- 1Instituto de Altos Estudios Espaciales Mario Gulich (CONAE), Universidad Nacional de Córdoba, Córdoba, Argentina
- 2National Council of Scientific and Technical Research (CONICET), Córdoba, Argentina
- 3 ICBIA, National Council of Scientific and Technical Research (CONICET), Córdoba, Argentina
- 4INFIQC, Physical Chemistry Department, Universidad Nacional de Córdoba, Córdoba, Argentina
- 5Catalan Institute of Nanoscience and Nanotechnology (ICN2), CSIC and BIST, Barcelona, Spain
- 6Instituto de Microelectrónica de Barcelona (IMB-CNM, CSIC), Barcelona, Spain
Nowadays, we witness remarkable technological progress alongside unprecedented challenges that threaten the delicate balance of our planet’s ecological system. Environmental contamination plays a central role in this, with rapid urbanization, industrialization, mining and agricultural practices intensifying the introduction of pollutants into the environment. This article highlights the potential synergy between two fields operating at vastly different scales: satellite technology and nanotechnology. This article delves into the offerings of each of these disciplines and examines how they can mutually contribute to the detection, prevention and mitigation of environmental pollution. Satellites play a crucial role in identifying and monitoring large-scale polluted areas, offering comprehensive insights into environmental challenges. They are indispensable in tracking air, water pollution levels, assessing land degradation, and monitoring changes in ocean health with relatively high spatial and temporal resolution. Nanotechnology leverages the unique properties of materials at sub-micron scale by offering amplified chemical reactivity and new optical, electronic, and magnetic attributes, enabling selective and sensitive sensors and rapid and efficient contaminant capture/degradation strategies. Emerging nanomaterials, along with nature-inspired and self-powered or self-sustaining designs, broaden capabilities for efficient solutions. Advanced nanocharacterization techniques deepen material understanding and quantification, while nanofabrication allows precise design of functional nano-devices. We believe the synergistic relationship between both fields can yield cooperative solutions, expediting effective measures and greatly influencing policy decisions. This article advocates for the collaboration between these two disciplines to foster impactful progress in facing global challenges.
1 Introduction
We confront a convergence of unprecedented challenges that threaten the fragile equilibrium of our planet’s ecological system. The concept of planetary boundaries, proposed in 2009, sheds light on pivotal thresholds (Steffen et al., 2015). These, if breached, could precipitate irreversible and catastrophic alterations in Earth’s biosphere. These boundaries encompass nine interconnected environmental processes that collectively delineate the planet stability and resilience: climate change, biosphere integrity change (biodiversity loss and species extinction), stratospheric ozone depletion, ocean acidification, biogeochemical flows, land-system change (deforestation, desertification), freshwater use, atmospheric aerosol loading, and introduction of novel entities. Each boundary represents a fundamental facet of our planet’s ecological integrity, necessitating vigilant stewardship.
Environmental pollution assumes a central role in shaping the status of these planetary boundaries. Rapid urbanization, industrialization, intensive agriculture, and deforestation have intensified the introduction of a wide array of pollutants into our environment, profoundly affecting biosphere integrity and influencing climate patterns. Moreover, contaminants disrupt nutrient cycles and modify ecosystem composition, exacerbating strain on Earth’s natural processes. Recognizing the intricate relationship between environmental pollution and climate change is paramount for formulating effective strategies to safeguard ecological balance and secure a sustainable future for next generations (Esplandiu et al., 2024).
This article aims to offer a perspective on the potent synergy between two fields operating at diametrically opposed dimensional scales: satellite technology and nanotechnology, but with a shared global concern. Satellite detection and imagery have a pivotal role in identifying and monitoring polluted areas, offering a comprehensive overview of environmental challenges. Satellites are now indispensable in tracking air pollution levels, pinpointing water pollution sources, assessing land degradation, monitoring changes in ocean health, and tracking the spread of hazardous materials. By amassing extensive geographical data from space, satellite technology facilitates source identification, tracks dispersion patterns, and aids in crafting effective mitigation strategies (Ustin and Middleton, 2021). However, in satellite remote sensing the measurements are indirect. The quantity measured is energy (radiance) and, consequently, complex inversion models must be applied to estimate the desired geophysical variables. In this context, nanoscience and nanotechnology can provide sensitive detection and relevant experimental and theoretical inputs of the targeted contaminant. This aids in developing novel and robust retrieval algorithms, validation tools, as well as efficient and precise environmental cleanup solutions for contaminant mitigation. Nanotechnology can also contribute with cost-effective, compact, light and high signal to noise remote sensors which can be equipped on-board in the payload of the satellites, among other hardware components. Indeed, a new time has started with the advent of the nanosatellites and picosatellites that allow to carry on technological tests at low cost, where nanotechnology is beginning to play a key role. Therefore, the cross talk between both disciplines is timely, especially for facing the pressing challenges of pollution and climate change (Kavvada et al., 2020). Accordingly, in this perspective we will detail potential synergies in this specific context.
2 What does satellite technology offer to environmental parameter assessment?
Satellite remote sensing offers unique advantages, lying in its ability to examine environmental processes with high spatial, temporal, and spectral resolution, from centimeters to kilometers, from minutes to months, and from nanometers to micrometers, respectively. Widely used remote sensors are the multispectral or hyperspectral ones. The first ones present few wide spectral bands while the second ones usually have more than one hundred narrow bands. Satellite technology enables answering fundamental environmental questions, such as: Where does the problem occur? When and for how long has it been occurring? What can be detected as an environmental indicator?
To address the first question (where), Earth Observation satellite sensors with medium to high spatial resolution are employed to detect spatial risk patterns and identify pollution sources. Examples of such sensors whose data are freely available include LANDSAT-OLI (30 m resolution), from NASA (National Aeronautics and Space Administration), and Sentinel 2-MSI (10–60 m resolution) from ESA (European Space Agency), among others (Germán et al., 2021).
For the second question (when), satellite missions with high temporal resolution, like TERRA, AQUA, Suomi-NNP, AURA from NASA, and Sentinel 3, 5, 6 from ESA, are the most used and freely available. While these satellites have lower spatial resolution as compared to those previously mentioned (starting from 250 m), they provide almost daily revisits, facilitating the study of system dynamics with high precision (Germán et al., 2020).
For the third question (what), a new generation of hyperspectral satellite missions is available, including PRISMA from the Italian Space Agency (ASI), EnMAP from the German Space Agency (DLR), and PACE from NASA, among others. These missions incorporate narrow bands (few nanometers) in the visible, near infrared and short wavelength infrared (VIS-NIR-SWIR) region, allowing for the discrimination of various materials, minerals, and pollutants from space (Peyghambari and Zhang, 2021).
Satellite remote sensing involves the measurement of radiation emitted, reflected, or scattered by a target, which must then be translated into a geophysical variable of interest, such as atmospheric temperature, chlorophyll-a concentration in waters, sea salinity, etc. (Cawse-Nicholson et al., 2021). This signal is a complex function of all elements involved in the measurement system, including the radiation source, the effects of the medium through which the wave propagates, and the physical/chemical characteristics of the illuminated target. The conversion of radiant energy into the geophysical data of interest is called the “inverse problem resolution” and involves the use of physical radiative transfer models, the development of empirical models or machine learning techniques (Rodgers, 2000; Topp et al., 2020) (Figure 1).
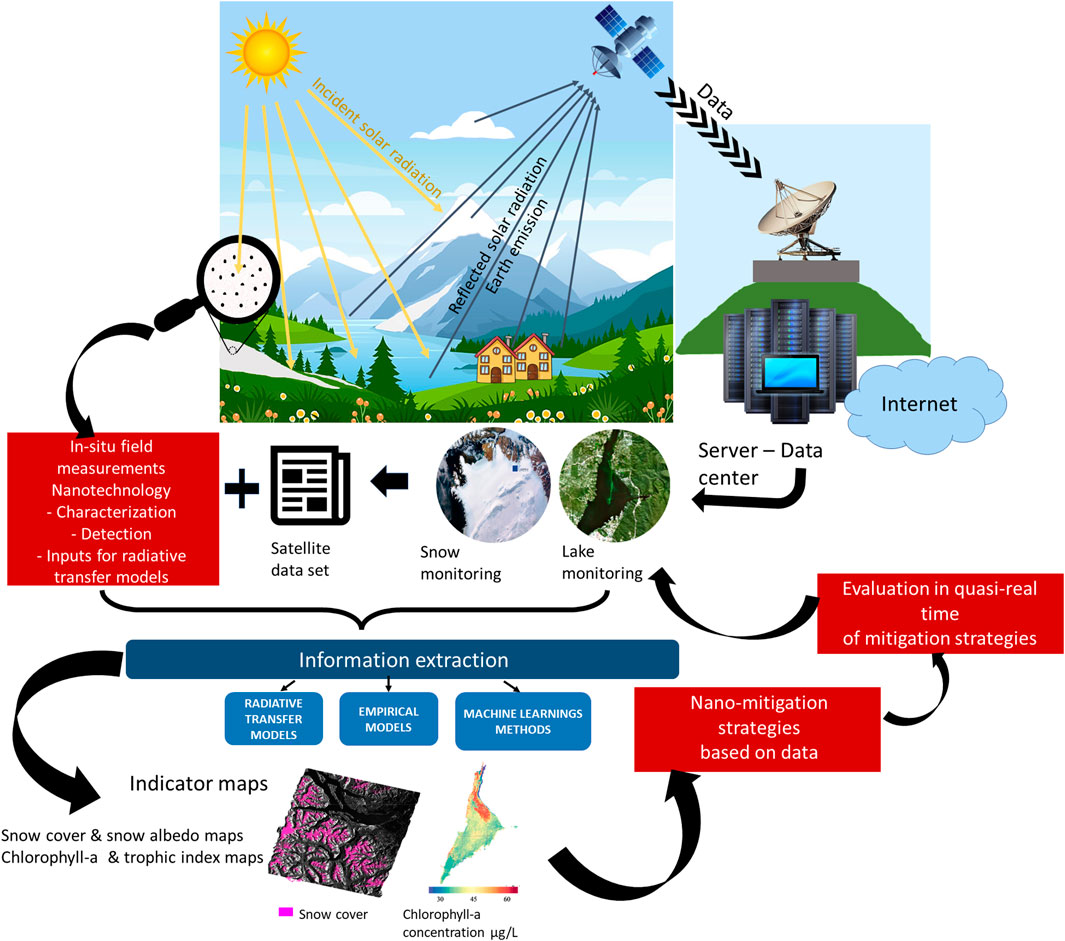
Figure 1. Conceptualizing the processes of remote sensing and nanotechnology in environmental contamination: Satellite sensors capture solar reflected radiation or emitted light from Earth’s surface across various electromagnetic wavelengths. The collected energy is transmitted, usually electronically, to a ground station. The data are then processed into maps or datasets. The information of the geophysical variable of interest (e.g., chlorophyll-a concentration, snow albedo, etc.) is extracted from the dataset by using radiative transfer models, empirical models and machine learning methods fed with on-field experimental inputs. The on-field inputs are achieved through the use of nanotechnological tools, enabling detection, characterization, and precise quantification. These inputs not only play a fundamental role in extracting environmental indicator information but also serve to validate the satellite data. The extracted information (illustrated here with indicator maps) can also be evaluated in terms of the United Nations’ 2030 Sustainable Development Goals directed to a more sustainable and equitable future. This evaluation guides the implementation of efficient nanotechnological strategies for pollution remediation, which can be effectively and rapidly monitored through remote sensing.
The use of radiative transfer models requires a detailed understanding of photons/target interaction and the wave propagating medium, considering the absorption and scattering processes. It must also include other phenomena such as fluorescence, which will depend on the illumination source characteristics and the target. It follows that for each system under study, there is a tailor-made model that is difficult to build, the most developed ones being those for atmospheric applications due to their lower complexity (Rodgers, 2000).
Empirical models are based on statistically significant relationships between field measurements and simultaneous radiance/reflectance data measured by the satellite sensor. One emblematic example is the regular and global measurement of chlorophyll-a concentration in oceans and seas, built from field and satellite data, by ESA or NASA. The good adjustment for this indicator enables high precision detection, reaching minimum thresholds on the order of 1.0 × 10−8 g/L (Hu et al., 2019). In addition to empirical modeling, machine learning techniques utilize data to train and validate models capable of estimating variables of interest from satellite measurements. However, in this case, a larger number of good-quality data is generally required (Zhang and Zhang, 2022). Both approaches inevitably require field measurements, either in-situ or on samples collected and taken to specific laboratories for characterization. Therefore, improved models require larger datasets and narrower sensor bands with adequate signal-to-noise ratios. Despite entering a new hyperspectral satellite era, the availability of field information to construct and validate globally applicable models for most applications remains limited (Dierssen et al., 2023).
There are few general operative algorithms for globally retrieving environmental pollutants from space. For instance, in the case of snow pollutants, i.e., black carbon, there is a lack of universally developed algorithms (Beltramone et al., 2021). Another emblematic issue is related to water-color applications, where most efforts are oriented towards developing models and implement algorithms based on biological causes (e.g., phytoplankton) rather than on organic/inorganic micro/nanoparticles (e.g., microplastics (Waqas et al., 2023), silicates (Wójcik-Długoborska et al., 2022) and acid drain mining residues (Isgró et al., 2022)). In these cases, nanotechnology applied to field detection and characterization can become an indispensable tool to address this gap.
3 What does nanoscience and nanotechnology offer to environmental sensing and remediation?
Nanotechnology offers transformative potential in preventing, detecting, and addressing natural resource contamination. It exploits surface effects and quantum confinement, which are the reasons behind the change of material properties and the rise-up of entirely new phenomena.
Nanoparticles and nanostructured surfaces possess a remarkable high surface/volume ratio, giving rise to a wealth of active sites (kinks, edges, steps, facets), promoting chemical reactivity, adsorption, and catalytic processes when compared to their larger-scale counterparts. This heightened reactivity can be harnessed to develop selective sensors by proper surface functionalization to introduce receptors to detect target contaminants, or by inducing surface interactions or reactions that lead to contaminant trapping or contaminant degradation/inactivation, respectively (Esplandiu et al., 2024).
Confinement effects in miniaturized materials lead to new optical, electronic, and magnetic properties, valuable for environmental sensing and resource restoration. Metallic nanoparticles display plasmonic effects leading to increased absorption/scattering processes, or induce local heat/hot electrons by light absorption (Kelly et al., 2003). These effects can be utilized for enhanced contaminant detectability even at low concentrations or for pollutant degradation treatments. Semiconducting nanostructures offer highly sensitive and stable electronic or photoluminescent sensing capabilities, with particle size-dependent properties due to changes in the band-gap with miniaturization. Nanostructuring and energy band engineering with doped homo/heterojunctions enable efficient light absorption and carrier separation, useful for light detection, power generation, and photocatalytic reactions for contaminant degradation (Xu et al., 2019). Miniaturization can also influence magnetic properties, allowing for rapid and reversible responses to magnetic fields, which can produce different forces/torques on magnetic nanoparticles inducing translation, rotation, and energy dissipation. All these processes can be exploited for magnetic trapping/transport and thermal degradation of the contaminants, as well as for promoting recyclability processes (Zhu et al., 2018).
Beyond the wealth of properties achieved with miniaturization, the advent of new materials such as carbon nanotubes, graphene, carbonitrides, transition metal dichalcogenides, and new porous nanoarchitectures, further expands the spectrum of available physical properties and phenomena for technological innovation (Konstantatos et al., 2012; Sun et al., 2016; Cao et al., 2022). Furthermore, nanotechnology draws on bioinspiration to create cutting-edge nanomaterials/systems, imitating natural structures for efficient photocatalysts and photodetectors (Serrà et al., 2019). Additionally, the concept of biomimetics is inspiring the development of self-propelled nanomotors/nanorobots that harness chemical energy for directed movement, enabling tasks such as water remediation on the fly (Esplandiu et al., 2018; Wang et al., 2019; Fraxedas et al., 2023). In this context, nanotechnology revolves around the search for self-sustaining systems or nanogenerators capable of collecting, converting and storing energy from the surrounding environment. Achieving autonomy without depending on external energy sources marks a significant step towards sustainability and self-sufficiency (Esplandiu et al., 2022).
The advancement/refinement of characterization and nanofabrication techniques have been crucial in unlocking the full potential of nanotechnology. Enhanced electron microscopes, scanning probe techniques, X-ray diffraction, photoemission spectroscopy, Raman spectroscopy, light scattering, and advanced spectrophotometry, have transformed nanomaterial studies, providing unprecedented information on their physicochemical properties. Additionally, in situ/operando synchrotron-based techniques offer real-time insights into dynamic nanomaterial behavior. Nanotechnology has also been boosted with the extensive battery of bottom-up/top-down nanofabrication techniques, enabling precise design of functional devices to exploit unique nano-scale phenomena. Controlled synthesis of a wide range of nanomaterials with tailored properties has revolutionized nanoscale materials engineering, leading to the creation of unprecedented structures and devices.
4 How can nanotechnology and satellite technology benefit each other?
The integration of nanotechnology and satellite imagery can offer a comprehensive multi-scale approach to enhance environmental contaminant detection/remediation. While satellite imagery is powerful for real-time, broad-scale pollution source identification with high spatial and spectral resolution at a relatively low cost (Cawse-Nicholson et al., 2021), its full exploitation in assessing environmental pollution is still in its early stages. There is an urgent need for the development of precise operative algorithms capable of retrieving information on a wide range of contaminants. Currently, these algorithms are based on models which are limited to a narrow scope of contaminants, underscoring the necessity for a more comprehensive approach to environmental indicators, particularly those in the form of organic/inorganic micro/nanoparticulates (Farré, 2020; Bakaeva and Le, 2022). To ensure accuracy and reliability in assessing environmental indicators, it is crucial to incorporate experimental inputs specific to the targeted contaminants to elaborate adjusted calibration tools. This involves experimental on-site/on-field assessments as an essential ingredient. Nanotechnology can play a significant role in providing highly sensitive on-ground nanosensors for concentration analysis in direct contact with the analyte with additional attributes such as being operative at low power, or self-powered, enabling remote/autonomous functionality, and importantly being in concert with satellite detection. On-field collection of samples for advanced nano-characterization techniques in research labs is equally important to improve/validate models, enabling researchers to delve into the physical, chemical, and biological features of contaminants, especially in the context of light/target radiation interactions. Theoretical developments in nanoscience, particularly in the realm of light interaction with micro/nanotargets, further contribute to such understanding by providing detailed insights (Kelly et al., 2003).
Incipient actions or proposals between these two disciplines are beginning to emerge. Vaseashta et al. have presented preliminary results of nano-based portable gas sensors at ground station, suggesting their potential synergy with satellite technology for air pollution analysis (Vaseashta et al., 2007). The authors proposed a strategy to provide complementarity in data collection. Ground sensors would offer detailed measurements at specific points, readily deployable to disseminate real-time pollution data to web servers providing a topological overview of the monitored areas. Meanwhile, satellites would provide a global view. However, actual results of this combined strategy working in concert are still lacking. This strategy would also allow for higher sampling rates and help validate satellite-derived data, enhancing confidence in remote sensing accuracy. Such a deeper cross-talk between on ground and satellite detection could hold promise for the accurate assessment of atmosphere pollution.
An example highlighting the necessity for selective model development and the integration of advanced nanocharacterization techniques is the case of black carbon nanoparticle contamination in snow (Rowe et al., 2019) (Figure 1). These particles, stemming from sources like incomplete combustion, biomass burning, volcanic activity, and other organic origins, absorb rather than reflect sunlight, reducing surface albedo. This leads to increased solar energy absorption, resulting in higher temperatures, accelerated snow and ice melt, and contributing to sea level rise. Such pollutants in snow are known as LAIs (Light Absorbing Impurities) which also include ash, dust, and algae, all of them lowering albedo in the VIS/NIR regions. In this scenario, the precise black carbon detection/quantification from radiation data presents ambiguities, due to the interference from other LAIs frequently mixed with black carbon. That should be overcome by using physical models and integrating experimental inputs as parameters to adjust physical-based equations. Another ambiguity comes from snow grain size that also decreases snow albedo in the VIS-NIR. This issue is also related to the snow melting process but is easily detected by microwave SAR (Synthetic Aperture Radar) data and can be included in the models. In this context, satellite sensors operating in the VIS-NIR and Microwave spectra (e.g., LANDSAT, Sentinel, SAOCOM Programs) facilitate the creation of maps with extensive spatial and temporal snow coverage and albedo patterns which can be related to the presence of LAIs, along with their impact on melt dynamics, through specific algorithms (Beltramone et al., 2021; Beltramone et al., 2023). In this context, the development and validation of the models that originate these algorithms, require laboratory-based characterization of these LAIs. Detecting, understanding the origin, and quantifying carbon pollution poses numerous challenges. Nanotechnology provides an array of techniques for this purpose, e.g., electron microscopy, X-ray diffraction, photoemission, Raman spectroscopy for structural/compositional characterization, among others. Precise carbon soot quantification can be achieved through advanced spectrophotometry (Integrating Sphere/Integrating Sandwich spectrophotometry ISSW), which can better account for carbon absorption without scattering interferences to accomplish improved calibration curves (Meinander et al., 2020). Moreover, through photothermal characterization deeper insights into their relative energy conversion impact in natural environments can be estimated and incorporated into the models.
Nanoscience can also provide useful theoretical/experimental inputs with respect to light interaction with contaminant nanoparticle dispersions in water and color development. Color serves as a compelling indicator closely linked to the contaminant shape, size, and surface modifications, which can be explored in research nanolabs (Kelly et al., 2003). Beyond color development by phytoplankton, there is a growing interest in utilizing satellite detection of environmental indicators through color mappings (Cael et al., 2023). This approach holds promise for correlating with inorganic nanopollutant dispersions resulting from natural processes, mining activities, or industrial wastes (Wójcik-Długoborska et al., 2022). Moreover, lab research on the absorption/fluorescence of biological and organic species in water samples further provides a useful avenue for refining fluorescence satellite detectors, enhancing their ability to detect and analyze such contaminants in water reservoirs (Garaba et al., 2023).
In this active feedback loop, the information gleaned from satellite detection enables nanotechnologists to address the critical areas for precise and efficient intervention. For instance, it can help to bring focus on the contaminant to be detected and deploy the corresponding sensors or organize a battery of advanced remediation tools. Let us illustrate this with a compelling example that leverages the combined efforts of satellite technology and nanotechnology in targeted sensing and remediation of algae bloom contamination (Germán et al., 2020). Specialized satellite sensors detect chlorophyll absorption/fluorescence patterns. Algorithms are then applied to interpret the spectral information and identify high chlorophyll-a concentration areas (as a proxy of potential algae blooms and eutrophication hazard). This data aids nanotechnologists in deploying sensors to detect algae-released toxins and implement effective and self-sustained remediation processes. For instance, innovative and ecological floatable platforms with tightly anchored photocatalytic nanomaterials can effectively neutralize algae and their toxins when exposed to sunlight. Satellite remote sensing can then offer insights into the remediation strategy’s effectiveness.
Additional points of interaction between nanotechnology and satellites extend to the satellite hardware development. Nanotechnology has the potential to reshape satellite technology through advanced nanofabrication tools, significantly impacting the remote sensing quality of environmental contaminants. A new era of small satellites like CubeSats (1–10 kg) and pico-satellites (<1 kg) is emerging, in which nanotechnology is key for developing highly efficient, compact sensors for diverse scientific data collection (Denizli et al., 2022). These satellites typically orbit in Low Earth Orbits (LEO), 160–2000 km above Earth, enabling high-resolution imaging and fast data transmission. LEO is ideal for Earth observation, environmental monitoring, and research. In contrast, Geostationary Orbits (GEO) at 35,786 km are mainly used for communication and meteorological satellites, although their recent increase in spatial resolution is expanding their capabilities to other applications (Denizli et al., 2022).
Nanotechnology offers countless contributions to improve the components of satellite technology. It plays a critical role in improving sensing and imaging capabilities, producing clearer and higher-resolution images. Optical components such as lenses, filters and mirrors, made from metamaterials and nanoscale optics, further improve satellite performance. Nanomaterials improve energy efficiency in components such as solar panels and energy storage systems. Nanocoatings can ensure satellite longevity by shielding against environmental factors. Nanoscale graphene-based transistors or novel 2D materials can enhance signal processing and data transmission. Moreover, the exceptional mechanical strength and lightweight nature of carbon-based materials, can reinforce structural satellite elements. The outstanding thermal conductivity and stability of carbon-based materials can be also harnessed to improve the satellite thermal control system. For instance, graphene-coated radiators efficiently dissipate excess heat and shield against electromagnetic interference, ensuring reliable communication/data transmission (Denizli et al., 2022).
Remote sensing devices play the pivotal role in detecting environmental hazards, including spectrometers, radiometers, high-resolution cameras, multispectral/hyperspectral sensors, and thermal infrared sensors. Most of these sensors operate by capturing a wide range of electromagnetic radiation, spanning from UV-visible to microwave wavelengths. Hyperspectral Imaging (HSI) and Multispectral Imaging (MSI) sensors are key for pollutant identification (Stuart et al., 2019). HSI systems collect over 100 spectral bands of 10–20 nm width, while MSI systems gather fewer, generally non-contiguous bands of a targeted object on the Earth’s surface (Vagni, 2007; Stuart et al., 2019). HSI excels in capturing targeted object fingerprints, discriminating among other materials due to its finer spectral resolution. The spectral image sensor is complex, including wavelength-dispersive devices (diffraction gratings, prisms), or interferometers to separate the collected broadband light into different wavelengths. These wavelengths are then projected to the image sensor detector based on photovoltaic semiconductor arrays, which constitute the pixels to build up the image. Although silicon photodiodes can be used for the visible region, planar HgCdTe or alloys of Group III-V (InAs, GaAs, InGaAs, and InSb) are utilized for extending the detection into the IR range (Xu et al., 2020). Recent advancements in nanomaterials are driving the development of novel photodetectors, leveraging nanostructured arrays composed of nanowires (NWs), nanotubes, nanosheets, 2D materials, quantum wells, dots, and discs, alongside integrating plasmonic nanostructures (Li et al., 2023). These nanostructured arrays enhance light trapping and absorption, besides offering size tunable optoelectronic responses conferring distinct properties from those achievable with planar photodetectors. Some studies are starting to show potential in using nanostructured III−V alloys (Li et al., 2023), dichalcogenides (Kim et al., 2021) or other layered semiconductors due to their direct and size-tunable bandgap, absorption coefficients, and carrier transport properties. These advancements open up opportunities for developing a next generation of low-cost and high-performance photodetection/imaging systems featuring high photoconductivity gain, responsivity, detectivity, high signal/noise ratio, low dark current, and compatibility with CMOS electronics. Some examples in this direction are the use of HgTe quantum dot arrays or NW arrays made of InP, InGaAs, GaAsSb or combined with other quantum nanoheterostructures (Tang et al., 2019; Li et al., 2023). These NWs with variable diameters and pitches, enabling efficient absorption of incoming radiation through optimal coupling to the optical modes of the NW arrays. Layered 2D-materials like MoS2, along with their combination with plasmonic/quantum dot materials, and plasmonic black phosphorus, also show potential in achieving enhanced optoelectronic remote sensing, especially in the NIR and mid-infrared (MIR) ranges (Chen et al., 2017; Miao et al., 2018; Kim et al., 2021). Moreover, nanotechnology could facilitate the development of filter-free image sensor pixels by harnessing the size-tunable optical properties of the nanomaterials that could be selective for specific wavelengths. One approach in this line is the development of a wavelength-selective multipixel photodetector based on GaAsSb nanowire arrays of different diameters (Li et al., 2021). Furthermore, nanofabrication enables the integration of these nanostructured photodetectors into flexible polymers such as PDMS, polyimide, or PET, paving the way for the production of flexible photodetectors (Li et al., 2023). In line with that, graphene is another promising material, enabling broadband light interaction (UV-microwaves) (Konstantatos et al., 2012; Cao et al., 2022; Pham et al., 2022; Zhang et al., 2022) and flexible optoelectronics. Ultrafast graphene-based photodetectors have been demonstrated, converting light into electrical signals with exceptional speed and sensitivity (Pham et al., 2022; Zhang et al., 2022). These advancements point towards flexible and versatile photodetectors with applications in imaging, remote sensing, high-speed communications, and infrared cameras, particularly compelling in the spectral range from THz to MIR due to the numerous spectral fingerprints of key chemicals, gases, and biological agents.
5 Outlook
According to this brief perspective, cross-talk between these fields can benefit various fronts:
- Alleviating economic burdens by boosting the development of picosatellite technology to test technological satellite endeavors at low cost as compared to satellites of higher earth orbits.
- Advancing satellite technology through robust, compact and light component development and innovative miniaturization/nanofabrication processes, including the creation of simplified remote sensors with unparalleled sensitivity.
- Refining satellite information retrieval models and validation tools for environmental indicators, using high quality on-ground experimental inputs of targeted objects from advanced nanocharacterization tools and sensitive ground nanosensors.
- Refining retrieval models with theoretical inputs from nanoscience leading to gain deeper insights into light/micro-nanoparticulate interactions.
- Improving accuracy in pollution assessment, source identification, and transport of contaminants, facilitating targeted remediation strategies and surveillance.
- Facilitating the creation of more accurate geospatial data libraries/maps for environmental quality indicators to strengthen scientific evidence and influence decision-makers toward environmental protection actions.
Therefore, more joint efforts in these fields could significantly aid in effectively addressing global challenges of environmental pollution and climate change.
Data availability statement
The original contributions presented in the study are included in the article, further inquiries can be directed to the corresponding author.
Author contributions
AF: Conceptualization, Writing–original draft. MB: Writing–review and editing. CS: Writing–review and editing. FN: Writing review and editing, Conceptualzation. MBP: Writing–review and editing. JR: Writing–review and editing. BS: Writing–review and editing. JF: Writing–review and editing. ME: Conceptualization, Writing–original draft, Writing–review and editing.
Funding
The author(s) declare that financial support was received for the research, authorship, and/or publication of this article. This research was supported by the Spanish Ministry of Economy and Competitiveness (MINECO) under Contracts No. PID 2021-124568NB-I00 and TED 2021-129898B-C21 and by CONICET grant PIBBA-2022–2023 (No 28720210100854CO), ITAREO project (Resol 2020-337-APN-MCT), MinCyT (Convocatoria Argentina Contra el Hambre 2020) and FONCyT (PICT-2021-GRF-TII00393) The ICN2 is funded by the CERCA program/Generalitat de Catalunya. The ICN2 is supported by the Severo Ochoa Centres of Excellence program, Grant CEX 2021-001214-S, funded by MCIN/AEI/10.13039.501100011033.
Acknowledgments
We also acknowledge the scientific exchange and support of the Centre for Molecular Water Science (CMWS).
Conflict of interest
The authors declare that the research was conducted in the absence of any commercial or financial relationships that could be construed as a potential conflict of interest.
The handling editor GF declared a past co-authorship with the authors JF, MJE.
Publisher’s note
All claims expressed in this article are solely those of the authors and do not necessarily represent those of their affiliated organizations, or those of the publisher, the editors and the reviewers. Any product that may be evaluated in this article, or claim that may be made by its manufacturer, is not guaranteed or endorsed by the publisher.
References
Bakaeva, N., and Le, M. T. (2022). Determination of urban pollution islands by using remote sensing technology in Moscow, Russia. Ecol. Inf. 67, 101493. doi:10.1016/j.ecoinf.2021.101493
Beltramone, G., Frery, A. C., Rotela, C., Germán, A., Bonansea, M., Scavuzzo, C. M., et al. (2023). Identification of seasonal snow phase changes from C-band SAR time series with dynamic thresholds. IEEE J. Sel. Top. Appl. Earth Observations Remote Sens., 1–14. doi:10.1109/jstars.2023.3281149
Beltramone, G., Scavuzzo, C. M., German, A., Bonansea, M., and Ferral, A. (2021). “Surface reflectance simulations of fresh and aged snow with light absorbing impurities,” in 2021 XIX workshop on information processing and control (RPIC) (Germany: IEEE), 1–6.
Cael, B. B., Bisson, K., Boss, E., Dutkiewicz, S., and Henson, S. (2023). Global climate-change trends detected in indicators of ocean ecology. Nature 619 (7970), 551–554. doi:10.1038/s41586-023-06321-z
Cao, R., Fan, S., Yin, P., Ma, C., Zeng, Y., Wang, H., et al. (2022). Mid-infrared optoelectronic devices based on two-dimensional materials beyond graphene: status and trends. Nanomaterials 12, 2260. doi:10.3390/nano12132260
Cawse-Nicholson, K., Townsend, P. A., Schimel, D., Assiri, A. M., Blake, P. L., Buongiorno, M. F., et al. (2021). NASA's surface biology and geology designated observable: a perspective on surface imaging algorithms. Remote Sens. Environ. 257, 112349. doi:10.1016/j.rse.2021.112349
Chen, P. Y., Argyropoulos, C., Farhat, M., and Gomez-Diaz, J. S. (2017). Flatland plasmonics and nanophotonics based on graphene and beyond. Nanophotonics 6, 1239–1262. doi:10.1515/nanoph-2016-0137
Denizli, A., Alencar, M. S., Nguyen, T. A., and Motaung, D. (2022). Nanotechnology-based smart remote sensing networks for disaster prevention. Germany: Elsevier.
Dierssen, H. M., Gierach, M., Guild, L. S., Mannino, A., Salisbury, J., Schollaert Uz, S., et al. (2023). Synergies between NASA's hyperspectral aquatic missions PACE, GLIMR, and SBG: opportunities for new science and applications. J. Geophys. Res. Biogeosciences 128 (10), e2023JG007574. doi:10.1029/2023jg007574
Esplandiu, M. J., Bastus, N., Fraxedas, J., Ihmaz, I., Puntes, V., Radjenovic, J., et al. (2024). Interfacial phenomena in nanotechnological applications for water remediation. Encycl. Solid-Liquid Interfaces, 465–484. doi:10.1016/b978-0-323-85669-0.00066-0
Esplandiu, M. J., Reguera, D., Romero-Guzmán, D., Gallardo-Moreno, A. M., and Fraxedas, J. (2022). From radial to unidirectional water pumping in zeta-potential modulated Nafion nanostructures. Nat. Commun. 13, 2812. doi:10.1038/s41467-022-30554-7
Esplandiu, M. J., Zhang, K., Fraxedas, J., Sepulveda, B., and Reguera, D. (2018). Unraveling the operational mechanisms of chemically propelled motors with micropumps. Acc. Chem. Res. 51, 1921–1930. doi:10.1021/acs.accounts.8b00241
Farré, M. (2020). Remote and in situ devices for the assessment of marine contaminants of emerging concern and plastic debris detection. Curr. Opin. Environ. Sci. Health 18, 79–94. doi:10.1016/j.coesh.2020.10.002
Fraxedas, J., Reguera, D., and Esplandiu, M. J. (2023). Collective motion of Nafion-based micromotors in water. Faraday Discuss. 249, 424–439. doi:10.1039/d3fd00098b
Garaba, S. P., Albinus, M., Bonthond, G., Flöder, S., Miranda, M. L., Rohde, S., et al. (2023). Bio-optical properties of cyanobacterium Nodularia spumigena. Earth Syst. Sci. Data Discuss. 2023, 1–27. doi:10.5194/essd-2023-23
Germán, A., Andreo, V., Tauro, C., Scavuzzo, C. M., and Ferral, A. (2020). A novel method based on time series satellite data analysis to detect algal blooms. Ecol. Inf. 59, 101131. doi:10.1016/j.ecoinf.2020.101131
Germán, A., Shimoni, M., Beltramone, G., Rodríguez, M. I., Muchiut, J., Bonansea, M., et al. (2021). Remote Sensing Applications: society and Environment Space-time monitoring of water quality in a eutrophic reservoir using SENTINEL-2 data - a case study of San Roque. Argentina 24, 100614. doi:10.1016/j.rsase.2021.100614
Hu, C., Feng, L., Lee, Z., Franz, B. A., Bailey, S. W., Werdell, P. J., et al. (2019). Improving satellite global chlorophyll-a data products through algorithm refinement and data recovery. J. Geophys. Res. Oceans 124 (3), 1524–1543. doi:10.1029/2019JC014941
Isgró, M. A., Basallote, M. D., Caballero, I., and Barbero, L. (2022). Comparison of UAS and sentinel-2 multispectral imagery for water quality monitoring: a case study for acid mine drainage affected areas (SW Spain). Remote Sens. 14 (16), 4053. doi:10.3390/rs14164053
Kavvada, A., Metternicht, G., Kerblat, F., Mudau, N., Haldorson, M., Laldaparsad, S., et al. (2020). Towards delivering on the sustainable development goals using earth observations. Remote Sens. Environ. 247, 111930. doi:10.1016/j.rse.2020.111930
Kelly, K. L., Coronado, E., Zhao, L. L., and Schatz, G. C. (2003). The optical properties of metal nanoparticles: the influence of size, shape, and dielectric environment. J. Phys. Chem. B 107 (3), 668–677. doi:10.1021/jp026731y
Kim, S., Zagni, N., Choo, S., Liu, N., Baek, S., Bala, A., et al. (2021). Highly sensitive active pixel image sensor array driven by large-area bilayer MoS2 transistor circuitry. Nat. Commun., 1–11. doi:10.1038/s41467-021-23711-x
Konstantatos, G., Badioli, M., Gaudreau, L., Osmond, J., Bernechea, M., De Arquer, F. P. G., et al. (2012). Hybrid graphene quantum dot phototransistors with ultrahigh gain. Nat. Nanotechnol. 7, 363–368. doi:10.1038/nnano.2012.60
Li, Z., He, Z., Xi, C., Zhang, F., Huang, L., Yu, Y., et al. (2023). Review on III–V semiconductor nanowire array infrared photodetectors. Adv. Mat. Technol. 8. doi:10.1002/admt.202202126
Li, Z., Trendafilov, S., Zhang, F., Allen, M. S., Allen, J. W., Dev, S. U., et al. (2021). Broadband GaAsSb nanowire array photodetectors for filter-free multispectral imaging. Nano Lett. 21, 7388–7395. doi:10.1021/acs.nanolett.1c02777
Meinander, O., Heikkinen, E., Aurela, M., and Hyvärinen, A. (2020). Sampling, filtering, and analysis protocols to detect black carbon, organic carbon, and total carbon in seasonal surface snow in an urban background and arctic Finland (>60° N). Atmosphere 11 (9), 923. doi:10.3390/atmos11090923
Miao, J., Song, B., Xu, Z., Cai, L., Zhang, S., Dong, L., et al. (2018). Single pixel black phosphorus photodetector for near-infrared imaging. Small 14, 1–7. doi:10.1002/smll.201702082
Peyghambari, S., and Zhang, Y. (2021). Hyperspectral remote sensing in lithological mapping, mineral exploration, and environmental geology: an updated review. J. Appl. Remote Sens. 15, 1–25. doi:10.1117/1.jrs.15.031501
Pham, P. V., Bodepudi, S. C., Shehzad, K., Liu, Y., Xu, Y., Yu, B., et al. (2022). 2D heterostructures for ubiquitous electronics and optoelectronics: principles, opportunities, and challenges. Chem. Rev. 122, 6514–6613. doi:10.1021/acs.chemrev.1c00735
Rodgers, C. (2000). Inverse methods for atmospheric sounding: theory and practice. Singapore: World Scientific.
Rowe, P. M., Cordero, R. R., Warren, S. G., Stewart, E., Doherty, S. J., Pankow, A., et al. (2019). Black carbon and other light-absorbing impurities in snow in the Chilean Andes. Sci. Rep. 1, 4008. doi:10.1038/s41598-019-39312-0
Serrà, A., Zhang, Y., Sepúlveda, B., Gómez, E., Nogués, J., Michler, J., et al. (2019). Highly active ZnO-based biomimetic fern-like microleaves for photocatalytic water decontamination using sunlight. Appl. Catal. B Environ. 248, 129–146. doi:10.1016/j.apcatb.2019.02.017
Steffen, W., Richardson, K., Rockström, J., Cornell, S. E., Fetzer, I., Bennett, E. M., et al. (2015). Sustainability. Planetary boundaries: guiding human development on a changing planet. Science 347, 1259855. doi:10.1126/science.1259855
Stuart, M. B., McGonigle, A. J. S., and Willmott, J. R. (2019). Hyperspectral imaging in environmental monitoring: a review of recent developments and technological advances in compact field deployable systems. Sensors Switz. 19, 3071. doi:10.3390/s19143071
Sun, P., Wang, K., and Zhu, H. (2016). Recent developments in graphene-based membranes: structure, mass-transport mechanism and potential applications. Adv. Mat. 28, 2287–2310. doi:10.1002/adma.201502595
Tang, X., Ackerman, M. M., and Guyot-Sionnest, P. (2019). Acquisition of hyperspectral data with colloidal quantum dots. Laser Photonics Rev. 13. doi:10.1002/lpor.201900165
Topp, S. N., Pavelsky, T. M., Jensen, D., Simard, M., and Ross, M. R. V. (2020). Research trends in the use of remote sensing for inland water quality science: moving towards multidisciplinary applications. WaterSwitzerl. 12, 169–234. doi:10.3390/w12010169
Ustin, S. L., and Middleton, E. M. (2021). Current and near-term advances in Earth observation for ecological applications. Ecol. Process. 10, 1–57. doi:10.1186/s13717-020-00255-4
Vagni, F. (2007). Survey of hyperspectral and multispectral imaging technologies. Available at: http://www.dtic.mil/docs/citations/ADA473675.
Vaseashta, A., Vaclavikova, M., Vaseashta, S., Gallios, G., Roy, P., and Pummakarnchana, O. (2007). Nanostructures in environmental pollution detection, monitoring, and remediation. Sci. Technol. Adv. Mat. 8, 47–59. doi:10.1016/j.stam.2006.11.003
Wang, L., Kaeppler, A., Fischer, D., and Simmchen, J. (2019). Photocatalytic TiO2 micromotors for removal of microplastics and suspended matter. ACS Appl. Mater. interfaces 11 (36), 32937–32944. doi:10.1021/acsami.9b06128
Waqas, M., Wong, M. S., Stocchino, A., Abbas, S., Hafeez, S., and Zhu, R. (2023). Marine plastic pollution detection and identification by using remote sensing-meta analysis. Mar. Pollut. Bull. 197, 115746. doi:10.1016/j.marpolbul.2023.115746
Wójcik-Długoborska, K. A., Osińska, M., and Bialik, R. J. (2022). The impact of glacial suspension color on the relationship between its properties and marine water spectral reflectance. IEEE J. Sel. Top. Appl. Earth Observations Remote Sens. 15, 3258–3268. doi:10.1109/jstars.2022.3166398
Xu, C., Anusuyadevi, P. R., Aymonier, C., Luque, R., and Marre, S. (2019). Nanostructured materials for photocatalysis. Chem. Soc. Rev. 48 (14), 3868–3902. doi:10.1039/c9cs00102f
Xu, Y., Xie, S., Liang, J., Sun, D., Qi, N., and Liu, Y. N. (2020). A novel method to remove interference fringes for hyperspectral SWIR imagers. IEEE Trans. Geosci. Remote Sens. 58, 7580–7588. doi:10.1109/TGRS.2020.2981640
Zhang, L., and Zhang, L. (2022). Artificial intelligence for remote sensing data analysis: a review of challenges and opportunities. IEEE Geoscience Remote Sens. Mag. 10 (2), 270–294. doi:10.1109/mgrs.2022.3145854
Zhang, S., Wei, S., Liu, Z., Li, T., Li, C., Huang, X. L., et al. (2022). The rise of AI optoelectronic sensors: from nanomaterial synthesis, device design to practical application. Mat. Today Phys. 27, 100812. doi:10.1016/j.mtphys.2022.100812
Keywords: environmental pollution, satellite remote sensing, nanomaterials, environmental sensing, environmental remediation, water and snow quality
Citation: Ferral A, Bonansea M, Scavuzzo CM, Nemiña F, Burgos Paci M, Ramirez JC, Sepúlveda B, Fraxedas J and Esplandiu MJ (2024) Bringing satellite and nanotechnologies together: unifying strengths against pollution and climate change. Front. Nanotechnol. 6:1332820. doi: 10.3389/fnano.2024.1332820
Received: 03 November 2023; Accepted: 12 March 2024;
Published: 16 April 2024.
Edited by:
Giancarlo Franzese, University of Barcelona, SpainReviewed by:
Sandra Torrusio, National University of La Plata, ArgentinaCopyright © 2024 Ferral, Bonansea, Scavuzzo, Nemiña, Burgos Paci, Ramirez, Sepúlveda, Fraxedas and Esplandiu. This is an open-access article distributed under the terms of the Creative Commons Attribution License (CC BY). The use, distribution or reproduction in other forums is permitted, provided the original author(s) and the copyright owner(s) are credited and that the original publication in this journal is cited, in accordance with accepted academic practice. No use, distribution or reproduction is permitted which does not comply with these terms.
*Correspondence: Maria Jose Esplandiu, bWFyaWFqb3NlLmVzcGxhbmRpdUBpY24yLmNhdA==