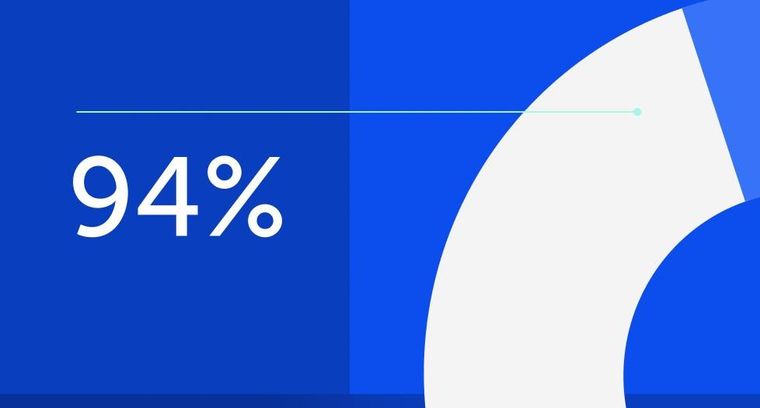
94% of researchers rate our articles as excellent or good
Learn more about the work of our research integrity team to safeguard the quality of each article we publish.
Find out more
REVIEW article
Front. Nanotechnol., 21 July 2023
Sec. Biomedical Nanotechnology
Volume 5 - 2023 | https://doi.org/10.3389/fnano.2023.1186386
This article is part of the Research TopicNanotechnology and Nanoscience to manage SARS-CoV-2 Variants of ConcernView all 14 articles
Violacein is a pigment produced by Gram-negative bacteria, which has shown several beneficial biological activities. The most relevant activities of violacein include the interference in the physiological activities of biological membranes, inhibition of cell proliferation, antioxidant, and anti-inflammatory activities. Moreover, the antiviral activities of violacein against some enveloped and non-enveloped viruses have also been reported. Violacein showed a wide spectrum of protease inhibition, both experimentally and in silico. Other in silico studies have suggested that violacein binds to the SARS-CoV-2 spike. Empirical physicochemical studies indicate that violacein (or, occasionally, its derivatives) may be administered orally to treat different disorders. In addition, different alternatives to product violacein, and molecular devices for delivery of this pigment are reviewed.
In the last five decades, viral infections have negatively affected the world population, mainly because of accelerated globalization and rapid urbanization, which are associated with strong changes in social habits. Many viral infections have spread worldwide through different vectors, such as dengue (DENV), Ebola, Hepatitis B virus, herpes virus, HIV/AIDS, Middle East respiratory syndrome, rotaviruses, and Zika. Recently, the global population has been in peril with the SARS-CoV-2 pandemic. The world death toll of SARS-CoV-2 overpassed 6.8 million people worldwide, and long-term deleterious consequences have been described in some of the 755 billion infected people based on the World Health Organization (WHO) website (WHO, 2023). Different therapeutic strategies have been developed worldwide for the treatment and prevention of SARS-CoV-2 infection. Many old and traditional antiviral drugs were on the battlefront trying to stop the viral pandemic but with poor or disappointing results (Das et al., 2020). The processes to develop SARS-CoV-2 vaccines, get the corresponding approval, and deliver them worldwide took approximately six to 12 months. Although large-scale worldwide vaccination has substantially reduced the death toll, new viral variants still challenge the effectiveness of marketed vaccines making their production more complex. Effective drug treatments are, however, still needed to complement vaccine development, especially for unvaccinated people or groups that may not appropriately respond to vaccination (such as immunocompromised patients), and to cover viral mutations not covered by available vaccines (Mei and Tan, 2021).
Nature provides an enormous number of secondary metabolites with a wide variety of molecular scaffolds produced from different biological kingdoms. Among secondary metabolites, pigments have been used in many applications since the beginning of human society and are presently employed in many industrial areas, such as cosmetics, textiles, foods, and healthcare (Kulandaisamy et al., 2020). Microorganisms are one of the relevant pigment producers because they can be cultured under controlled conditions, have simple nutritional requirements, fast and reproducible growth, are easy to scale up, and provide well-established purification methods that are available in the market; they are also environmentally friendly (Mumtaz et al., 2019). In silico screening is among the modern approaches to find novel drug candidates; in particular, structure-based virtual screening departs from experimental or in silico predicted protein structures to select from large public chemical databases or relatively small in-house libraries, those compounds that are more likely to bind to a known or putative binding site (Rahman et al., 2022). Virtual screening has been extensively applied to find potential therapeutics against COVID-19. For example, using this approach 26 synthetic derivatives of coumarins and quinolines were analyzed by molecular docking and molecular dynamics; among them, six compounds were predicted to possess high binding capacity against SARS CoV-2 main protease (Mpro) (Yañez et al., 2021).
Several microbial secondary metabolites are currently marketed for therapeutic applications that started at the beginning of the last century with penicillin, diverse anthracyclines, mitomycins, etc., and have recently been reviewed (Abdelghani et al., 2021). Since the sanitary SARS-CoV-2 emergency, antiviral applications of many molecules and pigments, and their biological mechanisms have recently been revisited (Azman et al., 2018; Ma et al., 2020; Selim et al., 2021).
Violacein has recently attracted the attention of researchers owing to its wide variety of biological activities. During the last two decades, several reports have described numerous biological activities of this pigment, including immunomodulatory, antimicrobial, antiparasitic, antifungal, anticancer, and antiviral activities (Duran et al., 2021a; Duran et al., 2021b; Duran et al., 2022). The relevance of the antioxidant properties of violacein must be analyzed in the context of COVID-19 pathology, as acute infections of SARS-CoV-2 can produce cell death and long-term neurological pathologies. In general, most viral infections are related to the reduction of antioxidant physiological pathways mainly by the inhibition of Nuclear Factor Erythroid 2 (NRF2), a transcription factor (i.e., leucine zipper), which triggers antioxidant proteins and hampers the NLRP3 inflammasome, which mediates the release of many cytokines (Zhu et al., 2021). Additionally, SARS-CoV-2 induces activation of Nuclear Factor kappa B (NF-κB), promoting inflammation and oxidative stress. The direct consequence of SARS-CoV-2 is the development of elevated levels of inflammation and generalized oxidative processes. These processes are developed by the activation of pro-inflammatory cytokines (i.e., Tumor Necrosis Factor-alpha o TNF-α, IL-1β, and IL-6) produced by macrophages and monocytes, high recruitment of immune and endothelial cells, and platelets (Tay et al., 2020). The NF-κB activation induces high activities of cyclooxygenase 2 (COX2) and NOX2 (NADPH oxidase) responsible for ROS production and mitochondrial oxidative stress. Some of the postulated molecular mechanisms of SARS-CoV-2 infection have recently been reviewed (Chernyak et al., 2020). Besides, COVID-19 is not only considered a respiratory viral disease, but is also associated with endotheliopathy, triggering many molecular markers such as angiopoietin 2 plasminogen activator inhibitor 1 (PAI-1) and Willebrand factor (vWF), among others, and compared to healthy individuals. These factors can be associated not only with fatigue and circulatory diseases (i.e., immune thrombosis and myocardial infarction) but also with neuropsychiatric pathologies (i.e., cognitive disorders and stroke) in acute COVID-19 infections and long-term COVID (Laforge et al., 2020; Fodor et al., 2021; Li et al., 2021). A recent study conducted in Nigeria showed that the levels of glutathione; vitamins A, C, and E; enzymes with antioxidant activities such as superoxide dismutase, catalase, and glutathione peroxidase; and the concentrations of Cu, Mg, Se, and Zn are lower in patients with COVID-19 than in healthy people (Muhammad et al., 2021). Intravenous administration of vitamin C, a well-known antioxidant compound, at high concentrations ameliorates inflammation and oxidative stress in patients with severe COVID-19 (Vollbracht and Kraft, 2022). COVID-19 pathology mediated by inflammation associated with the oxidative stress cascade unquestionably contributes to disease severity. Several trials of potential therapeutic antioxidant molecules to treat SARS-Co-2 infection are in progress in the US (https://clinicaltrials.gov/).
It is of particular interest to analyze the antiviral activities of violacein against viruses since the emergence of SARS-CoV-19 is correlated with the scarce activity of many therapeutic molecules.
The present work endeavors to describe the potential mechanisms and properties of violacein as a potential antiviral agent against SARS-CoV-2. Analyses of the effects of violacein on cellular membranes, and antioxidant, anti-inflammatory, and antiviral activities are overviewed (Figure 1). Studies on protease inhibition and in silico studies have also been summarized. Finally, alternatives for the production and delivery of violacein are discussed.
FIGURE 1. Potential antiviral violacein activities. Example: SARS-Cov-2. Abbreviations: IL-6 (Interleukin 6), CXCL-1 (chemokine ligand 1), TNFR1 (Tumor necrosis factor receptor 1), TLR8 (Toll-like receptor 8), HSV-1 (Herpes simplex virus 1), RV-SA11 (Simian rotavirus SA11), PV-2 (Poliovirus type 2), MHV (Murine hepatitis virus), Huh7 (human hepatocellular carcinoma cell line, which contains complete genome replicon of Hepatitis C virus), RBD:ACE2 (receptor-binding domain to angiotensin converting enzyme 2 [ACE2]), HIV-1-RT (human immunodeficiency virus -1 reverse transcriptase), MMP-2 and MMP-9 (matrix metalloprotease 2 and 9 respectively). Coronavirus COVID-19 structure illustration was created at the Centers for Disease Control and Prevention (CDC). Modified from the CDC, Alissa Eckert, MS; Dan Higgins, MAM (Public Domain).
Violacein is a low molecular weight (MW = 433.41 g/mol) bacterial purple pigment produced mainly by Gram (−) bacteria and has been recently reported (Duran et al., 2021a). Chromobacterium spp. and Janthinobacterium spp. are the most common violacein producers. (Duran et al., 2021b).
The IUPAC formula for violacein is 3-[2-hydroxy-5-(5-hydroxy-1H-indol-3-yl)-1H-pyrrol-3-yl]indol-2-one (C20H13N3O3). From a structural point of view, violacein is a bisindole molecule composed of three heterocycles and a 2-pyrrolidone molecule that links two indoles, an oxindole, and 5-hydroxyindole (Figure 2).
FIGURE 2. Chemical Structure of deoxyviolacein (A) and (B) violacein, adapted with permission from Nelson Durán et al., licensed under CC BY-SA 4.0.
Violacein is a very hydrophobic molecule characterized by a P of 2.7 (PubChem) and Log Po/w = 3.34 (Choi et al., 2020). Violacein is insoluble in aqueous media but is partially soluble in organic solvents such as acetone and dioxane, less soluble in ethanol and propanol, and soluble in ethyl acetate, DMSO, and methanol.
The UV-Visible spectra of violacein showed two maximum peaks at λmax = 577–585 nm depending on the organic solvent, and a UV peak at λmax = 260.3 nm in methanol (Abboud and Arment, 2013).
Violacein biosynthesis involves four genes arranged in a gene cluster vioABCD of 8 kb. The synthesis of violacein involves the condensation of two L-tryptophan molecules at the oxindole position, followed by intramolecular reorganization at the 5-hydroxy-indole ring (1→2 shift) (Duran et al., 2021a).
The physicochemical properties of violacein were analyzed in relation to some typical empirical rules, namely: Lipinski and Veber (which predict whether a drug like compound is likely to be orally bioavailable via passive absorption) (Lipinski et al., 2001; Veber et al., 2002) and Pfizer 3/75 rule (which suggests that a drug candidates in the chemical space of low calculated log P and high topological surface area are not likely to cause significant toxicological effects at total plasma concentrations below 10 μM) (Hughes et al., 2008). Violacein fulfills all of them, thus being a good candidate for oral administration with likely low toxicity (Supplementary Table S1).
The antioxidant activity of violacein was reported for the first time in 1998 and confirmed by several extensive studies by Duran’s research group during the 2000s and recently reviewed (Duran et al., 2021a; Durban et al., 2021b). The presence of several conjugated double bonds and two peaks in the UV-visible spectra suggests a protective effect against visible and UV radiation. The experimental antioxidant activity of violacein has been confirmed by theoretical studies based on an electron density model and spectroscopic analysis (Cao et al., 2007; Jehlička et al., 2015). The estimated ionization potential (IP) of violacein is 146.88 kcal/mol which is about 5% lower than a well-known antioxidant a-tocopherol (IP = 154.90 kcal/mol). The antioxidant activity of violacein has been attributed to the N7-H7 band of 5-hydroxyindole (Cao et al., 2007).
In 2006, violacein antioxidant activity against lipid peroxidation was experimentally evaluated in three models of lipid membranes, including egg and soybean phosphatidylcholine liposomes, and also in rat liver microsomes. Protection of lipid membranes by violacein, either in solution or reconstituted within the liposomes, was evaluated against nitric oxide, 1,1-diphenyl-2-picryl-hydrazyl (DPPH), and ascorbyl radicals in the presence or absence of the biodye showing IC50 values of 21, 30, and 125 μM, respectively (Konzen et al., 2006). It was also observed that reconstitution of violacein into the liposomes enhanced its antioxidant activities. Liposomes in the absence of violacein were used as control. A more recent in vitro study on violacein antioxidant properties evaluated violacein partially purified from the isolate Chromobacterium vaccinii CV5 against common radicals. The IC50 of violacein against DPPH, superoxide (produced from phenazine methosulphate), nitric oxide (produced from sodium nitroprusside), hydrogen peroxide, and hydroxyl (produced from ferrous ammonium sulfate) were 0.87 µM, 0.91 µM, 1.19 µM, 0.86 µM, and 0.85 µM, respectively (Vishnu and Palaniswamy, 2018). The lower in vitro IC50 values reported in this study could be attributed to the partial purification of violacein. In any case, all these results suggested a protective effect mediated by violacein at micromolar or sub-micromolar concentrations against peroxidation produced by radical species.
In another study, the antioxidant activity of violacein produced by C. violaceum wild-type and mutants less- and non-biodye producers (i.e., CV9, CV13, and CV14, respectively) was analyzed (Abboud and Arment, 2013). All C. violaceum strains were UV-irradiated at λ = 253.7 nm with 6,000 μW s-1 cm-2. Non-violacein-pigmented mutants did not grow, whereas the viability of the wild-type strain and violacein hyper-producer mutants was reduced by UV irradiation for 48 h. These results suggest the potential protective effects of Violacein against DNA UV-induced damage. Additionally, catalase activity in non-violacein-producing strains was enhanced compared with the enzymatic activity of violacein hyper-producer C. violaceum strains. This experiment strongly suggested an active scavenging role of violacein against reactive oxidative species (ROS). Violacein has been added to sunscreens to increase its protective effect against potential UV damage (Suryawanshi et al., 2015).
The analgesic, antipyretic, and immunomodulatory effects of violacein produced by the newly isolated C. violaceum ESBV4400 were evaluated in Wistar albino rats and mice (Antonisamy and Ignacimuthu, 2010). The effects of violacein against non-inflammatory and inflammatory pain, anaphylactic reactions and fever were assayed by injecting with ovalbumin, acetic acid, formalin, sheep red cells, and Saccharomyces cerevisiae yeast to create traumatic trials. The harmful effects in the treated animals were countered by using indomethacin, dexamethasone, disodium cromoglycate, naloxone, and morphine as control drugs. In the delayed hypersensitivity and provoked paw anaphylaxis tests employing red blood sheep cells and ovalbumin. Similar results were observed with 10 mg kg-1 dexamethasone paired with 5 mg kg-1 disodium cromoglycate and 40 mg kg-1 violacein, with less than 2% difference. In the case of severe pain induced by acetic acid, the results showed a 93.9% and 78% reduction in writhing using 5 mg kg-1 and 10 mg kg-1 morphine and indomethacin. Similar response was elicited by 40 mg kg-1 violacein. Comparable results were obtained using the formalin assay. Hyperthermia induced by the yeast S. cerevisiae injection in rodents showed normalization of temperature with 150 mg kg-1 paracetamol after 60 min, and a similar response was observed with 20 mg kg-1, and 40 mg kg-1 violacein after 120 min and 60 min, respectively. The authors concluded that violacein exerts an immunosuppressive effect on inflammatory physiological responses through T Cells, antiallergic activity to the anaphylactic Ig E-mediated response, and antipyretic response by inhibiting prostaglandin synthesis.
In another report, the effect of violacein on acute and chronic inflammation in mice (C57BL/6) produced by intraperitoneal injection of 1.0 µg LPS from Escherichia coli 0111:B4 was analyzed (Verinaud et al., 2015). Dendritic cells and their CD80 and CD86 markers treated with LPS and violacein did not show variations compared to control cells. Comparable results were obtained for B- and T-cells, which did not show signs of toxicity. In addition, mice treated with LPS-violacein exhibited a significant decrease in neutrophil infiltration in the peritoneal cavity compared to control mice. In addition, cytokines IL-6 and CXCL-1 were reduced, whereas IL-10 was increased in the serum. An Experimental Autoimmune Encephalomyelitis mouse model was developed by induction with myelin oligodendrocyte glycoprotein (MOG35–55). The authors reported an increase in scurfin protein levels (FoxP3, considered a primary regulator of T regulatory cells (Tregs) in mice treated with violacein.
A cellular study using immune lineages of human peripheral blood mononuclear cells (PBMCs) and two murine macrophage cells (ANA-1 and Raw 264.7, established by retroviral infections) treated with violacein was carried out (Venegas et al., 2019). Macrophages exposed to violacein displayed elevated levels of pro-inflammatory TNFα concomitant with TNF receptor 1 (TNFR1) activation, which induces cellular apoptosis. In addition, the authors suggested that Toll-like receptors (TLRs) could be activated by violacein in murine cells, which are strongly associated with PAMPs (i.e., pathogen-associated molecular patterns). Particularly, human TLR8 was activated by violacein in the transfected HEK-293 cell line, and an in silico docking proposed a binding mode of violacein to the receptor similar to the antiviral drug imidazoquinolinone (Venegas et al., 2019). Members of the imidazoquinolines and imidazoles, which resemble the heterocycle structure of violacein, are known to activate TLR7/8, triggering cytokine production by the immune system associated with the clearance of virally infected cells and treatment of skin cancer. The activation of TLR7/8 by imidazoquinolines and imidazoles depends on the electronic configuration of the heterocycles (Kaushik et al., 2021).
Briefly, the major anti-inflammatory effects of violacein were associated to the reduction of pro-inflammatory cytokines such as TNF-α, TGF-ß, IL-1β, and IL-6, and chemokines CXCL1 and CXCL12 involved in the cell recruitment but also increase the expression of IL-4 and IL-10 (Antonisamy and Ignacimuthu, 2010; Platt et al., 2014; Verinaud et al., 2015).
In a pioneering study, May et al. (1989), May et al. (1991) reported the in vitro antiviral activity of violacein/deoxyviolacein (90/10) in HeLa cells infected with poliovirus and herpes simplex virus (HSV-1). The IC50 value of violacein against HSV-1 infected cells was estimated to be 0.577 µM. A decade later, another study challenged the in vitro antiviral activity of violacein against HSV-1, Simian rotavirus SA11 (RV-SA11), and Poliovirus type 2 (PV-2) strains with pigment concentrations approximately 1/3–1/2 below the cytotoxicity levels on the tested cell lines (i.e., HEp-2, FRhK-4, MA104, or Vero cell cultures). The antiviral activity of 1.25 μM violacein against the tested virus by the MTT assay ranged from 24.3% to 8.5%, depending on the virus and strain (Andrighetti-Fröhner et al., 2003).
The differences in violacein effectiveness against the tested viruses entail analyzing the main characteristics of the viral surface to underpin the potential antiviral mechanism of the pigment. Rotaviruses are non-enveloped viruses that contain a glycoprotein, VP7, attached to the cell surface. Glycoprotein VP7 is composed of 326 amino acids, with two hydrophobic domains in the amino-terminal group (Poruchynsky et al., 1985). In addition, polioviruses are non-enveloped viruses with three main proteins, VP1, VP2, and VP3, on the capsid surface, that are folded in a hydrophobic ß-barrel (He et al., 2000). On the other hand, members of the herpes virus family are enveloped, having a lipid membrane structure obtained from the host covering the virion. Similarly, viral structures can be observed in coronaviruses and human immunodeficiency virus (HIV). Despite the different surface characteristics of the three tested viruses, all shared hydrophobic moieties on their surfaces that could interact with water-insoluble molecules, such as violacein. Since the interaction of violacein with molecules with hydrophobic motifs (i.e., non-ionic surfactants, cyclodextrins, aromatic ionic liquids, lipid carriers) were previously reported (de Azevedo et al., 2000; Rivero Berti et al., 2019; Rivero Berti et al., 2020; Rivero Berti et al., 2022). It is expected that hydrophobic interactions appear to be the unspecific major mechanism of interaction between the virus and violacein.
Murine hepatitis virus (MHV), like SARS-CoV-2, belongs to the genus Betacoronavirus. Both are enveloped, with glycoproteins on the surface and a genome composed of positive-sense single-stranded RNA within a nucleocapsid. The inhibition of MHV-3 in L929 (mouse fibroblasts, ATCC CCL-1) infected cells by 20 µM violacein was 42% after incubation at 37°C for 1 h (Gonçalves et al., 2023).
In a recent physicochemical study, the in vitro inhibitory effects of purified violacein and deoxyviolacein on CoV-2 spike RBD: ACE2 and HIV-1 Reverse transcriptase proteins were analyzed (Supplementary Table S2). These results indicated that deoxyviolacein had a low inhibitory effect on both proteins at millimolar concentrations. Meanwhile, 1 mM violacein inhibited 94.3% of HIV-1 Reverse transcriptase, and CoV-2 spike RBD: ACE2 was inhibited by 53% at 2 mM violacein (Dogancı et al., 2022). The concentration of the pigment needed to inhibit the activity of these proteins is approximately one thousand times higher than the high levels required to induce cytotoxicity in most of the reported cellular cell lines (de Sousa Leal et al., 2015; Duran et al., 2021a).
The low antiviral activity of violacein against HSV-1, Simian rotavirus 219 SA11, and Poliovirus type 2 reported previously (Andrighetti-Fröhner et al., 2003) could be attributed to its extremely poor solubility of the biodye under physiological environmental conditions, which may be a major obstacle to replicate the conditions of in vitro assays (e.g., the concentration of the dye) in cell assays or in vivo. The insolubility of violacein in aqueous media can be attributed to the lack of polar groups and the presence of aromatic motifs. The interaction of indole aromatic residues of different molecules that can pile on each other, which is attributed to π-π stacking, reduces violacein interactions with other molecules, including water. Consequently, molecular aggregates of violacein display reduced biological activities, similar to some low-solubility marketed drugs containing aromatic rings (Islan et al., 2012). It would be of most interest to study whether it is possible to generate violacein derivatives that retain or even potentiate the molecular interactions responsible for its antiviral effects (increasing potency) and, simultaneously, include structural modifications that diminish their π-π stacking potential, reducing their tendency to aggregate. It is important to bear in mind, however, that π-π stacking can be exploited in the design of last-generation drug delivery systems, including high purified carbon- or graphene-based nanomaterials, nanocomposites, targeted delivery systems, and prodrug delivery systems such as self-assembled polyprodrug amphiphiles (Zhuang et al., 2019). The key question appears to be: Can violacein molecular structure be modified in a way that the derivatives retain the ability to interact with viral constituents (which, as explained later, also requires π-π stacking) and load the dye into state-of-the-art drug delivery systems, and at the same time display enhanced aqueous solubility? Are these mutually exclusive properties? In any case, fine-tuning would likely be required at the molecular optimization step.
Besides that, the antiviral mechanisms of violacein were not reported yet, based on the biophysical properties of the biodye it could be speculate that the hydrophobic interaction between the virus moieties and violacein will be the major driven force for virus inactivation.
Enveloped viruses are causative agents of diverse infections. Enveloped viruses have a lipid membrane covering the viral structure (i.e., the capsid) that originates from the infected host. Among the advantages of encapsulated viruses, the fusion of the viral membrane with the cell membrane is considered to be one of the most effective strategies for infecting cells. In particular, many coronaviruses have a bilayer of lipids and proteins, forming an envelope around their capsids. This envelope is derived from host membranes during virion formation but also plays a crucial role during infection when virus particles adhere to the host cell membrane (Nardacci et al., 2021). Therefore, the role of lipids in SARS-CoV-2 infection and pathogenesis cannot be underestimated. In a previous study, the existence of pockets of high hydrophobicity in the SARS-CoV-2 protein S (spike), which firmly bind linoleic acid, was described. This binding seemingly locks the S protein in a state that decreases its association with the ACE2 receptor (Toelzer et al., 2020). These types of hydrophobic pockets have previously been used to develop drugs to treat rhinoviruses (Casanova et al., 2018).
Additionally, lipid membranes play a key role in the replication of all RNA (+) viruses, including coronaviruses. These viruses manipulate the host membranes to form viral replication organelles. In addition to sequestering the cellular machinery necessary for viral multiplication, these organelles may also play a role in evading the immune response. It is known that coronaviruses alter the cellular metabolism of lipids, favoring the synthesis of sterols and fatty acids that are propitious to them in an analogous way to that in which tumor cells reprogram lipid metabolism to ensure their survival (Borella et al., 2022; Rudiansyah et al., 2022). Furthermore, coronaviruses interfere with exosome formation, autophagy, and lipid rafts (Casari et al., 2021). This reorganization of lipid metabolism and membrane mechanisms ensures or promotes viral multiplication and is, therefore, a potential target for new drugs or therapeutic strategies.
Therefore, studies of violacein-related lipids and membrane systems are of interest. While violacein does not have a typical amphiphilic head-tail structure, it is hydrophobic and has some polar groups; therefore, direct interaction with cell or viral membranes is highly probable (de Souza et al., 2017). Cauz et al. (2019) described some interactions between violacein and membranes, initially, to explain its antimicrobial activity. However, these findings may be useful in explaining its antiviral activity. In vitro experiments have shown that the addition of violacein to large unilamellar vesicles (LUVs) disrupts these vesicles and produces leaks, thereby affecting their permeability. Additionally, because of the low concentration of violacein required to produce these effects, they hypothesized that violacein does not act as a tensioactive, like many antimicrobials, but rather produces defects and discontinuities in phospholipid organization that alter membrane permeability (Cauz et al., 2019). This partially contrasts with previous results; de Souza et al. (2017) explored violacein interactions with phospholipid thin films to model eukaryotic plasma membranes and found that violacein effectively interferes with the correct ordering of phospholipids, affecting their compressibility and viscoelasticity, and therefore fluidity, but does not produce changes in permeability in the tested models (de Souza et al., 2017). In a recent study, the effect of violacein on synthetic monolayer lipid membranes composed of 1,2-dipalmitoyl-sn-glycero-3-phosphocholine (DPPC), 1,2-distearoyl-sn-glycero-3-ethylphosphocholine chloride salt (DSEPC), and 1,2-dipalmitoyl-sn-glycero-3-phospho-rac-(1-glycerol) sodium salt (DPPG) was studied using the Langmuir-Blodgett technique. The isotherms of violacein in the cationic and zwitterionic lipid monolayers showed a decrease in lipid molecular area, suggesting compaction. However, compaction was not observed in negatively charged lipid monolayers. Further analyses with X-rays indicate that violacein decreases the lipid tilt angle, which consequently induces the thickening of lipid monolayers (Gupta et al., 2021; Gupta and Ghosh, 2023). These results indicate the interaction of the aromatic electrons of violacein with the positive residues of lipid-like π-σ non-specific molecular interactions.
A recent study showed that the release of violacein from the cells in one of the main bacterial pigment producers, Chromobacterium violaceum (ATCC 12472), occurred through approximately 100 nm extracellular membrane vesicles. The study revealed that 79.5% of the violacein encapsulated in the membrane vesicles remained in solution, and the membrane vesicles provided an increase of violacein estimated in 1740 folds (Choi et al., 2020). The strategy of violacein release in C. violaceum possesses two relevant characteristics: first, the pigment will not be attached to the cell surface because the bacteria grow in aqueous media and violacein is a very hydrophobic molecule; and second, the released membrane vesicles can be attached to the hydrophobic surfaces of several molecules and structures, such as the VP7 glycoprotein of rotaviruses and the ß-barrel structure of VP1-VP3 of poliovirus. The interaction of viral glycoproteins present on the virus surface handles virus-cell interactions, leading to causative infections (Cosset and Lavillette, 2011). Consequently, membrane vesicles containing violacein can easily merge with other membranous lipid structures of enveloped viruses (HSV-1, SARS-CoV-2, HIV, etc.).
The main cellular target of violacein are biological membranes and molecules because of violacein hydrophobicity (i.e., low solubility in watery environments). Additionally, the recent finding of violacein which is released from the producer cells in the form of membranous structures will favor the interaction within mammalian cell membranes and also facilitates the entrance of violacein to the cytoplasm.
The traditional mechanism of SARS-CoV-2 infection involves the binding of the spike (i.e., S glycoprotein) to ACE2 (i.e., angiotensin-converting enzyme type 2) to produce a membrane fusion by a serine protease (i.e., TMPRSS-2) or by the cysteine protease pathways (i.e., cathepsins). In recent studies, the spike in SARS-CoV-2 variants was found with a wider spectrum of action by activating the transmembrane serine protease 13 (i.e., TMPRSS-13) and the matrix metalloproteases 2 and 9 (i.e., MMP-2 and MMP-9). High MMP levels were found in patients with serious SARS-CoV-2 infections. Supporting the role of proteases in viral infection, protease inhibitors can reduce the infection of the SARS-CoV-2α in the human fibrosarcoma HT1080 cell line (Benlarbi et al., 2022).
In previous work, the inhibition of matrix metalloproteases (MMPs) by violacein was reported (Platt et al., 2014). The authors reported that violacein inhibited MMP-2 in the MCF-7 cell line, and the metalloprotease played a relevant role in the secretion of CXCL12, an inflammatory cytokine. Additionally, violacein inhibited MMP-9, which downregulates the a-chemokine receptor (CXCR4) specific for stromal-derived factor-1, a chemotactic molecule for the recruiting lymphocytes (Platt et al., 2014).
Recently it was suggested that violacein presumably acted as a protease inhibitor against the ACE2 receptor, and as an immunomodulator against COVID-19 (Durán et al., 2022). Mpro, also called 3CLpro, and also the papain-like protease (PLpro) is responsible for viral polyprotein disruption, a process important for the virus to survive and replicate. Mpro is a decisive coronavirus enzyme that plays essential roles in modulating two key processes: viral transcription and replication, which makes it an attractive pharmacological target against SARS-CoV-2.
Then, one strategy could be protease inhibition as a target to Mpro, once it was shown that inhibition of many proteases was discovered by researching the cytotoxic actions of violacein. Decease caused in CD34+/c-Kit+/P-glycoprotein+/MRP1+ TF1 leukemia progenitor cells was moderated by calpain (calcium-dependent protease-cysteine protease) suppression and by decease-associated protein kinase 1 (DAPK1). Comparative analysis showed that violacein also induced several protein kinases activities, such as protein kinase A (PKA), pyruvate dehydrogenase kinase (PDK), and protein kinase B (AKT), that were monitored by structural modifications induced by endoplasmic reticulum stress and Golgi apparatus collapse, as led to cell decease (Queiroz et al., 2012). It is attainable concluding some chemical structural comparison between violacein activity and that of protease suppressors such as Ebselen (Figure 3).
FIGURE 3. Ebselen molecular structure, reprinted from Benjah-bmm 27, Public Domain, via Wikimedia Commons
Following the suppression data, the HIV-1 RT suppression rate of violacein (1 mM) from the Janthinobacterium sp. GK strain was higher than 90%, and the SARS-CoV-2 spike RBD: ACE2 suppression rate of violacein (2 mM) was higher than 50%. In silico studies were performed to explore the potential interactions among deoxyviolacein and violacein and three reference compounds with the target proteins: ACE2, SARS-CoV-2 spike RBD (i.e., receptor binding domain), and HIV-1 RT (i.e., reverse transcriptase). Violacein seems to bind strongly to the three receptors as showed by their docking binding energies: −9.94 kcal/mol for ACE2, −9.32 kcal/mol for HIV-1 RT, and −9.32 kcal/mol for SARS-CoV-2 spike RBD. Comparable results were obtained for deoxyviolacein: −10.38 kcal/mol for ACE2, -9.50 kcal/mol for HIV-1 RT, and −8.06 kcal/mol for SARS-CoV-2 spike RBD. Following these outcomes, deoxyviolacein and violacein seem to bind to all the receptors with high efficiency. HIV-1-RT and SARS-CoV-2 spike protein suppression searches with deoxyviolacein and violacein were reported for the first time in the literature (Doganci et al., 2022).
The inhibition of diverse proteases involved in the viral infection process by violacein observed experimentally and by in silico molecular docking is a relevant advantage from therapeutic point of view because it is additional feature to the many biological activities of violacein which will reduce the chance of drug multi-resistance displayed by many molecules available in the market.
In vitro cell toxicity of violacein was evaluated in four mammalian cell lines (i.e., Vero, MA104, FRhK-4, and HEp-2) using cell morphology by light microscopy, cell viability by Trypan blue and by the MTT assays (Andrighetti-Fröhner et al., 2003). The violacein cytotoxic concentration 50% was in the range of 2.07 a 3.55 µM, which depends on the used technique and the cell line. While the concentrations of violacein showed the best antiviral activity at 1.25 µM against HSV-1 (i.e., strains KOS, VR-733, RV-SA11) with inhibition percentages in the range of 17.75%–24.27% and compared with acyclovir with inhibition percentages close to 100% Besides, the antiviral violacein concentration assayed was 35.5 to 8.9 times lower compared to the acyclovir (Andrighetti-Fröhner et al., 2003). In general, violacein cytotoxicity in non-tumoral cell lines is in the range of 5 µM–10 μM, meanwhile tumoral cell lines are more sensitive to the biodye with a cytotoxic range of 0.71 µM to about 5 µM (de Sousa Leal et al., 2015; Rivero Berti et al., 2020). Several mechanisms of violacein cytotoxicity including disturbance of mammalian cell membrane and polarization of mitochondrial membrane, disruption of actin function, p44/42 mitogen activated protein kinase (MAPK), apoptosis mediated by caspase 3, etc. were reviewed recently (Duran et al., 2016; Durban et al., 2021a). Besides, it is currently accepted that violacein displays low toxicity in-vitro against non-tumoral cell lines.
In an attempt to further study the potential binding site of violacein within the SARS-CoV-2 Spike protein, five potential binding sites reported in the literature were explored through molecular docking using AutoDock4 (Morris et al., 2009). The binding sites analyzed in this study are shown in Figure 4. Two sites are located within the S2 subunit: site 1 refers to the arbidol site previously published (Shuster et al., 2021) and site 2 corresponds to the fusion peptide binding site described in a recent report (Hu et al., 2021). The other sites are located within the S1 subunit: site 3 is a potential allosteric site reported by Wang et al. (2022), site 4 corresponds to the experimentally determined biliverdin site in the N-terminal domain (NTD) (Rosa et al., 2021), and site 5 addresses two possible reported sites in the RBD subunit, which are the Spike: ACE2 protein-protein interaction (PPI) interface (site 5α) (Bojadzic et al., 2021) and the site reported by Dogancı et al. (2022) for violacein from the blind docking study on the isolated RBD subunit (site 5β). In addition, the entire RBD subunit was considered for the docking calculations to explore other possible binding sites within such subunit (site 5γ). The biliverdin site has not been considered an inhibitor site, because biliverdin binding helps Spike evade antibody immunity (Rosa et al., 2021). Nevertheless, it was decided to include it in the present study because it is an experimentally validated binding site for small molecules.
FIGURE 4. 3D structure of the SARS-CoV-2 Spike protein, where all potential violacein binding sites studied by molecular docking are highlighted on protomer A. Protomer A is colored light blue while the corresponding RBD subunit is cornflower blue. Site 1 (navy blue) refers to the arbidol site located within the S2 subunit, site 2 (magenta) corresponds to a fusion peptide site, site 3 (salmon) is a potential allosteric site within the S1 subunit, site 4 (lime green) corresponds to the biliverdin site in the NTD, site 5α (red) refers to the Spike: ACE2 PPI interface, site 5β (yellow) corresponds to the site reported by Dogancı et al. (2022) for violacein from a blind docking study on the RBD subunit, and site 5γ (purple) emerged by considering the entire RBD subunit for docking calculations; to the best of our knowledge, this is the first report on this putative binding site.
The docking results obtained are shown in Supplementary Table S3. The most favorable score considering the three protomers was obtained for the 5γ site in the RBD subunit. While site 5γ is likely to be the most probable binding site, site 2 and site 3 are also probable candidates.
Considering site 5γ in protomer A, violacein was located in an environment defined by residues Phe338A, Tyr365A, Tyr369A, Ala372A, Ser373A, Phe374A, Asp405C, Glu406C, Arg408C, Gln409C, Thr415C, Gly416C, Lys417C (Figure 5A), finding a parallel-displaced π-stacking with Tyr365A, a T-shaped π-stacking with Tyr369A, hydrogen bonds with Tyr369A, Ser373A, and Thr415C, and a π-cation interaction with Arg408C (Figure 5B).
FIGURE 5. Binding site 5γ in protomer A predicted for violacein by molecular docking and characterized by the DoGSiteScorer web server (A). Pose prediction for violacein. Some relevant interactions are shown with black dashed lines (B). Violacein’s RMSD (Å) vs. simulation time plot (C).
To further study the different interactions between violacein and the 5γ binding site in the protomer A, 50 nanoseconds of molecular dynamics (MD) simulation was carried out using GROMACS 2020.4 Molecular Dynamics engine (Abraham et al., 2015).
Figure 5C shows the root-mean-square deviation (RMSD) for violacein as a function of the simulation time. The average RMSD value was 0.645 Å with a standard deviation of 0.152 Å, indicating high ligand stability at the 5γ site. By exploring the interactions that stabilize the complex during the MD simulation through the measurement of the distances between violacein atoms and the key residues of the protein defined by docking (Supplementary Figures S1–S5), it can be concluded that the stabilization of violacein at the 5γ site appears to be mediated mainly by π-stacking and π-cation interactions, with a coordinated contribution from hydrogen bonding. The docking and MD procedure are described in the Supplementary Material together with a detailed analysis of the interactions observed in this study.
Finally, due to the novelty of this site, the SARS-CoV-2 Spike protein was further analyzed through the DoGSiteScorer web server, a grid-based method that uses a Difference of Gaussian filter to the binding pocket prediction, characterization, and druggability estimation (Volkamer et al., 2012). This analysis ranked the 5γ site in third place out of more than 100 potential sites found in the protein, with a druggability score of 0.85 (0.86 being the highest druggability score obtained).
The large-scale production of microbial pigments is seriously limited by the production-purification costs and regulations in dominant pharmaceutical countries. In 2018, the world market for organic dyes was valued at U$D 3.5 billion, with an estimated growth of about 37% by 2024 (Cassarini et al., 2021). The most representative studies on violacein production are briefly described below, including the use of recombinant microorganisms and the new trend of recycling waste in the frame of the circular economy.
Violacein production was initially studied in the 2000s. The first work on violacein production was conducted in C. violaceum and Janthinobacterium lividum in synthetic liquid media (Mendes et al., 2001; Nakamura et al., 2003). The main guidelines for the production of violacein from C. violaceum can be found in the websites of the American Type Culture Collection (ATCC, United States, https://www.atcc.org/products/12472), and the National Collection of Type Cultures (NCTC, United Kingdom, https://www.culturecollections.org.uk/products/bacteria/detail.jsp?collection=nctcandrefId=NCTC+9757).
In general, it is currently accepted that carbon sources play a crucial role in pigment synthesis; in particular, glycerol increases while glucose represses its production (Pantanella et al., 2007; Duran et al., 2021). These results indicate shared pathways for simple carbon sources, as well as the presence of catabolic repression by glucose. A systematic statistical study of violacein production by C. violaceum CCT 3496 cultured in a 50 mL flask containing glucose-rich media supplemented with tryptophan was conducted using a fractional factorial design followed by a central composite design. The violacein production increased from 7.5 g L−1 dry cell mass and 0.17 g L−1 crude violacein concentration to 21 g L−1 and 0.43 g L−1, respectively (Mendes et al., 2001). The ratios of dry cell mass/crude violacein concentration were approximately 44 and 49 for initial and optimized production, respectively. These results indicate that coupled violacein production is related to bacterial cell mass.
The violacein producer Duganella sp. B2, isolated from a glacier in China, was statistically optimized using a two-level Placket-Burman, Box-Behnken, and surface response for biodye production. The experiments were carried out in 250 mL stirred flasks containing minimal saline medium and starch as a carbon source supplemented with tryptophan. The highest violacein concentration produced in the optimized medium was 1.62 g L-1 after 32 h of culture (Wang et al., 2009).
The production of violacein from a wild-type isolated psychotropic bacterium RT102, close to J. lividum, cultivated in a 3-L fermenter containing rich media (i.e., glucose, casein, and yeast extract) under physicochemical controlled parameters resulted in a maximum violacein concentration and productivity of 3.7 g L-1 and 0.12 g L-1 h-1 at 40 h culture (Nakamura et al., 2003).
Several efforts have been made to obtain recombinant bacterial strains that are capable of producing high concentrations of violacein. Most studies have focused on E. coli (Rodrigues et al., 2012; Rodrigues et al., 2013; Fang et al., 2015). The E. coli TOP10 strain transformed with the plasmids pJP1000 and pPSX-Vio + which are harboring the violacein operon pBvioABCDE from C. violaceum showed low levels of violacein production ranging from 0.006 to 0.025 g L-1, which was comparable to the biodye production by J. lividum DSM 1522 under the same experimental conditions (Rodrigues et al., 2012). In another study by the same research group, different E. coli strains were transformed with plasmids and the integrated gene responsible for violacein production. The mutant E. coli dVio-1 and dVio-6 expressing the vioABCDE gene cluster accumulate 0.18 g L-1 and 320 mg L-1 of deoxyviolacein intracellularly, respectively. Integration of the vioD gene into the E. coli genome, E. coli Vio-4, produced 0.71 g L-1 of intracellular violacein by fed-batch fermentation (Rodrigues et al., 2013). In future work, different recombinant E. coli BL21 strains were used as a chassis for the expression of violacein genes inserted in a plasmid associated with the enhanced production of tryptophan. Flask culture in glucose saline medium of the recombinant strain E. coli B2/pED + pVio produces 0.60 g L-1 violacein at a concentration of 48 h. Scale-up of violacein production in a fermenter with an optimized glucose medium showed a titer of 1.75 g L−1 associated with 36 mg L−1 h−1 biodye productivity (Fang et al., 2015).
In another microbial recombinant strategy, Citrobacter freundii containing the violacein gene cluster in a plasmid (i.e., pCom10vio) was cultured in a 2 L minimal salt medium supplemented with glycerol and tryptophan in a 5-L fermenter using a fed-batch technique. The optimized yield parameters were 3.34 g L-1 dry cell mass, maximum violacein concentration of 4.13 g L-1 with violacein productivity of 0.083 mg L−1 h−1 (Yang et al., 2011).
However, some issues must be taken into account for potential commercial applications of violacein in pharmaceutical applications because the high production and/or productivity of violacein in recombinant strains depends on the strain stability during scale-up and successive microbial cultures, violacein purification complexity (i.e., steps and procedures) because the biodye is located intracellularly in most recombinant microorganisms, and the presence of violacein precursors and/or cometabolites (i.e., protoviolaceinic acid, deoxyviolacein, etc.). Alternatively, the use of agricultural food waste can be an alternative to reduce violacein-producing costs as well as co-production with other metabolites of industrial interest. Pioneering investigations reported in the literature for violacein production were developed by Ahmad’s research group at the University Technical of Malaysia. A wild-type strain of C. violaceum isolated from a waste plant has been extensively studied for the production of violacein in liquid media supplemented with local agricultural wastes and/or cheap substrates, such as brown sugar, molasses, solid pineapple waste, sugarcane bagasse, and commercial-rich media. Violacein production in the rich medium was very low or negligible. While the high biodye productions were 0.19 g L-1 and 0.82 g L-1 obtained in a medium containing 1% and 3% sugarcane bagasse supplemented with tryptophan, a pigment precursor, respectively (Ahmad et al., 2012).
Violacein production in C. violaceum UTM5 was studied by combining static and stirred growth conditions, and violacein production was between 0.17 g L-1 to 0.26 g L-1. However, high production was observed when the microorganism was first cultivated under static conditions followed by stirring. After environmental optimized culture conditions, the microorganism produced 16.26 ± 0.44 g L-1 of violacein in a 50 L fermenter containing 45 L of the medium of filtered pineapple waste supplemented tryptophan at 30°C for 24 h (Aruldass et al., 2015).
Microparticulate wheat bran, a low-cost substrate with high lignocellulose content, was used to supplement the Luria-Bertani medium for violacein production using C. vaccinii in batch culture (Cassarini et al., 2021). The optimal violacein production was 0.208 g L-1 after 73 h of culture.
In a recent study, the simultaneous production of poly hydroxybutyrate (PHB) and violacein was reported (Kumar et al., 2021). The synthesis of PHB and violacein by wild-type Iodobacter sp. PCH194 was optimized in a 22-L fermenter containing glucose and tryptone in a saline medium. The maximum production of PHB was 11.0 ± 1.0 g L-1, and a mixture of violacein (50%–60%) and deoxyviolacein (40%–50%) was obtained. The highest concentration of violacein was 1.5 g L-1 at 48 h of cultivation.
The main data on violacein production using different microorganisms, methodologies, and brief culture conditions are displayed in Supplementary Table S4. Another factor to be considered is related to the obtention of violacein from the culture media. Briefly, at the late stationary phase, the bacterial cells were centrifuged, and the violacein was extracted from the pellet with ethanol (Mendes et al., 2001).
Among the advantages of violacein production are the standard conditions of production, short times for production, the facile bacterial cultivation in fermenters, and scale up with traditional strains, the low nutritional requirements of the microorganism for the biodye production which could include wastes (e.g. with the advantage of the circular economy), easy recovery of the pigment and purification because of high hydrophobicity.
Violacein attached to different materials has two main applications: as a filter barrier with antiviral activity or loaded into particulate systems for drug delivery (Khaksar et al., 2021; Fu et al., 2023).
Because the human coronavirus is mainly transmitted by aerosols caused by coughing, sneezing, breathing, or speaking, there is a need to establish a physical barrier for the dispersion of these aerosols. However, the infectivity over time of the coronavirus in a droplet of aerosol depends greatly on the surface where it is deposited, being able to remain active for several hours in some cases (Fu et al., 2023). This is why a functional material must not only retain droplets but also inactivate the virus.
Strategies have been developed to use violacein as a biodye in the development of functional fabrics, taking advantage of its antiviral properties. Three different methods for dyeing polyamide fabrics with violacein have been tested (Kanelli et al., 2018). Direct fermentation of J. lividum on the fabric, exposure of the fabric to the culture after fermentation, and exposure of the fabric to an acellular extract of the culture. Improved color retention and antimicrobial and biofilm inhibition activities were obtained using the first method (Kanelli et al., 2018). With the same goal of producing functional fabrics, Gao et al. (2019) developed a silk-based composite that takes advantage of the antimicrobial synergy between violacein and silver nanoparticles (Gao et al., 2019). Electrospinning techniques have also been tested to design fabrics based on violacein-containing synthetic polymers, such as Nylon-66 and Polyvinyl-alcohol/polyvinyl-pyrrolidone (Osman and Setu, 2018; Rosli and Setu, 2018). Also, by using electrospinning, Lee et al. (2022) have recently taken this concept further; developing prototype respiratory masks from polyacrylonitrile nanofibers with violacein, demonstrating aerosol retention from 0.8 to 3.4 µm (human-produced aerosols being between 0.74 and 2.12 µm), antimicrobial activity against S. aureus and antiviral activity against human influenza A and human coronavirus. Violacein also provides selective UV protection to the fabric (Lee et al., 2022). In particular, these violacein-loaded materials retain the inactivating activity of coronaviruses and influenza and could constitute products with real applications, such as surgical masks.
In another study, viscose fabric was incubated in the presence of violacein, followed by the incorporation of silver and titanium dioxide nanoparticles by sonication. The antimicrobial activity of the fabric containing violacein and nanoparticles showed a 3- to 6-log reduction in the growth of S. aureus, B. cereus, and E. coli, indicating the effectiveness of the fabric derivatized with violacein and nanoparticles (Khaksar et al., 2021).
Another potential application of violacein is to include biodye in 3D matrices for molecular delivery. In 2000, violacein was added to a ß-CD solution and precipitated. The violacein complexed in cyclodextrin showed the same biocide activity against E. coli, but the cytotoxicity in lung fibroblasts of Chinese hamster V-79 cell cultures was reduced, and lipid peroxidation was fully inhibited at 500 µM of the violacein-ß-cyclodextrin complex (). Later, a proof of concept was developed by the encapsulation of violacein in nanoparticles of poly-(D, L-lactic-co-glycolic acid) for assaying antimicrobial activity. PLGA nanoparticles with 116 and 139 nm diameter containing violacein efficiently inhibit five strains of S. aureus, including antibiotic-resistant (MRSA) strains, at micromolar concentrations three to five times lower than free violacein (Martins et al., 2011). In a subsequent study by Durán et al., violacein was encapsulated in nanoparticles made of poly (ε-caprolactone) covered with chitosan to produce a positively charged device and increase mucoadhesiveness (Berni et al., 2013). Violacein nanoparticles of 201–320 nm were tested to prevent bovine mastitis against S. aureus MRSA with a low minimal inhibitory concentration compared to free biodye. Additionally, violacein encapsulation diminished ecotoxicity by approximately 10 to 5 times using Daphnia similis according to the OCDE guidelines.
Another strategy for violacein encapsulation is the use of Arabic gum as a matrix (Venil et al., 2015). Violacein microparticles can be used to provide color to foods, such as jellies and yogurts. Violacein encapsulated in Arabic gum was spray-dried and displayed high stability in the range of 25°C–60°C for 30 days.
Similarly, based on the low violacein solubility in aqueous media, the biodye was first suspended in a polyoxyethylene sorbitan monolaurate solution and showed high stability at room temperature for 6 days. Later, the violacein emulsion was successfully encapsulated in gelatin-pectin coacervate and tested against HCT-116 colon cancer (Rivero Berti et al., 2019). More recently, violacein was encapsulated in a nanostructured lipid carrier with an active release by lipase and 3D printed using a hydroxypropyl cellulose-chitosan matrix. The formulation was tested against the A549 and HCT-116 cancer cell lines (Rivero Berti et al., 2022).
Other strategies have been developed to increase the biocidal activity of violacein by using metallic nanoparticles. Silver nanoparticles with violacein adsorbed in the surface displayed high antibacterial activities against multiresistant bacterial strains of P. aeruginosa, E. coli, and S. aureus, also tested against Aspergillus tamari, Aspergillus tubingensis, and Fusarium proliferatum, and also algicidal activities against Dictyosphaerium sp. strains DHM1 and DHM 2, and Pectinodesmus sp. strain PHM 3. In all cases, the biocide activity of AgNPs capped with violacein against bacteria, fungi, and algae were enhanced by the presence of the biodye (Arif et al., 2017). Similar results were obtained for silk coated with AgNPs and violacein, as previously described (Gao et al., 2019).
A novel strategy for repositioning old antibiotics is to develop a combination of antibiotics and violacein. The authors developed a 1:1 individual mixture of 20 antibiotics with violacein and tested these mixtures against Salmonella typhi, Vibrio cholerae, P. aeruginosa, K. pneumoniae, and S. aureus. In most cases, the biocidal activity of violacein is synergistic and/or additive concerning the antibiotic (Subramaniam et al., 2014). This strategy offers several advantages. First, the antibiotic-violacein mixtures can increase the biocide activity against pathogens because of different molecular targets, decreasing the amount of the antibiotic concomitantly with a decrease in potential toxicities, and increasing the bioavailability of the components.
Additionally, the interaction of ten imidazole ionic liquids with violacein was analyzed. Imidazole ionic liquids and violacein form stable micellar solutions that are encapsulated in solid lipid nanoparticles. The anticancer activity of the violacein-imidazole ionic liquids formulation against A549, HeLa, and HCT116 cells was tested. These results indicated that the imidazole group of the ionic liquids associated with violacein displayed strong anticancer activity (Rivero Berti et al., 2020). Further computational studies have demonstrated the effectiveness of imidazole derivatives against SARS-CoV-2 (Belhassan et al., 2020). Data from both studies showed promising results for the use of violacein–imidazole ionic liquid formulations for SARS-CoVid-2 therapy.
Taking into consideration what is stated in this section, not only should the biological activity of violacein be considered, but also its ability to form functional materials. The late one is a relevant challenge that will result in a improve biocide and antiviral activities.
Many biological activities of violacein were extensively described in the literature. The previous focus was to use violacein in the treatment of different types of cancer. However, recently found violacein properties such as its effect on cellular membranes, and anti-inflammatory, antioxidant, and antiviral activities suggest that another application is possible. Other detailed studies of violacein activities revealed a wide spectrum of protease inhibition. Additionally, in silico studies through molecular docking confirmed the binding activities of violacein within the SARS-CoV-2 spike in several sites. However, in some cases, the antiviral titers of violacein are not enough to be considered as a potential virucide agent. These results could be attributed to two factors, first the presence of other molecules in the violacein extracts because of a lack of appropriate purification steps and quality controls, and second because of violacein low biodisponibility due to its very low solubility in physiological media. Besides, the production of violacein from different strains and procedures is in the state of the art, but it is essential to standardize pigment purification procedures. Also, the potential oral administration of violacein will require the development of novel strategies for molecular delivery systems to obtain more efficient biocide activities.
IR, writing, drawing, reviewing. MG, docking and molecular studies, writing. SR, docking and molecular studies, writing. GI, editing, reviewing. WF, editing, reviewing. AT, critical review, and text edition, discussion, and manuscript revision. ND, writing, text edition, and discussion. GC, organization, writing, editing, critical review, discussion, and manuscript revision. All authors contributed to the article and approved the submitted version.
Financial support by UNLP (X815), CONICET (PIP 0034), and ANPCyT (PICT 2016-4597; PICT 2017-0359) is gratefully acknowledged.
The authors declare that the research was conducted in the absence of any commercial or financial relationships that could be construed as a potential conflict of interest.
All claims expressed in this article are solely those of the authors and do not necessarily represent those of their affiliated organizations, or those of the publisher, the editors and the reviewers. Any product that may be evaluated in this article, or claim that may be made by its manufacturer, is not guaranteed or endorsed by the publisher.
The Supplementary Material for this article can be found online at: https://www.frontiersin.org/articles/10.3389/fnano.2023.1186386/full#supplementary-material
Abboud, A. N., and Arment, A. (2013). The protective effects of the violacein pigment against UV-C irradiation in Chromobacterium violaceum. Ohio J. Sci. 111 (2-5), 28–32.
Abdelghani, Z., Hourani, N., Zaidan, Z., Dbaibo, G., Mrad, M., and ·Hage-Sleiman, R. (2021). Therapeutic applications and biological activities of bacterial bioactive extracts. Archives Microbiol. 203, 4755–4776. doi:10.1007/s00203-021-02505-1
Abraham, M. J., Murtola, T., Schulz, R., Páll, S., Smith, J. C., Hess, B., et al. (2015). Gromacs: High performance molecular simulations through multi-level parallelism from laptops to supercomputers. SoftwareX 1, 19–25. doi:10.1016/j.softx.2015.06.001
Ahmad, W. A., Yusof, N. Z., Nordin, N., Zakaria, Z. A., and Rezali, M. F. (2012). Production and characterization of violacein by locally isolated Chromobacterium violaceum grown in agricultural wastes. Appl. Biochem. Biotechnol. 167 (5), 1220–1234. doi:10.1007/s12010-012-9553-7
Andrighetti-Fröhner, C. R., Antonio, R. V., Creczynski-Pasa, T. B., Barardi, C. R., and Simões, C. M. (2003). Cytotoxicity and potential antiviral evaluation of violacein produced by Chromobacterium violaceum. Memorias do Inst. Oswaldo Cruz 98 (6), 843–848. doi:10.1590/s0074-02762003000600023
Antonisamy, P., and Ignacimuthu, S. (2010). Immunomodulatory, analgesic, and antipyretic effects of violacein isolated from Chromobacterium violaceum. Phytomedicine 17 (3-4), 300–304. doi:10.1016/j.phymed.2009.05.018
Arif, S., Batool, A., Khalid, N., Ahmed, I., and Janjua, H. A. (2017). Comparative analysis of stability and biological activities of violacein and starch-capped silver nanoparticles. RSC Adv. 7, 4468–4478. doi:10.1039/c6ra25806a
Aruldass, C. A., Venil, C. K., and Ahmad, W. A. (2015). Violet pigment production from liquid pineapple waste by Chromobacterium violaceum UTM5 and evaluation of its bioactivity. RSC Adv. 5, 51524–51536. doi:10.1039/c5ra05765e
Belhassan, A., En-Nahli, F., Zaki, H., Lakhlifi, T., and Bouachrine, M. (2020). Assessment of effective imidazole derivatives against SARS-CoV-2 main protease through computational approach. Life Sci. 262, 118469. doi:10.1016/j.lfs.2020.118469
Benlarbi, M., Laroche, G., Fink, C., Fu, K., Mulloy, R. P., Phan, A., et al. (2022). Identification and differential usage of a host metalloproteinase entry pathway by SARS-CoV-2 Delta and Omicron. iScience 25 (11), 105316. doi:10.1016/j.isci.2022.105316
Berni, E., Marcato, P. D., Nakazato, G., Kobayashi, R. K. T., Vacchi, F. I., Umbuzeiro, G. A., et al. (2013). Violacein/poly(ε-caprolactone)/chitosan nanoparticles against bovine mastistis: Antibacterial and ecotoxicity evaluation. J. Phys. Conf. Ser. 429, 012030. doi:10.1088/1742-6596/429/1/012030
Bojadzic, D., Alcazar, O., Chen, J, Chuang, S. T., Condor Capcha, J. M., Shehadeh, L. A., et al. (2021). Small-molecule inhibitors of the coronavirus spike: ACE2 protein-protein interaction as blockers of viral attachment and entry for SARS-CoV-2. ACS Infect. Dis. 7 (6), 1519–1534. doi:10.1021/acsinfecdis.1c00070
Borella, R., De Biasi, S., Paolini, A., Boraldi, F, Lo Tartaro, D., Mattioli, M., et al. (2022). Metabolic reprograming shapes neutrophil functions in severe COVID-19. Eur. J. Immunol. 52 (3), 484–502. doi:10.1002/eji.202149481
Cao, W., Chen, W., Sun, S., Guo, P., Song, J., and Tian, C. (2007). Investigating the antioxidant mechanism of violacein by density functional theory method. J. Mol. Struct. THEOCHEM 817 (1-3), 1–4. doi:10.1016/j.theochem.2007.04.022
Casanova, V., Sousa, F. H., Stevens, C., and Barlow, P. G. (2018). Antiviral therapeutic approaches for human rhinovirus infections. Future Virol. 13 (7), 505–518. doi:10.2217/fvl-2018-0016
Casari, I., Manfredi, M., Metharom, P., and Falasca, M. (2021). Dissecting lipid metabolism alterations in SARS-CoV-2. Prog. Lipid Res. 82, 101092. doi:10.1016/j.plipres.2021.101092
Cassarini, M., Besaury, L., and Rémond, C. (2021). Valorisation of wheat bran to produce natural pigments using selected microorganisms. J. Biotechnol. 339, 81–92. doi:10.1016/j.jbiotec.2021.08.003
Cauz, A. C., Carretero, G. P. B., Saraiva, G. K. V., Park, P., Mortara, L., Cuccovia, I. M., et al. (2019). Violacein targets the cytoplasmic membrane of bacteria. ACS Infect. Dis. 5 (4), 539–549. doi:10.1021/acsinfecdis.8b00245
Chernyak, B. V., Popova, E. N., Prikhodko, A. S., Grebenchikov, O. A., Zinovkina, L. A., and Zinovkin, R. A. (2020). COVID-19 and oxidative stress. Biochem. Mosc. 85, 1543–1553. doi:10.1134/S0006297920120068
Choi, S. Y., Lim, S., Cho, G., Kwon, J., Mun, W., Im, H., et al. (2020). Chromobacterium violaceum delivers violacein, a hydrophobic antibiotic, to other microbes in membrane vesicles. Environ. Microbiol. 22 (2), 705–713. doi:10.1111/1462-2920.14888
Cosset, F. L., and Lavillette, D. (2011). Cell entry of enveloped viruses. Adv. Genet. 73, 121–183. doi:10.1016/B978-0-12-380860-8.00004-5
Das, G., Ghosh, S., Garg, S., Ghosh, S., Jana, A., Samat, R., et al. (2020). An overview of key potential therapeutic strategies for combat in the COVID-19 battle. RSC Adv. 10 (47), 28243–28266. doi:10.1039/d0ra05434h
de Azevedo, M. B. M., Alderete, J., Rodriguez, J. A., Souza, A. O., Rettori, D., Torsoni, M. A., et al. (2000). Biological activities of violacein, a new antitumoral indole derivative, in an inclusion complex with ß-cyclodextrin. J. Inclusion Phenom. Macrocycl. Chem. 37, 93–101. doi:10.1023/A:1008138807481
de Sousa Leal, A. M., de Queiroz, J. D., de Medeiros, S. R., Lima, T. K., and Agnez-Lima, L. F. (2015). Violacein induces cell death by triggering mitochondrial membrane hyperpolarization in vitro. BMC Microbiol. 15, 115. doi:10.1186/s12866-015-0452-2
de Souza, K. D., Perez, K. R., Durán, N., Justo, G. Z., and Caseli, L. (2017). Interaction of violacein in models for cellular membranes: Regulation of the interaction by the lipid composition at the air-water interface. Colloids Surfaces B Biointerfaces 160, 247–253. doi:10.1016/j.colsurfb.2017.09.027
Doganci, M. A., Sal, F. A., Guler, H. I., Kat, H., Ceylan, E., Belduz, A. O., et al. (2022). Investigation of potential inhibitor properties of violacein against HIV-1 RT and CoV-2 Spike RBD:ACE-2. World J. Microbiol. Biotechnol. 38, 161. doi:10.1007/s11274-022-03350-0
Durán, N., Fávaro, W. J., Brocchi, M., Justo, G. Z., Castro, G. R., Durán, M., et al. (2021b). Patents on violacein: A compound with great diversity of biological activities and industrial potential. Recent Pat. Biotechnol. 15, 102–111. doi:10.2174/2213476X07666201221111655
Durán, N., Justo, G. Z., Duran, M., Brocchi, M., Cordi, L., Tasic, L., et al. (2016). Advances in Chromobacterium violaceum and properties of violacein, its main secondary metabolite. A review. Biotechnol. Adv. 34, 1030–1045. doi:10.1016/j.biotechadv.2016.06.003
Durán, N., Justo, G. Z., Fávaro, W. F., and Nakazato, G. (2022). Violacein, a microbial antiviral product: Does play key role as active agent against SARS-CoV-2? Int. J. Med. Rev. 9, 351–360. doi:10.30491/IJMR.2022.279715.1198
Durán, N., Nakazato, G., Durán, M., Berti, I. R., Castro, G. R., Stanisic, D., et al. (2021a). Multi-target drug with potential applications: Violacein in the spotlight. World J. Microbiol. Biotechnol. 37, 151. doi:10.1007/s11274-021-03120-4
Fang, M.-Y., Zhang, C., Yang, S., Cui, J.-Y., Jiang, P.-X., Lou, K., et al. (2015). High crude violacein production from glucose by Escherichia coli engineered with interactive control of tryptophan pathway and violacein biosynthetic pathway. Microb. Cell Factories 14, 8. doi:10.1186/s12934-015-0192-x
Fodor, A., Tiperciuc, B., Login, C., Orasan, O. H., Lazar, A. L., Buchman, C., et al. (2021). Endothelial dysfunction, inflammation, and oxidative stress in COVID-19 mechanisms and therapeutic targets. Oxidative Med. Cell. Longev. 2021, 1–15. doi:10.1155/2021/8671713
Fu, J., Liu, T., Binte Touhid, S. S., Fu, F., and Liu, X. (2023). Functional textile materials for blocking COVID-19 transmission. ACS Nano 17, 1739–1763. doi:10.1021/acsnano.2c08894
Gao, A., Chen, H., Hou, A., and Xie, K. (2019). Efficient antimicrobial silk composites using synergistic effects of violacein and silver nanoparticles. Mater. Sci. Eng. C 103, 109821. doi:10.1016/j.msec.2019.109821
Gonçalves, M. C., Spoladori, L. F. A., Medeiros, L. P., Woulk, J., Galhard, L. C. F., Yamada-Ogatta, S. F., et al. (2023). Synergistic effect of biological nanosilver and violacein: Green alternatives against Candida auris and coronavirus. Biotechnol. Lett. Submitt. 2023.
Gupta, R., and Ghosh, S. K. (2023). Discerning perturbed assembly of lipids in a model membrane in presence of violacein: Effects of membrane hydrophobicity. Biochimica biophysica acta. Biomembr. 1865 (4), 184130. doi:10.1016/j.bbamem.2023.184130
Gupta, R., Mitra, S., Chowdhury, S., Das, G., Priyadarshini, R., Mukhopadhyay, M. K., et al. (2021). Discerning perturbed assembly of lipids in a model membrane in presence of violacein. Biochimica Biophysica Acta - Biomembr. 1863 (9), 183647. doi:10.1016/j.bbamem.2021.183647
He, Y., Bowman, V. D., Mueller, S., Bator, C. M., Bella, J., Peng, X., et al. (2000). Interaction of the poliovirus receptor with poliovirus. Proc. Natl. Acad. Sci. U. S. A. 97 (1), 79–84. doi:10.1073/pnas.97.1.79
Hu, X., Chen, C. Z., Xu, M., Hu, Z., Guo, H., Itkin, Z., et al. (2021). Discovery of small molecule entry inhibitors targeting the fusion peptide of SARS-CoV-2 spike protein. ACS Med. Chem. Lett. 12 (8), 1267–1274. doi:10.1021/acsmedchemlett.1c00263
Hughes, J. D., Blagg, J., Price, D. A., Bailey, S., Decrescenzo, G. A., Devraj, R. V., et al. (2008). Physiochemical drug properties associated with in vivo toxicological outcomes. Bioorg. Med. Chem. Lett. 18, 4872–4875. doi:10.1016/j.bmcl.2008.07.071
Islan, G. A., Perez de Verti, I., Marchetti, S. G., and Castro, G. R. (2012). Studies of ciprofloxacin encapsulation on alginate/pectin matrixes and its relationship with biodisponibility. Appl. Biochem. Biotechnol. 167 (5), 1408–1420. doi:10.1007/s12010-012-9610-2
Jehlička, J., Edwards, H. G., Němec, I., and Oren, A. (2015). Raman spectroscopic study of the Chromobacterium violaceum pigment violacein using multiwavelength excitation and DFT calculations. Spectrochimica Acta Part A Mol. Biomol. Spectrosc. 151, 459–467. doi:10.1016/j.saa.2015.06.051
Kanelli, M., Mandic, M., Kalakona, M., Vasilakos, S., Kekos, D., Nikodinovic-Runic, J., et al. (2018). Microbial production of violacein and process optimization for dyeing polyamide fabrics with acquired antimicrobial properties. Front. Microbiol. 9, 1495. doi:10.3389/fmicb.2018.01495
Kaushik, D., Kaur, A., Petrovsky, N., and Salunke, D. B. (2021). Structural evolution of toll-like receptor 7/8 agonists from imidazoquinolines to imidazoles. RSC Med. Chem. 12 (7), 1065–1120. doi:10.1039/d1md00031d
Khaksar, F., Rigi, G., and Mirdamadian, S. H. (2021). Creation of a violacein pigment hybrid with silver and titanium dioxide nanoparticles to produce multifunctional textiles with antimicrobial properties. Nanomedicine Res. J. 6 (1), 60–72. doi:10.22034/nmrj.2021.01.007
Konzen, M., De Marco, D., Cordova, C. A., Vieira, T. O., Antonio, R. V., and Creczynski-Pasa, T. B. (2006). Antioxidant properties of violacein: Possible relation on its biological function. Bioorg. Med. Chem. 14 (24), 8307–8313. doi:10.1016/j.bmc.2006.09.013
Kulandaisamy, V. C., Laurent, D., and Ponnuswamy, R. D. (2020). Bacterial pigments: Sustainable compounds with Market potential for pharma and food industry. Front. Sustain. Food Syst. 4, 100. doi:10.3389/fsufs.2020.00100
Kumar, V., Darnal, S., Kumar, S., Kumar, S., and Singh, D. (2021). Bioprocess for co-production of polyhydroxybutyrate and violacein using Himalayan bacterium Iodobacter sp. PCH194. Bioresour. Technol. 319, 124235. doi:10.1016/j.biortech.2020.124235
Laforge, M., Elbim, C., Frère, C., Hémadi, M., Massaad, C., Nuss, P., et al. (2020). Tissue damage from neutrophil-induced oxidative stress in COVID-19. Nat. Rev. Immunol. 20. 515–516. doi:10.1038/s41577-020-0407-1
Lee, J., Bae, J., Youn, D. Y., Ahn, J., Hwang, W. T., Bae, H., et al. (2022). Violacein-embedded nanofiber filters with antiviral and antibacterial activities. Chem. Eng. J. 444, 136460. doi:10.1016/j.cej.2022.136460
Lipinski, C. A., Lombardo, F., Dominy, B. W., and Feeney, P. J. (2001). Experimental and computational approaches to estimate solubility and permeability in drug discovery and development settings 1PII of original article: S0169-409X(96)00423-1. The article was originally published in advanced drug delivery reviews 23 (1997) 3–25. 1. Adv. Drug Deliv. Rev. 46, 3–26. doi:10.1016/s0169-409x(00)00129-0
Ma, J., Gu, Y., and Xu, P. (2020). A roadmap to engineering antiviral natural products synthesis in microbes. Curr. Opin. Biotechnol. 66, 140–149. doi:10.1016/j.copbio.2020.07.008
Martins, D., Costa, F. T. M., Brocchi, M., and Durán, N. (2011). Evaluation of the antibacterial activity of poly-(D,L-lactide-co-glycolide) nanoparticles containing violacein. J. Nanoparticle Res. 13, 355–363. doi:10.1007/s11051-010-0037-9
May, G., Lenk, W., and Ott, H. (1989). Trans-hydroxyviolacein, process for preparing it pure and its use for the prophylaxis and therapy of viral diseases. Ger. Pat. 1989 (A1), DE3813465.
May, G., Brummer, B., and Ott, H. (1991). Treatment of prophylaxis of polio and herpes virus infections comprises admin. of 3-(1, 2-dihydro-5-(5-hydroxy-1H-indol-3-yl)-2-oxo-3H-pyrrole-3-ylidene)-1, 3-dihydro-2H-indol-2-one. Ger. Pat. De. 3935066 1991.
Mei, M., and Tan, X. (2021). Current strategies of antiviral drug discovery for COVID-19. Front. Mol. Biosci. 8, 671263. doi:10.3389/fmolb.2021.671263
Mendes, A. S., de Carvalho, J. E., Duarte, M. C.-T., Duran, N., and Bruns, R. E. (2001). Factorial design and response surface optimization of crude violacein for Chromobacterium violaceum production. Biotechnol. Lett. 23, 1963–1969. doi:10.1023/A:1013734315525
Morris, G. M., Huey, R., Lindstrom, W., Sanner, M. F., Belew, R. K., Goodsell, D. S., et al. (2009). AutoDock4 and AutoDockTools4: Automated docking with selective receptor flexibility. J. Comput. Chem. 30 (16), 2785–2791. doi:10.1002/jcc.21256
Muhammad, Y., Kani, Y. A., Iliya, S., Muhammad, J. B., Binji, A., El-Fulaty Ahmad, A., et al. (2021). Deficiency of antioxidants and increased oxidative stress in COVID-19 patients: A cross-sectional comparative study in jigawa, northwestern Nigeria. SAGE Open Med. 9, 205031212199124–205031212199128. doi:10.1177/2050312121991246
Mumtaz, R., Bashir, S., Numan, M., Shinwari, Z. K., and Muhammad Ali, M. (2019). Pigments from soil bacteria and their therapeutic properties: A mini review. Curr. Microbiol. 76, 783–790. doi:10.1007/s00284-018-1557-2
Nakamura, Y., Asada, C., and Sawada, T. (2003). Production of antibacterial violet pigment by psychrotropic bacterium RT102 strain. Biotechnol. Bioprocess Eng. 8, 37–40. doi:10.1007/BF02932896
Nardacci, R., Colavita, F., Castilletti, C., Lapa, D., Matusali, G., Meschi, S., et al. (2021). Evidences for lipid involvement in SARS-CoV-2 cytopathogenesis. Cell Death Dis. 12 (3), 263–312. doi:10.1038/s41419-021-03527-9
Osman, S. H., and Setu, S. A. (2018). Fabrication of nylon-66 membranes coated with violacein pigment for wound dressing application. EProceedings Chem. 3 (3), 23–30.
Pantanella, F., Berlutti, F., Passariello, C., Sarli, S., Morea, C., and Schippa, S. (2007). Violacein and biofilm production in Janthinobacterium lividum. J. Appl. Microbiol. 102 (4), 992–999. doi:10.1111/j.1365-2672.2006.03155.x
Platt, D., Amara, S., Mehta, T., Vercuyssee, K., Myles, E. L., Johnson, T., et al. (2014). Violacein inhibits matrix metalloproteinase mediated CXCR4 expression: Potential anti-tumor effect in cancer invasion and metastasis. Biochem. Biophysical Res. Commun. 455 (1-2), 107–112. doi:10.1016/j.bbrc.2014.10.124
Poruchynsky, M. S., Tyndall, C., Both, G. W., Sato, F., Bellamy, A. R., and Atkinson, P. H. (1985). Deletions into an NH2-terminal hydrophobic domain result in secretion of rotavirus VP7, a resident endoplasmic reticulum membrane glycoprotein. J. Cell Biol. 101 (6), 2199–2209. doi:10.1083/jcb.101.6.2199
Queiroz, K. C., Milani, R., Ruela-de-Sousa, R. R., Fuhler, G. M., Justo, G. Z., Zambuzzi, W. F., et al. (2012). Violacein induces death of resistant leukaemia cells via kinome reprogramming, endoplasmic reticulum stress and Golgi apparatus collapse. PLoS One 7, e45362. doi:10.1371/journal.pone.0045362
Rahman, M. M., Islam, M. R., Akash, S., Mim, S. A., Rahaman, M. S., Emran, T. B., et al. (2022). In silico investigation and potential therapeutic approaches of natural products for COVID-19: Computer-aided drug design perspective. Front. Cell. Infect. Microbiol. 12, 929430. doi:10.3389/fcimb.2022.929430
Rivero Berti, I., Rodenak-Kladniew, B. E., Onaindia, C., Islan, G. A., Adam, C., Durán, N., et al. (2020). Assessment of in vitro cytotoxicity of imidazole ionic liquids and inclusion in targeted drug carriers containing violacein. RSC Adv. 10, 29336–29346. doi:10.1039/d0ra05101b
Rivero Berti, I., Rodenak-Kladniew, B. E., Perez, A. A., Santiago, L., Durán, N., and Castro, G. R. (2019). Development of biocarrier for violacein controlled release in the treatment of cancer. React. Funct. Polym. 136, 122–130. doi:10.1016/j.reactfunctpolym.2019.01.001
Rivero Berti, I., Rodenak-Kladniew, , Katz, S. F., Arrúa, E. C., Alvarez, V. A., Duran, N., et al. (2022). Enzymatic active release of violacein present in nanostructured lipid carrier by lipase encapsulated in 3D-bio printed chitosan-hydroxypropyl methylcellulose matrix with anticancer activity. Front. Chem. (Green Sustain. Chem. 10, 914126. doi:10.3389/fchem.2022.914126
Rodrigues, A. L., Göcke, Y., Bolten, C., Brock, N. L., Dickschat, J. S., and Wittmann, C. (2012). Microbial production of the drugs violacein and deoxyviolacein: Analytical development and strain comparison. Biotechnol. Lett. 34, 717–720. doi:10.1007/s10529-011-0827-x
Rodrigues, A. L., Trachtmann, N., Becker, J., Lohanatha, A. F., Blotenberg, J., Bolten, C. J., et al. (2013). Systems metabolic engineering of Escherichia coli for production of the antitumor drugs violacein and deoxyviolacein. Metab. Eng. 20, 29–41. doi:10.1016/j.ymben.2013.08.004
Rosa, A., Pye, V. E., Graham, C., Muir, L., Seow, J., Ng, K. W., et al. (2021). SARS-CoV-2 can recruit a heme metabolite to evade antibody immunity. Sci. Adv. 7 (22), eabg7607. doi:10.1126/sciadv.abg7607
Rosli, N., and Setu, S. A. (2018). Polyvinyl-alcohol/polyvinyl-pyrrolidone membranes coated with violacein pigments as antibacterial agent for wound dressing application. eProceedings Chem. 3 (3), 152–159. doi:10.1007/s00044-011-9654-9
Rudiansyah, M., Jasim, S. A., Mohammad Pour, Z. G., Athar, S. S., Jeda, A. S., Doewes, R. I., et al. (2022). Coronavirus disease 2019 (COVID-19) update: From metabolic reprogramming to immunometabolism. J. Med. virology 94 (10), 4611–4627. doi:10.1002/jmv.27929
Shuster, A., Pechalrieu, D., Jackson, C. B., Abegg, D., Choe, H., and Adibekian, A. (2021). Clinical antiviral drug arabitol inhibits infection by SARS-CoV-2 and variants through direct binding to the spike protein. ACS Chem. Biol. 16 (12), 2845–2851. doi:10.1021/acschembio.1c00756
Subramaniam, S., Ravi, V., and Sivasubramanian, A. (2014). Synergistic antimicrobial profiling of violacein with commercial antibiotics against pathogenic micro-organisms. Pharm. Biol. 52 (1), 86–90. doi:10.3109/13880209.2013.815634
Suryawanshi, R. K., Patil, C. D., Borase, H. P., Narkhede, C. P., Stevenson, A., Hallsworth, J. E., et al. (2015). Towards an understanding of bacterial metabolites prodigiosin and violacein and their potential for use in commercial sunscreens. Int. J. Cosmet. Sci. 37 (1), 98–107. doi:10.1111/ics.12175
Tay, M. Z., Poh, C. M., Rénia, L., MacAry, P. A., and Ng, L. F. P. (2020). The trinity of COVID-19: Immunity, inflammation, and intervention. Nat. Rev. Immunol. 20 (6), 363–374. doi:10.1038/s41577-020-0311-8
Toelzer, C., Gupta, K., Yadav, S. K. N., Borucu, U., Davidson, A. D., Williamson, M. K., et al. (2020). Free fatty acid binding pocket in the locked structure of SARS-CoV-2 spike protein. Science 370 (6517), 725–730. doi:10.1126/science.abd3255
Veber, D. F., Johnson, S. R., Cheng, H. Y., Smith, B. R., Ward, K. W., and Kopple, K. D. (2002). Molecular properties that influence the oral bioavailability of drug candidates. J. Med. Chem. 45, 2615–2623. doi:10.1021/jm020017n
Venegas, F. A., Köllisch, G., Mark, K., Diederich, W. E., Kaufmann, A., Bauer, S., et al. (2019). The bacterial product violacein exerts an immunostimulatory effect via TLR8. Sci. Rep. 9 (1), 13661. doi:10.1038/s41598-019-50038-x
Venil, C. K., Aruldass, C. A., Abd Halim, M. H., Khasim, A. R., Zakaria, Z. A., and Ahmad, W. A. (2015). Spray drying of violet pigment from Chromobacterium violaceum UTM 5 and its application in food model systems. Int. Biodeterior. Biodegrad. 102, 324–329. doi:10.1016/j.ibiod.2015.02.006
Verinaud, L., Lopes, S. C., Naranjo Prado, I. C., Zanucoli, F., Alves da Costa, T., Di Gangi, R., et al. (2015). Violacein treatment modulates acute and chronic inflammation through the suppression of cytokine production and induction of regulatory T cells. PLoS One 10 (5), e0125409. doi:10.1371/journal.pone.0125409
Vishnu, T. S., and Palaniswamy, M. (2018). Systematic approach on evaluating the in vitro antioxidant activity of violacein; novel isolate Chromobacterium Vaccinii CV5. Biomed. Pharmacol. J. 11 (2), 703–709. doi:10.13005/bpj/1423
Volkamer, A., Kuhn, D., Rippmann, F., and Rarey, M. (2012). DoGSiteScorer: A web server for automatic binding site prediction, analysis and druggability assessment. Bioinforma. Oxf. Engl. 28 (15), 2074–2075. doi:10.1093/bioinformatics/bts310
Vollbracht, C., and Kraft, K. (2022). Oxidative stress and hyper-inflammation as major drivers of severe COVID-19 and long COVID: Implications for the benefit of high-dose intravenous vitamin C. Front. Pharmacol. 13, 899198. doi:10.3389/fphar.2022.899198
Wang, H., Jiang, P., Lu, Y., Ruan, Z., Jiang, R., Xing, X., et al. (2009). Optimization of culture conditions for violacein production by a new strain of Duganella sp. B2. Biochem. Eng. J. 44 (2-3), 119–124. doi:10.1016/j.bej.2008.11.008
Wang, Q., Wang, L., Zhang, Y., Zhang, X., Zhang, L., Shang, W., et al. (2022). Probing the allosteric inhibition mechanism of a spike protein using molecular dynamics simulations and active compound identifications. J. Med. Chem. 65 (4), 2827–2835. doi:10.1021/acs.jmedchem.1c00320
WHO (2023). WHO coronavirus (COVID-19) dashboard. Available at: https://covid19.who.int/. [Accessed on 14 2023].
Yañez, O., Osorio, M. I., Uriarte, E., Areche, C., Tiznado, W., Pérez-Donoso, J. M., et al. (2021). In silico study of coumarins and quinolines derivatives as potent inhibitors of SARS-CoV-2 main protease. Front. Chem. 8, 595097. doi:10.3389/fchem.2020.595097
Yang, C., Jiang, P., Xiao, S., Zhang, C., Lou, K., and Xing, X.-H. (2011). Fed-batch fermentation of recombinant Citrobacter freundii with expression of a violacein-synthesizing gene cluster for efficient violacein production from glycerol. Biochem. Eng. J. 57, 55–62. doi:10.1016/j.bej.2011.08.008
Zhu, D. D., Tan, X. M., Lu, L. Q., Yu, S. J., Jian, R. L., Liang, X. F., et al. (2021). Interplay between nuclear factor erythroid 2-related factor 2 and inflammatory mediators in COVID-19-related liver injury. World J. Gastroenterology 27 (22), 2944–2962. doi:10.3748/wjg.v27.i22.2944
Keywords: violacein, COVID-19, membrane interaction, antioxidant activity, anti-inflammatory activity, protease inhibition, violacein antiviral activities
Citation: Rivero Berti I, Gantner ME, Rodriguez S, Islan GA, Fávaro WJ, Talevi A, Castro GR and Durán N (2023) Potential biocide roles of violacein. Front. Nanotechnol. 5:1186386. doi: 10.3389/fnano.2023.1186386
Received: 14 March 2023; Accepted: 16 June 2023;
Published: 21 July 2023.
Edited by:
Raj Kumar, University of Nebraska Medical Center, United StatesReviewed by:
Rahul K. Suryawanshi, Gladstone Institutes, United StatesCopyright © 2023 Rivero Berti, Gantner, Rodriguez, Islan, Fávaro, Talevi, Castro and Durán. This is an open-access article distributed under the terms of the Creative Commons Attribution License (CC BY). The use, distribution or reproduction in other forums is permitted, provided the original author(s) and the copyright owner(s) are credited and that the original publication in this journal is cited, in accordance with accepted academic practice. No use, distribution or reproduction is permitted which does not comply with these terms.
*Correspondence: Guillermo R. Castro, Z3JjYXN0cm9AZ21haWwuY29t; Nelson Durán, bmR1cmFuQHVuaWNhbXAuYnI=
Disclaimer: All claims expressed in this article are solely those of the authors and do not necessarily represent those of their affiliated organizations, or those of the publisher, the editors and the reviewers. Any product that may be evaluated in this article or claim that may be made by its manufacturer is not guaranteed or endorsed by the publisher.
Research integrity at Frontiers
Learn more about the work of our research integrity team to safeguard the quality of each article we publish.