- Functional Atomic Force Microscope Group, Center for Nanophase Materials Sciences, Oak Ridge National Laboratory, Oak Ridge, TN, United States
The last decade has witnessed the emergence of the application of piezoelectric and ferroelectric materials for catalytic and photocatalytic applications that harness light, thermal and mechanical energy into chemical reactions. This article surveys the different concepts of pyro- and piezocatalysis and differences with respect to ferrocatalysis and switchable catalysis and delves into the current understanding of the mechanisms underlying piezocatalysis. The outlook for advancing in the surface science studies required for the design of new and better catalysts based on polar electromechanically active materials is discussed in the context of the state of the art experimental studies and potential future nanoscience developments.
1 Introduction
Catalysis, a multidisciplinary field by nature covering areas from physics to chemistry and biology and at the base of the chemical industry, uses catalysts as materials to intermediate chemical reactions that lead to the final reaction product through lower energetic pathways. In photogenerated catalysis, the photocatalytic activity of a material depends on the ability of the catalyst to create electron/hole pairs upon light absorption to accelerate desired reactions. A primary practical application is based on the discovery of photocatalytic water splitting by titanium dioxide (TiO2), a process of artificial photosynthesis that generates hydrogen (and oxygen) from water and light (Fujishima and Honda, 1972; Man, Su et al., 2011; Zhao and Liu, 2014; Lampimäki, Schreiber et al., 2015). The problem of photocatalysts is that they must meet restrictive bandgap requirements, and typically need dopants and/or additional co-catalysts to optimize their performance. Moreover, heterogeneous catalysis on surfaces is highly selective, since the catalysts efficiency is limited by the Sabatier principle which balances the catalytic efficiency against the optimal strength of the adsorbate-surface interaction (Medford, Vojvodic et al., 2015) and available active sites, and thus its optimization for each chemical reaction requires a dedicated research effort.
While photocatalysis is a straightforward green methodology to convert light to chemical energy, there are other possible sources of charge to be exploited to harvest green energy for catalysis applications. In this regard, electromechanically active oxide perovskites are emerging as one of the most promising material systems to open new opportunities in the area of catalysis (Kim, Nguyen et al., 2018; Li, Li et al., 2020; X.-K. Wei, Domingo et al., 2022). In this class of materials, the polarization is coupled to strain and temperature fields therefore making it possible to create polarization by means of mechanical and thermal energy. In this mini review, we will sketch the state of the art in the understanding of the fundamental mechanisms of ferrocatalytisis, piezocatalysis and pyrocatalysis and discuss the current research gaps and potential future developments in the field.
2 Polarization assisted catalysis
Polarization assisted catalysis is an emerging catalysis field that embraces the use of pyroelectric, piezoelectric and ferroelectric materials to harvest light, thermal and mechanical energy into chemical reactions. These different concepts sketched in Figure 1 have been coined in the last decade to refer to different mechanisms in which these polar and electromechanical active materials are observed to enhance catalytic efficiency in different type of redox reactions. Piezoelectric materials are generally non-centrosymmetric crystals that can create electrical dipoles as a response to an applied mechanical stress or deformation, leading to the emergence of a voltage drop across the material (also called piezopotential), based on the different charges accumulated on opposite surfaces. These are electromechanical active materials that will also deform under applied electric fields due to the inverse piezoelectric effect. A crystal that poses spontaneous polarization even under unstrained conditions is defined as a pyroelectric material, and its electric polarization changes as a function of temperature. A subclass of pyroelectric materials are ferroelectrics, exhibiting an spontaneous polarization that can be reversed upon the application of an electric field giving rise to ferroelectric hysteresis loops. Both, pyroelectric and ferroelectric materials are also piezoelectric and thus its polarization can be modulated by mechanical stress.
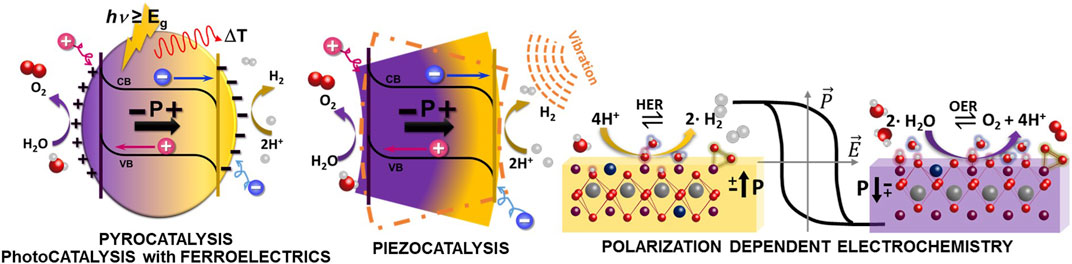
FIGURE 1. Illustration of the different concepts of pyrocatalysis, photocatalysis based on ferroelectric materials and piezocatalysis. The right panel illustrates the selectivity of ferroelectric surfaces to drive oxidation and reduction reactions as a function of the ferroelectric polarization.
2.1 Piezocatalysis and pyrocatalysis
In piezoelectric and pyroelectric materials, the change of its polar state creates a bound charge on the surfaces with stray electric fields that need to be compensated to reach stability, a property that has been exploited to build up piezoelectric nanogenerators by short-circuiting opposite surfaces (Wang and Song, 2006). However, when the polar materials are exposed to ambient, the most relevant screening mechanism is the appearance of surface adsorbates layers composed by different types of ionic species and dipolar chemical groups that compensate the surface stray electric fields. Being a subclass of piezoelectrics, ferroelectric materials are known to show the highest piezoelectric coefficients and also spontaneous stray electric fields that need to be compensated to stabilize the ferroelectric polarization. Thus, similar to photocatalysis, the thermal or mechanical induced change of polarization in any pyroelectric or piezoelectric material can be regarded as a source of potential that can lead to the accumulation of charge at the surfaces available to accelerate catalytic reactions, leading to “pyroelectrocatalysis” (Kakekhani and Ismail-Beigi, 2016; Wu, Mao et al., 2016) and “piezoelectrochemical” effects (Starr and Wang, 2013; Starr and Wang, 2015; Zhang, Xie et al., 2017; Wu, Qin et al., 2018; Liang, Yan et al., 2019; Yu, Liu et al., 2019) respectively. The efficiency of polar materials to implement pyrocatalysis and piezocatalysis will strongly depend on the balance between the polarization change (instantaneous upon the change of temperature and strain), the screening dynamics and the charge generation rates as compared to the physical chemical features of the reactions to catalyze.
However, the piezopotentials and pyroelectric potential differences created by thermal and mechanical changes in dielectric piezoelectrics cannot supply enough charges for efficient catalytic processes on their own, so most of the times practical applications are based on semiconductor piezoelectrics [and dopped ferroelectric (Dubey, Keat et al., 2022)] materials that can provide free and photoinduced carriers to enhance the catalytic reactivity (Mushtaq, Chen et al., 2018). In this case, the internal generated electric fields, similar to the concept of piezotronics and piezo-phototronics, are expected to regulate charge carrier transport behavior and force the free charges to move towards opposite directions, inhibiting the recombination of photocharge carriers and enabling more holes and electrons to reach the active sites on opposite surfaces of the catalysts for the redox reaction.
2.2 Ferrocatalysis and switchable catalysis
Ferroelectric materials are also known as wide band gap semiconductors and show a permanent internal polarization that can be rotated through the application of an electric field. This polarization leads to a discontinuity on the electric fields at their surface that needs to be screened to ensure their stability (Sergei, Yunseok et al., 2018). Archetypal ferroelectrics show polarization values in the range of P ϵ (10-70) μC·cm−2, leading to stray electric fields of the order of E = 1·e10 V/m on the surface (∼1.1 V at 1 nm above the surface for p = 10 μC cm−2). The most efficient way to cancel the electric field is the accumulation of equal amounts of compensating opposite charges, that would be the charge equivalent to about 1.5 electrons/nm2 for a ferroelectric material with p = 25 μC cm-2 like BaTiO3 (∼2.8V nm−1, enough field for water splitting). This can be achieved by internal screening mechanisms such as band bending near the surface to accumulate electrons, holes or ionic charges as oxygen vacancies, or by the adsorption of external species. In UHV, the adsorbates are species found in the bulk such as oxygen anions while in ambient conditions the range of available species is much wider. Among all the external adsorbates, water molecules either physisorbed or dissociated play the major role (Segura, Domingo et al., 2013; Cordero-Edwards, Rodríguez et al., 2016), since as a polar molecule is sensitive to the stray electric fields and can dissociate on the surface forming hydroxyls or providing ionic charges. Other known active species include carbonated organic molecules, with different carboxylic groups and Si oxides, or other salts formed from ions contained in the material. So far, the race towards understanding the interplay between ferroelectricity and surface electrochemistry still faces crucial unresolved issues, since at the nanoscale the ferroelectric state is fundamentally inseparable from the electrochemical state of the surface (Yang, Morozovska et al., 2017) and determines ferroelectric switching dynamics (Spasojevic, Verdaguer et al., 2022). This coupling has strong impact on the application of ferroelectric materials in nanodevices such as Tunnel Junctions (Lu, Lipatov et al., 2014; Guo, Wang et al., 2017) since it can create an imprint in the ferroelectric state (Lee, Kim et al., 2016) or alter associated fundamental physical properties such as tunnelling eletroresistance (Gruverman, Wu et al., 2009). On the other hand, it also opens the door to chemically control the ferroelectric polarization (Wang, Fong et al., 2009).
Finally, the family of ferroelectric materials has been targeted as a new generation of exciting heterogeneous catalysts for (electro)chemical reactions involving OER (Hong, Risch et al., 2015; Hwang, Rao Reshma et al., 2017; Wei, Rao et al., 2019; Sun, Alonso et al., 2021; Wang, Adiga et al., 2021; Zhang, Jeerh et al., 2021), and HER since i) some recent works demonstrate the feasibility to use strain to tune the electroactive sites in oxide perovskites for applications in electrocatalysis (Hwang, Feng et al., 2019) and ii) polar materials are known to show specific electrochemical reactivity of their surfaces as a function of the ferroelectric polarization, as depicted in the right panel of Figure 1 (Kalinin, Bonnell et al., 2004; Cordero-Edwards, Rodríguez et al., 2016; Li, Zhao et al., 2017; Domingo, Gaponenko et al., 2019; Tian, Wang et al., 2022). Thus, polarization becomes a switch to control surface chemical activity since the polarization direction (out of plane vs. in plane) modifies the surface strain and the polarization sense (or polarity, changing between up or down) can selectively change the reactions between oxidation and reduction. In this regard, catalysis based on ferroelectrics promises a controllable chemical reaction with boosted efficiency (Wan, Ge et al., 2021). However, the study of catalytic processes on polar surfaces is challenging, and therefore only a few ab initio and DFT studies of FE surface chemistry are available (Geneste and Dkhil, 2009; Rakotovelo, Moussounda et al., 2009; Sanna, Hölscher et al., 2012; Li, Wang et al., 2014; Tymińska, Wu et al., 2017). A direct consequence of the tunable surface electrochemistry of polar materials is the fact that polarization becomes a switch to adjust surface catalytic properties, thus opening a door to design and implement catalytic loops enabled by alternating two polarization states, overcoming the Sabatier principle. These catalytic loops could drive reactions that are otherwise impossible to achieve using a fixed substrate. Even though the exploitation of polar materials for switchable surface chemistry has recently been theoretically proposed (Kakekhani and Ismail-Beigi, 2015; Kakekhani, Ismail-Beigi et al., 2016; Efe, Spaldin et al., 2021), there has been no direct experimental verification. Ferroelectric materials, as a subgroup of piezoelectric and pyroelectric materials show spontaneous electric polarization that can be rotated by the application of electric fields but these requires the use of electrodes on the surfaces. It is important to note that since polarization is coupled to strain and temperature fields, it is possible to create polarization by means of mechanical and thermal energy, but these fields cannot switch polarisation between two different ferroelectric states as required for switchable surface chemistry.
2.3 Flexoelectricity to harness mechanical energy
A completely new approach as a renewable source of electrons is the mechanical energy obtained from environmental noise or friction by movement (triboelectricity). In this sense, “piezotronics” has recently emerged as a promising pragmatic area for low power consumption devices, and was proposed as a mechanism to engineer photoelectrochemical (PEC) cells (Yu and Wang, 2018). It exploits the polarization induced in piezoelectric materials under applied forces and thus it is limited to the use of non-centrosymmetric materials. Opposite to this, flexoelectricity is a universal property of materials whereby they generate a polarization and associated (free and structural) charge displacement when subjected to an inhomogeneous deformation such as bending, creating strain gradients. Moreover, the ubiquitous presence of strain gradients compels us to include flexoelectric effects in any serious attempt to understand the fundamental underlying mechanisms behind any mechanical-to-chemical energy conversion process. Flexoelectric effects are expected to be favored in ferroelectric materials due to their high dielectric permittivity, but we have demonstrated that they can also be surprisingly huge in oxide semiconductors with metal electrodes (Narvaez, 2016; Narvaez, Vasquez-Sancho et al., 2016), paving the way to exploit flexoelectricity at the macroscale by the design of the proper heterostructures and interface engineering. Of importance, flexoelectricity strongly couples to ferroelectricity leading to some of the most recent advances in nanoscale ferroelectrics such as the demonstration of flexoelectricity as an effective field for ferroelectric polarization switching (Lu, Bark et al., 2012; Bhaskar, Banerjee et al., 2016).
Recently, there has been a growing interest on the application of 2D and low dimensional materials for catalytic applications due to its huge surface to volume ratio (Wei, Domingo et al., 2022). While most of these materials are centrosymmetric, its characteristic geometry is expected to enable the appearance of huge strain gradients and eventually significant flexoelectric fields. In this regard, some recent studies of flexocatalysis in 2D centrosymmetric semiconductors already demonstrate the efficiency of this materials to drive redox reactions under ultrasonic stimulation (Wu, Liu et al., 2023).
3 Discussion
3.1 Enhanced efficiency of piezo and ferroelectric materials in catalytic applications
In the last few years, there has been an explosion of experimental studies on the enhanced efficiency of piezoelectric and ferroelectric materials for catalytic applications, mainly in the field of environmental remediation (e.g., organic pollutant decontamination) (Liu, Qi et al., 2023), but also for energy production (Zhang, Xie et al., 2017) from water splitting and CO2 reduction and materials synthesis (selective growth/deposition and organic synthesis) (Ren, Peng et al., 2023). In all cases, the catalysts are nanoparticles, nanowires or nanoplates/nanosheets and the catalytic activity is accelerated by the use of light and sonication, which is expected to maximize photocatalytic carriers and create polarization induced internal electric fields (piezopotential changes) within the material that play a crucial role in charge separation and transport of free and photo-carriers in these materials. Similar effects are also observed in dye degradation when the solution is subjected to thermal cycling in pyrocatalytic applications (Singh, Sharma et al., 2023). However, while all these experimental works demonstrate the enhanced catalytic efficiency of such combinations in unison, the mechanisms behind piezocatalysis are still under debate and there is a lack of fundamental studies to understand the coupling of induced bulk electric fields and chemical reactions at the interface. Moreover, in most of the cases, the mechanism by which sonication induces piezopotentials in the nanoparticles is speculative and based on simulations. Finally, there is also a sever lack of knowledge of distribution of the different redox active sites within the nanocatalysts, also associated with low understanding of the ferroelectric domain structure of the nanoparticles and nanosheets.
3.2 Energy band theory vs. screening charge effect
Considering that piezoelectric and ferroelectric materials under strain changes transform mechanical energy into a piezopotential, one can find two distinguishable mechanisms in the literature on the piezopotential coupling to catalysis (Wang, Han et al., 2022; Meng, Liu et al., 2023), with its main features summarized in Table 1. The first one, called the “energy band theory” is inspired by photocatalysis, and identifies the piezopotential as a gate to initiate the reaction by adjusting the band structure and controlling the internal charge carrier flow to the catalyst surface to allow the reaction. In this case, the catalytical process uses the material internal charges and the band level determines the catalytic capacity. By contrast, another mechanism called “screening charge effect” can be considered, which emphasizes the critical role of the piezopotential on generating external screening charges in the form of external surface charge adsorbates from external molecules. In this case, the piezopotential should exceed the required Gibbs free energy change to trigger a specific reaction (i.e., reaching the redox potential for the reaction) (Su, Wang et al., 2021) determining the catalytic activity very similar to the process of electrolysis. Also, now the charges participating in the redox reaction are surface adsorbates rather than internal carriers from the material (i.e., there is no charge transfer through the interface) (Dubey, Keat et al., 2022). While experimental works have been able to identify specific chemical compounds participating the overall chemical pathway of the reaction (by using specific blocking agents limiting the catalytic performance), the origin of the involved charge is not yet reasonably explained. Moreover, in some aspects both theories are contradictory, since in the second case the dynamic screening behavior is beneficial for piezocatalyis while the former demonstrates that capacitive current induced by screening phenomena would lead to adverse effects for piezocatalysis.
3.3 Ferroelectric structure at the nanoscale
One of the key elements for the understanding of the role of ferroelectric materials in piezocatalytic and photocatalytic applications is the ferroelectric domain structure of the nanocatalysts units. The most simple approach is to consider that the nanostructures are single crystal domains with a uniform polarization. However, ferroelectric polarization is extremely sensitive to boundary conditions and size effects, and the stability of a single domain can be strongly compromised at the nanoscale in single particles or nanosheets, where the surface to volume ratio is maximized and the strain cannot be tunned with a substrate. Moreover, some theoretical studies of ferroelectric nanoparticle domain configuration predict the formation of complex topological structures such as hopfions (Luk’yanchuk, Tikhonov et al., 2020). In this regard, it is important to take into account the local ferroelectric nanostructure, including the presence of domain walls and polarization rotation effects, morphotropic phase boundaries and defects manipulation and configuration (Meng, Liu et al., 2023). Functional studies of individual nanoscale entities are scarce and most of the times fall in the limit of spatial resolution. The most common study is based on structural spectroscopies or the change of work function between dark and illumination conditions that points to the appearance of a piezopotential due to photocharge separation. Beyond that, piezoelectric force microscopy and ferroelectric switching spectroscopy measurements are in general doubtful and cannot be extrapolated to the behavior of a single nanoparticles in the solution.
3.4 The effect of mechanical stimulation methods
There are several different mechanical stimulation methods available for catalytic processes, roughly divided into high-frequency ultrasonication, low-frequency shear force or mechanical bending. While the efficiency is typically maximized by ultrasonication at frequencies around 40 kHz, practical implementation of this methodology at the industrial scale is hindered by its high energy input. In these cases, the applied sonication frequency can maximize the piezopotential when it matches the resonance frequency of the nanostructure (You, Wu et al., 2019).
However, the piezoelectric effect in non-centrosymmetric crystalline materials is based on the piezoelectric tensor that correlates the application of a directional strain with the appearance of an electric field. Ultrasonic vibrations create intense pressure by the collapse of cavitation bubbles deforming the catalysts via a periodic external strain. Still, the effect of every single nanocatalytic unit is divers and there are only theoretical simulations available on the predicted piezopotential obtained. In this regard, strain gradients and associated flexoelectric effects cannot be neglected and should be included in the study of piezocatalysis.
Finally, the sonication processes are also known to accelerate the chemical reactions by maximizing the interaction time between reactants (in this case, the external molecules and the piezocatalysts), so it can have an important role in breaking the capacitive double layer and preventing the stabilization of screening charge at the surface of the piezoelectric and ferroelectric materials, thus enhancing the surface reactivity.
3.5 Research gaps and future challenges
Advances for the control and design of better piezocatalysts urgently require a deeper understanding of the underlying mechanism that should take into account the different time scales of all the processes involved in the two cases. In this regard, while the formation of piezoelectric potential and photocarriers can be considered instantaneous, the time constants for the mobility of internal carriers, the activation of different internal and external screening mechanisms, the stabilization of capacitive double layers at the surface of the catalysts and the chemical reaction times should be carefully balanced. In order to delve into a better knowledge of the coupling between internal electric fields and surface chemical reactions it is urgent to develop new characterization tools that allow fundamental studies of this coupling with nanoscale resolution, to correlate functional properties with chemical composition and reaction dynamics. To this end, it is important to control the ferroelectric state of the surface and isolate and identify the active sites of reduction and oxidation paths of the reactions and their local correlation with the polarization, as well as the size effect of the nanostructures on the ferroelectric and piezoelectric configuration. Up to now, pollutant decontamination has probably been the straightforward benchmarking application due to its direct correlation with reduction and oxidation reactions. However, its efficiency for market applications for CO2 reduction or water splitting are still to be demonstrated. Nonetheless, the singular role of piezoelectric and ferroelectric materials in this field is that they open a new paradigm by coupling the chemical reactions with bulk materials functional properties and new ways of energy harvesting. So, while there are evidences of their ability to reduce CO2 and water splitting, more fundamental research needs to be driven in order to enhance their efficiency and make it competitive enough. Only with a better understanding of the associated surface science underlying piezocatalytic and ferrocatalytic processes it will be possible to advance in the prediction, design and improvement of nanomaterials for such applications as well as future advances in biomedical applications (Chen, Zhu et al., 2023; Wang, Zhang et al., 2023).
Author contributions
ND: Conceptualization, Formal Analysis, Investigation, Resources, Validation, Visualization, Writing–original draft.
Funding
The author(s) declare financial support was received for the research, authorship, and/or publication of this article. This work was supported by Center for Nanophase Materials Sciences (CNMS), which is a U.S. Department of Energy, Office of Science User Facility at Oak Ridge National Laboratory.
Licenses and permissions
This manuscript has been authored by UT-Battelle, LLC, under Contract No. DEAC0500OR22725 with the U.S. Department of Energy. The United States Government retains and the publisher, by accepting the article for publication, acknowledges that the United States Government retains a non-exclusive, paid-up, irrevocable, worldwide license to publish or reproduce the published form of this manuscript, or allow others to do so, for the United States Government purposes.
Conflict of interest
The author ND is an employee of Oak Ridge National Laboratory, managed by UT-Battelle LLC, an M&O contractor for the U.S. Department of Energy.
Publisher’s note
All claims expressed in this article are solely those of the authors and do not necessarily represent those of their affiliated organizations, or those of the publisher, the editors and the reviewers. Any product that may be evaluated in this article, or claim that may be made by its manufacturer, is not guaranteed or endorsed by the publisher.
References
Bhaskar, U. K., Banerjee, N., Abdollahi, A., Wang, Z., Schlom, D. G., Rijnders, G., et al. (2016). A flexoelectric microelectromechanical system on silicon. Nat. Nanotechnol. 11 (3), 263–266. doi:10.1038/nnano.2015.260
Chen, S., Zhu, P., Mao, L., Wu, W., Lin, H., Xu, D., et al. (2023). Piezocatalytic medicine: an emerging frontier using piezoelectric materials for biomedical applications. Adv. Mater. 35 (25), 2208256. doi:10.1002/adma.202208256
Cordero-Edwards, K., Rodríguez, L., Calò, A., Esplandiu, M. J., Pérez-Dieste, V., Escudero, C., et al. (2016). Water affinity and surface charging at the z-cut and y-cut LiNbO3 surfaces: an ambient pressure X-ray photoelectron spectroscopy study. J. Phys. Chem. C 120 (42), 24048–24055. doi:10.1021/acs.jpcc.6b05465
Domingo, N., Gaponenko, I., Stucki, N., Cordero-Edwards, K., Pérez-Dieste, V., Escudero, C., et al. (2019). Surface charged species and electrochemistry of ferroelectric thin films. Nanoscale 11, 17920–17930. doi:10.1039/c9nr05526f
Dubey, A., Keat, C. H., Shvartsman, V. V., Yusenko, K. V., Castillo, M. E., Buzanich, A. G., et al. (2022). Mono-Di-and tri-valent cation doped BiFe0.95Mn0.05O3 nanoparticles: ferroelectric photocatalysts. Adv. Funct. Mater. 32 (43), 2207105. doi:10.1002/adfm.202207105
Efe, I., Spaldin, N. A., and Gattinoni, C. (2021). On the happiness of ferroelectric surfaces and its role in water dissociation: the example of bismuth ferrite. J. Chem. Phys. 154 (2), 024702. doi:10.1063/5.0033897
Fujishima, A., and Honda, K. (1972). Electrochemical photolysis of water at a semiconductor electrode. Nature 238, 37–38. doi:10.1038/238037a0
Geneste, G., and Dkhil, B. (2009). Adsorption and dissociation of H2O on in-plane-polarized BaTiO3 (001) surfaces and their relation to ferroelectricity. Phys. Rev. B 79 (23), 235420. doi:10.1103/physrevb.79.235420
Gruverman, A., Wu, D., Lu, H., Wang, Y., Jang, H. W., Folkman, C. M., et al. (2009). Tunneling electroresistance effect in ferroelectric tunnel junctions at the nanoscale. Nano Lett. 9 (10), 3539–3543. doi:10.1021/nl901754t
Guo, R., Wang, Y., Yoong, H. Y., Chai, J., Wang, H., Lin, W., et al. (2017). Effect of extrinsically introduced passive interface layer on the performance of ferroelectric tunnel junctions. ACS Appl. Mater. Interfaces 9 (6), 5050–5055. doi:10.1021/acsami.6b15564
Hong, W. T., Risch, M., Stoerzinger, K. A., Grimaud, A., Suntivich, J., and Shao-Horn, Y. (2015). Toward the rational design of non-precious transition metal oxides for oxygen electrocatalysis. Energy & Environ. Sci. 8 (5), 1404–1427. doi:10.1039/c4ee03869j
Hwang, J., Feng, Z., Charles, N., Wang, X. R., Lee, D., Stoerzinger, K. A., et al. (2019). Tuning perovskite oxides by strain: electronic structure, properties, and functions in (electro)catalysis and ferroelectricity. Mater. Today 31, 100–118. doi:10.1016/j.mattod.2019.03.014
Hwang, J., Rao Reshma, R., Giordano, L., Katayama, Y., Yu, Y., and Shao-Horn, Y. (2017). Perovskites in catalysis and electrocatalysis. Science 358 (6364), 751–756. doi:10.1126/science.aam7092
Kakekhani, A., and Ismail-Beigi, S. (2015). Ferroelectric-based catalysis: switchable surface chemistry. ACS Catal. 5 (8), 4537–4545. doi:10.1021/acscatal.5b00507
Kakekhani, A., and Ismail-Beigi, S. (2016). Ferroelectric oxide surface chemistry: water splitting via pyroelectricity. J. Mater. Chem. A 4 (14), 5235–5246. doi:10.1039/c6ta00513f
Kakekhani, A., Ismail-Beigi, S., and Altman, E. I. (2016). Ferroelectrics: a pathway to switchable surface chemistry and catalysis. Surf. Sci. 650, 302–316. doi:10.1016/j.susc.2015.10.055
Kalinin, S. V., Bonnell, D. A., Alvarez, T., Lei, X., Hu, Z., Shao, R., et al. (2004). Ferroelectric lithography of multicomponent nanostructures. Adv. Mater. 16 (9-10), 795–799. doi:10.1002/adma.200305702
Kim, S., Nguyen, N., and Bark, C. (2018). Ferroelectric materials: a novel pathway for efficient solar water splitting. Appl. Sci. 8 (9), 1526. doi:10.3390/app8091526
Lampimäki, M., Schreiber, S., Zelenay, V., Křepelová, A., Birrer, M., Axnanda, S., et al. (2015). Exploring the environmental photochemistry on the TiO2(110) surface in situ by near ambient pressure X-ray photoelectron spectroscopy. J. Phys. Chem. C 119 (13), 7076–7085. doi:10.1021/jp511340n
Lee, H., Kim, T. H., Patzner, J. J., Lu, H., Lee, J.-W., Zhou, H., et al. (2016). Imprint control of BaTiO3 thin films via chemically induced surface polarization pinning. Nano Lett. 16 (4), 2400–2406. doi:10.1021/acs.nanolett.5b05188
Li, R., Zhao, Y., and Li, C. (2017). Spatial distribution of active sites on a ferroelectric PbTiO3 photocatalyst for photocatalytic hydrogen production. Faraday Discuss. 198 (0), 463–472. doi:10.1039/c6fd00199h
Li, X., Wang, B., Zhang, T.-Y., and Su, Y. (2014). Water adsorption and dissociation on BaTiO3 single-crystal surfaces. J. Phys. Chem. C 118 (29), 15910–15918. doi:10.1021/jp5051386
Li, Y., Li, J., Yang, W., and Wang, X. (2020). Implementation of ferroelectric materials in photocatalytic and photoelectrochemical water splitting. Nanoscale Horizons 5 (8), 1174–1187. doi:10.1039/d0nh00219d
Liang, Z., Yan, C.-F., Rtimi, S., and Bandara, J. (2019). Piezoelectric materials for catalytic/photocatalytic removal of pollutants: recent advances and outlook. Appl. Catal. B Environ. 241, 256–269. doi:10.1016/j.apcatb.2018.09.028
Liu, J., Qi, W., Xu, M., Thomas, T., Liu, S., and Yang, M. (2023). Piezocatalytic techniques in environmental remediation. Angew. Chem. Int. Ed. 62 (5), e202213927. doi:10.1002/anie.202213927
Lu, H., Bark, C. W., Esque de los Ojos, D., Alcala, J., Eom, C. B., Catalan, G., et al. (2012). Mechanical writing of ferroelectric polarization. Science 336 (6077), 59–61. doi:10.1126/science.1218693
Lu, H., Lipatov, A., Ryu, S., Kim, D. J., Lee, H., Zhuravlev, M. Y., et al. (2014). Ferroelectric tunnel junctions with graphene electrodes. Nat. Commun. 5, 5518. doi:10.1038/ncomms6518
Luk’yanchuk, I., Tikhonov, Y., Razumnaya, A., and Vinokur, V. M. (2020). Hopfions emerge in ferroelectrics. Nat. Commun. 11 (1), 2433. doi:10.1038/s41467-020-16258-w
Man, I. C., Su, H.-Y., Calle-Vallejo, F., Hansen, H. A., Martínez, J. I., Inoglu, N. G., et al. (2011). Universality in oxygen evolution electrocatalysis on oxide surfaces. ChemCatChem 3 (7), 1159–1165. doi:10.1002/cctc.201000397
Medford, A. J., Vojvodic, A., Hummelshøj, J. S., Voss, J., Abild-Pedersen, F., Studt, F., et al. (2015). From the Sabatier principle to a predictive theory of transition-metal heterogeneous catalysis. J. Catal. 328, 36–42. doi:10.1016/j.jcat.2014.12.033
Meng, N., Liu, W., Jiang, R., Zhang, Y., Dunn, S., Wu, J., et al. (2023). Fundamentals, advances and perspectives of piezocatalysis: a marriage of solid-state physics and catalytic chemistry. Prog. Mater. Sci. 138, 101161. doi:10.1016/j.pmatsci.2023.101161
Mushtaq, F., Chen, X., Hoop, M., Torlakcik, H., Pellicer, E., Sort, J., et al. (2018). Piezoelectrically enhanced photocatalysis with BiFeO3 nanostructures for efficient water remediation. iScience 4, 236–246. doi:10.1016/j.isci.2018.06.003
Narvaez, J. (2016). Bellaterra, Spain. Universitat Autònoma de Barcelona, Flexoelectricity in single crystals
Narvaez, J., Vasquez-Sancho, F., and Catalan, G. (2016). Enhanced flexoelectric-like response in oxide semiconductors. Nature 538 (7624), 219–221. doi:10.1038/nature19761
Rakotovelo, G., Moussounda, P. S., Haroun, M. F., Légaré, P., Rakotomahevitra, A., Rakotomalala, M., et al. (2009). Adsorption of CO, CO2 and NO molecules on a BaTiO3 (001) surface. Surf. Sci. 603 (9), 1221–1228. doi:10.1016/j.susc.2009.03.006
Ren, Z., Peng, Y., He, H., Ding, C., Wang, J., Wang, Z., et al. (2023). Piezoelectrically mediated reactions: from catalytic reactions to organic transformations. Chin. J. Chem. 41 (1), 111–128. doi:10.1002/cjoc.202200443
Sanna, S., Hölscher, R., and Schmidt, W. G. (2012). Polarization-dependent water adsorption on the LiNbO3 (0001) surface. Phys. Rev. B 86 (20), 205407. doi:10.1103/physrevb.86.205407
Segura, J. J., Domingo, N., Fraxedas, J., and Verdaguer, A. (2013). Surface screening of written ferroelectric domains in ambient conditions. J. Appl. Phys. 113 (18), 187213. doi:10.1063/1.4801983
Sergei, V. K., Yunseok, K., Dillon, D. F., and Anna, N. M. (2018). Surface-screening mechanisms in ferroelectric thin films and their effect on polarization dynamics and domain structures. Rep. Prog. Phys. 81 (3), 036502. doi:10.1088/1361-6633/aa915a
Singh, G., Sharma, M., Sharma, J. D., Kumar, S., and Vaish, R. (2023). Ferroelectric ceramics for pyrocatalytic applications. Prog. Solid State Chem. 72, 100428. doi:10.1016/j.progsolidstchem.2023.100428
Spasojevic, I., Verdaguer, A., Catalan, G., and Domingo, N. (2022). Effect of humidity on the writing speed and domain wall dynamics of ferroelectric domains. Adv. Electron. Mater. 8 (6), 2100650. doi:10.1002/aelm.202100650
Starr, M. B., and Wang, X. (2013). Fundamental Analysis of piezocatalysis process on the surfaces of strained piezoelectric materials. Sci. Rep. 3, 2160. doi:10.1038/srep02160
Starr, M. B., and Wang, X. (2015). Coupling of piezoelectric effect with electrochemical processes. Nano Energy 14, 296–311. doi:10.1016/j.nanoen.2015.01.035
Su, R., Wang, Z., Zhu, L., Pan, Y., Zhang, D., Wen, H., et al. (2021). Strain-engineered nano-ferroelectrics for high-efficiency piezocatalytic overall water splitting. Angew. Chem. Int. Ed. 60 (29), 16019–16026. doi:10.1002/anie.202103112
Sun, C., Alonso, J. A., and Bian, J. (2021). Recent advances in perovskite-type oxides for energy conversion and storage applications. Adv. Energy Mater. 11 (2). doi:10.1002/aenm.202000459
Tian, Y., Wang, A., Wei, Y., Pei, M., Cao, R., Gu, Z., et al. (2022). Large-area printing of ferroelectric surface and super-domain for solar water splitting. Adv. Funct. Mater. 32, 2111180. doi:10.1002/adfm.202111180
Tymińska, N., Wu, G., and Dupuis, M. (2017). Water oxidation on oxygen-deficient barium titanate: a first-principles study. J. Phys. Chem. C 121 (15), 8378–8389. doi:10.1021/acs.jpcc.6b12425
Wan, T. L., Ge, L., Pan, Y., Yuan, Q., Liu, L., Sarina, S., et al. (2021). Catalysis based on ferroelectrics: controllable chemical reaction with boosted efficiency. Nanoscale 13 (15), 7096–7107. doi:10.1039/d1nr00847a
Wang, K., Han, C., Li, J., Qiu, J., Sunarso, J., and Liu, S. (2022). The mechanism of piezocatalysis: energy band theory or screening charge effect? Angew. Chem. Int. Ed. 61 (6), e202110429. doi:10.1002/anie.202110429
Wang, L., Adiga, P., Zhao, J., Samarakoon, W. S., Stoerzinger, K. A., Spurgeon, S. R., et al. (2021). Understanding the electronic structure evolution of epitaxial LaNi1–xFexO3 thin films for water oxidation. Nano Lett. 21 (19), 8324–8331. doi:10.1021/acs.nanolett.1c02901
Wang, L., Zhang, S., Zhang, Y., and An, Q. (2023). Piezodynamic therapy: mechanisms and biomedical applications. Nano Energy 110, 108342. doi:10.1016/j.nanoen.2023.108342
Wang, R. V., Fong, D. D., Jiang, F., Highland, M. J., Fuoss, P. H., Thompson, C., et al. (2009). Reversible chemical switching of a ferroelectric film. Phys. Rev. Lett. 102 (4), 047601. doi:10.1103/physrevlett.102.047601
Wang, Z. L., and Song, J. (2006). Piezoelectric nanogenerators based on zinc oxide nanowire arrays. Science 312 (5771), 242–246. doi:10.1126/science.1124005
Wei, C., Rao, R. R., Peng, J., Huang, B., Stephens, I. E. L., Risch, M., et al. (2019). Recommended practices and benchmark activity for hydrogen and oxygen electrocatalysis in water splitting and fuel cells. Adv. Mater. 31 (31), 1806296. doi:10.1002/adma.201806296
Wei, X.-K., Domingo, N., Sun, Y., Balke, N., Dunin-Borkowski, R. E., and Mayer, J. (2022). Progress on emerging ferroelectric materials for energy harvesting, storage and conversion. Adv. Energy Mater. 12, 2201199. doi:10.1002/aenm.202201199
Wei, X.-K., Domingo, N., Sun, Y., Balke, N., Dunin-Borkowski, R. E., and Mayer, J. (2022). Progress on emerging ferroelectric materials for energy harvesting, storage and conversion. Adv. Energy Mater. 2022, 01199. doi:10.1002/aenm.202201199
Wu, J., Mao, W., Wu, Z., Xu, X., You, H., Xue, A. X., et al. (2016). Strong pyro-catalysis of pyroelectric BiFeO3 nanoparticles under a room-temperature cold–hot alternation. Nanoscale 8 (13), 7343–7350. doi:10.1039/c6nr00972g
Wu, J., Qin, N., and Bao, D. (2018). Effective enhancement of piezocatalytic activity of BaTiO3 nanowires under ultrasonic vibration. Nano Energy 45, 44–51. doi:10.1016/j.nanoen.2017.12.034
Wu, T., Liu, K., Liu, S., Feng, X., Wang, X., Wang, L., et al. (2023). Highly efficient flexocatalysis of two-dimensional semiconductors. Adv. Mater. 35 (3), 2208121. doi:10.1002/adma.202208121
Yang, S. M., Morozovska, A. N., Kumar, R., Eliseev, E. A., Cao, Y., Mazet, L., et al. (2017). Mixed electrochemical-ferroelectric states in nanoscale ferroelectrics. Nat. Phys. 13, 812–818. doi:10.1038/nphys4103
You, H., Wu, Z., Zhang, L., Ying, Y., Liu, Y., Fei, L., et al. (2019). Harvesting the vibration energy of BiFeO3 nanosheets for hydrogen evolution. Angew. Chem. Int. Ed. 58 (34), 11779–11784. doi:10.1002/anie.201906181
Yu, D., Liu, Z., Zhang, J., Li, S., Zhao, Z., Zhu, L., et al. (2019). Enhanced catalytic performance by multi-field coupling in KNbO3 nanostructures: piezo-photocatalytic and ferro-photoelectrochemical effects. Nano Energy 58, 695–705. doi:10.1016/j.nanoen.2019.01.095
Yu, Y., and Wang, X. (2018). Piezotronics in photo-electrochemistry. Adv. Mater. 30 (43), 1800154. doi:10.1002/adma.201800154
Zhang, M., Jeerh, G., Zou, P., Lan, R., Wang, M., Wang, H., et al. (2021). Recent development of perovskite oxide-based electrocatalysts and their applications in low to intermediate temperature electrochemical devices. Mater. Today 49, 351–377. doi:10.1016/j.mattod.2021.05.004
Zhang, Y., Xie, M., Adamaki, V., Khanbareh, H., and Bowen, C. R. (2017). Control of electro-chemical processes using energy harvesting materials and devices. Chem. Soc. Rev. 46 (24), 7757–7786. doi:10.1039/c7cs00387k
Keywords: ferrocatalysis, ferroelectrics, photocatalysis, piezocatalysis, pyrocatalysis
Citation: Domingo N (2024) Understanding piezocatalysis, pyrocatalysis and ferrocatalysis. Front. Nanotechnol. 6:1320503. doi: 10.3389/fnano.2024.1320503
Received: 12 October 2023; Accepted: 15 February 2024;
Published: 06 March 2024.
Edited by:
Theresa Schoetz, University of Illinois at Urbana-Champaign, United StatesReviewed by:
Svetlozar Ivanov, Technische Universität Ilmenau, GermanyCopyright © 2024 Domingo. This is an open-access article distributed under the terms of the Creative Commons Attribution License (CC BY). The use, distribution or reproduction in other forums is permitted, provided the original author(s) and the copyright owner(s) are credited and that the original publication in this journal is cited, in accordance with accepted academic practice. No use, distribution or reproduction is permitted which does not comply with these terms.
*Correspondence: Neus Domingo, bmRvbWluZ29Ab3JubC5nb3Y=