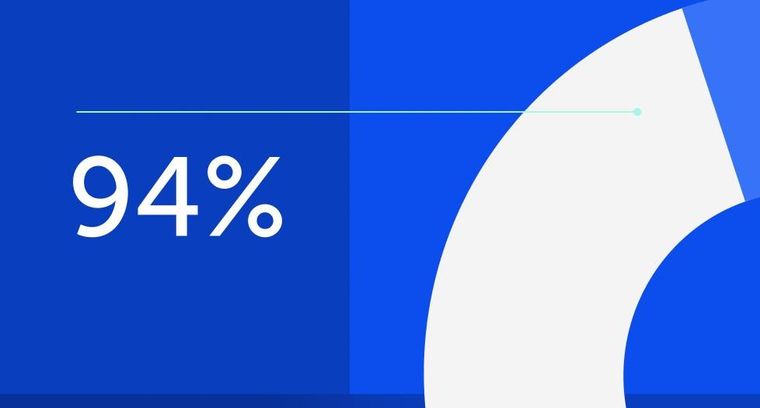
94% of researchers rate our articles as excellent or good
Learn more about the work of our research integrity team to safeguard the quality of each article we publish.
Find out more
ORIGINAL RESEARCH article
Front. Nanotechnol., 20 June 2024
Sec. Biomedical Nanotechnology
Volume 6 - 2024 | https://doi.org/10.3389/fnano.2024.1386312
This article is part of the Research TopicSpotlight on Nanotechnology: Latin AmericaView all 6 articles
Silver nanoparticles (AgNP) are widely used globally due to their numerous properties, mainly because of their antimicrobial activity. However, the need for further regulations and legislation regarding their presence in products has led to concerns about their biosecurity, necessitating relevant biological studies at different levels. The present work aimed to evaluate the effect of the polyvinylpyrrolidone (PVP)-coated AgNP on the small intestine contraction and other mediators involved and the mediators involved (nitric oxide and serotonin) in an ex vivo model. Intestinal segments (duodenum, jejunum, and ileum) were treated in the presence and absence of five cumulative, increasing concentrations of AgNP (0.01, 0.1, 1, 10, and 100 μg/mL) and three individual concentrations of PVP-coated AgNP (0.01, 1, and 100 μg/mL), showing a transient decrease in the contractile effect in the small intestine segments treated with cumulative, increasing concentrations. This effect did not allow the isolated small intestine rings to return to their contractile basal level, and it was associated with an increase in nitric oxide (NO) production and variations in the amount of serotonin. A similar effect was observed at individual AgNP administrations but with a lesser influence on NO production. These results show that AgNP, in the function of the administration (cumulative or individual) and the segment type, can modify the contraction of the small intestine, mediated in part by NO and exerting a differential pattern in the amount of serotonin. Histological analysis revealed that specific morphological alterations induced by AgNP, such as necrosis and villi detachment, were more evident in the ileum segment. Furthermore, the accumulation of silver was observed in the small intestine villi, which could determine the physiological effects exerted upon the intestinal contraction associated with the release of a specific mediator like NO production and the presence of serotonin. These findings suggest that the small intestine is an essential target of the actions induced by AgNP, which could be ingested and reach this conduit because of the consumption of products containing these materials, modifying the intestinal motility that influences the efficiency of nutrient absorption—aspects that need to be explored and investigated in the short, medium, and long terms.
The present work investigated the role of polyvinylpyrrolidone (PVP)-coated silver nanoparticles (AgNP) on the contractile state of the three small intestine rat segment types and the influence of two endogenous agents: NO and serotonin. AgNP are the most prevalent nanomaterial used in a wide range of applications, mainly because of their antimicrobial properties (Gaillet and Rouanet, 2015; Gonzalez et al., 2016; Chávez-Hernández et al., 2024). Nowadays, the consumption of food that could contain AgNP due to migration from packages or through trophic transfer may well-exert some physiological alteration in the small intestine, an anatomic structure where the nutrients from the diet are absorbed selectively in the function of the segment. Absorption depends on the movement of the food through the small intestine, and this is mediated by peristaltic movements, which consist of the alternated contraction and relaxation of the circular and longitudinal smooth muscle regulated by the secretion of chemical agents controlled by the enteric nervous system (Hall, 2016). Two of the most related mediators of intestinal motility are serotonin (5-HT or 5-hydroxytryptamine) and nitric oxide (NO) (Garcia and Stein, 2006; Hasler, 2009). Serotonin acts as a signaling molecule that starts the peristaltic movement, absorption, and secretory reflex activated from the enterochromaffin cells (ECs) located in the intestinal mucosa layer cells, where it is synthesized from amino acid tryptophan (Trp) and released from ECs due to calcium-dependent external processes, promoted by muscarinic, nicotinic, and 5-HT3 receptors, all of which facilitate muscle contraction (Bertrand, 2006). It is known that 95% of serotonin is produced, stored, and secreted by the ECs in the mucosa layer of the intestine (Hasler, 2009). On the other hand, NO is a free radical generated from the amino acid arginine through the enzymatic action of nitric oxide synthase (NOS) family and is essential in regulating the intestinal blood flow, relaxing smooth muscle cells, facilitating gastric emptying, and promoting vasodilatation (Calignano et al., 1992; Shah et al., 2004). There is an important relation between serotonin and NO in regulating intestinal function, which consists of the serotonin (5-HT) non-adrenergic non-cholinergic (NANC) NO-mediated relaxation in the enteric nervous system (Bogers et al., 1991). Most of the products consumed by humans are absorbed in the small intestine. Nevertheless, some of those products could be elaborated with a base of nanomaterials, which could cause adverse effects on the integrity of the small intestine, therefore changing its correct function. An example of these materials is AgNP (Qi et al., 2023).
Most commercial AgNP are coated with polymers for stabilization purposes, promoting a significant impact on living beings and the environment. For example, PVP, a non-ionic polymer that is highly soluble in water (Koczkur et al., 2015), is commonly used due to the absence of toxic effects reported in several biological systems (Zhao and Wang, 2012; Lacave et al., 2017). However, evidence shows that even if PVP is coating AgNP, these nanomaterials can promote harmful effects such as bioaccumulation in the small intestine and liver, embryo mortality, metabolism marker alterations in shrimps, reduction of fecundity in zebrafish, and tissue necrosis (Lacave et al., 2017; Orbea et al., 2017; Ribeiro et al., 2023).
The intake of AgNP in food, either by trophic transfer due to soil, water, or food contamination (Ottoni et al., 2020), reaches the small intestine, causing some effects. Several authors have reported relevant studies on the effects of AgNP in the small intestine using different biological models and health impact approaches (Osborne et al., 2015; Xia et al., 2015; Mosselhy et al., 2016; Lacave et al., 2017; Orbea et al., 2017; de Souza Azevedo et al., 2020). Osborne et al., 2014 found that a 20 nm-sized citrate-coated AgNP at a concentration of 1 ppm was deposited in the basolateral membrane of the small intestine mucosa of zebrafish exposed for 4 days (Osborne et al., 2015). Lacave et al. (2017) studied the bioaccumulation of AgNP in the small intestine and liver of zebrafish through trophic transference by consuming brine shrimp larvae exposed to PVP/PEI-coated AgNP (5 nm) at concentrations of 100 ng/L and 100 μg/L for 21 days, showing a dose-dependent metal accumulation in the intestine and liver, associated with deleterious effects in the liver such as vacuolization, necrosis, and reduction of lysosomal membrane stability; furthermore, Lacave et al. (2018) identified and studied the bioaccumulation of zebrafish waterborne exposed to maltose-coated AgNP (20 nm) and ionic silver, which resulted in the bioaccumulation of the two forms of silver in the liver, gills, and intestine, showing hyperplasia and inflammation in gills. In addition, Williams et al. (2015) found that citrate-stabilized AgNP (10, 75, and 110 nm) induced changes in the ileal–mucosal microbiota as a dose-dependent effect in Sprague–Dawley rats; Ognik et al. (2017) studied the effects of 5 nm AgNP in the small intestine of broiler chickens, finding a dose-dependent accumulation of silver (Ag) in the jejunum. Another interesting finding was the reduced absorption of K and Fe ions due to Ag presence in the small intestine (Ognik et al., 2017). In 2013, Shahare et al. found that AgNP ranging from 5 to 20 nm orally administered at 5, 10, 15, and 20 mg/kg in Swiss albino male mice caused epithelial cell microvilli and intestinal gland damage(Shahare and Yashpal, 2013). Despite these approaches, there are no close reports today about the physiological strategies that may explain the underlying mechanisms of action induced by the AgNP in the small intestine. For this reason, the present work investigates the role of PVP-coated AgNP on the contraction magnitude exerted upon the three small rat intestine segment types and the influence of NO and serotonin. This one also contributes to providing information linked to generating knowledge about the physiological behavior of micro-doses of AgNP, which is critical for establishing security margins in their use in healthcare as a potential antimicrobial agent.
PVP-coated AgNP (20 nm) were purchased from U.S. Research Nanomaterials Inc., Houston, Texas, and they were provided through the Sistema Nacional de Evaluacion Toxicologica de Nanomateriales (SINANOTOX). AgNP (100 mg/mL stock solution) were dispersed in sterile deionized water, and final concentrations of AgNP were prepared in Tyrode’s solution (physiological solution) at concentrations of 0.01, 0.1, 1, 10, and 100 μg/mL. For Tyrode’s solution, reagents sodium bicarbonate (NaHCO3), calcium chloride (CaCl2), and sodium chloride (NaCl) were purchased from Thermo Fisher Scientific (Waltham, MA, USA); magnesium chloride (MgCl2) was purchased from PQM® (Monterrey, N.L., Mexico); dextrose (C6H12O6) was purchased from CTR Scientific (Monterrey, N.L., Mexico); and sodium phosphate monobasic (NaH2PO4) was purchased from TIQ (Monterrey, N.L., Mexico). For nitric oxide determination using the Griess method, reagents vanadium (III) chloride (VCL3), sulfanilamide, and N-(1-naphthyl) ethylenediamine dihydro-chloride were purchased from Sigma-Aldrich (St Louis, MO, USA). Calibration standards for serotonin were purchased from Sigma-Aldrich (St. Louis, MO).
PVP-coated AgNP were suspended in Tyrode’s solution (NaCl, 136.9 mM; KCl, 2.69 mM; MgCl2, 1.05 mM; CaCl2, 1.8 mM; dextrose, 5.55 mM; NaH2PO4, 0.42 mM; and NaHCO3, 11.9 mM) (Okazaki et al., 1975) to perform the physicochemical NP characterization. To determine the NP morphology, a JSM-7401F Scanning Electron Microscope coupled with an energy-dispersive X-ray spectroscopy (EDS) detector at 2 kV with a 1.0 nm field resolution (Oxford) was used. Dynamic light scattering (DLS) analysis was conducted using a Particle Analyzer Litesizer 500 (Anton Paar, Graz, Austria) equipped with a semiconductor laser diode at a lengthwave of 658 nm and a disposable cell. The X-ray diffraction analysis was performed using a Bruker D8 Advance X-ray Diffractometer applying monochromatic radiation Cu Ka with 1.541 Ǻ in a measuring range from 10° to 90°. The Z potential was measured using the zeta meter SurPASS 3.
The experimental procedures in rats were performed following the guidelines of the National Institute of Health Guide for the Use and Care of Laboratory Animals and approved by the Animal Care and Use Committee from the Facultad de Ciencias Quimicas de la Universidad Autonoma de San Luis Potosi, Mexico (CONBIOETICA-24-CEI-003-20190726; Protocol CEID 2020-03). In brief, male Wistar rats (250-350 g) were housed in clear plastic containers under a 12-h dark/light cycle with water and food ad libitum. After 24 h of fasting, the rats were administered pentobarbital (50 mg/kg via intraperitoneal); once deep sedation was achieved, evidenced by the disappearance of the foot reflex, ear reflex, tail pinch reflex, and decreased heart rate (Tremoleda et al., 2012), the small intestine was excised and stored in a cold Tyrode’s solution for one hour once fecal matter and connective tissue were romeved. Then, 1-cm-long rings were dissected from each segment type (duodenum, jejunum, and ileum). Individual rings were placed in an isometric transducer in tissue baths containing aerated Tyrode’s solution (95% O2/5% CO2). A passive load of 1 g was applied, and the tissue was stabilized for 30 min. Then, the calibration was set to 0 for 10 min before adding KCl. Small intestine segments were pre-contracted by the administration of 0.03 M KCl, followed by five cumulative and increasing concentrations of PVP-coated AgNP (0.01, 0.1, 1, 10, and 100 μg/mL) or individual concentrations (0.01, 1, and 100 μg/mL). After those treatments, 100 nM sodium nitroprusside (SNP) was added to the chamber as a positive control for small intestine segment relaxation. Data were collected in real time using Poliview software.
NO was indirectly determined by measuring the ratio of the concentration of nitrites (NO2) and nitrates (NO3) from small intestine ring samples collected in the Tyrode’s solution after treatments were completed using the Griess method (Miranda et al., 2001). Samples (100 μL) were placed in a 96-well microplate, followed by the addition of 80 μL of VCl3 and a final addition of 10 μL of Griess A and Griess B reagents each. Microplates were stored in an incubator at 37°C for 45 min. A calibration curve was performed using the NO2/NO3 standard solution at 1, 2.5, 5, 10, 25, 50, 100, and 200 μM concentrations. Absorbance was measured using a 595-nm microplate reader (Bio-Rad, Hercules, CA, United States).
Serotonin was measured by high-performance liquid chromatography using a UV-diode detector (HPLC-UV) (Alliance HPLC, Waters Co). Acid extraction from a pool of small intestine segments under the previously described treatments was performed by homogenization (Omni THG homogenizer®) using 0.1 M perchloric acid, centrifuged at 10,000 rpm (MicroCL 21R Thermo®), and then filtered using an Acrodisc CR 13-mm Filter with a 0.2-μm polytetrafluoroethylene (PTFE) membrane. The supernatant obtained was then injected into the HPLC injection system. The mobile phase was acetonitrile: acetic acid (0.1) (10:90 v/v). A C18 ZORBAX (2.6 × 25 cm) column with a pore size of 5 μm and a rated flow of 1 mL/min at a length of 280 nm was used (Lesniak et al., 2013). Calibration standards for serotonin (Sigma-Aldrich, St. Louis, MO) were prepared in Milli-Q water, and calibration ranges were chosen based on previously reported papers (Lesniak et al., 2013). This method was beforehand validated by triplicate. Results were obtained using Empower Software.
Small intestine segments with or without treatment with AgNP were fixed in 10% paraformaldehyde-PBS for 24 h. Samples were processed with the automated tissue processor W-PR10 (Van Wessel, Queretaro, Mexico). Sample processing started with a dehydration process with sequential steps using xylene, ethanol 100%, ethanol 95%, and deionized water before the process finished with paraffin (Leica®) embedding. Paraffin sections of 3 μm were mounted on saline-coated slides using a microtome Olympus CUT 4060 (American Inc.), Serial No. 600368. Slides were observed using a Leica DM500 Microscope (Wetzlar, Germany) at × 4 and × 40 magnifications. Hematoxylin–eosin staining was performed to unveil whether PVP-coated AgNP could cause bioaccumulation and damage in tissue. It was carried out as follows: slides were hydrated with xylene, followed by ethanol 100% (two times for 2 minutes); then, slides were dipped in ethanol 95% (2 minutes) and rinsed with deionized water for 2 minutes; after that, slides were dipped in hematoxylin reagent for 3 min and rinsed with deionized water for 1 minute, and differentiator was added for 1 min and rinsed again with deionized water for 1 minute; then, slides were dipped in the blue reagent for 1 minute, then rinsed for 1 minute with deionized water, and then dipped again in the eosin reagent for 45 s; and then, sequential dipping in ethanol 95% (one time for 1 minute) and ethanol 100% (two times for 1 minute) was performed, followed by final dipping in xylene (two times for 2 minutes each) (Ramirez-Lee Manuel et al., 2017). All reagents were purchased from Hycel (Zap, Mexico). Grimelius staining (Grimelius Kit for special staining, Diapath), based on reducing silver ions to elemental silver (Ag0), was performed to evidence silver deposits in the tissues of small segments. Histological sections in the slides were deparaffinized and then hydrated with xylene, 100% ethanol, 95% ethanol, and deionized water. Slides were immersed in a 1% silver nitrate (AgNO3) for 3 h at 60°C. After impregnation, slides were immersed in a reduction solution of hydroquinone with sodium sulfate (Na2SO4) for 5 min at 60°C and then left to cool at room temperature and washed with deionized water for 3 min. For the second impregnation, slides were immersed in a 1% AgNO3 solution for 10 min at room temperature. Slides were drained and then covered with the reduction solution for 5 min at room temperature. Slides were washed for 3 min and then covered with 5% sodium thiosulfate (Na2S2O3) for 2 min. Finally, slides were washed with deionized water, dehydrated, and balsam mounted. Slides were observed using a Leica DM500 Microscope at × 4 and × 40 magnifications.
Data are expressed as the mean ± standard error and were subject to statistical analysis by one-way ANOVA with Dunnett’s correction. A value of p ≤ 0.05 was considered significant using GraphPad Prism 9 software (Dotmatics®, Boston, MA).
Figure 1 displays the characterization of the PVP-coated AgNP. Figure 1A shows the SEM micrograph of the PVP-coated AgNP suspended in Tyrode’s solution (physiological solution). AgNP exhibited a semispherical morphology with slight agglomerates and NPs with a size of ca. 20-40 nm. In addition, EDS analysis (Figure 1B) corroborated the presence of silver with slight traces of nitrogen, indicating the organic coating of PVP. As shown in Figure 1B, the zeta potential of different AgNP suspensions (0.01, 0.1, 1, 10, and 100 μg/mL) ranges from −16.2 to −22.2 mV, suggesting that AgNP tend to agglomerate due to Tyrode’s solution effect, associated with electrostatic attraction forces induced by the presence of salts, in agreement with Millour et al. (2020). The authors indicate the effect of increasing salt concentrations (NaCl) in 2-h aggregation kinetics, finding that AgNP hydrodynamic diameter started to increase at concentrations of 10 mM NaCl in a time range between 0 and 3,000 s, with a more evident increase at concentrations of 500 mM in a time range between 0 and 1,000 s (Millour et al., 2020). Furthermore, Kejlová et al. (2015) reported that the AgNP size increased from 48 ± 2 nm when the measure was performed in water, ranging from 122 to 202 nm when AgNP were diluted in phosphate-buffered saline (PBS). This effect was associated with salts in PBS (Kejlová et al., 2015). The polydispersity index (PDI) shows the distribution of particle sizes, providing information about the homogeneity or heterogeneity of particles in the physiological solution. A higher PDI indicates the formation of the cluster in the physiological solution as a function of the AgNP concentration (Hou et al., 2003; Gaumet et al., 2008). To corroborate the characteristic crystal structure of AgNP, Figure 1C shows the diffractogram of AgNP powder, demonstrating the presence of four crystalline patterns located at 38.2, 44.5, 64.8, and 77.4 in 2θ, which correspond to the planes (111), (200), (220), and (311) of the characteristic face-centered cubic structure (FCC) of the AgNP, consistent with the crystallographic chart JCPDS No. 04-0783, as shown in Figure 1D.
Figure 1. Physicochemical characterization of the PVP-coated AgNP. (A) SEM micrographs. (B) Elemental mapping (EDS) of AgNP; inset describes a table of the z-potential, DLS values with its respective SD, and the polydispersity index of different AgNP suspensions; (C) XRD pattern of AgNP; and (D) crystallographic chart JCPDS 04-0783 of AgNP.
To investigate whether PVP-coated AgNP administrations caused small intestine contraction modifications, the cumulative and increased concentrations of PVP-coated AgNP (0.01, 0.1, 1, 10, and 100 μg/mL) were administered to isolated small intestine rings. Treatments decreased the KCl-induced concentration-dependent contractile effect in each intestinal segment after PVP-coated AgNP administration. For all the segments, the final contraction percentage exerted a decrease in the contraction magnitude (Figures 2A–C, G). When SNP was administered, after the treatments with PVP-coated AgNP, the intestinal rings decreased, even more, the contraction percentage (relaxation) (Figures 2A–C).
Figure 2. Representative tension recordings, the correspondent graphs, NO production, and serotonin quantity derived from small intestine segments precontracted with 0.03 M KCl and treated in the presence of cumulative and increasing concentrations of PVP-coated AgNP and SNP (100 nM): (A) representative tension recording in the duodenum segments; (B) representative tension recording in the jejunum segments; (C) representative tension recording in the ileum segments; (D) NO quantification in the physiological solution that contained the duodenum segments; (E) NO quantification in the physiological solution that contained the jejunum segments; and (F) NO quantification in the physiological solution that contained the ileum segments. (G) Comparative contraction percentage graph of the three types of small intestinal segments treated with cumulative increasing administrations of PVP-coated AgNP; (H) serotonin quantification in the small intestine segment types. Results are representative of five independent experiments (in triplicate). For concentration of AgNP ranging from 0.1 to 100 μg/mL in all segments ****p < 0.0001; *p < 0.05 vs. control; **p < 0.01 vs. control; ***p < 0.001 vs. control; and ****p < 0.0001 vs. control.
Meanwhile, the decrease in the contraction percentage reached in the ileum segment due to SNP administration was 1.5 times greater than the control. Another important observation was that PVP-coated AgNP administrations also induced a dual profile and a tension decrease (Figure 2A–C).
To know whether NO was one of the mediators of PVP-coated AgNP responsible for decreasing the contractile actions or promoting relaxing effects, NO was measured using the Griess method in the physiological solution containing the small intestine segments. PVP-coated AgNP induced NO production, which increased the function of the intestine type of segment, and these NO quantities increased with the addition of SNP once the AgNP was administered (Figure 2D–F). In other words, NO quantification due to PVP-coated AgNP significantly increased vs. the correspondent control in the three small intestine segment types.
The serotonin in the small intestine segment types was measured to identify and evaluate its potential role as a mediator responsible for the contraction effect on the small intestine due to increasing and cumulative PVP-coated AgNP administration. Serotonin was detected in all the segments; however, the ileum was the only segment that showed a significant increase in this molecule, two-fold over the control. The serotonin levels in the duodenum and jejunum were not different from the basal quantification (Figure 2H).
As the physiological profile (relaxation) was observed due to the cumulative administrations of PVP-coated AgNP, the question was whether the individual and increasing concentrations of them could exert different physiological profiles. In this regard, we studied the effects of those single concentrations of PVP-coated AgNP in isolated small intestine segments (0.01, 1, and 100 μg/mL), and changes in small intestine tone were observed (Figures 3A, 4A, 5A). For all the segment types (the duodenum, jejunum, and ileum), these PVP-coated AgNP concentrations did not significantly decrease their contraction percentage. However, after the SNP administration, once the AgNP was administered, a significant relaxation was observed in all the single concentrations tested in all the intestine segment types (the duodenum, jejunum, and ileum), confirming, in addition, the tissue viability (Figures 3B, 4B, 5B). Another important observation is that this contractile profile was also observed in cumulative and increased PVP-coated AgNP administrations only when the lowest concentration of the first administration of the PVP-coated AgNP was done (Figure 2A–C).
Figure 3. Representative tension recordings, the correspondent graphs, NO production, and serotonin quantity derived from small intestine segments of duodenum precontracted with 0.03 M KCl and treated with individual PVP-coated AgNP and SNP (100 nM): (A) representative tension recording of the duodenum segment treated with PVP-coated AgNP; (B) contraction percentage of the duodenum segments treated with individual concentrations of PVP-coated AgNP (0.01, 1, and 100 μg/mL); and (C) NO quantification in the physiological solution that contained the duodenum treated with individual concentrations of PVP-coated AgNP (0.01, 1, and 100 μg/mL). Results are representative of five independent experiments (in triplicate). (D) Serotonin quantification from the duodenum segments treated with individual concentrations of PVP-coated AgNP at 0.01, 1, and 100 μg/mL. Results are representative of five independent experiments (in triplicate). *p < 0.05 vs. control; **p < 0.01 vs. control; ***p < 0.001 vs. control; and ****p < 0.0001 vs. control.
Figure 4. Representative tension recordings and graphs, NO production, and serotonin quantity derived from small intestine segments of the jejunum precontracted with 0.03 M KCl and treated with individual PVP-coated AgNP and SNP (100 nM): (A) representative tension recording of the jejunum segment treated with PVP-coated AgNP; (B) contraction percentage of the jejunum segments treated with individual concentrations of PVP-coated AgNP (0.01, 1, and 100 μg/mL); (C) NO quantification in the physiological solution that contained the jejunum treated with individual concentrations of PVP-coated AgNP (0.01, 1, and 100 μg/mL); and (D) serotonin quantification from the jejunum segments treated with individual concentrations of PVP-coated AgNP at 0.01, 1, and 100 μg/mL. Results are representative of five independent experiments (in triplicate). Values represent the mean ± SEM *p < 0.05 vs. control; **p < 0.01 vs. control; ***p < 0.001 vs. control; and ****p < 0.0001 vs. control.
Figure 5. Representative tension recordings and graphs, NO production, and serotonin quantity derived from small intestine segments of the ileum precontracted with 0.03 M KCl and treated with individual PVP-coated AgNP and SNP (100 nM): (A) representative tension recording of the ileum segment treated with PVP-coated AgNP; (B) contraction percentage of the ileum segments treated with individual concentrations of PVP-coated AgNP (0.01, 1, and 100 μg/mL); (C) NO quantification in the physiological solution that contained the ileum treated with individual concentrations of PVP-coated AgNP (0.01, 1, and 100 μg/mL); and (D) serotonin quantification from the ileum segments treated with individual PVP-coated AgNP at 0.01, 1, and 100 μg/mL. Results are representative of five independent experiments (in triplicate). Values represent the mean ± SEM *p < 0.05 vs. control; **p < 0.01 vs. control; ***p < 0.001 vs. control; and ****p < 0.0001 vs. control.
After SNP administration, the final contraction percentage reached for small intestine segments treated with PVP-coated AgNP-induced relaxation (Figures 3A, B, 4A, B, 5A, B) was similar to their controls (Supplementary Figure S1).
NO was measured to evaluate its influence on isolated small intestine segment tension. NO measurement in the physiological solution containing the correspondent segment type of the small intestine did not show an increase under their treatment with individual concentrations of PVP-coated AgNP (0.01, 1, and 100 μg/mL). However, significance in NO production was only reached after SNP administration, even in the presence of the previous AgNP tested (Figures 3C, 4C, 5C). These results showed that NO quantification was not different from the control in all the AgNP concentrations tested, even though the SNP increased NO levels after the AgNP treatments, and it was directly associated with the relaxation induced in the duodenum, jejunum, and ileum segments.
After evaluating the contractile effects and NO production from administering three individual PVP-coated AgNP concentrations (0.01, 1, and 100 μg/mL) in the three segment types, serotonin was quantified in the correspondent small intestine segment. When PVP-coated AgNP individual concentrations were administered, serotonin decreased in the three segments around two to three times versus the control, except for the 0.01 μg/mL of AgNP tested in the duodenum (Figures 3D, 4D, 5D).
Hematoxylin–eosin staining was carried out in small intestine segments treated with individual concentrations of PVP-coated AgNP (0.01, 1, 100 μg/mL) to find whether these NPs caused tissue damage. PVP-coated AgNP individual administrations cause epithelial structural impairment in small intestine segments and epithelial impairment at three concentrations (0.01, 1, and 100 μg/mL), mainly characterized by superficial epithelium sloughing and coagulative necrosis identified as a ghost of the pre-existing villous cores when hematoxylin–eosin staining was performed.
As shown in Figure 6, epithelial impairments were present in the mucosa layer to different degrees in the segment compared to their controls, showing the villi attached to the mucosal layer with all their cores intact. Moreover, morphological differences were found among segments; villi length changes according to the segment, being larger in the duodenum than in the jejunum and ileum, and villi in the jejunum are larger than villi found in the ileum (Figure 6). Yellow arrows show the intestinal layers (M, mucosa; SM, submucosa; MC: muscular; and S: serose). The PVP-coated AgNP individual administrations showed detachment of their villi in the duodenum and jejunum segments, with some villi or parts of them separated from the mucosal layer (Figures 6B–D, F–H) and focal necrosis in jejunum segment (Figure 6G). The ileum segments also showed epithelial detachment (Figures 6J–L) and coagulative necrosis, identified as ghosts of the pre-existing villi cores, as shown in the figures as detached tissue with blank inner holes (Supplementary Figure S6). These results indicate that these alterations are due to PVP-coated AgNP administration compared to their respective controls. (Figure 6A, E, I).
Figure 6. Hematoxylin–eosin staining. Image amplification (4 ×). Small intestine segments were treated with individual concentrations of AgNP (0.01, 1, and 100 μg/mL). (A) Duodenum control. (B) Duodenum treated with AgNP (0.01 μg/mL). (C) Duodenum treated with AgNP (1 μg/mL). (D) Duodenum treated with AgNP (100 μg/mL). (E) Jejunum control. (F) Jejunum treated with AgNP (0.01 μg/mL). (G) Jejunum treated with AgNP (1 μg/mL). (H) Jejunum treated with AgNP (100 μg/mL). (I) Ileum control. (J) Ileum treated with AgNP (0.01 μg/mL). (K) Ileum treated with AgNP (1 μg/mL). (L) Ileum treated with AgNP (100 μg/mL). Yellow arrows indicate small intestine layers (M, mucosa; SM, submucosa; MC, muscular; S, serose). Small intestine segments showing necrosis were indicated as (*), and superficial epithelium sloughing was indicated as (**).
Due to the structural changes observed in the intestinal segment types after the PVP-coated AgNP, we wonder whether AgNP could have accumulated in the intestinal villi and caused alterations in the tissue. Grimelius staining was performed to appreciate whether silver accumulations in the small intestine tissue existed after exposure to individual concentrations (0.01, 1, and 100 μg/mL) of PVP-coated AgNP for 15 min at 37°C. Intestinal tissue treated with AgNO3 was used as a positive control and stained through the Grimelius technique, showing darkened ECs (*) (Figure 7A). Staining showed an accumulation of silver in the apical region of the small intestine villi. This staining method showed that PVP-coated AgNP could accumulate in intestinal villi, observed as brown cumulus (*) (Figures 7C–F) but not in the control (Figure 7 B). Figures 7C–F display the brown cumulus in the duodenum and ileum segments treated with PVP-coated AgNP at 0.01 μg/mL concentrations.
Figure 7. Grimelius staining in villi of small intestine segments. (A) Small intestine positive control (enterochromaffin cell stained); (B) small intestine control (untreated tissue); (C) villi of the duodenum segments treated with AgNP (0.01 μg/mL); (D) villi of the duodenum segments treated with AgNP (0.01 μg/mL) (amplification from C); (E) villi of the ileum segments treated with AgNP (0.01 μg/mL); (F) villi of the ileum segments treated with AgNP (0.01 μg/mL) (amplification from E).
In the present work, we investigated the effects of PVP-coated AgNP administrations on small intestine segments by evaluating the contraction changes, chemical mediators’ production, and histological changes. In our findings, we identified that both PVP-coated AgNP-increasing administrations, cumulative and individual, exerted a transient tension decrease in the physiological profile, modifications in the NO production and quantity of serotonin in the isolated small intestine segments (the duodenum, jejunum, and ileum). However, differences were detected in the intestine contraction and the relation to the mediators studied in the function of the kind of administration.
The cumulative administrations of PVP-coated AgNP promoted 1) a decrease in contraction or relaxation of the same magnitude in all the segment types (the duodenum, jejunum, and ileum); 2) the NO production in all the segment types increased significantly in comparison to the respective control. However, the amount of NO production was lower in the jejunum than in the duodenum and ileum; 3) the addition of SNP at the end of the experiments further increased the amount of NO concerning that induced by PVP-coated AgNP, and this increase was more evident in the jejunum and ileum than in the duodenum; 4) the amount of serotonin was significantly increased selectively in the ileum segment but not in the duodenum and jejunum. These data suggest that NO could mediate the decrease in the intestinal contraction percentage or increase the relaxation promoted by the AgNP studied.
NO is a gas with a short lifetime that can be produced by endogenous and exogenous factors in epitheliums and diffuses into the smooth muscle cells, where it interacts with the soluble enzyme guanylate cyclase (sGC), which, in turn, promotes an increase in intracellular cyclic guanosine monophosphate (cGMP) levels, a second messenger that triggers the activation of the enzyme kinase G (PKG), activating potassium channels, facilitating their exit, inducing hyperpolarization of the cell, and a decrease in the opening of calcium channels (Gonzalez et al., 2004; Goodman and Gilman, 2011; Katzung, 2018). The addition of SNP, as an exogenous donor of NO, increased even more the levels of this molecule induced by the NPs, confirming the viability of the intestinal segments that responded to this stimulus, and it was visualized as relaxation, suggesting in addition that the presence of the cumulative concentrations of PVP-coated AgNP does not interfere in the NO donor mechanism of action inducing relaxation, contributing to the maintenance of the small intestine motility (Bulbul et al., 2013).
On the other hand, serotonin was selectively increased in the ileum segment, probably regulating in part the transient contractile decrement effect induced by PVP-coated AgNP; however, in the other intestinal segments (duodenum and jejunum), even if the effects were of the same magnitude, probably other mediators could be involved to get the same kind of effect in profile and magnitude.
The release of serotonin in the EC is carried out at the apical and basal borders. It acts as a paracrine tryptophan-derived molecule that, when activated, initiates signaling pathways that are responsible for physiological intestinal processes related to the peristaltic and secretory reflexes, ensuring efficient nutrient absorption (Larsson et al., 1990; Martel, 2006; De Schepper et al., 2008; Margolis and Pothoulakis, 2009; Alcalde Herrero, 2011). However, the enterocytes of the intestinal epithelium are also located in the mucosa layer and are responsible for recapturing and internalizing the serotonin in these cells through the expression of the serotonin transporter (SERT) located in the apical and basal borders (Chen et al., 1998; Martel et al., 2003; Iceta et al., 2006; Alcalde Herrero, 2011). In the present study, the cumulative and total relaxant effect induced by increasing concentrations of PVP-coated AgNP could specifically increase the amount of serotonin in the ileum in comparison to the other segments (the duodenum and jejunum), probably due to an impairment of SERT, and/or the critical activation of the serotonin receptors by the PVP-coated AgNP. In addition, it may be possible that the natural anatomy of the different intestinal segment types influences the common effect between them but with different mechanisms of action. It is reported that the organization of the segment type could influence the molecules involved in their effects. Ferrufino et al. identified that the ileum displays a minor number of villi and goblet cells, which produce mucoproteins, protecting the surface of the epithelial cells against different substances and microorganisms in comparison with the duodenum and jejunum (Ferrufino et al., 1996). Our histological analysis also confirmed, under control conditions, anatomic differences between the duodenum, jejunum, and ileum. Serotonin was probably partly increased by the additive stimulus induced by PVP-coated AgNP, which could be relevant in the ileum segment in response to an adaptative condition due to fewer protective systems. Another factor in this experimental approach is that the cumulative administration of those NPs could generate multiple signaling pathways due to the summative effects induced by each application. For this reason, evaluating the single administrations of PVP-coated AgNP was important to evaluate the physiological profile and the role that NO and serotonin could play in each concentration tested.
The individual administrations of increasing PVP-coated AgNP concentrations showed that 1) in all the segment types (the duodenum, jejunum, and ileum), they did not exert a significant decrease in the contraction percentage, in contrast to those induced in the cumulative and increasing concentrations; 2) no changes with the NO production existed in comparison to the corresponding control; 3) after the SNP administration and once each single/individual PVP-coated AgNP was administered, a significant relaxation associated with a NO increment was observed in all the single concentrations tested in all the intestinal segments (the duodenum, jejunum, and ileum), confirming the tissue viability, and again, in common to the cumulative concentrations of PVP-coated AgNP, these data suggest no interference of the NPs with NO and its relaxant effect; and 4) serotonin in all the segments and concentrations of PVP-coated AgNP significantly decreased (except in 0.01 μg/mL of PVP-coated AgNP tested in duodenum) in contrast to the cumulative experimental approach, where serotonin did not decrease in all the segments in comparison to the control.
This experimental approach (individual administrations of NPs) shows significant differences compared to the actions induced by cumulative concentrations of PVP-coated AgNP. The results indicate that even all the single and increasing concentrations of AgNP can confer a similar physiological profile on the intestinal contraction (no significant effect vs. the control with KCl), with no modifications in NO production but with a substantial decrease in the serotonin levels. This suggests that both the physiological effect and the mediators involved are independent of the concentration of the PVP-coated AgNP, unlike the effects observed with the cumulative concentrations of PVP-coated AgNP previously evaluated. These individual actions reveal and suggest, in contrast, that additive and continuous administrations over 15 min per administration could gradually condition the intestinal tissue to develop and activate cellular and molecular processes, distinguishing them from single administrations. Differences in the cumulative and individual concentrations of endogenous molecules are reported with differential mechanistic actions; for instance, Gonzalez et al., 2015 showed that cumulative and increasing concentrations of the hormone prolactin in isolated rat aortic rings promote a vasodilation effect in the first administration; however, the administration of subsequent concentrations promotes a biphasic effect consisting first of contraction followed by relaxation, which was not observed when single administrations were done, displaying only a relaxation dependent on endothelium but not NO. The contractile action observed in the biphasic effect could be associated with an endothelial mediator that could modify the vascular tone, such as arachidonic acid-derived or actions interrelated to the modulation of adrenergic receptors (Molinari et al., 2007; Gonzalez et al., 2015).
Regarding the intestinal relaxation induced by AgNP through individual administrations of PVP-coated AgNP, these data suggest no physiological interference by them with respect to the SNP relaxation as the NO donor, at least in the experimental conditions in this study. However, these NPs, per se, modified the NO and serotonin production profiles. Previously, and according to these findings, our research group demonstrated in isolated rat aortic rings that AgNP (37.5 nm) did not modify the effect of SNP in previous treatment with a low concentration of AgNP (5 μg/mL). However, the prior administration of these NPs specifically blocked the effect of acetylcholine (ACh)-induced endothelial NO-mediated relaxation, suggesting that AgNP are modifying the signaling pathway activated by ACh at the endothelial cell membrane level but not the effect of SNP as NO donor-induced independent endothelial relaxation, destined to diffuse through the cell membranes, reducing the intracellular calcium in the cytosol, promoting vasorelaxation, and maintaining the viability of the tissue (Rosas-Hernández et al., 2009). As we stated, NO production, as a mediator of these actions, showed that only the cumulative but no individual concentrations of PVP-coated AgNP increased this molecule. It is well-known that NO plays a pivotal role in the actions induced by AgNP. Earlier, we demonstrated that AgNP in different biological models can generate NO-regulating cytotoxic or protective actions. In coronary and aortic blood vessels, using ex vivo models, isolated and perfused rat hearts and isolated rat aortic rings, respectively, showed that AgNP (37.5 nm) induced dual effects. At low concentrations, they induced vasoconstriction, while at high concentrations, they stimulated vasodilation mediated by the activation of endothelial nitric oxide synthase (eNOS). eNOS, which is constitutively expressed, regulates and maintains organic homeostasis, such as the smooth muscle tone maintenance of biologic conducts, by producing low concentrations of nitric oxide (NO). However, in coronary blood vessels, AgNP (15 nm) promoted sustained vasoconstriction due, in part, to the increase in NO through the activation of inducible nitric oxide synthase (iNOS), stimulated when the tissues are injured or damaged (Rosas-Hernández et al., 2009). Furthermore, in isolated rat trachea rings that conform to part of the respiratory system, we show that AgNP (37.5 nm) induced toxic effects when modified by the contractile action in the presence of the endogenous contractile molecule, ACh, causing hyper-reactivity mediated by iNOS producing large amounts of NO related to allergic mechanisms (González et al., 2011); Park et al. (2010) found that the levels of NO in RAW264.7 monocyte/macrophage cell lines increased by two-fold with respect to their basal levels when they were exposed to AgNP (68.9 nm) concentrations of 1.6 ppm for 24 h (Park et al., 2010).
PVP-coated AgNP showed that cumulative concentrations induced an increase in the amount of serotonin only in the ileum but not in the duodenum and jejunum, in contrast to the different profiles observed in individual administrations of those NPs. Even though the serotonin levels in both experimental approaches appear unrelated to the physiological effects of the transient tension decrease of the isolated intestinal segment types, the individual administrations of PVP-coated AgNP induced a decrease in serotonin below the control values obtained in each type of intestinal segment compared to the cumulative concentrations of those NPs, whose values were equal to or greater than the control values of the serotonin measure. In this regard, cumulative PVP-coated AgNP administrations could trigger different signaling pathways related to the Trp and serotonin metabolisms (see Supplementary Figure S5). We expected that at specific concentrations of PVP-coated AgNP, serotonin synthesis could be triggered differently depending on the type of intestinal segment and in accordance with the histological differences between segments. A series of pieces of evidence demonstrate that serotonin and its metabolism can be the targets of metallic NPs at the intestinal level. For instance, Moringa oleífera-AgNP (25 nm) increased the regulation of the lipoprotein receptor-related protein 5 (LRP5) gene in two human cancer cell lines (HTC116 and SW480), inhibiting tryptophan hydroxylase-1 expression, the rate-limiting enzyme for serotonin biosynthesis, thereby conferring anticancer properties to these NPs in these cells (Althomali et al., 2022); Ahmed and Hussein (2017) found that in brain tissue, a depletion in serotonin levels occurred when male Wistar rats were given oral doses of 30 mg/kg body weight of PVP-coated AgNP (<100 nm). The serotonin levels decreased from 7.72 μg/g to 5.86 μg/g, causing brain neurotoxicity (Ahmed and Hussein, 2017). Özel et al. (2014) studied the variations in serotonin synthesis in zebrafish (Danio rerio) embryos and found that exposure to 20-nm nickel nanoparticles (NiNPs) and 40-nm copper oxide nanoparticles (CuONPs) at different concentrations (1, 5, 10, 20, 50, and 100 ppm) selectively modified serotonin levels depending on the concentration and developmental stage. They found that serotonin levels decreased significantly to 5.1 (±3.8), 8.8 (±0.4), and 3.9 (±3.8) nM at exposure concentrations of 20, 50, and 100 ppm NiNPs, respectively. Meanwhile, a serotonin concentration of 55.1 (±3.8) nM was obtained at a CuONP concentration of 5 ppm (Özel et al., 2014). Özel et al. (2013) also found that zebrafish embryos exposed to cerium oxide nanoparticles (CeO2NPs) at concentrations of 50 ppm experienced an almost six-fold depletion of serotonin in the small intestine (Özel et al., 2013). However, in our findings, the serotonin decrease was between two- and three-times vs. the control (in individual administrations of PVP-coated AgNP).
Therefore, further investigation is needed; as for cumulative and increasing concentrations, there could be a differential serotonin presence in the duodenum and jejunum compared with the ileum. We hypothesized that this difference induced by these NPs could trigger a mechanism of serotonin synthesis by the activation of tryptophan hydroxylase, the rate-limiting enzyme responsible for serotonin synthesis from Trp, and being increased in the ileum. Bogers et al. (1991) investigated the serotonin relaxation induced by NO in the canine isolated terminal ileum. They found that a concentration of 10−4 M of serotonin caused a NANC relaxation (a relaxation effect induced by other neurotransmitters but no adrenaline or acetylcholine), followed by a contraction, whereas concentrations of 10−5 M of serotonin caused a transient relaxation (Bogers et al., 1991). Other works reported that serotonin concentrations between 6.6 × 10−11 and 6.6 × 10−8 mol induced relaxation mediated by NO in Guinean pig stomachs (Meulemans et al., 1993), aspects that need to be explored to elucidate the fine mechanisms of action that crosslink NO and serotonin induced by PVP-coated AgNP.
To evaluate whether the intestinal tissues could be altered under that type of NP administration, hematoxylin and eosin histologic analyses demonstrated the degree of tissue integrity induced by PVP-coated AgNP at individual concentrations. Superficial epithelium sloughing and coagulative necrosis were identified as ghosts of the pre-existing villi cores from the small intestine isolated segments damage. However, the most altered segment type was the ileum, which displayed superficial epithelium sloughing and coagulative necrosis identified as a ghost of the pre-existing villi cores, although, focal necrosis was also observed in jejunum segment.
As mentioned, the small intestinal histology evidence showed mostly detached villus from the epithelium in the three segments when exposed to the PVP-coated AgNP, which were more evident and susceptible in the ileum segment. These results are based on previous evidence from zebrafish models. Yazdanparast et al. found that zebrafish fed with artificial fishmeal mixed with different doses (1, 4, and 6 mg/kg) of AgNP (16.6 nm) for 56 days caused necrosis and detachment of mucoid columnar epithelium (Yazdanparast et al., 2016); Pecoraro et al. also found that the adult zebrafish exposed to increased doses (8, 45, and 70 μg/kg) of AgNP (25 nm) for 30 days caused necrosis in the intestinal villi; Osborne et al. found that exposure to citrate-coated AgNP (20 nm) at 1 ppm for 4 days caused loss of microvilli, unlike 110 nm citrate-coated AgNP that did not show microvilli damage (Osborne et al., 2015).
The Grimelius staining method was used to determine whether PVP-coated AgNP internalize in small intestine tissue; our results showed these NPs were found in the villus after exposure to PVP-coated AgNP individual administrations. Bioaccumulation of silver in the small intestine has been seen in previous studies; Lacave et al., after feeding zebrafish for 3 and 26 days with brine shrimp exposed to 0.1, 1, 10, 100, and 1,000 μg/L of silver, found black silver deposits in the small intestine, showing a superior amount of silver after 26 days of exposure (Lacave et al., 2017); Osborne et al. found that zebrafish exposed for 4 days to 20-nm citrate-coated AgNP bioaccumulated this material in the basolateral membrane of the intestinal mucosa when it was stained using the silver-enhancing staining method (Osborne et al., 2015). Even in our experimental approach, the exposure time to PVP-coated AgNP (minutes) differed from the Lacave and Osborne studies; the bioaccumulation effect persists as a standard parameter, suggesting a rapid AgNP intestinal bioaccumulation process. The epithelial damage and the deposits of silver in the villus could also partly explain the differences in NO production and serotonin amounts in the three segment types after the PVP-coated AgNP exposure. However, this effect needs to be explored in detail.
In addition to the present results and evidence, we do not discard possible PVP-coated AgNP ionization processes that could influence the intestinal physiologic effects and their mediators. One of the reasons is that even though PVP represents an inert protective layer of the NPs; this polymer is soluble in water, which could let uncoated, to some degree, the AgNP, which could consequently suffer ionization in the intestinal microenvironment. Then, we asked how an ionization-silver process could affect intestinal contraction. An ionization control (silver nitrate-AgNO3) was tested to evaluate the physiological profile in all the segment types, observing that increasing and cumulative concentrations of AgNO3 (0.01–100 μg/mL) promoted a relaxation of the intestinal segment types lesser in magnitude than those induced by the PVP-coated AgNP (Supplementary Figure S3), suggesting that PVP-coated AgNP could exert their effects in part by the ionization process and in part by the intrinsic nature of the nanosized particle (Qi et al., 2023). Possibly, PVP could have dissolved (Odriscoll and Corrigan, 1982; Javed et al., 2020) in the intestinal microenvironment, leaving AgNP susceptible to ionization. Moreover, we confirmed that PVP alone did not modify the intestinal physiological effect neither NO production (Supplementary Figure S4).
In summary, since the intestine plays a fundamental role in nutrient absorption, the immune system, and the protective barrier against pathogens, understanding how AgNP can affect intestinal function is very relevant to evaluating their impact on gastrointestinal health and the whole body, providing valuable information on their safety, potential health effects, and present and future applications.
In conclusion, PVP-coated AgNP promoted differential physiologic profiles using an ex vivo model in the function of the cumulative or individual concentrations administered upon the small intestine at different segments (the duodenum, jejunum, and ileum), varying the magnitude of the intestinal contraction and mediators involved and studied here: NO and serotonin. These variations are likely associated with modifications in the intestinal tissue structure and the presence and bioaccumulation of silver in the villi of the small intestine mucosa. Previous research papers are based on the cellular, molecular, histological, and bioaccumulation effects of the AgNP approach. Nevertheless, limited information is available on the impact of AgNP associated with motility in intestinal processes. Thus, this work represents one of the first exploratory approaches related to the effects of these nanoparticles on the contraction of small intestine segments and the mediators involved and implicated. Thus, further studies are needed to understand the delicate mechanisms of action that underlie how AgNP could modify the peristaltic movements and, consequently, the absorption of nutrients. This research generates scientific knowledge that directs these efforts toward establishing safety margins related to the adequate use of AgNP and products elaborated based on AgNP in the digestive system, their implications in developing and progressing diseases, and their use in healthcare as antimicrobial agents.
The original contributions presented in the study are included in the article/Supplementary Material; further inquiries can be directed to the corresponding author.
The animal study was approved by the committee from the School of Chemistry of the University of San Luis Potosi, Mexico (CONBIOETICA-24-CEI-003-20190726; protocol number: CEID 2020-03). The study was conducted in accordance with the local legislation and institutional requirements.
JC-H: investigation, methodology, visualization, writing–original draft, writing–review and editing, data curation, and formal analysis. BE-S: investigation, methodology, supervision, visualization, and writing–review and editing, data curation, and formal analysis. PA-B: data curation, investigation, methodology, supervision, validation, visualization, and writing–review and editing. LG-L: investigation, methodology, visualization, and writing–review and editing. AV-S: investigation, methodology, visualization, and writing–review and editing. GL-B: investigation, methodology, and writing–review and editing. CG: investigation, methodology, conceptualization, funding acquisition, project administration, resources, supervision, validation, visualization, writing–original draft, and writing–review and editing.
The author(s) declare that financial support was received for the research, authorship, and/or publication of this article. This work was supported by Consejo Nacional de Humanidades, Ciencia y Tecnología (CONAHCyT) through the grants PN-2017-01-4710 and F003 - 316826. BE-S acknowledges to CONAHCYT through the project PRONAII-90 (2024).
The authors thank Dr. Ricardo Espinosa Tanguma for technical support in preserving small intestine samples and Dr. Gabriela Navarro Tovar for conceptual support related to the use of PVP. The authors are grateful to CONAHCyT for the scholarship (No. 756756) to JC-H.
The authors declare that the research was conducted in the absence of any commercial or financial relationships that could be construed as a potential conflict of interest.
All claims expressed in this article are solely those of the authors and do not necessarily represent those of their affiliated organizations, or those of the publisher, the editors, and the reviewers. Any product that may be evaluated in this article, or claim that may be made by its manufacturer, is not guaranteed or endorsed by the publisher.
The Supplementary Material for this article can be found online at: https://www.frontiersin.org/articles/10.3389/fnano.2024.1386312/full#supplementary-material
Ahmed, M. M., and Hussein, M. M. A. (2017). Neurotoxic effects of silver nanoparticles and the protective role of rutin. Biomed. Pharmacother. 90, 731–739. doi:10.1016/j.biopha.2017.04.026
Alcalde Herrero, A. I. (2011). “Importancia del sistema serotoninérgico en la fisiopatología intestinal,” in Libro de Ponencias: Discurso Leído En el acto de su recepción académica (Zaragoza: Cometa S.A), 1–64.
Althomali, A., Daghestani, M. H., Basil Almukaynizi, F., Al-Zahrani, S. A., Awad, M. A., Merghani, N. M., et al. (2022). Anti-colon cancer activities of green-synthesized Moringa oleifera –AgNPs against human colon cancer cells. Green Process Synth. 11 (1), 545–554. doi:10.1515/gps-2022-0052
Bertrand, P. P. (2006). Real-time measurement of serotonin release and motility in Guinea pig ileum. J. Physiol. 577 (2), 689–704. doi:10.1113/jphysiol.2006.117804
Bogers, J. J., Pelckmans, P. A., Boeckxstaens, G. E., De Man, J. G., Herman, A. G., and Van Maercke, Y. M. (1991). The role of nitric oxide in serotonin-induced relaxations in the canine terminal ileum and ileocolonic junction. Naunyn Schmiedeb. Arch. Pharmacol. 344 (6), 716–719. doi:10.1007/bf00174756
Bulbul, A., Bulbul, T., Sevimli, A., and Yilmaz, O. (2013). The effect of dietary supplementation of nitric oxide donor and inhibitor on nNOS expression in and motility of the small intestine of broilers. Biotech. Histochem 88 (5), 258–266. doi:10.3109/10520295.2013.769631
Calignano, A., Whittle, B. J. R., Di Rosa, M., and Moncada, S. (1992). Involvement of endogenous nitric oxide in the regulation of rat intestinal motility in vivo. Eur. J. Pharmacol. 229 (2–3), 273–276. doi:10.1016/0014-2999(92)90567-n
Chávez-Hernández, J. A., Velarde-Salcedo, A. J., Navarro-Tovar, G., and Gonzalez, C. (2024). Safe nanomaterials: from their use, application, and disposal to regulations. Nanoscale Adv. 6 (6), 1583–1610. doi:10.1039/d3na01097j
Chen, J.-X., Pan, H., Rothman, T. P., Wade, P. R., and Gershon, M. D. (1998). Guinea pig 5-HT transporter: cloning, expression, distribution, and function in intestinal sensory reception. Am. J. Physiol. Liver Physiol. 275 (3), G433–G448. doi:10.1152/ajpgi.1998.275.3.g433
De Schepper, H. U., De Man, J. G., Moreels, T. G., Pelksmans, P. A., and De Winter, B. Y. (2008). Review article: gastrointestinal sensory and motor disturbances in inflammatory bowel disease – clinical relevance and pathophysiological mechanisms. Aliment. Pharmacol. Ther. 27 (8), 621–637. doi:10.1111/j.1365-2036.2008.03624.x
de Souza Azevedo, J., Tâmisa Araújo Quintino, M., Ota Rogero, S., Tessari Zampieri, M. C., and Rogero, J. R. (2020). Histopathological changes in zebrafish exposed to sublethal concentrations of 89 nm silver nanoparticles for application in environmental diagnostics. Hum Ecol Risk Assess Int J 26 (5), 1207–1220. doi:10.1080/10807039.2018.1560219
Ferrufino, J. C., Taxa, L., and Gladis, A. (1996). Histología normal del intestino delgado. Rev. Medica Hered. 7 (1), 46–57. doi:10.20453/rmh.v7i1.507
Gaillet, S., and Rouanet, J.-M. (2015). Silver nanoparticles: their potential toxic effects after oral exposure and underlying mechanisms – a review. Food Chem. Toxicol. 77, 58–63. doi:10.1016/j.fct.2014.12.019
Garcia, X., and Stein, F. (2006). Nitric oxide. Semin. Pediatr. Infect. Dis. 17 (2), 55–57. doi:10.1053/j.spid.2006.04.002
Gaumet, M., Vargas, A., Gurny, R., and Delie, F. (2008). Nanoparticles for drug delivery: the need for precision in reporting particle size parameters. Eur. J. Pharm. Biopharm. 69 (1), 1–9. doi:10.1016/j.ejpb.2007.08.001
Gonzalez, C., Corbacho, A. M., Eiserich, J. P., Garcia, C., Lopez-Barrera, F., Morales-Tlalpan, V., et al. (2004). 16K-Prolactin inhibits activation of endothelial nitric oxide synthase, intracellular calcium mobilization, and endothelium-dependent vasorelaxation. Endocrinology 145 (12), 5714–5722. doi:10.1210/en.2004-0647
Gonzalez, C., Rosas-Hernandez, H., Jurado-manzano, B., Ramirez-Lee, M. A., Salazar-Garcia, S., Martinez-Cuevas, P. P., et al. (2015). The prolactin family hormones regulate vascular tone through NO and prostacyclin production in isolated rat aortic rings. Acta Pharmacol. Sin. 36 (5), 572–586. doi:10.1038/aps.2014.159
Gonzalez, C., Rosas-Hernandez, H., Ramirez-Lee, M. A., Salazar-García, S., and Ali, S. F. (2016). Role of silver nanoparticles (AgNPs) on the cardiovascular system. Arch. Toxicol. 90 (3), 493–511. doi:10.1007/s00204-014-1447-8
González, C., Salazar-García, S., Palestino, G., Martínez-Cuevas, P. P., Ramírez-Lee, M. A., Jurado-Manzano, B. B., et al. (2011). Effect of 45nm silver nanoparticles (AgNPs) upon the smooth muscle of rat trachea: role of nitric oxide. Toxicol. Lett. 207 (3), 306–313. doi:10.1016/j.toxlet.2011.09.024
Hall, J. E. (2016) Guyton and Hall textbook of medical physiology. 13th ed. Philadephia: Elsevier, 1046.
Hasler, W. L. (2009). Serotonin and the GI tract. Curr. Gastroenterol. Rep. 11 (5), 383–391. doi:10.1007/s11894-009-0058-7
Hou, D., Xie, C., Huang, K., and Zhu, C. (2003). The production and characteristics of solid lipid nanoparticles (SLNs). Biomaterials 24 (10), 1781–1785. doi:10.1016/s0142-9612(02)00578-1
Iceta, R., Mesonero, J. E., Aramayona, J. J., and Alcalde, A. I. (2006). Molecular characterization and intracellular regulation of the human serotonin transporter in Caco-2 cells. J. Physiol. Pharmacol. 57 (1), 119–130.
Javed, R., Zia, M., Naz, S., Aisida, S. O., Ain, N. ul, and Ao, Q. (2020). Role of capping agents in the application of nanoparticles in biomedicine and environmental remediation: recent trends and future prospects. J. Nanobiotechnology 18 (1), 172. doi:10.1186/s12951-020-00704-4
Katzung, B. G. (2018). Basic and clinical pharmacology. 12th ed. (United States: McGraw-Hill), 411–419.
Kejlová, K., Kašpárková, V., Krsek, D., Jírová, D., Kolářová, H., Dvořáková, M., et al. (2015). Characteristics of silver nanoparticles in vehicles for biological applications. Int. J. Pharm. 496 (2), 878–885. doi:10.1016/j.ijpharm.2015.10.024
Koczkur, K. M., Mourdikoudis, S., Polavarapu, L., and Skrabalak, S. E. (2015). Polyvinylpyrrolidone (PVP) in nanoparticle synthesis. Dalt Trans. 44 (41), 17883–17905. doi:10.1039/c5dt02964c
Lacave, J. M., Fanjul, Á., Bilbao, E., Gutierrez, N., Barrio, I., Arostegui, I., et al. (2017). Acute toxicity, bioaccumulation and effects of dietary transfer of silver from brine shrimp exposed to PVP/PEI-coated silver nanoparticles to zebrafish. Comp. Biochem. Physiol. Part C Toxicol. Pharmacol. 199, 69–80. doi:10.1016/j.cbpc.2017.03.008
Lacave, J. M., Vicario-Parés, U., Bilbao, E., Gilliland, D., Mura, F., Dini, L., et al. (2018). Waterborne exposure of adult zebrafish to silver nanoparticles and to ionic silver results in differential silver accumulation and effects at cellular and molecular levels. Sci. Total Environ. 642, 1209–1220. doi:10.1016/j.scitotenv.2018.06.128
Larsson, I., Grönstad, K. O., Dahlström, A., and Ahlman, H. (1990). Transport of serotonin from the rat jejunal lumen into mesenteric veins in vivo. Acta Physiol. Scand. 138 (3), 403–407. doi:10.1111/j.1748-1716.1990.tb08863.x
Lesniak, W. G., Jyoti, A., Mishra, M. K., Louissaint, N., Romero, R., Chugani, D. C., et al. (2013). Concurrent quantification of tryptophan and its major metabolites. Anal. Biochem. 443 (2), 222–231. doi:10.1016/j.ab.2013.09.001
Margolis, K. G., and Pothoulakis, C. (2009). Serotonin has a critical role in the pathogenesis of experimental colitis. Gastroenterology 137 (5), 1562–1566. doi:10.1053/j.gastro.2009.09.027
Martel, F. (2006). Recent advances on the importance of the serotonin transporter SERT in the rat intestine. Pharmacol. Res. 54 (2), 73–76. doi:10.1016/j.phrs.2006.04.005
Martel, F., Monteiro, R., and Lemos, C. (2003). Uptake of serotonin at the apical and basolateral membranes of human intestinal epithelial (Caco-2) cells occurs through the neuronal serotonin transporter (SERT). J. Pharmacol. Exp. Ther. 306 (1), 355–362. doi:10.1124/jpet.103.049668
Meulemans, A. L., Heisen, L. E., and Schuurkes, J. A. J. (1993). The role of nitric oxide (NO) in 5-HT-induced relaxations of the Guinea-pig stomach. Arch. Pharmacol. 348, 424–430. doi:10.1007/bf00171343
Millour, M., Gagné, J.-P., Doiron, K., Lemarchand, K., and Pelletier, É. (2020). Silver nanoparticles aggregative behavior at low concentrations in aqueous solutions. Colloids Surfaces A Physicochem Eng. Asp. 603, 125191. doi:10.1016/j.colsurfa.2020.125191
Miranda, K. M., Espey, M. G., and WinkRapid, D. A. A. (2001). A rapid, simple spectrophotometric method for simultaneous detection of nitrate and nitrite. Nitric Oxide 5 (1), 62–71. doi:10.1006/niox.2000.0319
Molinari, C., Grossini, E., Mary, DASG, Uberti, F., Ghigo, E., Ribichini, F., et al. (2007). Prolactin induces regional vasoconstriction through the β2-adrenergic and nitric oxide mechanisms. Endocrinology 148 (8), 4080–4090. doi:10.1210/en.2006-1577
Mosselhy, D. A., He, W., Li, D., Meng, Y., and Feng, Q. (2016). Silver nanoparticles: in vivo toxicity in zebrafish embryos and a comparison to silver nitrate. J. Nanoparticle Res. 18 (8), 222. doi:10.1007/s11051-016-3514-y
Odriscoll, K. M., and Corrigan, O. I. (1982). Chlorothiazide-polyvinylpyrrolidone (PVP) interactions: influence on membrane permeation (everted rat intestine) and dissolution. Drug Dev. Ind. Pharm. 8 (4), 547–564. doi:10.3109/03639048209022120
Ognik, K., Stępniowska, A., and Kozłowski, K. (2017). The effect of administration of silver nanoparticles to broiler chickens on estimated intestinal absorption of iron, calcium, and potassium. Livest. Sci. 200 (June), 40–45. doi:10.1016/j.livsci.2017.04.002
Okazaki, T., Ilea, V., Okazaki, A., Reisman, R. E., and Arbesman, C. E. (1975). The effect of Tris-buffered media and Tyrode physiologic saline solution on the antigenic release of histamine from human leukocytes. J. Allergy Clin. Immunol. 56 (1), 27–32. doi:10.1016/0091-6749(75)90031-7
Orbea, A., González-Soto, N., Lacave, J. M., Barrio, I., and Cajaraville, M. P. (2017). Developmental and reproductive toxicity of PVP/PEI-coated silver nanoparticles to zebrafish. Comp. Biochem. Physiol. Part C Toxicol. Pharmacol. 199, 59–68. doi:10.1016/j.cbpc.2017.03.004
Osborne, O. J., Lin, S., Chang, C. H., Ji, Z., Yu, X., Wang, X., et al. (2015). Organ-specific and size-dependent Ag nanoparticle toxicity in gills and intestines of adult zebrafish. ACS Nano 9 (10), 9573–9584. doi:10.1021/acsnano.5b04583
Ottoni, C. A., Lima Neto, M. C., Léo, P., Ortolan, B. D., Barbieri, E., and De Souza, A. O. (2020). Environmental impact of biogenic silver nanoparticles in soil and aquatic organisms. Chemosphere 239, 124698. doi:10.1016/j.chemosphere.2019.124698
Özel, R. E., Hayat, A., Wallace, K. N., and Andreescu, S. (2013). Effect of cerium oxide nanoparticles on intestinal serotonin in zebrafish. RSC Adv. 3 (35), 15298. doi:10.1039/c3ra41739e
Özel, R. E., Wallace, K. N., and Andreescu, S. (2014). Alterations of intestinal serotonin following nanoparticle exposure in embryonic zebrafish. Environ. Sci. Nano 1 (1), 27–36. doi:10.1039/c3en00001j
Park, E. J., Yi, J., Kim, Y., Choi, K., and Park, K. (2010). Silver nanoparticles induce cytotoxicity by a Trojan-horse type mechanism. Toxicol. Vitr. 24 (3), 872–878. doi:10.1016/j.tiv.2009.12.001
Qi, M., Wang, X., Chen, J., Liu, Y., Liu, Y., Jia, J., et al. (2023). Transformation, absorption and toxicological mechanisms of silver nanoparticles in the gastrointestinal tract following oral exposure. ACS Nano 17 (10), 8851–8865. doi:10.1021/acsnano.3c00024
Ramirez-Lee Manuel, A., Martinez-Cuevas, P. P., Rosas-Hernandez, H., Oros-Ovalle, C., Bravo-Sanchez, M., Martinez-Castañon, G. A., et al. (2017). Evaluation of vascular tone and cardiac contractility in response to silver nanoparticles, using Langendorff rat heart preparation. Nanomedicine Nanotechnol. Biol. Med. 13 (4), 1507–1518. doi:10.1016/j.nano.2017.01.017
Ribeiro, L. G., Rezende, K. F. O., Barbieri, E., and de Souza, A. O. (2023). Study of routine metabolism and acute toxicity of mycogenic silver nanoparticles on Palaemon pandaliformis (shrimp). Environ. Sci. Nano 10 (6), 1715–1729. doi:10.1039/d2en00726f
Rosas-Hernández, H., Jiménez-Badillo, S., Martínez-Cuevas, P. P., Gracia-Espino, E., Terrones, H., Terrones, M., et al. (2009). Effects of 45-nm silver nanoparticles on coronary endothelial cells and isolated rat aortic rings. Toxicol. Lett. 191 (2–3), 305–313. doi:10.1016/j.toxlet.2009.09.014
Shah, V., Lyford, G., Gores, G., and Farrugia, G. (2004). Nitric oxide in gastrointestinal health and disease. Gastroenterology 126 (3), 903–913. doi:10.1053/j.gastro.2003.11.046
Shahare, B., and Yashpal, M. (2013). Toxic effects of repeated oral exposure of silver nanoparticles on small intestine mucosa of mice. Toxicol. Mech. Methods 23 (3), 161–167. doi:10.3109/15376516.2013.764950
Tremoleda, J. L., Kerton, A., and Gsell, W. (2012). Anaesthesia and physiological monitoring during in vivo imaging of laboratory rodents: considerations on experimental outcomes and animal welfare. EJNMMI Res. 2 (1), 44. EJNMMI Research. doi:10.1186/2191-219x-2-44
Williams, K., Milner, J., Boudreau, M. D., Gokulan, K., Cerniglia, C. E., and Khare, S. (2015). Effects of subchronic exposure of silver nanoparticles on intestinal microbiota and gut-associated immune responses in the ileum of Sprague-Dawley rats. Nanotoxicology 9 (3), 279–289. doi:10.3109/17435390.2014.921346
Xia, G., Liu, T., Wang, Z., Hou, Y., Dong, L., Zhu, J., et al. (2015). The effect of silver nanoparticles on zebrafish embryonic development and toxicology. Nanomedicine, Biotechnol. 44 (4), 1116–1121. doi:10.3109/21691401.2015.1011803
Yazdanparast, T., Sharifpour, I., Soltani, M., and Esfahani, H. K. (2016). Evaluation of silver retention in different organs of zebrafish (Danio rerio) fed diet supplemented with silver nanoparticles. Int. J. Eng. Res. 5 (4), 269–274.
Keywords: silver nanoparticles, nitric oxide, serotonin, motility, nanomaterial, polyvinylpyrrolidone
Citation: Chávez-Hernández JA, España-Sánchez BL, Aguirre-Bañuelos P, Granados-López L, Velarde-Salcedo AJ, Luna-Bárcenas G and Gonzalez C (2024) Physiological evaluation of PVP-coated AgNP in the rat small intestine: an ex vivo approach. Front. Nanotechnol. 6:1386312. doi: 10.3389/fnano.2024.1386312
Received: 15 February 2024; Accepted: 13 May 2024;
Published: 20 June 2024.
Edited by:
René D. Peralta, CONACYT Center for Research in Applied Chemistry (CIQA), MexicoReviewed by:
Iram Maqsood, University of Maryland, United StatesCopyright © 2024 Chávez-Hernández, España-Sánchez, Aguirre-Bañuelos, Granados-López, Velarde-Salcedo, Luna-Bárcenas and Gonzalez. This is an open-access article distributed under the terms of the Creative Commons Attribution License (CC BY). The use, distribution or reproduction in other forums is permitted, provided the original author(s) and the copyright owner(s) are credited and that the original publication in this journal is cited, in accordance with accepted academic practice. No use, distribution or reproduction is permitted which does not comply with these terms.
*Correspondence: Carmen Gonzalez, Z29uemFsZXouY2FzdGlsbG9jYXJtZW5AdWFzbHAubXg=
Disclaimer: All claims expressed in this article are solely those of the authors and do not necessarily represent those of their affiliated organizations, or those of the publisher, the editors and the reviewers. Any product that may be evaluated in this article or claim that may be made by its manufacturer is not guaranteed or endorsed by the publisher.
Research integrity at Frontiers
Learn more about the work of our research integrity team to safeguard the quality of each article we publish.