escalating challenge (Aber et al., 2023; Aijaz et al., 2023). In response, nanomaterials have been identified as effective tools for addressing these growing concerns, as shown in Table 1. Nanoparticles (NPs) are classified relative to composition, morphology, and application. Common classifications include metallic NPs, such as silver, copper, zinc, and gold, among others. They are mostly explored for antimicrobial and medical diagnostics. Nanoparticles (NPs), with their distinct physicochemical properties, are gaining prominence as potent agents for antimicrobial applications. Their effectiveness in microbial control is notably attributed to their potential for non-toxic release after application (Rikta, 2019). Notable examples of nanomaterials that exhibit antimicrobial properties include silver, titanium dioxide, copper, and carbon-based nanoparticles (Mahendra et al., 2009). These nanoparticles disrupt microbial activity primarily by damaging cell membranes, thus providing a viable mechanism for inhibiting microbial growth. Furthermore, the ability to tailor the surface properties of nanoparticles enables the development of targeted strategies against specific pathogens, thus opening new avenues in the design of antimicrobial agents.
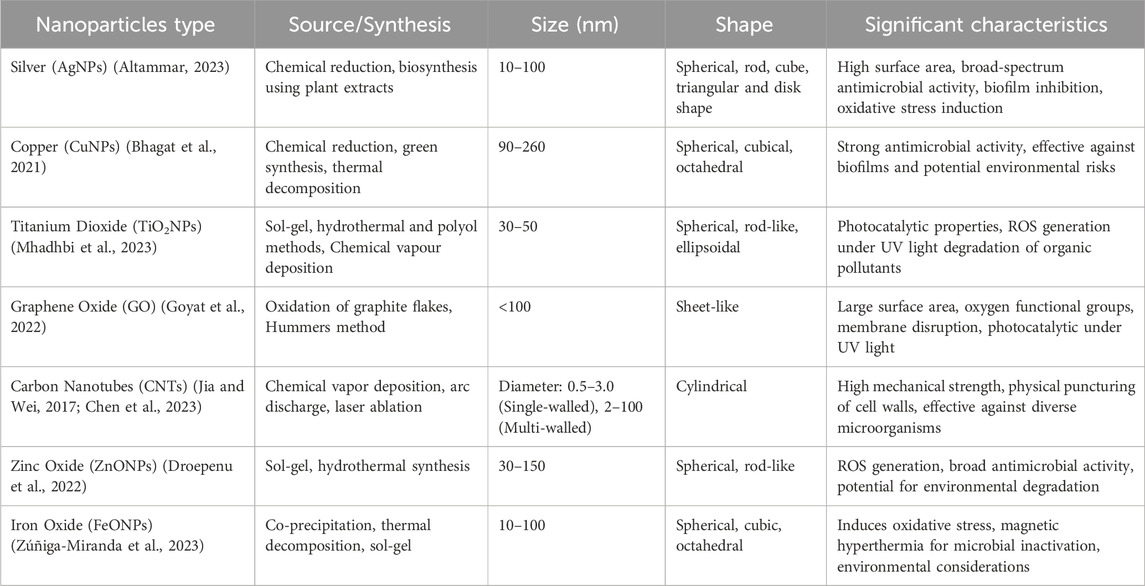
Table 1. Recent Advances in Nanoparticles for Microbial Control: Synthesis, Properties, and significant Characteristics.
Likewise, polymer-based NPs, like dendrimers, are explored in drug delivery systems because of their biocompatibility and modified release. Another significant category is carbon-based NPs, including fullerenes and carbon nanotubes, which are notable for their excellent electrical conductivity and strength. In this paper, we extensively examine the commonly applied nanoparticles for microbial control in water.
3.1 Silver nanoparticles
Silver nanoparticles (AgNPs) have emerged as promising antimicrobial agents with broad-spectrum activity against various microorganisms, including bacteria, viruses, fungi, and protozoa (Yah and Simate, 2015). Their antimicrobial efficacy is attributed to their high surface area-to-volume ratio, which facilitates interactions with microbial cells (Sayed et al., 2022). AgNPs exert their antimicrobial effects through multiple mechanisms, including disruption of cell membrane integrity, inhibition of cellular respiration, and induction of oxidative stress. Several studies have demonstrated the effectiveness of AgNPs in water disinfection applications, showing significant reductions in microbial populations even at low concentrations (Bahcelioglu et al., 2021; Khan Y. et al., 2022). Furthermore, AgNPs have shown potential for inhibiting biofilm formation and controlling antibiotic-resistant bacteria, highlighting their versatility and promising applications in combating microbial contamination in water sources (Polinarski et al., 2021). In addition to documented AgNP antimicrobial properties, they have been the subject of recent studies, especially their mechanism of action at a molecular level. In this study, we have gathered that silver ions released from AgNPs not only disrupt the outer membrane but also precipitate vital cellular components, leading to rapid microbial death.
3.2 Copper nanoparticles
Copper nanoparticles (CuNPs) have garnered attention for their potent antimicrobial properties, particularly against bacteria (Edis et al., 2019; Nisar et al., 2019). CuNPs release copper ions that penetrate microbial cell membranes, leading to disruption of membrane integrity and interference with cellular processes. Studies have shown that CuNPs exhibit strong antimicrobial activity against a wide range of bacterial pathogens, including both Gram-positive and Gram-negative bacteria, with impressive reports of 99% microbial inhibition concentration and 100% minimum bactericidal concentration (Yadav L. et al., 2017; Sharma et al., 2022). Additionally, CuNPs have been explored for their potential to control bacterial biofilms, which are notoriously resistant to conventional disinfection methods. Despite their effectiveness, the use of CuNPs in water treatment raises concerns about potential environmental impacts, such as copper accumulation in aquatic ecosystems and the development of copper-resistant microorganisms, necessitating further research to evaluate their long-term efficacy and safety (Keller et al., 2017; Malhotra et al., 2020). Meanwhile, for minimal environmental impact, a controlled release or application of CuNPs would significantly prevent the rapid development of resistance among the microbial populations.
3.3 Titanium dioxide nanoparticles
Titanium dioxide nanoparticles (TiO2NPs) have garnered attention for their photocatalytic properties, which enable them to generate reactive oxygen species (ROS) upon exposure to UV irradiation. These ROS, including hydroxyl radicals and superoxide ions, exhibit strong oxidizing potential, leading to damage to microbial cell membranes, proteins, and nucleic acids (Canaparo et al., 2020; Juan et al., 2021; Sargazi et al., 2022). TiO2NPs have demonstrated effectiveness against a wide range of microorganisms, including bacteria, viruses, and algae, making them promising candidates for water disinfection and purification. Moreover, TiO2NPs have been investigated for their potential to degrade organic pollutants and remove heavy metals from water sources (Mohammad Gheimasi et al., 2021), further highlighting their versatility and potential for sustainable water treatment applications. Additionally, ongoing research is optimizing the doping of TiO2NPs with other metals to tune their photocatalytic activity across a broader spectrum of light.
3.4 Carbon-based nanoparticles
Carbon-based nanoparticles, such as graphene oxide (GO) and carbon nanotubes (CNTs), have shown promise as antimicrobial agents due to their unique physicochemical properties (Smith and Rodrigues, 2015; Hadidi and Mohebbi, 2022). GO possesses a large surface area and numerous oxygen-containing functional groups, allowing it to interact with microbial cells and disrupt their membranes. Additionally, GO exhibits photocatalytic activity under UV irradiation, further enhancing its antimicrobial efficacy (Sun et al., 2017). Similarly, CNTs exhibit sharp edges that can physically puncture microbial cell walls, leading to cell lysis (Al-Jumaili et al., 2017). Both GO and CNTs have demonstrated effectiveness against a wide range of microorganisms, including bacteria, viruses, and fungi, making them potential candidates for water disinfection and biofilm control (Al-Jumaili et al., 2017; Mohammed et al., 2020). However, concerns about the environmental fate and toxicity of carbon-based nanoparticles necessitate further research to evaluate their long-term impacts on aquatic ecosystems and human health (Patil and Lekhak, 2020).
3.5 Other nanoparticles
In addition to the aforementioned nanoparticles, various other types of nanoparticles are being explored for their antimicrobial properties and potential applications in water treatment. Zinc oxide nanoparticles (ZnONPs) have shown effectiveness against bacteria, viruses, and fungi due to their ability to generate ROS and disrupt microbial cell membranes. Iron oxide nanoparticles (FeONPs) have been investigated for their potential to induce oxidative stress and magnetic hyperthermia in microbial cells, leading to microbial inactivation. Hybrid nanoparticles, composed of multiple materials, offer synergistic antimicrobial effects and enhanced stability, presenting additional options for microbial control in water treatment. Overall, nanoparticles represent a diverse and promising array of antimicrobial agents for addressing microbial contamination in water sources, offering innovative solutions for sustainable water treatment and public health protection. Table 1 provides a summary of the different nanoparticle types and their distinct characteristics, while Table 2 delves into their mechanism of action in water disinfection processes.
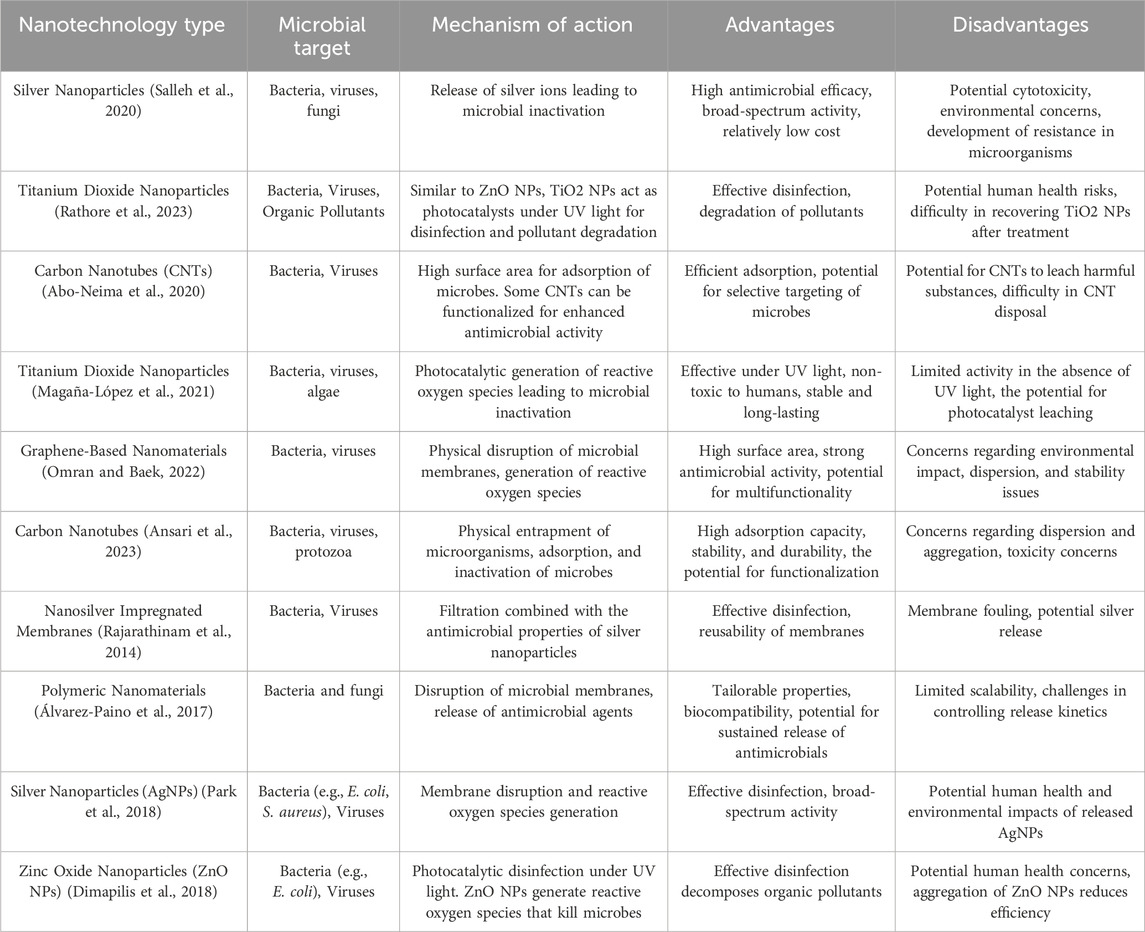
Table 2. Innovative nanotechnologies for targeted water disinfection: Mechanisms, advantages, and disadvantages.
4 Antimicrobial mechanisms of nanoparticles
Nanoparticles have gained recognition for their potent antimicrobial properties, which arise from diverse mechanisms of action (Oluwasanu et al., 2019; Rikta, 2019; Zhao et al., 2023), as shown in Figure 1. Understanding these mechanisms is crucial for harnessing the full potential of nanoparticles in combatting microbial contamination in various settings, including water treatment, healthcare, and food preservation.
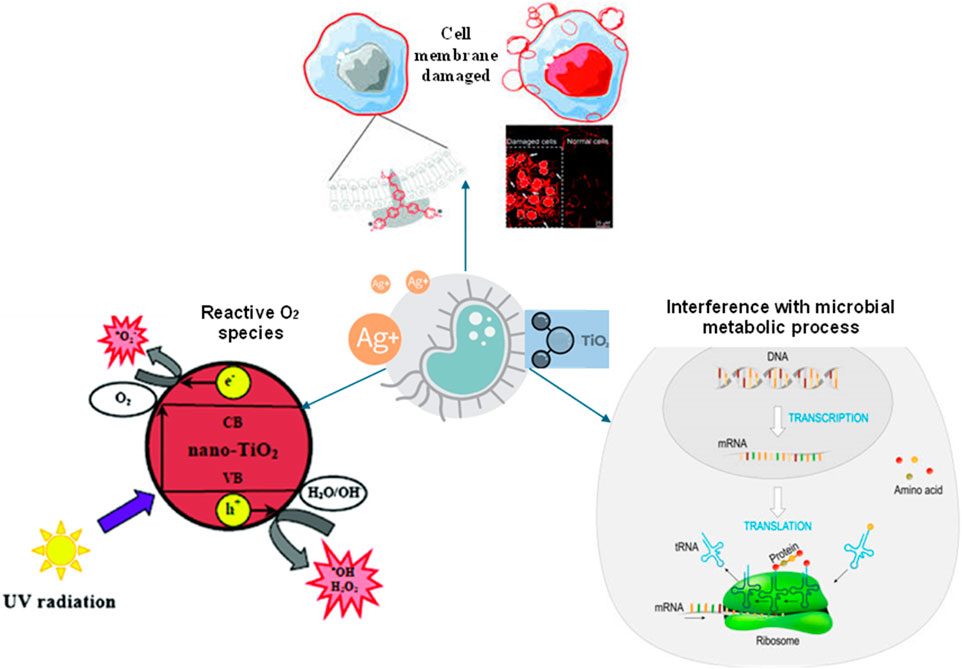
Figure 1. Illustration of the diverse mechanisms through which nanoparticles exert antimicrobial effects, including cell membrane damage, reactive oxygen species (ROS) generation, and interference with microbial metabolic processes.
4.1 Cell membrane damage
One of the primary mechanisms through which nanoparticles exert their antimicrobial effects is by inducing damage to microbial cell membranes. Nanoparticles, particularly those with sharp edges or high surface reactivity, can physically penetrate microbial cell walls, leading to disruption of membrane integrity and leakage of cellular contents (Wu et al., 2018; Sinha et al., 2022). This disruption impairs essential cellular functions, such as nutrient uptake, waste removal, and maintenance of osmotic balance, ultimately leading to microbial death. Several studies have demonstrated the ability of nanoparticles, such as AgNPs, CNTs, and GO, to cause structural damage to microbial cell membranes, resulting in increased membrane permeability and loss of membrane integrity (Kunduru et al., 2017; Wu et al., 2018; Rikta, 2019). Additionally, nanoparticles undergo dissolution, leading to leaching out of ions, which may interact with membrane-bound proteins and lipids, further compromising membrane stability and function (Ritu et al., 2023). Overall, cell membrane damage represents a crucial antimicrobial mechanism of nanoparticles, contributing to their efficacy against a wide range of microbial pathogens.
4.2 Reactive oxygen species (ROS) generation
Another important antimicrobial mechanism of nanoparticles involves the generation of reactive oxygen species (ROS), such as hydroxyl radicals (•OH), superoxide ions (O2•−), and singlet oxygen (1O2) (Dey et al., 2018; Rikta, 2019; Franco et al., 2022; Ikram et al., 2023). Nanoparticles, particularly those composed of metal oxides (e.g., titanium dioxide nanoparticles, zinc oxide nanoparticles) or capable of photocatalysis (e.g., titanium dioxide nanoparticles), can absorb photons and transfer energy to surrounding oxygen molecules, leading to the generation of ROS through photochemical reactions (El-Sayyad et al., 2023; Younis et al., 2023). These ROS exhibit potent oxidizing properties, causing damage to microbial cell membranes, proteins, and nucleic acids. Moreover, ROS induces oxidative stress responses in microbial cells, leading to the activation of antioxidant enzymes and DNA repair mechanisms, as shown in Figure 2. However, excessive ROS production overwhelms these defense mechanisms, ultimately leading to oxidative damage and microbial death. Several studies have demonstrated the ability of nanoparticles to generate ROS and induce oxidative stress in microbial cells, highlighting the importance of this mechanism in nanoparticle-mediated antimicrobial activity (Wu et al., 2018; Shi et al., 2019; Khan M. I. et al., 2021; Dutta et al., 2023; Guerrero-Almonacid et al., 2023; Ikram et al., 2023).
4.3 Interference with microbial metabolic processes
Nanoparticles can also exert antimicrobial effects by interfering with microbial metabolic processes essential for cell survival and proliferation (Mtavangu et al., 2022). For example, nanoparticles may disrupt enzymatic reactions involved in energy production, biosynthesis, and nutrient metabolism, leading to metabolic dysfunction and cellular toxicity (Ritu et al., 2023). Additionally, nanoparticles may interfere with microbial DNA replication, transcription, and translation processes, impairing cellular proliferation and growth (Crisan et al., 2021; Rosli et al., 2021), as shown in Figure 2. Furthermore, nanoparticles may disrupt microbial quorum sensing mechanisms, which regulate gene expression and coordinate population-wide behaviours, such as biofilm formation and virulence factor production (Franci et al., 2015). By targeting multiple metabolic pathways and cellular processes, nanoparticles can effectively inhibit microbial growth and survival (He et al., 2022). Several studies have demonstrated the ability of nanoparticles, such as silver nanoparticles and copper nanoparticles, to disrupt microbial metabolic processes and inhibit the growth of bacterial and fungal pathogens (Rajabi et al., 2017; Oquendo-Cruz and Perales-Pérez, 2018; Saha et al., 2018; Shi et al., 2019; Adeyemi et al., 2020; Noohpisheh et al., 2020; Xiao et al., 2021; Sinha et al., 2022). Overall, interference with microbial metabolic processes represents a multifaceted antimicrobial mechanism of nanoparticles, contributing to their broad-spectrum activity against diverse microbial pathogens.
4.4 Combination therapy
Combination therapy, involving the simultaneous or sequential administration of multiple antimicrobial agents, represents a promising strategy for enhancing the efficacy of nanoparticle-based antimicrobial treatments (Ahmed et al., 2019; Singh et al., 2022). Combining nanoparticles with different mechanisms of action or synergistic antimicrobial agents, such as antibiotics, antimicrobial peptides, or natural antimicrobial compounds, can achieve enhanced antimicrobial activity, overcome microbial resistance, and reduce the risk of treatment failure. Several studies have demonstrated the effectiveness of combination therapy using nanoparticles and conventional antimicrobial agents against multidrug-resistant bacterial and fungal pathogens (Ahmed et al., 2019; Yang et al., 2019; Singh et al., 2022; Markowicz, 2023). Moreover, combination therapy allows for lower doses of individual agents, minimizing potential adverse effects and toxicity. However, optimal combination regimens, dosage formulations, and treatment protocols need to be carefully evaluated to maximize therapeutic efficacy while minimizing the risk of adverse effects and antimicrobial resistance development. Overall, combination therapy represents a promising approach for enhancing the antimicrobial efficacy of nanoparticles and combating microbial contamination in various settings. Figure 1 summarises the different mechanisms employed by NPs for antimicrobial activities.
4.5 Influence of environmental parameters
The antibacterial efficacy of nanoparticles, such as silver nanoparticles (AgNPs), copper nanoparticles (CuNPs), titanium dioxide nanoparticles (TiO₂NPs), and zinc oxide nanoparticles (ZnONPs), is significantly influenced by various environmental parameters, including pH, temperature, ionic strength, and the presence of organic matter (Dobrescu et al., 2023; Adetuyi et al., 2024). For instance, the pH of the environment can alter the surface charge and solubility of these nanoparticles, affecting their interaction with microbial cells (Badawy et al., 2010). Studies have shown that AgNPs exhibit optimal antibacterial activity at neutral to slightly alkaline pH levels, where their stability and reactivity are enhanced (Bélteky et al., 2019; Gibała et al., 2021). Similarly, CuNPs and ZnONPs also display varying degrees of antimicrobial effectiveness depending on the pH conditions (Wang et al., 2015; Ribut et al., 2018). Temperature is another crucial factor that affects the antibacterial activity of nanoparticles. Higher and lower temperatures were reported to affect the antibacterial activity of nanoparticles (Qu et al., 2010; Pourali et al., 2013). Ionic strength, determined by the concentration of salts in the water, can affect the aggregation state of nanoparticles. High ionic strength environments can induce nanoparticle aggregation, reducing their surface area and subsequent antimicrobial activity (Chambers et al., 2014; Wang et al., 2020). Additionally, the presence of organic matter, such as humic substances, can interact with nanoparticles, forming a corona that may either enhance or hinder their antibacterial properties (Fabrega et al., 2009; Wang et al., 2015). For example, organic matter can increase the stability and dispersion of nanoparticles in water, but it can also reduce their availability to interact with bacterial cells by acting as a physical barrier. Therefore, understanding and optimizing these environmental parameters is essential for maximizing the antibacterial activity of nanoparticles like AgNPs, CuNPs, TiO₂NPs, and ZnONPs in various water treatment applications.
5 Applications of nanoparticles in water treatment
Nanoparticles are rapidly evolving in the field of water treatment, offering innovative approaches to combat water pollution and scarcity, as shown in Figure 2. Their unique physicochemical characteristics, coupled with a remarkably high surface area-to-volume ratio, enable a diverse range of applications that contribute to purifying, remediating, and disinfecting water sources. From eliminating contaminants to improving filtration processes, nanoparticles play a crucial role in enhancing water quality and ensuring access to clean drinking water. Their versatile applications span various techniques and technologies, including adsorption, catalysis, membrane filtration, and advanced oxidation processes. These multifaceted uses highlight the potential of nanoparticles to transform water treatment methods, providing sustainable and effective solutions to environmental challenges and public health concerns. Figure 2 provides a summary of NPs’ utilization in water treatment.
5.1 Disinfection of drinking water
In the realm of drinking water treatment, nanoparticles offer novel approaches to disinfection, aiming to improve water quality and safeguard public health (Eloffy et al., 2021). AgNPs have been extensively studied for their efficacy in disinfecting drinking water due to their potent antimicrobial properties (Rus et al., 2017; Deshmukh, et al., 2019; Bhardwaj et al., 2021). Silver nanoparticle-infused ceramic filters outperformed those lacking nanoparticles and exhibited superior bacteria removal in both laboratory and field trials in Guatemala. Deployed to 62 households, these filters achieved an average 90% reduction in total coliforms and E. coli while maintaining an effluent concentration of ionic silver 0.02 mg/L below the U.S. EPA standard of 0.1 mg/L (Kallman et al., 2011). AgNPs can effectively target a wide range of microorganisms, including bacteria, viruses, and protozoa, by disrupting cellular functions and inhibiting microbial growth (Kowalczyk et al., 2021). Studies have demonstrated that AgNPs can achieve rapid and effective disinfection of water, even at low concentrations, making them promising candidates for replacing or supplementing traditional disinfection methods such as chlorination (Yang, 2016) The antiviral effects of AgNPs within a continuous system were investigated, alongside their antibacterial properties, utilizing a “phase inversion” technique to synthesize membranes impregnated with AgNPs, which exhibited potent inactivation against MS2 coliphage, E. coli K12, and Pseudomonas mendocina KR1(Zodrow et al., 2009). A Belgian team explored viral inactivation using biogenic nanosilver immobilized in polyvinylidene fluoride (PVDF) membranes. Employing a continuous flow system with these membranes, they achieved approximately 4-log removal of UZ1 bacteriophage after 2 h at a flow rate of 0.375 L/h and a hydraulic retention time (HRT) of 1 h while maintaining a low Ag+ concentration of 27 ± 8 μg/L in the treated water (De Gusseme et al., 2010).
AgNPs have shown capabilities in enhancing water disinfection processes. Unlike conventional methods, they minimize the risk of creating disinfection by-products, often associated with carcinogenic risks. This property, alongside their efficient performance and lower energy requirements, makes AgNPs a favorable alternative in water treatment technologies (Bahcelioglu et al., 2021). Additionally, TiO2NPs have gained attention for their photocatalytic activity, which enables them to generate ROS under UV irradiation, causing them to act as bacterial disinfectants (Liao et al., 2020). TiO2NPs have shown effectiveness against bacteria, viruses, and protozoa, making them suitable for disinfection applications in drinking water treatment plants (Michael-Kordatou et al., 2018). Despite their stability during the degradation of contaminants and microorganisms, TiO2 nanoparticles require UV lamps for activation due to their large bandgap energy. A molecularly imprinted TiO2 photocatalyst was developed via the sol-gel method, demonstrating its efficacy in selectively degrading the herbicide- 2,4-dichlorophenoxyacetic acid and the insecticide-imidacloprid (Fiorenza et al., 2019). Overall, nanoparticles offer promising solutions for enhancing the disinfection of drinking water, providing safer and healthier water sources for communities worldwide.
5.2 Wastewater treatment
Nanoparticles also hold significant potential for wastewater treatment, offering opportunities to remove contaminants and pollutants from wastewater streams effectively. In wastewater treatment plants, nanoparticles can be employed in various processes, including coagulation, flocculation, adsorption, and photocatalysis, to improve the removal efficiency of organic and inorganic pollutants (Khan S. et al., 2021; Jabbar et al., 2022). For example, iron-based nanoparticles, such as iron oxide nanoparticles (FeONPs), have been utilized as adsorbents for the removal of heavy metals and organic pollutants from wastewater because they can selectively adsorb contaminants onto their surfaces, facilitating their removal through sedimentation or filtration processes (Ajith et al., 2021). Efficacy of FeNPs and FeONPs in removing Cd2+ (Ebrahim et al., 2015), Cu2+ (Poguberović et al., 2016), Co2+ and Ni2+ (Hooshyar et al., 2013) and chlorinated organic contaminants ((Guo et al., 2017) has been extensively studied and reported.
Similarly, carbon-based nanoparticles, such as GO and CNTs, have shown promise for adsorbing organic compounds and pharmaceuticals from wastewater streams owing to their high surface area and affinity for hydrophobic molecules (Thakur et al., 2024). Furthermore, photocatalytic nanoparticles, such as TiO2NPs and zinc oxide nanoparticles (ZnONPs), can be employed to degrade organic pollutants and disinfect wastewater under UV irradiation (Yashni et al., 2021). These nanoparticles generate ROS, which reacts with organic molecules, leading to their degradation and mineralization (Sultana et al., 2020). Overall, nanoparticles offer versatile and effective solutions for wastewater treatment, enabling the removal of contaminants and the production of clean water for either reuse or discharge into the environment (Hlongwane et al., 2019).
5.3 Water purification in remote areas
Nanoparticles also hold promise for water purification in remote or resource-limited areas where access to clean and safe drinking water is limited. Portable and low-cost nanoparticle-based water purification technologies have been developed to provide sustainable solutions for addressing waterborne diseases and improving public health outcomes in underserved communities (Kumar, 2023). For example, AgNPs can be incorporated into point-of-use water treatment devices, such as ceramic filters or membrane cartridges, to disinfect water and remove microbial contaminants (Kannan et al., 2023; Rowles, et al., 2023). These devices utilize the antimicrobial properties of AgNPs to inactivate pathogens and provide safe drinking water to households without access to centralized water treatment infrastructure. Similarly, GO-based membranes have been developed for desalination and water purification applications, offering high water permeability and selectivity for removing salts and organic compounds from water sources (Song et al., 2018). These nanoparticle-based technologies have the potential to significantly improve access to clean water in remote areas, contributing to poverty alleviation, health promotion, and sustainable development.
5.4 Biofilm control
Biofilms, complex microbial communities embedded in a matrix of extracellular polymeric substances, pose significant challenges in water treatment systems, leading to fouling, corrosion, and contamination (Shukla et al., 2021). Nanoparticles offer innovative strategies for controlling biofilm formation and mitigating its adverse effects on water quality and system performance (Mohanta et al., 2023). Metal-based nanoparticles, such as AgNPs and CuNPs, have shown efficacy in inhibiting biofilm formation and disrupting established biofilms through their antimicrobial properties (Mammari et al., 2022).
Using mixed metal oxides in precise ratios was reported to have a more significant impact than utilizing a free metal oxide, such as ZnO:MgO NPs, which at low concentrations prevent Bacillus subtilis and P. mirabilis from forming biofilms (Mohanta et al., 2023) and the zinc-doped copper oxide (Zn:CuO NPs) coated teeth improved the killing of S. mutans and lowered biofilm development by 88% as opposed to 70% with free CuO NPs (Eshed et al., 2014). These nanoparticles can penetrate the biofilm matrix and target microbial cells, leading to cell membrane damage and microbial death (Li et al., 2021). Additionally, nanoparticles can be incorporated into coatings, membranes, or surfaces to prevent biofilm formation and promote the self-cleaning properties of water treatment equipment (Zhang et al., 2016; Mi et al., 2018). For example, GO coatings have been shown to inhibit biofilm formation on surfaces by preventing microbial adhesion and growth, with biofilm inhibition percentage reaching 90%–100% at GO contents of 180 and 200 μg (Yadav N. et al., 2017). Furthermore, nanoparticles can be employed in combination with conventional biocides or antimicrobial agents to enhance biofilm control efficacy and minimize the risk of microbial resistance development (Liu et al., 2017). Therefore, nanoparticles offer promising solutions for biofilm control in water treatment systems, improving system efficiency, longevity, and water quality.
Table 3 provides a comparative analysis of the antimicrobial activity of NPs, their applications, and ecological implications.
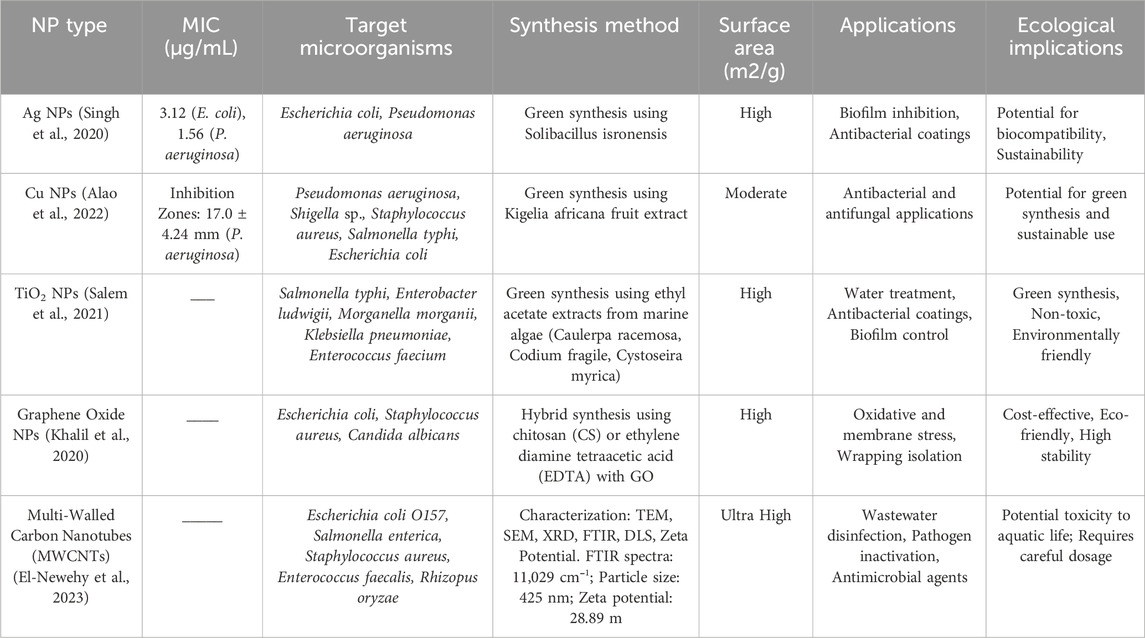
Table 3. Comparative analysis of antibacterial activity, applications and implications of nanoparticles in water treatment.
6 Ecological implications of nanoparticle-based water treatment
The integration of engineered nanomaterials in water treatment processes represents a groundbreaking advancement in environmental management. Nanoparticles have shown immense promise in water treatment, but their use raises concerns regarding potential ecological implications. The potential ecological impacts of nanoparticle residues in treated water are multifaceted. There is a risk that nanoparticles could exhibit toxicity to aquatic organisms, affecting their health and biodiversity. Likewise, studies have shown the possibilities of nanoparticle bioaccumulation in living tissue (Lasagna-Reeves et al., 2010; Lin et al., 2015), leading to physiological and reproductive toxicity (Liu et al., 2016; Brohi et al., 2017). There is also concern about the half-life of these nanoparticles and their potential to catalyze unintended chemical reactions in the environment, leading to secondary pollution. Hence, understanding these implications is crucial for ensuring the sustainable and safe implementation of nanoparticle-based water treatment technologies. Moreover, the complexity of ecosystems necessitates a careful examination of the long-term ecological impacts of nanoparticle-based water treatment technologies. As these technologies become more widespread, it is imperative to develop frameworks for continuous environmental monitoring to detect and mitigate any unforeseen effects of nanoparticles on non-target organisms. Also, the knowledge of nanoparticle half-life before its usage will be vital in ensuring that environmental benefits outweigh the potential risks associated with their use.
6.1 Nanoparticle release into the environment
One of the primary ecological concerns associated with nanoparticle-based water treatment is the release of nanoparticles into the environment. During water treatment processes, nanoparticles may be released into receiving water bodies through effluent discharge or residual nanoparticle accumulation in sludge. Once released, nanoparticles can undergo transformations, such as aggregation, dissolution, and surface modification, which may alter their behaviour, fate, and toxicity in the environment (Levard et al., 2012; Turan et al., 2019). Moreover, nanoparticles may adsorb onto natural particles and colloids, facilitating their transport and dispersal in aquatic ecosystems. Studies have shown that nanoparticles, such as AgNPs and TiO2NPs, can persist in the environment for extended periods and accumulate in sediments, soils, and biota, raising concerns about their potential ecological impacts (Schaumann et al., 2015; Sinouvassane et al., 2016).
6.2 Environmental persistence
Another ecological implication of nanoparticle-based water treatment is the environmental persistence of nanoparticles. Nanoparticles can resist degradation and persist in the environment for long periods (Bundschuh et al., 2018; Abbas et al., 2020), leading to their accumulation and bioaccumulation in environmental compartments. The long-term environmental fate and behaviour of nanoparticles depend on various factors, including their physicochemical properties, interactions with environmental matrices, and exposure conditions. For example, metal-based nanoparticles, such as silver nanoparticles and copper nanoparticles, may undergo transformation processes, such as oxidation and sulfidation, which can influence their stability, mobility, and toxicity in aquatic environments. Additionally, carbon-based nanoparticles, such as GO and CNTs, may exhibit varying degrees of stability and persistence in natural waters, depending on their surface chemistry, aggregation state, and interactions with dissolved organic matter (Burdușel et al., 2018; Lead et al., 2018; Yu et al., 2018; Turan et al., 2019). Furthermore, nanoparticles can aggregate or disaggregate depending on the ionic strength and pH of their environment, which affects their distribution and persistence (Baalousha, 2009; Badawy et al., 2010). Understanding the environmental persistence of nanoparticles is essential for assessing their potential risks and designing effective mitigation strategies to minimize their environmental impacts.
6.3 Toxicity to non-target organisms
Nanoparticle-based water treatment also raises concerns about the potential toxicity of nanoparticles to non-target organisms in aquatic ecosystems (Bellanthudawa et al., 2023). While nanoparticles are designed to target microbial pathogens, they may inadvertently affect non-target organisms, including aquatic plants, invertebrates, and fish. The toxicity of nanoparticles to non-target organisms depends on various factors, including nanoparticle properties (e.g., size, shape, surface chemistry), exposure duration and concentration, and organism sensitivity. Studies have shown that nanoparticles, such as AgNPs and TiO2NPs, can induce adverse effects in aquatic organisms, including oxidative stress, DNA damage, and alterations in gene expression (Zou et al., 2014; Zeumer et al., 2020). Additionally, nanoparticles may bioaccumulate in aquatic organisms through dietary uptake or direct exposure, leading to potential biomagnification in food webs (Powell et al., 2010; Zhao and Wang, 2010). Therefore, assessing the ecotoxicity of nanoparticles to non-target organisms is essential for evaluating their environmental risks and establishing regulatory guidelines for their safe use in water treatment.
6.4 Risk assessment and regulation
Addressing the ecological implications of nanoparticle-based water treatment requires comprehensive risk assessment and regulation to ensure the protection of environmental health and ecosystem integrity. Risk assessment involves evaluating the hazards, exposure pathways, and potential risks associated with nanoparticle release and exposure in aquatic environments. This includes assessing the environmental fate and behaviour of nanoparticles, predicting their ecological impacts, and identifying sensitive receptors and ecosystems. Furthermore, regulatory frameworks and guidelines are needed to govern the safe use, disposal, and monitoring of nanoparticles in water treatment processes. Regulatory agencies, such as the Environmental Protection Agency (EPA) and the European Chemicals Agency (ECHA), play a crucial role in developing and implementing regulations for nanoparticle-based water treatment technologies (Hegde et al., 2016). These regulations may include restrictions on nanoparticle use, labelling requirements, monitoring and reporting obligations, and risk management measures to minimize environmental risks and ensure the sustainable use of nanoparticles in water treatment. Additionally, research efforts should focus on developing eco-friendly nanoparticle formulations, designing effective nanoparticle recovery and recycling technologies, and exploring alternative water treatment approaches that minimize environmental impacts while ensuring water quality and public health protection. Overall, addressing the ecological implications of nanoparticle-based water treatment requires a multidisciplinary approach involving collaboration between scientists, policymakers, industry stakeholders, and regulatory agencies to balance technological innovation with environmental sustainability.
7 Future perspectives and challenges
Nanoparticle-based water treatment technologies hold immense promise for addressing microbial contamination and improving water quality. However, several key challenges must be overcome to realize their full potential and ensure their sustainable implementation. Future research and development efforts should focus on addressing these challenges and advancing nanoparticle-based water treatment toward widespread adoption.
7.1 Enhanced nanoparticle synthesis and stability
One of the key challenges in nanoparticle-based water treatment is the synthesis of nanoparticles with enhanced stability, efficacy, and environmental compatibility (Nehra and Chauhan, 2019; Zhao et al., 2023). Current synthesis methods often involve complex chemical processes and may result in nanoparticles with variable properties and stability (Yang et al., 2016; Saha et al., 2018). Future research should focus on developing novel synthesis techniques that allow precise control over nanoparticle size, shape, surface chemistry, and stability. Additionally, efforts should be made to improve nanoparticle scalability and reproducibility to facilitate large-scale production and commercialization (Xiao et al., 2015). Moreover, strategies for enhancing nanoparticle stability in diverse water matrices and environmental conditions should be explored to ensure their long-term efficacy and safety in water treatment applications (Xiao et al., 2021).
7.2 Development of sustainable nanoparticle-based water treatment technologies
Another challenge in nanoparticle-based water treatment is the development of sustainable and environmentally friendly technologies that minimize energy consumption, chemical usage, and waste generation (Rosli et al., 2021). Current nanoparticle-based water treatment methods may involve high energy inputs, use of toxic chemicals, or produce secondary pollutants, raising concerns about their environmental impact (Rajabi et al., 2017; Yu et al., 2022; Zikalala et al., 2022). Future research should focus on developing sustainable nanoparticle synthesis methods using green chemistry principles and renewable resources (Rajabi et al., 2017; Saha et al., 2018; Noohpisheh et al., 2020; Ritu et al., 2023; Sachin et al., 2023). Additionally, efforts should be made to design nanoparticle-based water treatment systems that operate efficiently, consume minimal resources, and generate minimal waste. Integration of renewable energy sources, such as solar or hydroelectric power, into nanoparticle-based water treatment processes, can further enhance their sustainability and reduce their carbon footprint.
7.3 Integration of nanoparticles with conventional water treatment methods
To overcome the limitations of nanoparticle-based water treatment and maximize their effectiveness, nanoparticles must be integrated with conventional water treatment methods (Rikta, 2019). Combining nanoparticles with techniques such as filtration, coagulation, and membrane separation can enhance water treatment efficiency, improve pathogen removal, and reduce treatment costs. For example, nanoparticles can be immobilized onto filtration membranes to enhance their antimicrobial properties and prevent biofouling (Yu et al., 2022; Chakachaka et al., 2023; Zhan et al., 2023). Similarly, nanoparticles can be incorporated into coagulants or disinfectants to improve their efficacy and reduce chemical dosage requirements. Future research should focus on optimizing the integration of nanoparticles with conventional water treatment processes to achieve synergistic effects and overcome the limitations of individual techniques.
7.4 Addressing knowledge gaps and research needs
Despite significant advancements in nanoparticle-based water treatment, there remain several knowledge gaps and research needs that must be addressed to facilitate further progress in this field. One critical area for future research is the assessment of nanoparticle environmental fate, transport, and ecotoxicity in aquatic ecosystems. Understanding how nanoparticles interact with environmental matrices, undergo transformations, and affect non-target organisms is essential for evaluating their environmental risks and designing effective mitigation strategies (Parmar et al., 2022; Qi et al., 2022). Additionally, research efforts should focus on developing standardized methods for nanoparticle characterization, toxicity testing, and risk assessment to ensure consistency and comparability across studies. Moreover, further research is needed to explore the long-term impacts of nanoparticle-based water treatment on ecosystem health, biodiversity, and ecosystem services to inform sustainable water management practices (Mohammed, 2021).
8 Conclusion
This review has systematically explored the significant strides made in the application of nanoparticles for water disinfection, illuminating their potential to revolutionize water treatment methodologies. Across various sections, we delved into the antimicrobial mechanisms of nanoparticles such as silver, titanium dioxide, and copper, which include the generation of reactive oxygen species, disruption of microbial cell membranes, and interference with microbial metabolic processes. These mechanisms underline the capacity of nanoparticles to address pathogens resistant to conventional treatment methods while minimizing the adverse effects associated with traditional disinfectants such as chlorination.
Despite their promising antimicrobial efficacy, the application of nanoparticles in water disinfection is not devoid of challenges. Among these, the potential ecological impacts stand out, including the toxicity to non-target organisms and the environmental persistence of nanoparticles, which could lead to unforeseen ecological imbalances. The review also highlighted the technological advancements in the synthesis and stability of nanoparticles, which are critical for their practical application in large-scale water treatment systems.
Looking forward, it is essential to bridge the existing knowledge gaps in the long-term environmental and health impacts of nanoparticles. Future research should focus on developing safer nanoparticle formulations, enhancing their biodegradability, and understanding their interactions within complex environmental matrices. Moreover, integrating nanoparticles with existing water treatment technologies could provide a synergistic approach to enhance water quality and safety effectively. Ensuring regulatory compliance and conducting comprehensive risk assessments will be vital in advancing the sustainable use of nanoparticle technologies in water disinfection. Overall, this review underscores the necessity for continued research and development to optimize the benefits of nanotechnology in water treatment while mitigating its risks.
Author contributions
DBO: Conceptualization, Methodology, Project administration, Resources, Writing–original draft, Writing–review and editing. OZW: Conceptualization, Formal Analysis, Validation, Writing–original draft, Writing–review and editing. OF: Methodology, Validation, Writing–original draft, Writing–review and editing. BE: Data curation, Writing–original draft, Writing–review and editing. OA: Data curation, Writing–original draft, Writing–review and editing. AOI: Software, Writing–original draft, Writing–review and editing.
Funding
The author(s) declare that no financial support was received for the research, authorship, and/or publication of this article.
Conflict of interest
The authors declare that the research was conducted in the absence of any commercial or financial relationships that could be construed as a potential conflict of interest.
Publisher’s note
All claims expressed in this article are solely those of the authors and do not necessarily represent those of their affiliated organizations, or those of the publisher, the editors and the reviewers. Any product that may be evaluated in this article, or claim that may be made by its manufacturer, is not guaranteed or endorsed by the publisher.
References
Abbas, Q., Yousaf, B., Ali, M. U., Munir, M. A. M., El-Naggar, A., Rinklebe, J., et al. (2020). Transformation pathways and fate of engineered nanoparticles (ENPs) in distinct interactive environmental compartments: a review. Environ. Int. 138, 105646. doi:10.1016/j.envint.2020.105646
Aber, S., Shi, Z., Xing, K., Rameezdeen, R., Chow, C. W. K., Hagare, D., et al. (2023). Microbial desalination cell for sustainable water treatment: a critical review. Glob. Challenges 7 (10), 2300138. doi:10.1002/gch2.202300138
Abo-Neima, S. E., Motaweh, H. A., and Elsehly, E. M. (2020). Antimicrobial activity of functionalised carbon nanotubes against pathogenic microorganisms. IET Nanobiotechnology 14 (6), 457–464. doi:10.1049/iet-nbt.2019.0342
Adetuyi, B. O., Olajide, P. A., Omowumi, O. S., and Adetunji, C. O. (2024) “Application of plant-based nanobiopesticides as disinfectant,” in Handbook of agricultural biotechnology, volume 1: nanopesticides: Wiley, 63.
Adeyemi, O. S., Shittu, E. O., Akpor, O. B., Rotimi, D., and Batiha, G.E.-S. (2020). Silver nanoparticles restrict microbial growth by promoting oxidative stress and DNA damage. EXCLI J. 19, 492–500. doi:10.17179/EXCLI2020-1244
Ahmed, B., Solanki, B., Zaidi, A., Khan, M. S., and Musarrat, J. (2019). Bacterial toxicity of biomimetic green zinc oxide nanoantibiotic: insights into ZnONP uptake and nanocolloid–bacteria interface. Toxicol. Res. 8, 246–261. doi:10.1039/c8tx00267c
Aijaz, M., Ahmad, M., Ansari, M. A., and Ahmad, S. (2023). Antimicrobial resistance in a globalized world: current challenges and future perspectives. Int. J. Pharm. Drug Des. 1 (1), 7–23.
Ajith, M. P., Aswathi, M., Priyadarshini, E., and Rajamani, P. (2021). Recent innovations of nanotechnology in water treatment: a comprehensive review. Bioresour. Technol. 342, 126000. doi:10.1016/j.biortech.2021.126000
Alao, I. I., Oyekunle, I. P., Iwuozor, K. O., and Emenike, E. C. (2022). Green synthesis of copper nanoparticles and investigation of its anti-microbial properties. Adv. J. Chemistry-Section B 4 (1), 39–52.
Al-Jumaili, A., Alancherry, S., Bazaka, K., and Jacob, M. V. (2017). Review on the antimicrobial properties of carbon nanostructures. Materials 10 (9), 1066. doi:10.3390/ma10091066
Altammar, K. A. (2023). A review on nanoparticles: characteristics, synthesis, applications, and challenges. Front. Microbiol. 14, 1155622. doi:10.3389/fmicb.2023.1155622
Álvarez-Paino, M., Muñoz-Bonilla, A., and Fernández-García, M. (2017). Antimicrobial polymers in the nano-world. Nanomaterials 7 (2), 48. doi:10.3390/nano7020048
Ansari, A. A., Shamim, M., Manea, Y. K., Anwar, K., and Wani, A. A. (2023). Nanomaterials as a cutting edge in the removal of toxic contaminants from water. Mater. Chem. Phys. 295, 127092. doi:10.1016/j.matchemphys.2022.127092
Asaftei, M., Lucidi, M., Cirtoaje, C., Holban, A.-M., Charitidis, C. A., Yang, F., et al. (2023). Fighting bacterial pathogens with carbon nanotubes: focused review of recent progress. RSC Adv. 13 (29), 19682–19694. doi:10.1039/D3RA01745A
Baalousha, M. (2009). Aggregation and disaggregation of iron oxide nanoparticles: influence of particle concentration, pH and natural organic matter. Sci. Total Environ. 407 (6), 2093–2101. doi:10.1016/j.scitotenv.2008.11.022
Badawy, A. M. E., Luxton, T. P., Silva, R. G., Scheckel, K. G., Suidan, M. T., and Tolaymat, T. M. (2010). Impact of environmental conditions (pH, ionic strength, and electrolyte type) on the surface charge and aggregation of silver nanoparticles suspensions. Environ. Sci. Technol. 44 (4), 1260–1266. doi:10.1021/es902240k
Bahcelioglu, E., Unalan, H. E., and Erguder, T. H. (2021). Silver-based nanomaterials: a critical review on factors affecting water disinfection performance and silver release. Crit. Rev. Environ. Sci. Technol. 51 (20), 2389–2423. doi:10.1080/10643389.2020.1784666
Bellanthudawa, B., Nawalage, N., Handapangoda, H., Suvendran, S., Wijayasenarathne, K., Rathnasuriya, M., et al. (2023). A perspective on biodegradable and non-biodegradable nanoparticles in industrial sectors: applications, challenges, and future prospects. Nanotechnol. Environ. Eng. 8 (4), 975–1013. doi:10.1007/s41204-023-00344-7
Bélteky, P., Rónavári, A., Igaz, N., Szerencsés, B., Tóth, I. Y., Pfeiffer, I., et al. (2019). Silver nanoparticles: aggregation behavior in biorelevant conditions and its impact on biological activity. Int. J. nanomedicine 14, 667–687. doi:10.2147/ijn.s185965
Bhagat, M., Anand, R., Sharma, P., Rajput, P., Sharma, N., and Singh, K. (2021). Review—multifunctional copper nanoparticles: synthesis and applications. ECS J. Solid State Sci. Technol. 10 (6), 063011. doi:10.1149/2162-8777/ac07f8
Bhardwaj, A. K., Sundaram, S., Yadav, K. K., and Srivastav, A. L. (2021). An overview of silver nano-particles as promising materials for water disinfection. Environ. Technol. Innovation 23, 101721. doi:10.1016/j.eti.2021.101721
Brohi, R. D., Wang, L., Talpur, H. S., Wu, D., Khan, F. A., Bhattarai, D., et al. (2017). Toxicity of nanoparticles on the reproductive system in animal models: a review. Front. Pharmacol. 8, 606. doi:10.3389/fphar.2017.00606
Bundschuh, M., Filser, J., Lüderwald, S., McKee, M. S., Metreveli, G., Schaumann, G. E., et al. (2018). Nanoparticles in the environment: where do we come from, where do we go to? Environ. Sci. Eur. 30, 1–17. doi:10.1186/s12302-018-0132-6
Burdusel, A.-C., Gherasim, O., Grumezescu, A. M., Mogoantă, L., Ficai, A., and Andronescu, E. (2018). Biomedical applications of silver nanoparticles: an up-to-date overview. Nanomaterials 8, 681. doi:10.3390/nano8090681
Canaparo, R., Foglietta, F., Limongi, T., and Serpe, L. (2020). Biomedical applications of reactive oxygen species generation by metal nanoparticles. Materials 14 (1), 53. doi:10.3390/ma14010053
Chakachaka, V. M., Mahlangu, O. T., Tshangana, C. S., Mamba, B. B., and Muleja, A. A. (2023). Highly adhesive CoFe2O4 nanoengineered PES membranes for salts and Naproxen removal and antimicrobial activities. J. Membr. Sci. 676, 121612. doi:10.1016/j.memsci.2023.121612
Chakhtouna, H., Benzeid, H., Zari, N., Qaiss, A. E. K., and Bouhfid, R. (2021). Recent progress on Ag/TiO2 photocatalysts: photocatalytic and bactericidal behaviors. Environ. Sci. Pollut. Res. 28 (33), 44638–44666. doi:10.1007/s11356-021-14996-y
Chambers, B. A., Afrooz, A. N., Bae, S., Aich, N., Katz, L., Saleh, N. B., et al. (2014). Effects of chloride and ionic strength on physical morphology, dissolution, and bacterial toxicity of silver nanoparticles. Environ. Sci. Technol. 48, 761–769. doi:10.1021/es403969x
Chen, S., Chen, Y., Xu, H., Lyu, M., Zhang, X., Han, Z., et al. (2023). Single-walled carbon nanotubes synthesized by laser ablation from coal for field-effect transistors. Mater. Horizons 10 (11), 5185–5191. doi:10.1039/d3mh01053h
Crisan, C. M., Mocan, T., Manolea, M., Lasca, L. I., Tăbăran, F.-A., and Mocan, L. (2021). Review on silver nanoparticles as a novel class of antibacterial solutions. Appl. Sci. 11, 1120. doi:10.3390/app11031120
De Gusseme, B., Sintubin, L., Baert, L., Thibo, E., Hennebel, T., Vermeulen, G., et al. (2010). Biogenic silver for disinfection of water contaminated with viruses. Appl. Environ. Microbiol. 76 (4), 1082–1087. doi:10.1128/AEM.02433-09
Deshmukh, S. P., Patil, S. M., Mullani, S. B., and Delekar, S. D. (2019). Silver nanoparticles as an effective disinfectant: a review. Mater. Sci. Eng. C 97, 954–965. doi:10.1016/j.msec.2018.12.102
Dey, S., Podder, S., Roychowdhury, A., Das, D., and Ghosh, C.Kr. (2018). Facile synthesis of hierarchical nickel (III) oxide nanostructure: a synergistic remediating action towards water contaminants. J. Environ. Manage. 211, 356–366. doi:10.1016/j.jenvman.2018.01.009
Dimapilis, E. A. S., Hsu, C., Mendoza, R. M. O., and Lu, M. (2018). Zinc oxide nanoparticles for water disinfection. Sustain. Environ. Res. 28 (2), 47–56. doi:10.1016/j.serj.2017.10.001
Dobrescu, C. M., Dorobăț, L. M., and Angela, M. (2023). 10 health, safety and environmental management and risk mitigation of nanomaterials. Nanoformulations Sustain. Agric. Environ. Risk Mitig. 177.
Droepenu, E. K., Wee, B. S., Chin, S. F., Kok, K. Y., and Maligan, M. F.Resource Chemistry Program (2022). Zinc oxide nanoparticles synthesis methods and its effect on morphology: a review. Biointerface Res. Appl. Chem. 3, 4261–4292. doi:10.33263/BRIAC123.42614292
Dutta, G., Chinnaiyan, S. K., Sugumaran, A., and Narayanasamy, D. (2023). Sustainable bioactivity enhancement of ZnO–Ag nanoparticles in antimicrobial, antibiofilm, lung cancer, and photocatalytic applications. RSC Adv. 13, 26663–26682. doi:10.1039/D3RA03736C
Ebrahim, S. E., Sulaymon, A. H., and Alhares, H. S. (2015). Competitive removal of Cu2+, Cd2+, Zn2+, and Ni2+ ions onto iron oxide nanoparticles from wastewater. New pub Balaban 57, 20915–20929. doi:10.1080/19443994.2015.1112310
Edis, Z., Haj Bloukh, S., Ashames, A., and Ibrahim, M. (2019). Copper-based Nanoparticles, their chemistry and Antibacterial properties: a review. Chem. a clean healthy Planet, 401–428. doi:10.1007/978-3-030-20283-5_24
El-Newehy, M., Thamer, B. M., El-Hamshary, H., and AbdulHameed, M. M. (2023). Engineered multi-walled carbon nanotubes for disinfecting wastewater. Mater. Chem. Phys. 308, 128262. doi:10.1016/j.matchemphys.2023.128262
Eloffy, M. G., El-Sherif, D. M., Abouzid, M., Elkodous, M. A., El-nakhas, H. S., Sadek, R. F., et al. (2021). Proposed approaches for coronaviruses elimination from wastewater: membrane techniques and nanotechnology solutions. Nanotechnol. Rev. 11 (1), 1–25. doi:10.1515/ntrev-2022-0001
El-Sayyad, G. S., Abd Elkodous, M., El-Bastawisy, H. S., and El Rouby, W. M. A. (2023). Potential antibacterial, antibiofilm, and photocatalytic performance of gamma-irradiated novel nanocomposite for enhanced disinfection applications with an investigated reaction mechanism. BMC Microbiol. 23, 1–21. doi:10.1186/s12866-023-03016-3
Epelle, E. I., Okoye, P. U., Roddy, S., Gunes, B., and Okolie, J. A. (2022). Advances in the applications of nanomaterials for wastewater treatment. Environments 9 (11), 141. doi:10.3390/environments9110141
Eshed, M., Lellouche, J., Gedanken, A., and Banin, E. (2014). A Zn-doped CuO Nanocomposite shows enhanced Antibiofilm and antibacterial activities against streptococcus Mutans compared to Nanosized CuO. Adv. Funct. Mat. 24, 1382–1390. doi:10.1002/adfm.201302425
Fabrega, J., Fawcett, S. R., Renshaw, J. C., and Lead, J. R. (2009). Silver nanoparticle impact on bacterial growth: effect of pH, concentration, and organic matter. Environ. Sci. Technol. 43, 7285–7290. doi:10.1021/es803259g
Fiorenza, R., Di Mauro, A., Cantarella, M., Privitera, V., and Impellizzeri, G. (2019). Selective photodegradation of 2, 4-D pesticide from water by molecularly imprinted TiO2. Journal of Photochemistry and Photobiology A: Chemistry 380, 111872. doi:10.1016/j.jphotochem.2019.111872
Franci, G., Falanga, A., Galdiero, S., Palomba, L., Rai, M., Morelli, G., et al. (2015). Silver nanoparticles as potential antibacterial agents. Molecules 20, 8856–8874. doi:10.3390/molecules20058856
Franco, D., Calabrese, G., Guglielmino, S. P. P., and Conoci, S. (2022). Metal-based nanoparticles: antibacterial mechanisms and biomedical application. Microorganisms 10, 1778. doi:10.3390/microorganisms10091778
Gibała, A., Żeliszewska, P., Gosiewski, T., Krawczyk, A., Duraczyńska, D., Szaleniec, J., et al. (2021). Antibacterial and antifungal properties of silver nanoparticles—effect of a surface-stabilizing agent. Biomolecules 11, 1481. doi:10.3390/biom11101481
Goyat, R., Saharan, Y., Singh, J., Umar, A., and Akbar, S. (2022). Synthesis of graphene-based nanocomposites for environmental remediation applications: a review. Molecules/Molecules Online/Molecules Annu. 27 (19), 6433. doi:10.3390/molecules27196433
Guerrero-Almonacid, M., Pariguana, M., Gonzalez, G., Durán-Lara, E., and López-Cabaña, Z. (2023). Antibacterial activity of metal nanoparticles against bacteria of clinical origin: acta Microscopica. Acta Microsc. 32, 42–55.
Guo, M., Weng, X., Wang, T., and Chen, Z. (2017). Biosynthesized iron-based nanoparticles used as a heterogeneous catalyst for the removal of 2,4-dichlorophenol. Sep. Purif. Technol. 175, 222–228. doi:10.1016/J.SEPPUR.2016.11.042
Hadidi, N., and Mohebbi, M. (2022). Anti-infective and toxicity properties of carbon based materials: graphene and functionalized carbon nanotubes. Microorganisms 10 (12), 2439. doi:10.3390/microorganisms10122439
He, J., Hong, M., Xie, W., Chen, Z., Chen, D., and Xie, S. (2022). Progress and prospects of nanomaterials against resistant bacteria. J. Control. Release 351, 301–323. doi:10.1016/j.jconrel.2022.09.030
Hegde, K., Brar, S. K., Verma, M., and Surampalli, R. Y. (2016). Current understandings of toxicity, risks and regulations of engineered nanoparticles with respect to environmental microorganisms. Nanotechnol. Environ. Eng. 1, 1–12. doi:10.1007/s41204-016-0005-4
Hlongwane, G. N., Sekoai, P. T., Meyyappan, M., and Moothi, K. (2019). Simultaneous removal of pollutants from water using nanoparticles: a shift from single pollutant control to multiple pollutant control. Sci. Total Environ. 656, 808–833. doi:10.1016/j.scitotenv.2018.11.257
Hooshyar, Z., Rezanejade Bardajee, G., and Ghayeb, Y. (2013). Sonication enhanced removal of nickel and cobalt ions from polluted water using an iron based sorbent. J. Chem. 2013, 1–5. doi:10.1155/2013/786954
Huang, J.-J., Hu, H.-Y., Wu, Y.-H., Wei, B., and Lu, Y. (2013). Effect of chlorination and ultraviolet disinfection on tetA-mediated tetracycline resistance of Escherichia coli. Chemosphere 90 (8), 2247–2253. doi:10.1016/j.chemosphere.2012.10.008
Huang, J.-J., Xi, J., Hu, H.-Y., Li, Y., Lu, S.-Q., Tang, F., et al. (2016). UV light tolerance and reactivation potential of tetracycline-resistant bacteria from secondary effluents of a wastewater treatment plant. J. Environ. Sci. 41, 146–153. doi:10.1016/j.jes.2015.04.034
Ikram, M., Haider, A., Imran, M., Haider, J., Ul-Hamid, A., Shahzadi, A., et al. (2023). Graphitic-C3N4/chitosan-doped NiO nanostructure to treat the polluted water and their bactericidal with in silico molecular docking analysis. Int. J. Biol. Macromol. 227, 962–973. doi:10.1016/j.ijbiomac.2022.11.273
Jabbar, K. Q., Barzinjy, A. A., and Hamad, S. M. (2022). Iron oxide nanoparticles: preparation methods, functions, adsorption and coagulation/flocculation in wastewater treatment. Environ. Nanotechnol. Monit. Manag. 17, 100661. doi:10.1016/j.enmm.2022.100661
Jia, X., and Wei, F. (2017). Advances in production and applications of carbon nanotubes. Top. Curr. Chem. 375 (1), 18. doi:10.1007/s41061-017-0102-2
Juan, C. A., Pérez de la Lastra, J. M., Plou, F. J., and Pérez-Lebeña, E. (2021). The chemistry of reactive oxygen species (ROS) revisited: outlining their role in biological macromolecules (DNA, lipids and proteins) and induced pathologies. Int. J. Mol. 22, 4642. doi:10.3390/ijms22094642
Kallman, E. N., Oyanedel-Craver, V. A., and Smith, J. A. (2011). Ceramic filters impregnated with silver nanoparticles for point-of-use water treatment in rural Guatemala. J. Environ. Eng. 137, 407–415. doi:10.1061/(asce)ee.1943-7870.0000330
Kannan, U., Pullangott, G., Singh, S. P., and Maliyekkal, S. M. (2023). “Nanoscale silver enabled drinking water disinfection system,” in Nanoremediation (Elsevier), 127–166.
Keller, A. A., Adeleye, A. S., Conway, J. R., Garner, K. L., Zhao, L., Cherr, G. N., et al. (2017). Comparative environmental fate and toxicity of copper nanomaterials. NanoImpact 7, 28–40. doi:10.1016/j.impact.2017.05.003
Khalil, W. F., El-Sayyad, G. S., Rouby, W. M. E., Sadek, M., Farghali, A. A., and El-Batal, A. I. (2020). Graphene oxide-based nanocomposites (GO-chitosan and GO-EDTA) for outstanding antimicrobial potential against some Candida species and pathogenic bacteria. Int. J. Biol. Macromol. 164, 1370–1383. doi:10.1016/j.ijbiomac.2020.07.205
Khan, M. I., Paul, P., Behera, S. K., Jena, B., Tripathy, S. K., Stålsby Lundborg, C., et al. (2021a). To decipher the antibacterial mechanism and promotion of wound healing activity by hydrogels embedded with biogenic Ag@ZnO core-shell nanocomposites. Chem. Eng. J. 417, 128025. doi:10.1016/j.cej.2020.128025
Khan, S., Naushad, M., Al-Gheethi, A., and Iqbal, J. (2021b). Engineered nanoparticles for removal of pollutants from wastewater: current status and future prospects of nanotechnology for remediation strategies. J. Environ. Chem. Eng. 9 (5), 106160. doi:10.1016/j.jece.2021.106160
Khan, S. A., Jain, M., Pandey, A., Pant, K. K., Ziora, Z. M., Blaskovich, M. A., et al. (2022a). Leveraging the potential of silver nanoparticles-based materials towards sustainable water treatment. J. Environ. Manag. 319, 115675. doi:10.1016/j.jenvman.2022.115675
Khan, Y., Sadia, H., Ali Shah, S. Z., Khan, M. N., Shah, A. A., Ullah, N., et al. (2022b). Classification, synthetic, and characterization approaches to nanoparticles, and their applications in various fields of nanotechnology: a review. Catalysts 12 (11), 1386. doi:10.3390/catal12111386
Kowalczyk, P., Szymczak, M., Maciejewska, M., Laskowski, Ł., Laskowska, M., Ostaszewski, R., et al. (2021). All that glitters is not silver—a new look at microbiological and medical applications of silver nanoparticles. Int. J. Mol. Sci. 22 (2), 854. doi:10.3390/ijms22020854
Kumar, S. (2023). Smart and innovative nanotechnology applications for water purification. Hybrid. Adv. 3, 100044. doi:10.1016/j.hybadv.2023.100044
Kunduru, K. R., Nazarkovsky, M., Farah, S., Pawar, R. P., Basu, A., and Domb, A. J. (2017). “2 - nanotechnology for water purification: applications of nanotechnology methods in wastewater treatment,” in Water purification. Editor A. M. Grumezescu (Academic Press), 33–74. doi:10.1016/B978-0-12-804300-4.00002-2
Lasagna-Reeves, C., Gonzalez-Romero, D., Barria, M., Olmedo, I., Clos, A., Ramanujam, V. S., et al. (2010). Bioaccumulation and toxicity of gold nanoparticles after repeated administration in mice. Biochem. biophysical Res. Commun. 393 (4), 649–655. doi:10.1016/j.bbrc.2010.02.046
Lead, J. R., Batley, G. E., Alvarez, P. J. J., Croteau, M.-N., Handy, R. D., McLaughlin, M. J., et al. (2018). Nanomaterials in the environment: behavior, fate, bioavailability, and effects—an updated review. Environ. Toxicol. Chem. 37, 2029–2063. doi:10.1002/etc.4147
Levard, C., Hotze, E. M., Lowry, G. V., and Brown Jr, G. E. (2012). Environmental transformations of silver nanoparticles: impact on stability and toxicity. Environ. Sci. Technol. 46 (13), 6900–6914. doi:10.1021/es2037405
Li, Q., Mahendra, S., Lyon, D. Y., Brunet, L., Liga, M. V., Li, D., et al. (2008). Antimicrobial nanomaterials for water disinfection and microbial control: potential applications and implications. Water Res. 42 (18), 4591–4602. doi:10.1016/j.watres.2008.08.015
Li, X., Chen, D., and Xie, S. (2021). Current progress and prospects of organic nanoparticles against bacterial biofilm. Adv. Colloid Interface Sci. 294, 102475. doi:10.1016/j.cis.2021.102475
Liao, C., Li, Y., and Tjong, S. C. (2020). Visible-light active titanium dioxide nanomaterials with bactericidal properties. Nanomaterials 10 (1), 124. doi:10.3390/nano10010124
Lin, Z., Monteiro-Riviere, N. A., and Riviere, J. E. (2015). Pharmacokinetics of metallic nanoparticles. Wiley Interdiscip. Rev. Nanomedicine Nanobiotechnology 7 (2), 189–217. doi:10.1002/wnan.1304
Liu, C., Guo, J., Yan, X., Tang, Y., Mazumder, A., Wu, S., et al. (2017). Antimicrobial nanomaterials against biofilms: an alternative strategy. Environ. Rev. 25 (2), 225–244. doi:10.1139/er-2016-0046
Liu, Y., Li, H., and Xiao, K. (2016). Distribution and biological effects of nanoparticles in the reproductive system. Curr. drug Metab. 17 (5), 478–496. doi:10.2174/1389200217666160105111436
Magaña-López, R., Zaragoza-Sánchez, P. I., Jiménez–Cisneros, B., and Chávez-Mejía, A. C. (2021). The use of TIO2 as a disinfectant in water sanitation applications. Water 13 (12), 1641. doi:10.3390/w13121641
Mahendra, S., Li, Q., Lyon, D. Y., Brunet, L., and Alvarez, P. J. J. (2009). “Chapter 12 - nanotechnology-enabled water disinfection and microbial control: merits and limitations,” in Nanotechnology Applications for Clean Water. Editors N. Savage, M. Diallo, J. Duncan, A. Street, and R. Sustich (William Andrew Publishing), 157–166. doi:10.1016/B978-0-8155-1578-4.50021-4
Malhotra, N., Ger, T.-R., Uapipatanakul, B., Huang, J.-C., Chen, K. H.-C., and Hsiao, C.-D. (2020). Review of copper and copper nanoparticle toxicity in fish. Nanomaterials 10 (6), 1126. doi:10.3390/nano10061126
Mammari, N., Lamouroux, E., Boudier, A., and Duval, R. E. (2022). Current knowledge on the oxidative-stress-mediated antimicrobial properties of metal-based nanoparticles. Microorganisms 10 (2), 437. doi:10.3390/microorganisms10020437
Markowicz, A. (2023). The significance of metallic nanoparticles in the emerging, development and spread of antibiotic resistance. Sci. Total Environ. 871, 162029. doi:10.1016/j.scitotenv.2023.162029
Mhadhbi, M., Abderazzak, H., and Avar, B. (2023) “Synthesis and properties of titanium dioxide nanoparticles,” in IntechOpen eBooks. doi:10.5772/intechopen.111577
Mi, G., Shi, D., Wang, M., and Webster, T. J. (2018). Reducing bacterial infections and biofilm formation using nanoparticles and nanostructured antibacterial surfaces. Adv. Healthc. Mater. 7 (13), 1800103. doi:10.1002/adhm.201800103
Michael-Kordatou, I., Karaolia, P., and Fatta-Kassinos, D. (2018). The role of operating parameters and oxidative damage mechanisms of advanced chemical oxidation processes in the combat against antibiotic-resistant bacteria and resistance genes present in urban wastewater. Water Res. 129, 208–230. doi:10.1016/j.watres.2017.10.007
Mohammad Gheimasi, M. H., Lorestani, B., Kiani Sadr, M., Cheraghi, M., and Emadzadeh, D. (2021). Synthesis of novel hybrid NF/FO nanocomposite membrane by incorporating black TiO 2 nanoparticles for highly efficient heavy metals removal. Int. J. Environ. Res. 15, 475–485. doi:10.1007/s41742-021-00317-1
Mohammed, A. N. (2021). “Implementation of novel nanosilver composites in drinking water treatment,” in Polymer nanocomposites based on silver nanoparticles: synthesis, characterization and applications. Editors H. M. Lal, S. Thomas, T. Li, and H. J. Maria (Cham: Springer International Publishing), 267–286. doi:10.1007/978-3-030-44259-0_11
Mohammed, H., Kumar, A., Bekyarova, E., Al-Hadeethi, Y., Zhang, X., Chen, M., et al. (2020). Antimicrobial mechanisms and effectiveness of graphene and graphene-functionalized biomaterials. A scope review. Front. Bioeng. Biotechnol. 8, 465. doi:10.3389/fbioe.2020.00465
Mohanta, Y. K., Chakrabartty, I., Mishra, A. K., Chopra, H., Mahanta, S., Avula, S. K., et al. (2023). Nanotechnology in combating biofilm: a smart and promising therapeutic strategy. Front. Microbiol. 13, 1028086. doi:10.3389/fmicb.2022.1028086
Mtavangu, S. G., Machunda, R. L., van der Bruggen, B., and Njau, K. N. (2022). In situ facile green synthesis of Ag–ZnO nanocomposites using Tetradenia riperia leaf extract and its antimicrobial efficacy on water disinfection. Sci. Rep. 12, 15359. doi:10.1038/s41598-022-19403-1
Nehra, P., and Chauhan, R. P. (2019). “Antimicrobial activity of magnetic nanostructures,” in Magnetic Nanostructures: Environmental and Agricultural Applications. Editors K. A. Abd-Elsalam, M. A. Mohamed, and R. Prasad (Cham: Springer International Publishing), 301–318. doi:10.1007/978-3-030-16439-3_16
Nisar, P., Ali, N., Rahman, L., Ali, M., and Shinwari, Z. K. (2019). Antimicrobial activities of biologically synthesized metal nanoparticles: an insight into the mechanism of action. JBIC J. Biol. Inorg. Chem. 24, 929–941. doi:10.1007/s00775-019-01717-7
Noohpisheh, Z., Amiri, H., Farhadi, S., and Mohammadi-gholami, A. (2020). Green synthesis of Ag-ZnO nanocomposites using Trigonella foenum-graecum leaf extract and their antibacterial, antifungal, antioxidant and photocatalytic properties. Spectrochim. Acta. A. Mol. Biomol. Spectrosc. 240, 118595. doi:10.1016/j.saa.2020.118595
Oluwasanu, A. A., Oluwaseun, F., Teslim, J. A., Isaiah, T. T., Olalekan, I. A., and Chris, O. A. (2019). Scientific applications and prospects of nanomaterials: a multidisciplinary review. Afr. J. Biotechnol. 18, 946–961. doi:10.5897/AJB2019.16812
Omran, B. A., and Baek, K. (2022). Graphene-derived antibacterial nanocomposites for water disinfection: current and future perspectives. Environ. Pollut. 298, 118836. doi:10.1016/j.envpol.2022.118836
Oquendo-Cruz, A., and Perales-Pérez, O. (2018). Synthesis, characterization and bactericide properties of pure and li doped ZnO nanoparticles for alternative water disinfection methods. J. Electron. Mat. 47, 6260–6265. doi:10.1007/s11664-018-6541-x
Park, S., Ko, Y., Jung, H., Lee, C., Woo, K., and Ko, G. (2018). Disinfection of waterborne viruses using silver nanoparticle-decorated silica hybrid composites in water environments. Sci. Total Environ. 625, 477–485. doi:10.1016/j.scitotenv.2017.12.318
Parmar, S., Kaur, H., Singh, J., Matharu, A. S., Ramakrishna, S., and Bechelany, M. (2022). Recent advances in green synthesis of Ag NPs for extenuating antimicrobial resistance. Nanomaterials 12, 1115. doi:10.3390/nano12071115
Patil, S. S., and Lekhak, U. M. (2020). “Toxic effects of engineered carbon nanoparticles on environment,” in Carbon Nanomaterials for Agri-Food and Environmental Applications (Elsevier), 237–260.
Poguberović, S. S., Krčmar, D. M., Maletić, S. P., Kónya, Z., Pilipović, D. D. T., Kerkez, D. V., et al. (2016). Removal of As(III) and Cr(VI) from aqueous solutions using “green” zero-valent iron nanoparticles produced by oak, mulberry and cherry leaf extracts. Ecol. Eng. 90, 42–49. doi:10.1016/j.ecoleng.2016.01.083
Polinarski, M. A., Beal, A. L., Silva, F. E., Bernardi-Wenzel, J., Burin, G. R., de Muniz, G. I., et al. (2021). New perspectives of using chitosan, silver, and chitosan–silver nanoparticles against multidrug-resistant bacteria. Part. Part. Syst. Charact. 38 (4), 2100009. doi:10.1002/ppsc.202100009
Pourali, P., Baserisalehi, M., Afsharnezhad, S., Behravan, J., Ganjali, R., Bahador, N., et al. (2013). The effect of temperature on antibacterial activity of biosynthesized silver nanoparticles. Biometals 26, 189–196. doi:10.1007/s10534-012-9606-y
Powell, J. J., Faria, N., Thomas-McKay, E., and Pele, L. C. (2010). Origin and fate of dietary nanoparticles and microparticles in the gastrointestinal tract. J. Autoimmun. 34 (3), J226–J233. doi:10.1016/j.jaut.2009.11.006
Qi, Z., Xue, X., Zhou, H., Yuan, H., Li, W., Yang, G., et al. (2022). The aqueous assembly preparation of OPs-AgNPs with phenols from olive mill wastewater and its mechanism on antimicrobial function study. Food Chem. 376, 131924. doi:10.1016/j.foodchem.2021.131924
Qu, F., Xu, H., Wei, H., Lai, W., Xiong, Y., Xu, F., et al. (2010). “Effects of pH and temperature on antibacterial activity of silver nanoparticles,” in 2010 3rd international conference on biomedical engineering and informatics. Presented at the 2010 3rd international conference on biomedical engineering and informatics, 2033–2037.
Rajabi, H. R., Naghiha, R., Kheirizadeh, M., Sadatfaraji, H., Mirzaei, A., and Alvand, Z. M. (2017). Microwave assisted extraction as an efficient approach for biosynthesis of zinc oxide nanoparticles: synthesis, characterization, and biological properties. Mat. Sci. Eng. C 78, 1109–1118. doi:10.1016/j.msec.2017.03.090
Rajarathinam, M., Duraibabu, D., Sudha, J., and Kalaichelvan, P. T. (2014). Biogenic nanosilver incorporated reverse osmosis membrane for antibacterial and antifungal activities against selected pathogenic strains: an enhanced eco-friendly water disinfection approach. J. Environ. Sci. Health. A. Tox. Hazard. Subst. Environ. Eng. 49 (10), 1125–1133. doi:10.1080/10934529.2014.897149
Rathore, C., Yadav, V. K., Gacem, A., AbdelRahim, S. K., Verma, R. K., Chundawat, R. S., et al. (2023). Microbial synthesis of titanium dioxide nanoparticles and their importance in wastewater treatment and antimicrobial activities: a review. Front. Microbiol. 14, 1270245. doi:10.3389/fmicb.2023.1270245
Ribut, S. H., Abdullah, C. A. C., Mustafa, M., Yusoff, M. Z. M., and Azman, S. N. A. (2018). Influence of pH variations on zinc oxide nanoparticles and their antibacterial activity. Mater. Res. Express 6, 025016. doi:10.1088/2053-1591/aaecbc
Rikta, S. Y. (2019). “9 - application of nanoparticles for disinfection and microbial control of water and wastewater,” in Nanotechnology in Water and Wastewater Treatment, Micro and Nano Technologies. Editors A. Ahsan, and A. F. Ismail (Elsevier), 159–176. doi:10.1016/B978-0-12-813902-8.00009-5
Ritu, , Verma, K. K., Das, A., and Chandra, P. (2023). Phytochemical-based synthesis of silver nanoparticle: mechanism and potential applications. BioNanoScience 13, 1359–1380. doi:10.1007/s12668-023-01125-x
Rosli, N. A., Teow, Y. H., and Mahmoudi, E. (2021). Current approaches for the exploration of antimicrobial activities of nanoparticles. Sci. Technol. Adv. Mat. 22, 885–907. doi:10.1080/14686996.2021.1978801
Rowles, L. S., Tso, D., Dolocan, A., Kirisits, M. J., Lawler, D. F., and Saleh, N. B. (2023). Integrating Navajo pottery techniques to improve silver nanoparticle-enabled ceramic water filters for disinfection. Environ. Sci. Technol. 57 (44), 17132–17143. doi:10.1021/acs.est.3c03462
Rus, A., Leordean, V. D., and Berce, P., 2017. Silver Nanoparticles (AgNP) impregnated filters in drinking water disinfection. In MATEC web of conferences 137. EDP Sciences, 07007. doi:10.1051/matecconf/201713707007
Sachin, J., Singh, N., Singh, R., Shah, K., and Pramanik, B. K. (2023). Green synthesis of zinc oxide nanoparticles using lychee peel and its application in anti-bacterial properties and CR dye removal from wastewater. Chemosphere 327, 138497. doi:10.1016/j.chemosphere.2023.138497
Saha, R., Subramani, K., Petchi Muthu Raju, S. A. K., Rangaraj, S., and Venkatachalam, R. (2018). Psidium guajava leaf extract-mediated synthesis of ZnO nanoparticles under different processing parameters for hydrophobic and antibacterial finishing over cotton fabrics. Prog. Org. Coat. 124, 80–91. doi:10.1016/j.porgcoat.2018.08.004
Salem, S. S., Hammad, E. N., Mohamed, A. A., and El-Dougdoug, W. (2022). A comprehensive review of nanomaterials: types, synthesis, characterization, and applications. Biointerface Res. Appl. Chem. 13 (1), 41. doi:10.33263/BRIAC131.041
Salem, W., El-Deen, F. N., Ebnalwaleed, K., Badry, M., and Latef, A. H. A. (2021). UV-Induced antibacterial activity of Green-Synthesized TIO2 nanoparticles for the potential reuse of raw surface and underground water. J. Plant Growth Regul. 41 (3), 1344–1358. doi:10.1007/s00344-021-10391-6
Salleh, A., Naomi, R., Utami, N. D., Mohammad, A. W., Mahmoudi, E., Mustafa, N., et al. (2020). The potential of silver nanoparticles for antiviral and antibacterial applications: a mechanism of action. Nanomaterials 10 (8), 1566. doi:10.3390/nano10081566
Sargazi, S., Simge, E., Gelen, S. S., Rahdar, A., Bilal, M., Arshad, R., et al. (2022). Application of titanium dioxide nanoparticles in photothermal and photodynamic therapy of cancer: an updated and comprehensive review. J. Drug. Deliv. Sci. Technol. 75, 103605. doi:10.1016/j.jddst.2022.103605
Sayed, F. A.-Z., Eissa, N. G., Shen, Y., Hunstad, D. A., Wooley, K. L., and Elsabahy, M. (2022). Morphologic design of nanostructures for enhanced antimicrobial activity. J. Nanobiotechnology 20 (1), 536. doi:10.1186/s12951-022-01733-x
Schaumann, G. E., Philippe, A., Bundschuh, M., Metreveli, G., Klitzke, S., Rakcheev, D., et al. (2015). Understanding the fate and biological effects of Ag-and TiO2-nanoparticles in the environment: the quest for advanced analytics and interdisciplinary concepts. Sci. Total Environ. 535, 3–19. doi:10.1016/j.scitotenv.2014.10.035
Sharma, P., Goyal, D., and Chudasama, B. (2022). Antibacterial activity of colloidal copper nanoparticles against Gram-negative (Escherichia coli and Proteus vulgaris) bacteria. Lett. Appl. Microbiol. 74 (5), 695–706. doi:10.1111/lam.13655
Shi, T., Wei, Q., Wang, Z., Zhang, G., Sun, X., and He, Q.-Y. (2019). Photocatalytic protein damage by silver nanoparticles circumvents bacterial stress response and multidrug resistance. mSphere 4, e00175. doi:10.1128/msphere.00175-19
Shukla, S. K., Khan, A., and Rao, T. S. (2021). “Microbial fouling in water treatment plants,” in Microbial and Natural Macromolecules (Academic Press), 589–622.
Singh, A., Dasauni, K., Nailwal, T., and Nenavathu, B. P. (2022). TeO2 deposited ZnO nanotubes combined with cefotaxime as a nanoantibiotic against Klebsiella pneumoniae. Mat. Today. Proc. 67, 451–455. doi:10.1016/j.matpr.2022.05.350
Singh, P., Pandit, S., Mokkapati, V. R. S. S., Garnæs, J., and Mijakovic, I. (2020). A sustainable approach for the green synthesis of silver nanoparticles from solibacillus isronensis sp. and their application in biofilm inhibition. Molecules/Molecules Online/Molecules Annu. 25 (12), 2783. doi:10.3390/molecules25122783
Sinha, T., Adhikari, P. P., and Bhandari, V. M. (2022). Sustainable fabrication of copper nanoparticles: a potent and affordable candidate for water treatment, water disinfection, antioxidant activity and theranostic agent. ChemistrySelect 7, e202103552. doi:10.1002/slct.202103552
Sinouvassane, D., Wong, L. S., Lim, Y. M., and Lee, P. (2016). A review on bio-distribution and toxicity of silver, titanium dioxide and zinc oxide nanoparticles in aquatic environment. Pollut. Res. 35, 701–712.
Smith, S. C., and Rodrigues, D. F. (2015). Carbon-based nanomaterials for removal of chemical and biological contaminants from water: a review of mechanisms and applications. Carbon 91, 122–143. doi:10.1016/j.carbon.2015.04.043
Song, N., Gao, X., Ma, Z., Wang, X., Wei, Y., and Gao, C. (2018). A review of graphene-based separation membrane: materials, characteristics, preparation and applications. Desalination 437, 59–72. doi:10.1016/j.desal.2018.02.024
Srivastav, A. L., Patel, N., and Chaudhary, V. K. (2020). Disinfection by-products in drinking water: occurrence, toxicity and abatement. Environ. Pollut. 267, 115474. doi:10.1016/j.envpol.2020.115474
Sultana, K. A., Islam, M. T., Silva, J. A., Turley, R. S., Hernandez-Viezcas, J. A., Gardea-Torresdey, J. L., et al. (2020). Sustainable synthesis of zinc oxide nanoparticles for photocatalytic degradation of organic pollutant and generation of hydroxyl radical. J. Mol. Liq. 307, 112931. doi:10.1016/j.molliq.2020.112931
Sun, L., Du, T., Hu, C., Chen, J., Lu, J., Lu, Z., et al. (2017). Antibacterial activity of graphene oxide/g-C3N4 composite through photocatalytic disinfection under visible light. ACS Sustain. Chem. Eng. 5 (10), 8693–8701. doi:10.1021/acssuschemeng.7b01431
Thakur, A., Kumar, A., and Singh, A. (2024). Adsorptive removal of heavy metals, dyes, and pharmaceuticals: carbon-based nanomaterials in focus. Carbon 217, 118621. doi:10.1016/j.carbon.2023.118621
Turan, N. B., Erkan, H. S., Engin, G. O., and Bilgili, M. S. (2019). Nanoparticles in the aquatic environment: usage, properties, transformation and toxicity—a review. Process Saf. Environ. Prot. 130, 238–249. doi:10.1016/j.psep.2019.08.014
Vasiliev, G., Kubo, A.-L., Vija, H., Kahru, A., Bondar, D., Karpichev, Y., et al. (2023). Synergistic antibacterial effect of copper and silver nanoparticles and their mechanism of action. Sci. Rep. 13 (1), 9202. doi:10.1038/s41598-023-36460-2
Wang, L.-F., Habibul, N., He, D.-Q., Li, W.-W., Zhang, X., Jiang, H., et al. (2015). Copper release from copper nanoparticles in the presence of natural organic matter. Water Res. 68, 12–23. doi:10.1016/j.watres.2014.09.031
Wang, X., Sun, T., Zhu, H., Han, T., Wang, J., and Dai, H. (2020). Roles of pH, cation valence, and ionic strength in the stability and aggregation behavior of zinc oxide nanoparticles. J. Environ. Manag. 267, 110656. doi:10.1016/j.jenvman.2020.110656
Wu, Y., Yang, Y., Zhang, Z., Wang, Z., Zhao, Y., and Sun, L. (2018). A facile method to prepare size-tunable silver nanoparticles and its antibacterial mechanism. Adv. Powder Technol. 29, 407–415. doi:10.1016/j.apt.2017.11.028
Xiao, G., Zhang, X., Zhang, W., Zhang, S., Su, H., and Tan, T. (2015). Visible-light-mediated synergistic photocatalytic antimicrobial effects and mechanism of Ag-nanoparticles@chitosan–TiO2 organic–inorganic composites for water disinfection. Appl. Catal. B Environ. 170-171, 255–262. doi:10.1016/j.apcatb.2015.01.042
Xiao, J., Huang, J., Wang, M., Huang, M., and Wang, Y. (2021). The fate and long-term toxic effects of NiO nanoparticles at environmental concentration in constructed wetland: enzyme activity, microbial property, metabolic pathway and functional genes. J. Hazard. Mat. 413, 125295. doi:10.1016/j.jhazmat.2021.125295
Yadav, L., Tripathi, R. M., Prasad, R., Pudake, R. N., and Mittal, J. (2017a). Antibacterial activity of Cu nanoparticles against E. coli, Staphylococcus aureus and Pseudomonas aeruginosa. Nano. Biomed. Eng. 9 (1), 9–14. doi:10.5101/nbe.v9i1.p9-14
Yadav, N., Dubey, A., Shukla, S., Saini, C. P., Gupta, G., Priyadarshini, R., et al. (2017b). Graphene oxide-coated surface: inhibition of bacterial biofilm formation due to specific surface–interface interactions. Acs Omega 2 (7), 3070–3082. doi:10.1021/acsomega.7b00371
Yah, C. S., and Simate, G. S. (2015). Nanoparticles as potential new generation broad spectrum antimicrobial agents. DARU J. Pharm. Sci. 23 (1), 43. doi:10.1186/s40199-015-0125-6
Yang, H., Ren, Y., Wang, T., and Wang, C. (2016). Preparation and antibacterial activities of Ag/Ag+/Ag3+ nanoparticle composites made by pomegranate (Punica granatum) rind extract. Results Phys. 6, 299–304. doi:10.1016/j.rinp.2016.05.012
Yang, P., Pageni, P., Rahman, M. A., Bam, M., Zhu, T., Chen, Y. P., et al. (2019). Gold nanoparticles with antibiotic-metallopolymers toward broad-spectrum antibacterial effects. Adv. Healthc. Mat. 8, 1800854. doi:10.1002/adhm.201800854
Yang, X. (2016). A study on antimicrobial effects of nanosilver for drinking water disinfection. Springer.
Yashni, G., Al-Gheethi, A., Mohamed, R., Hossain, M. S., Kamil, A. F., and Abirama Shanmugan, V. (2021). Photocatalysis of xenobiotic organic compounds in greywater using zinc oxide nanoparticles: a critical review. Water Environ. J. 35 (1), 190–217. doi:10.1111/wej.12619
Younis, A. B., Haddad, Y., Kosaristanova, L., and Smerkova, K. (2023). Titanium dioxide nanoparticles: recent progress in antimicrobial applications. WIREs Nanomedicine Nanobiotechnology 15, e1860. doi:10.1002/wnan.1860
Yu, S., Liu, J., Yin, Y., and Shen, M. (2018). Interactions between engineered nanoparticles and dissolved organic matter: a review on mechanisms and environmental effects. J. Environ. Sci. 63, 198–217. doi:10.1016/j.jes.2017.06.021
Yu, Y., Zhou, Z., Huang, G., Cheng, H., Han, L., Zhao, S., et al. (2022). Purifying water with silver nanoparticles (AgNPs)-incorporated membranes: recent advancements and critical challenges. Water Res. 222, 118901. doi:10.1016/j.watres.2022.118901
Zeumer, R., Galhano, V., Monteiro, M. S., Kuehr, S., Knopf, B., Meisterjahn, B., et al. (2020). Chronic effects of wastewater-borne silver and titanium dioxide nanoparticles on the rainbow trout (Oncorhynchus mykiss). Sci. Total Environ. 723, 137974. doi:10.1016/j.scitotenv.2020.137974
Zhan, Y., Chen, X., Sun, A., Jia, H., Liu, Y., Li, L., et al. (2023). Design and assembly of Ag-decorated Bi2O3 @ 3D MXene Schottky heterojunction for the highly permeable and multiple-antifouling of fibrous membrane in the purification of complex emulsified oil pollutants. J. Hazard. Mat. 458, 131965. doi:10.1016/j.jhazmat.2023.131965
Zhang, R., Liu, Y., He, M., Su, Y., Zhao, X., Elimelech, M., et al. (2016). Antifouling membranes for sustainable water purification: strategies and mechanisms. Chem. Soc. Rev. 45 (21), 5888–5924. doi:10.1039/c5cs00579e
Zhao, C.-M., and Wang, W.-X. (2010). Biokinetic uptake and efflux of silver nanoparticles in Daphnia magna. Environ. Sci. Technol. 44 (19), 7699–7704. doi:10.1021/es101484s
Zhao, J., Yan, P., Qureshi, A., and Chiang, Y. W. (2023). How do material characteristics and antimicrobial mechanisms affect microbial control and water disinfection performance of metal nanoparticles? Blue-Green Syst. 5, 75–101. doi:10.2166/bgs.2023.007
Zikalala, N. E., Azizi, S., Zikalala, S. A., Kamika, I., Maaza, M., Zinatizadeh, A. A., et al. (2022). An evaluation of the biocatalyst for the synthesis and application of zinc oxide nanoparticles for water remediation—a review. Catalysts 12, 1442. doi:10.3390/catal12111442
Zodrow, K., Brunet, L., Mahendra, S., Li, D., Zhang, A., Li, Q., et al. (2009). Polysulfone ultrafiltration membranes impregnated with silver nanoparticles show improved biofouling resistance and virus removal. Water research 43 (3), 715–723. doi:10.1016/j.watres.2008.11.014
Zou, X., Shi, J., and Zhang, H. (2014). Coexistence of silver and titanium dioxide nanoparticles: enhancing or reducing environmental risks? Aquat. Toxicol. 154, 168–175. doi:10.1016/j.aquatox.2014.05.020
Keywords: nanoparticles, microbial control, water treatment, antimicrobial mechanisms, ecological impacts
Citation: Olawade DB, Wada OZ, Fapohunda O, Egbewole BI, Ajisafe O and Ige AO (2024) Nanoparticles for microbial control in water: mechanisms, applications, and ecological implications. Front. Nanotechnol. 6:1427843. doi: 10.3389/fnano.2024.1427843
Received: 04 May 2024; Accepted: 08 July 2024;
Published: 25 July 2024.
Edited by:
Mithun Nath, Wuhan University of Science and Technology, ChinaReviewed by:
Priya Banerjee, Rabindra Bharati University, IndiaSriyutha Murthy, Bhabha Atomic Research Centre, India
Copyright © 2024 Olawade, Wada, Fapohunda, Egbewole, Ajisafe and Ige. This is an open-access article distributed under the terms of the Creative Commons Attribution License (CC BY). The use, distribution or reproduction in other forums is permitted, provided the original author(s) and the copyright owner(s) are credited and that the original publication in this journal is cited, in accordance with accepted academic practice. No use, distribution or reproduction is permitted which does not comply with these terms.
*Correspondence: David B. Olawade, ZC5vbGF3YWRlQHVlbC5hYy51aw==