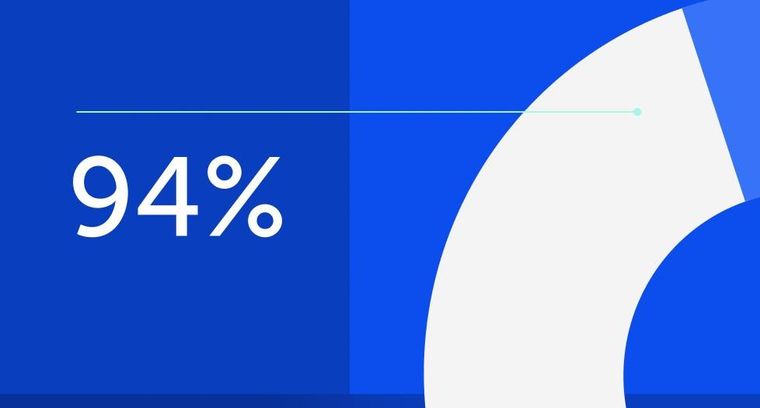
94% of researchers rate our articles as excellent or good
Learn more about the work of our research integrity team to safeguard the quality of each article we publish.
Find out more
REVIEW article
Front. Nanotechnol., 31 August 2022
Sec. Biomedical Nanotechnology
Volume 4 - 2022 | https://doi.org/10.3389/fnano.2022.987034
This article is part of the Research TopicWomen in Nanotechnology, voIume IView all 10 articles
Extracellular vesicles (EVs) are widely recognized as intercellular communication mediators. Among the different biological processes, EVs play a role in viral infections, supporting virus entrance and spread into host cells and immune response evasion. Severe acute respiratory syndrome coronavirus 2 (SARS-CoV-2) infection became an urgent public health issue with significant morbidity and mortality worldwide, being responsible for the current COVID-19 pandemic. Since EVs are implicated in SARS-CoV-2 infection in a morphological and functional level, they have gained growing interest for a better understanding of SARS-CoV-2 pathogenesis and represent possible diagnostic tools to track the disease progression. Furthermore, thanks to their biocompatibility and efficient immune activation, the use of EVs may also represent a promising strategy for the development of new therapeutic strategies against COVID-19. In this review, we explore the role of EVs in viral infections with a focus on SARS-CoV-2 biology and pathogenesis, considering recent morphometric studies. The common biogenesis aspects and structural similarities between EVs and SARS-CoV-2 will be examined, offering a panoramic of their multifaceted interplay and presenting EVs as a machinery supporting the viral cycle. On the other hand, EVs may be exploited as early diagnostic biomarkers and efficient carriers for drug delivery and vaccination, and ongoing studies will be reviewed to highlight EVs as potential alternative therapeutic strategies against SARS-CoV-2 infection.
Severe acute respiratory syndrome coronavirus 2 (SARS-CoV-2) is a novel strain of coronavirus emerged first in China in 2019 and etiological agent of the current coronavirus disease 2019 (COVID-19) outbreak (Kulkarni et al., 2022). Due to its ease of spread, SARS-CoV-2 represents a serious threat to global health, also having a strong socio-economic impact. To date, on 27 June 2022, there have been 540,923,532 confirmed cases of COVID-19, including 6,325,785 deaths, reported to WHO (https://covid19.who.int). The vaccination campaign helped to counteract severe clinical outcomes, but the ability of SARS-CoV-2 to mutate and evade immune responses still implies the need to find new intervention strategies, requiring in turn a deeper understanding of SARS-CoV-2 biology (Boni et al., 2020). Several morphometric studies are ongoing to elucidate SARS-CoV-2 infection mechanisms, especially egress events and cell-to-cell spreading that are still poorly characterized (Klein et al., 2020; Eymieux et al., 2021b; Kumar et al., 2021; Laue et al., 2021; Mendonça et al., 2021). According to these, extracellular vesicles (EVs) trafficking seems to be a deeply involved pathway in SARS-CoV-2 infection, contributing to viral replication, immune evasion, egression from infected cells, and spread to other cell targets, through the so-called “replicative organelles” (Pleet et al., 2018; Bello-Morales et al., 2020). Several hypotheses further support a role of EVs in SARS-CoV-2 infection, like the existence of “quasi-enveloped viruses” (Feng et al., 2014), the “Trojan exosome” hypothesis (Hildreth, 2017), and the “secretory autophagy” model (Bunz et al., 2022), but none of these has been confirmed as the prevalent and further investigations are needed. EVs are well known as membrane-enveloped, nano- and micro-sized particles secreted by different cell types, distinct in their size, composition and function according to their biogenesis, delivering different cargoes to distant targets and thus affecting intercellular signaling (Kalluri and LeBleu, 2020). Thanks to their intrinsic properties, EVs display an important role in a physiological level, as well as in several pathological conditions, being mediators of host-pathogen interactions. Depending on their cargo, EVs may also functionally support or counteract viral infection. Next to supplying a replication machinery, EVs may also transport and present antigens, modulating the host immune responses against the virus (Babaei et al., 2022). The intricate morpho-functional interplay between SARS-CoV-2 and EVs gained particular interest among the scientific community and defining EVs dynamics and cargo may be pivotal to get not only a better comprehension of the viral cycle, but also to take advantage of it, to find new clues for biomarker tracing, disease treatment, and vaccine development. To date, EVs have been exploited to deliver immunomodulatory cargoes, acting prevalently on the cytokine storm occurring in COVID-19, in combination with antiviral drugs (Romagnoli et al., 2015; Somiya et al., 2017). Some clinical trials are currently underway to test the efficacy and safety of EVs formulations, but the results are still fragmentary and incomplete (Karn et al., 2021). Despite the use of EVs for therapeutic purposes seems promising, further investigations are needed to find effective strategies with minimal side effects. In this paper, multiple aspects of the SARS-CoV-2 and EVs network are reviewed, from the role of EVs in SARS-CoV-2 viral cycle and COVID-19 pathogenesis, to their potential as diagnostic biomarkers and tools for treatment, drug delivery and vaccine design.
Historically, EVs have been considered as cellular “junk bags”, assigned to remove unwanted material. To date, EVs have great importance in different biological systems as carriers of biomolecules, such as proteins, lipids, and nucleic acids, playing a crucial role in cell-to-cell communication. Furthermore, EVs have been used as therapeutic drug carriers and as diagnostic tools for several diseases (Dini et al., 2020). EVs are small membrane-enclosed nano- and micro-structures released by different cells and can be found in several human biofluids (i.e., blood, urine, cerebrospinal fluid, saliva, breast milk, bronchoalveolar lavage fluid) (Doyle and Wang, 2019). Due to their heterogeneity, EVs are difficult to categorize. However, according to their biogenesis pathways, EVs are generally distinguished in three main subtypes: exosomes (small EVs, 30–100 nm in size), microvesicles (large EVs, 100–1,000 nm in size), and apoptotic bodies (bigger than 1,000 nm) (Rai et al., 2021). Exosomes, also referred as small EVs, are derived from multivesicular bodies (MVBs) (Yue et al., 2020) and formed by the inward budding of the late endosomal membrane, which encapsulates biomolecules and generates intraluminal vesicles (ILVs) within the MVBs. Then, MVBs fuse with the plasma membrane and release ILVs into the extracellular space in the form of exosomes via exocytosis (Vella et al., 2008). Several peripheral membrane protein complexes, necessary for the formation of ILVs in MVBs and the budding of microvesicles from the plasma membrane, are known as “endosomal sorting complexes required for transport”. In the ESCRT system, there are four complexes known as ESCRT-0, ESCRT-I, ESCRT-II, and ESCRT-III with associated proteins (Tsg101, ALIX, and VPS4) and, during biogenesis, each complex has its own role. To initiate the pathway, ESCRT-0 forms a protein network on endosomal membranes that captures ubiquitinated cargo proteins and allows their sorting based on the MVBs pathway (Raiborg et al., 2001; Mayers et al., 2011). ESCRT-I binds to ubiquitinated cargo proteins on the endosomes and activates ESCRT-II, which plays a direct role in cargo sorting at multivesicular endosomes (MVEs). In turn, ESCRT III, with the charged multivesicular body proteins, performs membrane remodeling, including fission, protein dissociation and recycling of the endosomal sorting machinery, which depends on the participation of the Vps4 AAA-ATPase (Obita et al., 2007). Besides these processes, ESCRT-III and its accessory proteins, like Vps4, Vta1, Vsp60 and ALIX, are also involved in virus budding, cytokinesis and nuclear envelope remodeling (Hurley, 2015). Another pathway for exosome biogenesis involves the syndecan/syntenin complex, which requires the activity of phospholipase D 2 (PLD2) and ADP-ribosylation factor 6 (Arf6) (Tricarico et al., 2016). Despite the central role of the ESCRT-dependent pathway for EVs formation, the SMase2/ceramide pathway, independent from the canonical ESCRT pathway, has been also characterized (Kosaka et al., 2010). For EVs transport and release, distinct effectors are implicated, such as RAB GTPases (Rocha et al., 2009). RAB GTPases regulate trafficking in both the endocytic and secretory pathways, by recruiting specific effector proteins onto membrane surfaces to either facilitate cargo collection, organelle movement, or vesicle docking. There is growing evidence linking RAB GTPases to the secretion of exosomes, mainly RAB5 and RAB7 (Hutagalung & Novick, 2011). Further studies have also demonstrated that RAB27A and RAB27B participate in MVEs transport, while RAB11 and RAB35 proteins regulate MVEs fusion with the plasma membrane and secretion directly or indirectly (van Niel et al., 2018). Furthermore, RAB22A has been found to colocalize with budding microvesicles, participating in cargo sorting in hypoxic breast cancer cells (Wang et al., 2014).
Microvesicles (MVs), also referred as large EVs, are instead formed by the direct plasma membrane budding and fission. Several proteins are involved in MVs biogenesis, such as Ca2+ dependent aminophospholipid translocases, scramblases, and calpains, driving the rearrangement of membrane phospholipids and the reconstitution of the actin cytoskeleton, and finally determining the plasma membrane curvature and MVs release in the extracellular space (Lynch and Ludlam, 2007). Despite the apparent distinct biogenesis modality, exosomes and microvesicles may share common sorting machinery and intracellular mechanisms, often displaying an overlapping size and similar composition that hamper discriminating different subpopulations. Indeed, there is still a need to uncover the exact dynamics of EVs biogenesis (van Niel et al., 2018). Here, we adhere to the nomenclature guidelines published by the International Society of Extracellular Vesicles (ISEV), that suggests the use of the general term “EVs”, as there is still no definitive distinctive marker of each EVs subpopulation (Théry et al., 2018; Witwer et al., 2021).
Apoptotic bodies, or apoptosomes, are formed only during apoptosis, characterized by the condensation of nuclear chromatin and involving nuclear chromatin condensation followed by membrane blebbing (Kerr et al., 1972). As well as ESCRT proteins, tetraspanins (CD81, CD82, CD63, CD9), and proteins responsible for cargo transport and membrane fusion (annexins, RABs), EVs may be enriched in proteins involved in many processes including cell adhesion (integrins), antigen presentation (MHC-I, MHC-II), stress regulation (HSP70, HSP90), cell migration (actin, myosin, tubulin) and metabolic enzymes (Lakkaraju and Rodriguez-Boulan, 2008; Colombo et al., 2014). EVs may also contain several types of nucleic acids, such as messenger RNAs (mRNAs), micro-RNAs (miRNAs), transfer RNAs (tRNA) and long non-coding RNAs (lncRNAs) (Valadi et al., 2007; Gusachenko et al., 2013). The ability of EVs to carry cargo, budding, shedding, and being reacquired by neighboring cells, may contribute to the spread of infections, progression of diseases, as well as the modulation of immune-related processes. In particular, the role of EVs in the pathogenesis of infectious diseases suggests that EVs may serve as potential biomarkers to track mild bacterial infection and chronic viral diseases, with the possibility of harnessing EVs to prevent infection or spread (Jones et al., 2018; Pérez et al., 2019).
Viruses are intracellular pathogens exploiting the cellular compartments to carry out their life cycle. Given the role of EVs in intercellular communication and in pathological disorders, the interplay between viruses and EVs gained a lot of attention, especially for their similarities in structure and biogenesis (Bello-Morales et al., 2020). Indeed, EVs display a multifaceted role in viral infections, contributing in first place to viral replication, dissemination, and immune evasion modulating the host immune system activation. Furthermore, EVs may block (Ipinmoroti and Matthews, 2020) or otherwise trigger the antiviral response, exacerbating the damage and causing several detrimental effects, such as oncogenesis (Zheng et al., 2019), inflammation (Sadri Nahand et al., 2020) or autoimmune responses (Gunasekaran et al., 2020). Several viruses exploit intracellular membrane rearrangements, creating peculiar double-membrane structures known as “replicative organelles”, to complete their replication facilitating new virions formation and budding from the host cell (Wolff et al., 2020). Viral infection seems to have influence on lipid metabolism and processing, driving the formation of protective structures representing the main strategy for a successful viral cycle (Müller et al., 2018). Lipid rafts and myelin and lymphocyte protein (MAL), an integral membrane protein found in cholesterol and glycolipids-enriched membrane, are believed to be implicated in virion uptake, constituting platforms for viral particle assembly (Kulkarni et al., 2022). Besides the formation of replication organelles, viruses must exit the infected cell and disseminate, evading at the same time the host immune system, to ensure their biological success. To accomplish this, both enveloped and non-enveloped viruses exploit EVs as a strategy to maximize their tropism without being recognized by the host immune cells (Schorey et al., 2015). Indeed, EVs may mediate and enhance viral propagation creating the so-called “collective infectious units”, consisting in multiple virions within a single vesicle or multiple viral genomes in a single virion. Through this dispersal strategy, viruses increase their multiplicity of infection (MOI) (Leeks et al., 2019; Andreu-Moreno and Sanjuán, 2020). Whether these vesicles are EVs containing viral molecules or defective viral particles is still under debate but, since EVs and viruses share similar size, density, and composition, it is difficult to isolate and analyze distinct fractions and improved strategies of isolation are needed (van der Grein et al., 2018). An overlap between viral particles release and EVs biogenesis pathway is represented by the “quasi-enveloped” viruses, non-enveloped viruses generally promoting cell lysis for their release and not surrounded by host membrane, which have been found to be enclosed inside vesicles during their propagation. In this phenomenon, viruses take advantage of the host ESCRT machinery to acquire a protective membrane and exit the infected cell without lysis, avoiding then the recognition by the host immune system (Feng et al., 2014). This behavior, first reported in hepatitis A virus (HAV) (Feng et al., 2013; Jiang et al., 2020), was found also in hepatitis E virus (HEV) (Nagashima et al., 2014), Coxsackie B virus (CBV) (Sin et al., 2017), but also in enveloped viruses, like hepatitis C virus (HCV) (Bunz et al., 2022) and others. Furthermore, the “Trojan exosome” hypothesis states that retroviruses, like human immunodeficiency virus (HIV), use EVs biogenesis pathways for their egression, spread and uptake, enhancing their infectivity and modulating host gene expression and immune responses (Hildreth, 2017; Chen et al., 2021). Another model proposes the “secretory autophagy” for virus non-cytolytic egression from the infected cell through LC3-positive vesicles, originating from autophagosomes fusing with the plasma membrane instead of lysosomes (Altan-Bonnet, 2016; Bunz et al., 2022). According to this model, first described by Chen YH et al., multiple viral particles are selectively captured by LC3-positive “autophagosome-like” organelles, enriched in phosphatidylserine lipids, and released non-cytolitically from the infected cells (Chen et al., 2015). Indeed, several pathogens are able to perturbate the autophagic pathway by different mechanisms (Pleet et al., 2018; Zhao et al., 2021). Great evidence suggests that viral infection massively alters EVs composition causing multiple effects depending on the virus-host landscape, for instance supporting viral replication, transmission, and stability by transferring viral RNAs and proteins and by modulating interactions between membranes and receptors, making other cells more susceptible to infection (Bello-Morales and López-Guerrero, 2020; Dubrovsky et al., 2020; Hassanpour et al., 2020). However, EVs trafficking exploited by viruses may also imply differential immune responses helping the host to fight the infection, activating other cell targets (Velandia-Romero et al., 2020), inducing the production of pro-inflammatory molecules (Maemura et al., 2018) and promoting antiviral response (Yao et al., 2018). In conclusion, EVs can exacerbate or counteract viral infection, and sometimes both effects may be caused by the same virus. Since viruses and EVs are closely related in terms of biogenesis, dynamics and mechanisms of action, a deeper understanding of their interplay would be of great importance for translation in therapeutic approaches (Hoen et al., 2016).
Severe acute respiratory syndrome coronavirus 2 (SARS-CoV-2) is an enveloped, positive-sense single-stranded RNA virus (family Coronaviridae, genus betacoronavirus) and causative agent of the running pandemic of coronavirus disease 19 (COVID-19) (V’kovski et al., 2021). Affecting mammals and birds and able to cross between the species, coronaviruses are generally associated with respiratory diseases, from the common cold to severe respiratory pathologies, such as Middle East respiratory syndrome (MERS), severe acute respiratory syndrome (SARS), and COVID-19. It is likely that SARS-CoV-2 has emerged during SARS-CoV evolution, by frequent recombination events occurred between severe acute respiratory syndrome-related coronaviruses coexisting in bats, being then transmitted to humans by an unknown intermediate host (Boni et al., 2020). Like SARS-CoV, SARS-CoV-2 is generally transmitted through respiratory droplets, but also by direct contact with contaminated surfaces and by oro-fecal transmission (Chan et al., 2020; Li et al., 2020). SARS-CoV-2 primarily affects the respiratory tract, causing fever, fatigue, and dry cough as common symptoms. However, SARS-CoV-2 may disseminate into other organs, leading to systemic infection and finally resulting in multiorgan failure (Cao, 2020). For a successful viral cycle, including virus entry, replication, egress and diffusion, SARS-CoV-2 relies on its own structure and on molecular interaction with host factors (Knoops et al., 2010; V’kovski et al., 2019). SARS-CoV-2 virions are composed by structural proteins, namely spike (S), envelope (E), membrane (M), and nucleocapsid (N), ensuring the incorporation of the positive-sense, single-stranded RNA genome (+ssRNA) and thus the viral particle assembly. The SARS-CoV-2 entrance into the host cell requires a specific binding of the S protein to the angiotensin-converting enzyme 2 (ACE2), shared also by SARS-CoV, influencing its tropism and pathogenicity (Lu et al., 2020). The S protein and ACE2 binding affinity determines the severity of infection, and ACE2 is expressed in lungs, gut, kidney, heart, and adipose tissues (Adli et al., 2022). ACE2 receptor is also expressed by platelets, which have been demonstrated to be hyperactivated in COVID-19 patients, being the main cause of the prothrombotic state occurring in SARS-CoV-2 infection (Zhang et al., 2020). Coronavirus S proteins are homotrimeric class I fusion glycoproteins, divided into two functionally distinct domains. The S1 domain, exposed to the surface, constitutes the receptor-binding domain, allowing the specific interaction with the host cell receptor, while the transmembrane S2 domain includes the fusion peptide, enabling the fusion between the viral and cell membranes by conformational rearrangements (Letko et al., 2020). Next to receptor binding, SARS-CoV-2 exploits cell-derived proteases, such as the cell-surface serine protease TMPRSS2, expressed in the human respiratory tract and mediating the S protein proteolytic cleavage, essential to permit the viral-host membrane fusion (Gierer et al., 2013; Hoffmann et al., 2020). The viral genome release into the host cell gives rise to the onset of a finely space and time-regulated gene expression program, which includes several non-structural proteins, involved in the formation of the viral replication and transcription complex, RNA synthesis, proofreading and modification, host cell factors interactions, intracellular membrane remodeling and host immune evasion (Snijder et al., 2016; Thoms et al., 2020). More importantly, non-structural proteins participate in the biogenesis of peculiar viral replication organelles, located at the perinuclear level and creating a protective microenvironment for genomic viral RNA replication, namely double-membrane vesicles (DMVs), convoluted membranes (CMs) and small open double membrane spherules (DMSs) (Knoops et al., 2008; Snijder et al., 2020). Conserved between coronaviruses, these characteristic structures are highly dynamic and, besides constituting a protective barrier to evade the cell cytosolic innate immune sensors, they contribute to the formation of new viral particles at the endoplasmic reticulum (ER)-to-Golgi intermediate compartment (ERGIC) and guide virus egression from the cell, supporting a role of the exocytic pathway in virus spread (Ghosh et al., 2020; Klein et al., 2020). Considering these observations, and since the cellular pathways involved in SARS-CoV-2 progression are still under investigation, vesicular trafficking gained a lot of attention as a mechanism implicated in COVID-19 pathogenesis. In the next paragraphs, the role of EVs in COVID-19 progression, treatment, and diagnosis will be reviewed.
As previously discussed in Introduction Section, EVs are nano- and micro-sized particles present in all body fluids and produced by all cell types, containing several biomolecules (such as proteins, lipids and nucleic acids) which are delivered to other cell targets, reprogramming their function and morphology and thus serving as modulators of intercellular communication (Van Niel et al., 2018; Kalluri and LeBleu, 2020). Given their vectorial properties, EVs are implicated in several pathological conditions (Robbins and Morelli, 2014; Dini et al., 2020; Xiao et al., 2021; Bao et al., 2022), including viral infections (Hassanpour et al., 2020). EVs and viruses share similarities in terms of size, structure, and biochemical composition, using similar biogenesis and membrane fusion pathways (Urciuoli and Peruzzi, 2020). As stated for several other viruses, vesicular structures seem to be first implicated in SARS-CoV-2 infection in a morphological level, participating in virus assembly, egression from infected cells, and dissemination to other cell targets. Similar mechanisms of replication, virion assembly and egression have been found to be shared between SARS-CoV and SARS-CoV-2, since both the coronaviruses may exploit the ERGIC or the Golgi apparatus, to exit from the cell probably through the exocytic pathway (Figure 1) (Knoops et al., 2010; Snijder et al., 2020; Saraste and Prydz, 2021). A recent study suggested that coronaviruses may use lysosomes rather than the secretory pathway, and this hypothesis is further supported by the fact that viral particles have been observed inside lysosomal compartments by Transmission Electron Microscopy (TEM) (Ghosh et al., 2020). However, the exact fusion mechanism between these organelles and the plasma membrane that would allow the virus release in the extracellular space remains unclear. Morphometric and ultrastructure analyses, although still in infancy, have been performed to fill the gap in the knowledge of the SARS-CoV-2 dynamics in its replicative cycle, especially regarding egress events (Klein et al., 2020; Eymieux et al., 2021b; Kumar et al., 2021; Laue et al., 2021; Mendonça et al., 2021). The presence of “exit tunnels”, creating a link between viral particles-rich intracellular structures and the extracellular environment, has been proposed (Mendonça et al., 2021). On the other hand, another group highlighted the presence of morphologically distinct regions within infected cells from which SARS-CoV-2 could egress, including areas with a large cytoplasm invagination and plasma membrane microvilli, plasma membrane portions without prominent rearrangements, and intermediate situations. These regions were characterized by large or small intracellular vesicles, containing several viral particles or a single viral particle, respectively. According to this work, the prevalent egression route exploited by SARS-CoV-2 particles should be through small secretory vesicles crossing invaginated, microvilli-rich regions (Eymieux et al., 2021a; 2021b). Still, further investigations are needed in order to clarify the mechanism driving the exit of such viral particles-containing vesicles into the extracellular space. Vesicular pathways are involved in SARS-CoV-2 infection not only supporting morphologically the viral life cycle, but also in a functional way. It is well known that EVs are involved in several biological processes, maintaining tissue homeostasis, participating in disease progression, and mediating interactions between host and pathogens, including viral infections (Babaei et al., 2022). Viruses may exploit EVs for their entrance, packaging, spreading, and evading immune system responses. As well as for other viral infections, in which EVs play a role in promoting or counteracting viral spread depending on the virus-host landscape, EVs may act positively or negatively towards SARS-CoV-2 infection, according to their cargo. Virus-infected cells display an increase in EVs production, transferring viral nucleic acids, proteins, or entire virions, as well as viral entry receptors, to healthy cells, contributing not only to virus dissemination, but also to modulate target cell immune responses and susceptibility to infection. CD9 tetraspanin and ACE2 receptor are two examples of EVs-included elements contributing to SARS-CoV-2 infection, as CD9 tetraspanin, naturally enriched in EVs membranes, may be implicated in coronavirus fusion events, while the ACE2 receptor can be sorted in the EVs and transferred to target receptor-null cells, making them more permissive to viral infection (van Dongen et al., 2016; Wang et al., 2020). Furthermore, EVs from infected cells can also activate inflammatory responses and induce apoptosis and cytotoxicity, contributing to tissue damage (Urbanelli et al., 2019). On the other hand, the ability of EVs in transporting viral and self-antigens may represent a double-edged sword, allowing the host immune system to recognize the virus and modulate immune responses against the virus itself (Hassanpour et al., 2020). A few studies found an increased circulation of EVs containing lung-associated self-antigens, viral antigens, and 20S proteasome during coronavirus infection, further supporting the hypothesis of a potential implication of EVs also in SARS-CoV-2 dissemination, still under investigation as well as the intracellular vesicular structures supporting viral replication (Gunasekaran et al., 2020; Goodlet et al., 2021). Since EVs derived from infected cells display a distinct molecular signature than healthy cells, characterizing their cargo, as well as their dynamics, may result pivotal for a better understanding of COVID-19 pathogenesis, to add new clues to diagnostic and therapeutic strategies.
FIGURE 1. Different hypotheses have been stated and are still under investigation to define SARS-CoV-2 egression routes. Several morphological studies support a role of EVs in SARS-CoV-2 egression, especially the exocytic pathway, which involves membrane remodeling and formation of peculiar structures, as microvilli-rich or invaginated regions. Another hypothesis is the lysosomal pathway, but the fusion of these organelles with the plasma membrane is still unknown. Created with BioRender.com.
As previously introduced, SARS-CoV-2 infection may lead to a differential clinical picture, from mild symptoms, as fever and cough, to severe, systemic disorders, as acute respiratory distress syndrome (ARDS), septic shock, coagulation disorders and multiorgan failure (Guan et al., 2020). Generally, the host responses to infection are driven by several factors, including disease progression and patients’ genetic factors (Adli et al., 2022). Due to the heterogeneity of COVID-19 patients’ responses, it is hard to detect individuals predisposed to a severe disease. However, the early identification of patients that will face up to serious outcomes results of great matter to improve therapeutic approaches for a reduced mortality (Fujita et al., 2021). In this context, multi-omic approaches are representing the first line strategy to evaluate EVs molecular profiles (Lam et al., 2021). SARS-CoV-2 infection seems to influence EVs cargo, playing a role in inflammation, virus spreading and immunomodulation, and thus displaying a potential relationship with disease severity (Song et al., 2020; Barberis et al., 2021). Different types of extracellular RNAs (exRNAs), including mRNAs, miRNAs, tRNAs, lncRNAs and small nuclear RNAs (snRNAs), have been found to be released during antiviral responses, being incorporated into exosomes or lipoproteic complexes and protected from degradation once in circulation, and thus having effects in mediating host-virus interactions and modulating the host immune responses (Tenoever, 2013). Based on this evidence, a recent study analyzed EVs proteins and exRNAs profiles from a cohort of COVID-19 patients to find correlations between molecular profiles and disease trajectory, using a proteomic approach. Three distinct groups of components (liver damage-related exRNAs, antiviral response-related EVs proteins, and coagulation-related markers) have been revealed as potential early biomarkers for COVID-19, indicating that EVs proteins and exRNAs profiles in patients’ sera may reflect specific host reactions to the infection, as well as disease progression (Fujita et al., 2021). Characterizing the patients’ responses exploiting EVs molecular profile to find biomarkers of disease progression may result of great importance to find therapeutic targets to mitigate SARS-CoV-2 infection effects. To date, no currently available EVs-associated biomarker has been detected for SARS-CoV-2, and, since studies in the diagnostic field are still in infancy, further studies are required.
To date, SARS-CoV-2 infection has no specific antiviral treatment, but several clinical trials are ongoing to find new therapeutic approaches and vaccines exploiting the knowledge of the viral life cycle. The ability of EVs to communicate between cells and organs, protecting bioactive cargoes, being biocompatible, having small sizes and negatively charged surfaces, and crossing biological membranes including the blood-brain barrier (BBB), targeting at the same time specific cells, makes EVs promising therapeutic agents for COVID-19 (Nguyen et al., 2020). Small EVs, also referred as exosomes, have been proposed as a therapeutic option for SARS-CoV-2, being divided into three subtypes: natural exosomes, engineered small exosomes containing ACE2 receptor, and engineered exosomes containing antiviral drugs (Popowski et al., 2021). Recent advances in stem cell therapy are bringing out promising treatment approaches, mitigating inflammation and regenerating lung damage caused by the “cytokine storm syndrome” occurring during COVID-19 infection. Mesenchymal stem cells (MSCs) are widely used in regenerative medicine thanks to their ability to differentiate into several cell types, such as bone, nerve, skin, and muscle cells. MSCs, next to their ability to transdifferentiate into tissue cells, can also produce chemokines, cytokines, growth factors, and EVs (Satija et al., 2009; Zhao et al., 2019; Nikfarjam et al., 2020). In SARS-CoV-2 patients, MSCs present three distinct mechanisms of action, including immune response modulation, homeostasis maintenance and regeneration during lung-specific injuries, and inhibition of inflammation through EVs-mediated release of anti-inflammatory cytokines (Figure 2) (Morrison et al., 2017; Xu et al., 2019). SARS-CoV-2 infection is characterized by the so-called “cytokine storm syndrome”, a massive release of pro-inflammatory cytokines and chemokines. For this reason, the main objective of SARS-CoV-2 treatment is to suppress the immune response induced by the virus, reducing the damage to both alveolar and capillary epithelial cells and allowing the recovery of lung tissue and function. Studies have demonstrated that, in experimental models of acute lung injury and sepsis, MSCs-derived EVs can modulate immune responses by increasing the secretion of anti-inflammatory cytokines (IL-4, IL-10 and TGF-β) and reducing pro-inflammatory factors (TNF-α, IFN-γ, IL-6, IL-17, and IL-1β), being effective against SARS-CoV-2 infection (Devaney et al., 2015; Monsel et al., 2015; Li et al., 2016; Noronha Nc et al., 2019). IL-17-producing T cells are inhibited by MSC-derived EVs by inducing the activation of IL-10-producing regulatory T cells, involved in the suppression of inflammation. Through the release of molecules like TGF-β, MSC-derived EVs suppress CD4+ and CD8+ T cell activation, reducing the inflammation (Álvarez et al., 2018). Treatment with MSCs-derived EVs also significantly reduced the activation and proliferation of NK cells, promoting the survival of alveolar macrophages and polarizing their phenotype from M1 pro-inflammatory to M2 anti-inflammatory by suppressing the NF-κB signaling pathway. MSCs-derived EVs immunomodulatory properties have also been associated with the anti-inflammatory activity of their cargo, such as IDO, HLA-G, PD-L1 and galectin-1 (Blazquez et al., 2014; Galland et al., 2017; Sicco et al., 2017). MSC-derived EVs miRNAs, for instance miR-124–3p, miR-21–5p, miR-146a and miR-145, have been shown to exert epigenetic effects, affecting cell receptor expression and the exchange of genetic material. Among these miRNAs, miR-124–3p binds to P2X ligand-gated ion channel 7 and suppresses oxidative stress and inflammation, miR-21–5p inhibits lung cell apoptosis, and miR-146a affects macrophages polarization. Lastly, by increasing macrophages phagocytosis, miR-145 facilitates a rapid clearance of infection-causing pathogens. These changes also prevent viruses, such as SARS-CoV-2, from invading cell systems (Wang et al., 2018; Hao et al., 2019; Xia et al., 2019). The results of the current studies are promising, but further research is needed to establish safety and efficacy of MSCs-derived EVs to treat and control symptoms associated with COVID-19.
FIGURE 2. Mesenchymal Stem Cells (MSC)-derived exosomes display several mechanisms of action in counteracting SARS-CoV-2 infection, modulating the host immune responses and acting on the “cytokine storm syndrome”. Specifically, treatment with MSC-derived exosomes may promote an anti-inflammatory environment by reducing pro-inflammatory factors and NK cells activation, producing anti-inflammatory factors, and polarizing macrophages towards an M2 phenotype. Created with BioRender.com.
A drug delivery system based on EVs has a great potential to maximize drug loading in the target cells and inhibit any off-target effects. To reduce viral spread and replication in recipient cells, drugs or biomodulators may be delivered via EVs. To stimulate antigen-specific immune responses, antiviral drugs loaded into EVs can be delivered directly to targeted sites, such as the nasal mucosa and lungs, to exert immunomodulatory effects. As a result of the encapsulation strategy, drugs are delivered to targeted organs more efficiently, and the toxicity associated with native drugs are minimized. To improve the efficiency and specificity of EVs-mediated delivery, there are two methodological approaches for drug loading into EVs, namely exogenous and endogenous (Romagnoli et al., 2015; Somiya et al., 2017). Depending on drug size, shape, and treatment needs, specific loading methods are selected for each drug of interest. Endogenous loading can be achieved by incubating drugs with disease-targeted cell lines, so drug internalization occurs by natural sorting. Then, cells can produce EVs containing the drug of interest, allowing to obtain large quantities of drug-loaded EVs (Vader et al., 2016). On the other hand, the exogenous approach consists in a therapeutic agent, mostly synthetic molecules, or small biomolecules, that can be included into EVs previously isolated from cells or plasma. EVs loading may be either passive or active. Passive loading occurs with the incubation of EVs with therapeutic molecules without further stimulation. Conversely, hydrophilic molecules, including nucleic acids and proteins, cannot spontaneously penetrate into EVs and, therefore, physically or chemically induced active loading is required. Physically induced loading utilizes sonication, electroporation, freeze-thaw, and extrusion methods. The membrane of EVs can also be permeated with chemicals, such as saponin and transfection reagents (Fuhrmann et al., 2015; Janas et al., 2015; Lener et al., 2015; Mantel et al., 2016). In the case of SARS-CoV-2, protease inhibitors (PIs), like anti-HIV PIs, or other antiviral drugs can be encapsulated by endogenous loading technique in Vero CCL-81, Vero E6, and/or STAT1 knock-out cell lines, which can be infected by the virus. In addition, PIs can also be loaded exogenously into EVs isolated from patients’ plasma for personalized therapy (Harcourt et al., 2020). Currently, only a few clinical trials are ongoing to test EVs formulations for therapeutic purposes towards COVID-19 (Karn et al., 2021). The Direct Biologics company, for instance, has promoted and tested in 24 COVID-19 patients an exosome-based drug derived from bone marrow MSCs (ExoFlo™). A single dose of this formulation came out to be safe and without severe side effects in all the patients, reducing inflammatory markers and rising oxygen levels (Sengupta et al., 2020). Despite the promising results of ExoFlo™, the biological activity, dose concentrations, and long-term effects should be also considered for therapeutic purposes, as well as for other EVs formulations. Furthermore, more EVs-based clinical trials on a larger number of COVID-19 patients must take place to assess their therapeutic relevance (Lim et al., 2020).
Virus entry into cells is determined by the receptor-binding domain of SARS-CoV-2 spike protein that specifically binds to ACE2. For this reason, the main potential strategy for vaccine and small drug molecule development against COVID-19 is to block the receptor binding domain (RBD) and ACE2 association sites (Benítez-Cardoza and Vique-Sánchez, 2020; Tai et al., 2020). As stated before, next to their role in facilitating cell-to-cell communication, EVs can also induce an immune response due to their ability in presenting antigens. Therefore, EVs can be engineered to display viral antigens, evoking strong immune responses, and acting as a novel vaccine strategy. To date, several vaccines have been approved for SARS-CoV-2 treatment but, in the face of the emerging SARS-CoV-2 variants and the pandemic prosecution, there is still an urgent need to develop new, efficient vaccine strategies (Tregoning et al., 2021). As an alternative to viral attenuated and inactivated vaccines, viral Spike proteins represent the main target for vaccines realized with different molecular approaches, such as viral-vector-based vaccines (particularly adenoviruses), mRNA vaccines, or vaccines with the full-length S protein or a RBD (Ghaebi et al., 2020). However, when a viral infection is present or when a vaccine against viral antigens is given, a phenomenon called “antibody-dependent enhancement” (ADE) may occur, resulting in an increased burden of the disease. In this context, the recognition and binding of viral particles by antibodies may promote the virus entry and replication in target cells, amplifying an infection rather than protecting from it. To avoid the possibility of side effects with an EVs-based vaccine, it is important to investigate the safety of EVs-producing cells employed for therapeutic purposes. Many studies have shown that human embryonic kidney HEK293T cells are a safe source for EVs production for therapeutic purposes, since they do not express disease markers (Arvin et al., 2020; van Doremalen et al., 2020; Greinacher et al., 2021; Kim et al., 2021). Two types of EVs-based vaccines have been developed to stimulate a long-term immune response to SARS-CoV-2 (Figure 3). The first type consists in HEK293T cells transfected with vectors encoding the four SARS-CoV-2 structural proteins (Spike, Nucleocapsid, Membrane, Envelope). Transfected cells, in turn, produce EVs enriched with viral proteins that may trigger immune responses. It has been shown that immunizing with multiple protein forms allows the modulation of the magnitude and nature of the immune response through cytokines production and Th1 or Th2 stimulation (Morel et al., 2004). The second HEK293T-derived EVs vaccine implies the direct loading of EVs with different mRNAs, encoding modified SARS-CoV-2 Nucleocapsid, Membrane, and Envelope proteins, and the full-length Spike of Wuhan-1 (SW1) isolate, all inserted in the extracellular domain of human Lysosomal Associated Membrane Protein 1 (LAMP1). This protein undergoes degradation into peptides for antigen presentation, inducing the immune response. The LSNME/SW1 vaccine induced a significant increase in both CD4+ and CD8+ T cell proliferation upon addition of N and S recombinant proteins to splenocyte culture medium, confirming the effectiveness of this formulation in triggering cellular immunity (Tsai et al., 2021). Additionally, an EVs-derived vaccine containing the antitumoral cytokine IL-12 from HEK293T cells has been tested in a Phase I trial (Lewis et al., 2021). To improve the immune responses generated by EVs-based vaccines, it might be worth to consider using modified mRNAs encoding for the prefusion conformation of the Spike protein, as has been done by Pfizer/BioNTech and Moderna. To fuse with the host cell membrane, Spike protein undergoes structural rearrangements, from an unstable pre-fusion conformation to a highly stable post-fusion conformation, and there has been indication that stabilizing prefusion-immunogens, preserving epitopes, offers a promising vaccine strategy for enveloped viruses. As part of the prefusion-stabilization process, Moderna substituted prolines in the SARS-CoV-2 S protein residues 986 and 987, and started the production of the mRNA-LNP for the SARS-CoV-2 S (2P) protein (mRNA-1273) (Corbett et al., 2020). Pfizer/BioNTech also developed vaccines using S protein mRNA (2P), although with further modifications. For instance, their vaccine consists of a lipid-nanoparticle containing a N-methyl-pseudouridine (m1Ψ) nucleoside-modified mRNA, encoding for an S protein (2P) that contains a native furin-cleavage site resulting in two fragments (Bansal et al., 2021; Dooley et al., 2021). The fact that this recombinant trimeric S (2P) protein can bind the human ACE2 receptor and human anti-RBD antibodies with high affinity clearly demonstrates its structure and function (Vogel et al., 2020). Furthermore, EVs can be used at high concentrations without adverse effects on cells or animals, so their future use in dosing regimens that require ongoing repeated injections is promising.
FIGURE 3. Next to being mediators of intercellular communication, EVs can present antigens and induce immune responses, and then being engineered to express viral proteins and used as vaccines. The methods currently used to realize EVs-based vaccines are the transfection of HEK293T cells with vectors expressing SARS-CoV-2 structural proteins, then expressed by EVs that are recognized by the host immune system, or the direct loading of mRNAs encoding for the SARS-CoV-2 proteins into EVs, that are then transferred to recipient cells that, in turn, are recognized by the host immune system. Created with BioRender.com.
EVs are nano- and micro-sized particles released by several cell types and capable of trespassing biological membranes, protecting and delivering their cargo to other targets. For this reason, EVs have been recognized as intercellular signaling mediators in both physiological and pathological conditions, including in virus-host interactions (Kalluri and LeBleu, 2020). Viruses and EVs share some similarities in terms of morphology and biogenesis, so EVs trafficking is now gaining growing emphasis in the study of viral infections, such as COVID-19 (V’kovski et al., 2021). Several morphological studies highlighted the involvement of EVs during SARS-CoV-2 infection, in which the virus exploits intracellular membranes forming the so-called “replicative organelles”. SARS-CoV-2 would take advantage of these structures to enhance its replication within the infected cell, but also to maximize its multiplicity of infection, and thus its diffusion, to other cells, creating “collective infectious units” as previously described (Bello-Morales et al., 2020; Eymieux et al., 2021b). However, concerning the mechanisms through which SARS-CoV-2 virions inside vesicular structures egress from infected cells and spread towards other cell targets, several hypotheses have been proposed, but there are still discrepancies and further investigations are needed (Klein et al., 2020; Eymieux et al., 2021b; Kumar et al., 2021; Laue et al., 2021; Mendonça et al., 2021). The contribute of EVs in SARS-CoV-2 infection occurs not only at a morphological level, but also functionally. Indeed, in relationship with their cargo, EVs may exert beneficial or detrimental effects towards SARS-CoV-2 infection, promoting viral spread (Wang et al., 2020) or stimulating host immune responses towards the virus (Hassanpour et al., 2020), respectively. Further studies to elucidate the morphological and functional role of EVs in SARS-CoV-2 infection, especially in terms of EVs dynamics that can be characterized by microscopy methods, and definition of their molecular profiles that can be achieved by multi-omics approaches, are needed for a better understanding of SARS-CoV-2 infection process and a potential exploiting EVs pathway as a target for COVID-19 in diagnosis and treatment applications. Indeed, EVs intrinsic properties and their potential to maximize drug delivery, reducing at the same time the risks of off-targets, make them appealing as tools for therapeutic applications, such as drug delivery and vaccine development. However, there are some limitations that must be considered. The heterogeneity of EVs populations and the lack of standardized protocols for their isolation and downstream processing make it challenging to predict their effects for therapeutic applications. For this reason, it is pivotal to set up proper experimental conditions in pre-clinical models, recapitulating in the best way the physiopathological state of interest, and to consider that the isolation of a heterogeneous population of EVs may provide a more precise overview of their synergic effects respect to the purification of single subpopulations, which may offer instead unreliable information. Concerning clinical trials, MSCs-derived EVs displayed positive effects in reducing the cytokine storm characterizing SARS-CoV-2 infection (Devaney et al., 2015; Monsel et al., 2015; Li et al., 2016; Noronha Nc et al., 2019). Despite the encouraging potential of EVs-based treatment for COVID-19 and the fact that several companies are developing new EVs-based formulations (Karn et al., 2021), there is still a paucity of data and clinical trials need to be improved, assessing next to the effectiveness of the system also the safety and long-term effects. Since EVs display several benefits, such as reduced off-targets, minimal immunogenicity, the ability to transport drugs and high biocompatibility, they still represent a valid alternative to offer new perspectives in the diagnostic and therapeutic field. In conclusion, EVs display an intricate role in SARS-CoV-2 pathogenesis, diagnosis, and treatment (summarized in Table 1). In light of these observations, a deeper understanding of the role of EVs in SARS-CoV-2 pathogenesis, next to improved pre-clinical and clinical studies for an improvement of their use in the diagnostic and therapeutic field, may offer a new weapon to face the emerging SARS-CoV-2 variants that are contributing to an unceasing pandemic.
Conceptualization, CS, DV, and LD; writing-original draft preparation, CS and DV; graphics, LB; writing-review and editing final draft, ST and LD.
The authors declare that the research was conducted in the absence of any commercial or financial relationships that could be construed as a potential conflict of interest.
All claims expressed in this article are solely those of the authors and do not necessarily represent those of their affiliated organizations, or those of the publisher, the editors and the reviewers. Any product that may be evaluated in this article, or claim that may be made by its manufacturer, is not guaranteed or endorsed by the publisher.
Adli, A., Rahimi, M., Khodaie, R., Hashemzaei, N., and Hosseini, S. M. (2022). Role of genetic variants and host polymorphisms on COVID-19: From viral entrance mechanisms to immunological reactions. J. Med. Virol. 94, 1846–1865. doi:10.1002/JMV.27615
Altan-Bonnet, N. (2016). Extracellular vesicles are the Trojan horses of viral infection. Curr. Opin. Microbiol. 32, 77–81. doi:10.1016/J.MIB.2016.05.004
Álvarez, V., Sánchez-Margallo, F. M., Macías-García, B., Gómez-Serrano, M., Jorge, I., Vázquez, J., et al. (2018). The immunomodulatory activity of extracellular vesicles derived from endometrial mesenchymal stem cells on CD4+ T cells is partially mediated by TGFbeta. J. Tissue Eng. Regen. Med. 12, 2088–2098. doi:10.1002/TERM.2743
Andreu-Moreno, I., and Sanjuán, R. (2020). Collective viral spread mediated by virion aggregates promotes the evolution of defective interfering particles. MBio 11, e02156-19. doi:10.1128/MBIO.02156-19
Arvin, A. M., Fink, K., Schmid, M. A., Cathcart, A., Spreafico, R., Havenar-Daughton, C., et al. (2020). A perspective on potential antibody-dependent enhancement of SARS-CoV-2. Nature 584, 353–363. doi:10.1038/s41586-020-2538-8
Babaei, G., Zare, N., Mihanfar, A., and Ansari, M. H. K. (2022). Exosomes and COVID-19: Challenges and opportunities. Comp. Clin. Path. 31, 347–354. doi:10.1007/S00580-021-03311-3
Bansal, S., Perincheri, S., Fleming, T., Poulson, C., Tiffany, B., Bremner, R. M., et al. (2021). Cutting edge: Circulating exosomes with covid spike protein are induced by BNT162b2 (Pfizer–BioNTech) vaccination prior to development of antibodies: A novel mechanism for immune activation by mRNA vaccines. J. I. 207, 2405–2410. doi:10.4049/JIMMUNOL.2100637
Bao, Q., Huang, Q., Chen, Y., Wang, Q., Sang, R., Wang, L., et al. (2022). Tumor-derived extracellular vesicles regulate cancer progression in the tumor microenvironment. Front. Mol. Biosci. 8, 796385. doi:10.3389/FMOLB.2021.796385
Barberis, E., Vanella, V. V., Falasca, M., Caneapero, V., Cappellano, G., Raineri, D., et al. (2021). Circulating exosomes are strongly involved in SARS-CoV-2 infection. Front. Mol. Biosci. 8, 632290. doi:10.3389/FMOLB.2021.632290
Bello-Morales, R., and López-Guerrero, J. A. (2020). Isolation/Analysis of extracellular microvesicles from HSV-1-Infected cells. Methods Mol. Biol. 2060, 305–317. doi:10.1007/978-1-4939-9814-2_17
Bello-Morales, R., Ripa, I., and López-Guerrero, J. A. (2020). Extracellular vesicles in viral spread and antiviral response. Viruses 12, 623. doi:10.3390/V12060623
Benítez-Cardoza, C. G., and Vique-Sánchez, J. L. (2020). Potential inhibitors of the interaction between ACE2 and SARS-CoV-2 (RBD), to develop a drug. Life Sci. 256, 117970. doi:10.1016/J.LFS.2020.117970
Blazquez, R., Sanchez-Margallo, F. M., de la Rosa, O., Dalemans, W., Álvarez, V., Tarazona, R., et al. (2014). Immunomodulatory potential of human adipose mesenchymal stem cells derived exosomes on in vitro stimulated T cells. Front. Immunol. 5, 556. doi:10.3389/FIMMU.2014.00556
Boni, M. F., Lemey, P., Jiang, X., Lam, T. T. Y., Perry, B. W., Castoe, T. A., et al. (2020). Evolutionary origins of the SARS-CoV-2 sarbecovirus lineage responsible for the COVID-19 pandemic. Nat. Microbiol. 511 (5), 1408–1417. doi:10.1038/s41564-020-0771-4
Bunz, M., Ritter, M., and Schindler, M. (2022). HCV egress - unconventional secretion of assembled viral particles. Trends Microbiol. 30, 364–378. doi:10.1016/J.TIM.2021.08.005
Cao, X. (2020). COVID-19: Immunopathology and its implications for therapy. Nat. Rev. Immunol. 20, 269–270. doi:10.1038/s41577-020-0308-3
Chan, J. F. W., Yuan, S., Kok, K. H., To, K. K. W., Chu, H., Yang, J., et al. (2020). A familial cluster of pneumonia associated with the 2019 novel coronavirus indicating person-to-person transmission: A study of a family cluster. Lancet 395, 514–523. doi:10.1016/S0140-6736(20)30154-9
Chen, J., Li, C., Li, R., Chen, H., Chen, D., and Li, W. (2021). Exosomes in HIV infection. Curr. Opin. HIV AIDS 16, 262–270. doi:10.1097/COH.0000000000000694
Chen, Y. H., Du, W., Hagemeijer, M. C., Takvorian, P. M., Pau, C., Cali, A., et al. (2015). Phosphatidylserine vesicles enable efficient en bloc transmission of enteroviruses. Cell 160 (4), 619–630. doi:10.1016/j.cell.2015.01.032
Colombo, M., Raposo, G., and Théry, C. (2014). Biogenesis, secretion, and intercellular interactions of exosomes and other extracellular vesicles. Annu. Rev. Cell Dev. Biol. 30, 255–289. doi:10.1146/ANNUREV-CELLBIO-101512-122326
Corbett, K. S., Edwards, D. K., Leist, S. R., Abiona, O. M., Boyoglu-Barnum, S., Gillespie, R. A., et al. (2020). SARS-CoV-2 mRNA vaccine design enabled by prototype pathogen preparedness. Nature 586, 567–571. doi:10.1038/s41586-020-2622-0
Devaney, J., Horie, S., Masterson, C., Elliman, S., Barry, F., O’Brien, T., et al. (2015). Human mesenchymal stromal cells decrease the severity of acute lung injury induced by E. coli in the rat. Thorax 70, 625–635. doi:10.1136/THORAXJNL-2015-206813
Dini, L., Tacconi, S., Carata, E., Tata, A. M., Vergallo, C., and Panzarini, E. (2020). Microvesicles and exosomes in metabolic diseases and inflammation. Cytokine Growth Factor Rev. 51, 27–39. doi:10.1016/J.CYTOGFR.2019.12.008
Dooley, K., McConnell, R. E., Xu, K., Lewis, N. D., Haupt, S., Youniss, M. R., et al. (2021). A versatile platform for generating engineered extracellular vesicles with defined therapeutic properties. Mol. Ther. 29, 1729–1743. doi:10.1016/J.YMTHE.2021.01.020
Doyle, L. M., and Wang, M. Z. (2019). Overview of extracellular vesicles, their origin, composition, purpose, and methods for exosome isolation and analysis. Cells 8, 727. doi:10.3390/CELLS8070727
Dubrovsky, L., Ward, A., Choi, S. H., Pushkarsky, T., Brichacek, B., Vanpouille, C., et al. (2020). Inhibition of HIV replication by apolipoprotein A-I binding protein targeting the lipid rafts. MBio 11, e02956. doi:10.1128/MBIO.02956-19
Eymieux, S., Rouillé, Y., Terrier, O., Seron, K., Blanchard, E., Rosa-Calatrava, M., et al. (2021a). Ultrastructural modifications induced by SARS-CoV-2 in Vero cells: A kinetic analysis of viral factory formation, viral particle morphogenesis and virion release. Cell. Mol. Life Sci. 78, 3565–3576. doi:10.1007/S00018-020-03745-Y
Eymieux, S., Uzbekov, R., Rouillé, Y., Blanchard, E., Hourioux, C., Dubuisson, J., et al. (2021b). Secretory vesicles are the principal means of SARS-CoV-2 egress. Cells 10, 2047. doi:10.3390/CELLS10082047
Feng, Z., Hensley, L., McKnight, K. L., Hu, F., Madden, V., Ping, L., et al. (2013). A pathogenic picornavirus acquires an envelope by hijacking cellular membranes. Nature 496, 367–371. doi:10.1038/nature12029
Feng, Z., Hirai-Yuki, A., McKnight, K. L., and Lemon, S. M. (2014). Naked viruses that aren’t always naked: Quasi-enveloped agents of acute hepatitis. Annu. Rev. Virol. 1, 539–560. doi:10.1146/annurev-virology-031413-08535910.1146/ANNUREV-VIROLOGY-031413-085359
Fuhrmann, G., Serio, A., Mazo, M., Nair, R., and Stevens, M. M. (2015). Active loading into extracellular vesicles significantly improves the cellular uptake and photodynamic effect of porphyrins. J. Control. Release 205, 35–44. doi:10.1016/J.JCONREL.2014.11.029
Fujita, Y., Hoshina, T., Matsuzaki, J., Yoshioka, Y., Kadota, T., Hosaka, Y., et al. (2021). Early prediction of COVID-19 severity using extracellular vesicle COPB2. J. Extracell. Vesicles 10, e12092. doi:10.1002/JEV2.12092
Galland, S., Vuille, J., Martin, P., Letovanec, I., Caignard, A., Fregni, G., et al. (2017). Tumor-derived mesenchymal stem cells use distinct mechanisms to block the activity of natural killer cell subsets. Cell Rep. 20, 2891–2905. doi:10.1016/J.CELREP.2017.08.089
Ghaebi, M., Osali, A., Valizadeh, H., Roshangar, L., and Ahmadi, M. (2020). Vaccine development and therapeutic design for 2019-nCoV/SARS-CoV-2: Challenges and chances. J. Cell. Physiol. 235, 9098–9109. doi:10.1002/JCP.29771
Ghosh, S., Dellibovi-Ragheb, T. A., Kerviel, A., Pak, E., Qiu, Q., Fisher, M., et al. (2020). β-Coronaviruses use lysosomes for egress instead of the biosynthetic secretory pathway. Cell 183, 1520–1535.e14. doi:10.1016/J.CELL.2020.10.039
Gierer, S., Bertram, S., Kaup, F., Wrensch, F., Heurich, A., Krämer-Kühl, A., et al. (2013). The spike protein of the emerging betacoronavirus EMC uses a novel coronavirus receptor for entry, can Be activated by TMPRSS2, and is targeted by neutralizing antibodies. J. Virol. 87, 5502–5511. doi:10.1128/JVI.00128-13
Goodlet, K. J., Bansal, S., Arjuna, A., Nailor, M. D., Buddhdev, B., Abdelrazek, H., et al. (2021). COVID-19 in a lung transplant recipient: Exploring the diagnostic role of circulating exosomes and the clinical impact of advanced immunosuppression. Transpl. Infect. Dis. 23, e13480. doi:10.1111/TID.13480
Greinacher, A., Thiele, T., Warkentin, T. E., Weisser, K., Kyrle, P. A., and Eichinger, S. (2021). Thrombotic thrombocytopenia after ChAdOx1 nCov-19 vaccination. N. Engl. J. Med. 384, 2092–2101. doi:10.1056/NEJMOA2104840
Guan, W., Ni, Z., Hu, Y., Liang, W., Ou, C., He, J., et al. (2020). Clinical characteristics of coronavirus disease 2019 in China. N. Engl. J. Med. 382, 1708–1720. doi:10.1056/NEJMOA2002032
Gunasekaran, M., Bansal, S., Ravichandran, R., Sharma, M., Perincheri, S., Rodriguez, F., et al. (2020). Respiratory viral infection in lung transplantation induces exosomes that trigger chronic rejection. J. Heart Lung Transpl. 39, 379–388. doi:10.1016/J.HEALUN.2019.12.009
Gusachenko, O. N., Zenkova, M. A., and Vlassov, V. V. (2013). Nucleic acids in exosomes: Disease markers and intercellular communication molecules. Biochem. Mosc. 78, 1–7. doi:10.1134/S000629791301001X
Hao, Q., Gudapati, V., Monsel, A., Park, J. H., Hu, S., Kato, H., et al. (2019). Mesenchymal stem cell–derived extracellular vesicles decrease lung injury in mice. J. I. 203, 1961–1972. doi:10.4049/JIMMUNOL.1801534
Harcourt, J., Tamin, A., Lu, X., Kamili, S., Kumar Sakthivel, S., Murray, J., et al. (2020). Isolation and characterization of SARS-CoV-2 from the first US COVID-19 patient. bioRxiv. 2020, 2020.03.02.972935. doi:10.1101/2020.03.02.972935
Hassanpour, M., Rezaie, J., Nouri, M., and Panahi, Y. (2020). The role of extracellular vesicles in COVID-19 virus infection. Infect. Genet. Evol. 85, 104422. doi:10.1016/J.MEEGID.2020.104422
Hildreth, J. E. K. (2017). HIV as trojan exosome: Immunological paradox explained? Front. Immunol. 8, 1715. doi:10.3389/FIMMU.2017.01715
Hoen, E. N., Cremer, T., Gallo, R. C., and Margolis, L. B. (2016). Extracellular vesicles and viruses: Are they close relatives? Proc. Natl. Acad. Sci. U. S. A. 113, 9155–9161. doi:10.1073/PNAS.1605146113
Hoffmann, M., Kleine-Weber, H., Schroeder, S., Krüger, N., Herrler, T., Erichsen, S., et al. (2020). SARS-CoV-2 cell entry depends on ACE2 and TMPRSS2 and is blocked by a clinically proven protease inhibitor. Cell 181, 271–280.e8. doi:10.1016/j.cell.2020.02.052
Hutagalung, A. H., and Novick, P. J. (2011). Role of Rab GTPases in membrane traffic and cell physiology. Physiol. Rev. 91 (1), 119–149. doi:10.1152/physrev.00059.2009
Ipinmoroti, A. O., and Matthews, Q. L. (2020). Extracellular vesicles: Roles in human viral infections, immune-diagnostic, and therapeutic applications. Pathogens 20209, 1056. doi:10.3390/PATHOGENS9121056
Janas, T., Janas, M. M., Sapoń, K., and Janas, T. (2015). Mechanisms of RNA loading into exosomes. FEBS Lett. 589, 1391–1398. doi:10.1016/J.FEBSLET.2015.04.036
Jiang, W., Ma, P., Deng, L., Liu, Z., Wang, X., Liu, X., et al. (2020). Hepatitis A virus structural protein pX interacts with ALIX and promotes the secretion of virions and foreign proteins through exosome-like vesicles. J. Extracell. Vesicles 9, 1716513. doi:10.1080/20013078.2020.1716513
Jones, L. B., Bell, C. R., Bibb, K. E., Gu, L., Coats, M. T., and Matthews, Q. L. (2018). Pathogens and their effect on exosome biogenesis and composition. Biomedicines 6, 79. doi:10.3390/BIOMEDICINES6030079
Kalluri, R., and LeBleu, V. S. (2020). The biology, function, and biomedical applications of exosomes. Science 80, eaau6977. doi:10.1126/SCIENCE.AAU6977
Karn, V., Ahmed, S., Tsai, L. W., Dubey, R., Ojha, S., Singh, H. N., et al. (2021). Extracellular vesicle-based therapy for COVID-19: Promises, challenges and future prospects. Biomedicines 9 (10), 1373. doi:10.3390/biomedicines9101373
Kerr, J. F. R., Wyllie, A. H., and Currie, A. R. (1972). Apoptosis: A basic biological phenomenon with wideranging implications in tissue kinetics. Br. J. Cancer 26, 239–257. doi:10.1038/bjc.1972.33
Kim, J., Song, Y., Park, C. H., and Choi, C. (2021). Platform technologies and human cell lines for the production of therapeutic exosomes. Extracell. Vesicles Circ. Nucl. Acids 2, 3–17. doi:10.20517/EVCNA.2020.01
Klein, S., Cortese, M., Winter, S. L., Wachsmuth-Melm, M., Neufeldt, C. J., Cerikan, B., et al. (2020). SARS-CoV-2 structure and replication characterized by in situ cryo-electron tomography. Nat. Commun. 11, 5885–5910. doi:10.1038/s41467-020-19619-7
Knoops, K., Kikkert, M., Van Den Worm, S. H. E., Zevenhoven-Dobbe, J. C., Van Der Meer, Y., Koster, A. J., et al. (2008). SARS-coronavirus replication is supported by a reticulovesicular network of modified endoplasmic reticulum. PLoS Biol. 6, e226. doi:10.1371/JOURNAL.PBIO.0060226
Knoops, K., Swett-Tapia, C., van den Worm, S. H. E., te Velthuis, A. J. W., Koster, A. J., Mommaas, A. M., et al. (2010). Integrity of the early secretory pathway promotes, but is not required for, severe acute respiratory syndrome coronavirus RNA synthesis and virus-induced remodeling of endoplasmic reticulum membranes. J. Virol. 84, 833–846. doi:10.1128/JVI.01826-09
Kosaka, N., Iguchi, H., Yoshioka, Y., Takeshita, F., Matsuki, Y., and Ochiya, T. (2010). Secretory mechanisms and intercellular transfer of microRNAs in living cells. J. Biol. Chem. 285, 17442–17452. doi:10.1074/JBC.M110.107821
Kulkarni, R., Wiemer, E. A. C., and Chang, W. (2022). Role of lipid rafts in pathogen-host interaction - a mini review. Front. Immunol. 12, 815020. doi:10.3389/FIMMU.2021.815020
Kumar, B., Hawkins, G. M., Kicmal, T., Qing, E., Timm, E., and Gallagher, T. (2021). Assembly and entry of severe acute respiratory syndrome coronavirus 2 (SARS-CoV2): Evaluation using virus-like particles. Cells 10, 853. doi:10.3390/cells10040853
Lakkaraju, A., and Rodriguez-Boulan, E. (2008). Itinerant exosomes: Emerging roles in cell and tissue polarity. Trends Cell Biol. 18, 199–209. doi:10.1016/J.TCB.2008.03.002
Lam, S. M., Zhang, C., Wang, Z., Ni, Z., Zhang, S., Yang, S., et al. (2021). A multi-omics investigation of the composition and function of extracellular vesicles along the temporal trajectory of COVID-19. Nat. Metab. 3 (7), 909–922. doi:10.1038/s42255-021-00425-4
Laue, M., Kauter, A., Hoffmann, T., Möller, L., Michel, J., and Nitsche, A. (2021). Morphometry of SARS-CoV and SARS-CoV-2 particles in ultrathin plastic sections of infected Vero cell cultures. Sci. Rep. 11, 3515–3611. doi:10.1038/s41598-021-82852-7
Leeks, A., Sanjuán, R., and West, S. A. (2019). The evolution of collective infectious units in viruses. Virus Res. 265, 94–101. doi:10.1016/J.VIRUSRES.2019.03.013
Lener, T., Gimona, M., Aigner, L., Börger, V., Buzas, E., Camussi, G., et al. (2015). Applying extracellular vesicles based therapeutics in clinical trials – An ISEV position paper. J. Extracell. Vesicles 4, 30087. doi:10.3402/JEV.V4.30087
Letko, M., Marzi, A., and Munster, V. (2020). Functional assessment of cell entry and receptor usage for SARS-CoV-2 and other lineage B betacoronaviruses. Nat. Microbiol. 54 (5), 562–569. doi:10.1038/s41564-020-0688-y
Lewis, N. D., Sia, C. L., Kirwin, K., Haupt, S., Mahimkar, G., Zi, T., et al. (2021). Exosome surface display of IL12 results in tumor-retained pharmacology with superior potency and limited systemic exposure compared with recombinant IL12. Mol. Cancer Ther. 20, 523–534. doi:10.1158/1535-7163.MCT-20-0484
Li, Q., Guan, X., Wu, P., Wang, X., Zhou, L., Tong, Y., et al. (2020). Early transmission dynamics in wuhan, China, of novel coronavirus–infected pneumonia. N. Engl. J. Med. 382, 1199–1207. doi:10.1056/NEJMOA2001316
Li, Y., Xu, J., Shi, W., Chen, C., Shao, Y., Zhu, L., et al. (2016). Mesenchymal stromal cell treatment prevents H9N2 avian influenza virus-induced acute lung injury in mice. Stem Cell Res. Ther. 7, 159–211. doi:10.1186/S13287-016-0395-Z
Lim, S. K., Giebel, B., Weiss, D. J., Witwer, K. W., and Rohde, E. (2020). Re: "Exosomes Derived from Bone Marrow Mesenchymal Stem Cells as Treatment for Severe COVID-19" by Sengupta et al. Stem cells Dev. 29 (14), 877–878. doi:10.1089/scd.2020.0089
Lu, R., Zhao, X., Li, J., Niu, P., Yang, B., Wu, H., et al. (2020). Genomic characterisation and epidemiology of 2019 novel coronavirus: Implications for virus origins and receptor binding. Lancet 395, 565–574. doi:10.1016/S0140-6736(20)30251-8
Lynch, S. F., and Ludlam, C. A. (2007). Plasma microparticles and vascular disorders. Br. J. Haematol. 137, 36–48. doi:10.1111/J.1365-2141.2007.06514.X
Maemura, T., Fukuyama, S., Sugita, Y., Lopes, T. J. S., Nakao, T., Noda, T., et al. (2018). Lung-derived exosomal miR-483-3p regulates the innate immune response to influenza virus infection. J. Infect. Dis. 217, 1372–1382. doi:10.1093/INFDIS/JIY035
Mantel, P. Y., Hjelmqvist, D., Walch, M., Kharoubi-Hess, S., Nilsson, S., Ravel, D., et al. (2016). Infected erythrocyte-derived extracellular vesicles alter vascular function via regulatory Ago2-miRNA complexes in malaria. Nat. Commun. 7, 12727–12815. doi:10.1038/ncomms12727
Mayers, J. R., Fyfe, I., Schuh, A. L., Chapman, E. R., Edwardson, J. M., and Audhya, A. (2011). ESCRT-0 assembles as a heterotetrameric complex on membranes and binds multiple ubiquitinylated cargoes simultaneously. J. Biol. Chem. 286, 9636–9645. doi:10.1074/JBC.M110.185363
Mendonça, L., Howe, A., Gilchrist, J. B., Sheng, Y., Sun, D., Knight, M. L., et al. (2021). Correlative multi-scale cryo-imaging unveils SARS-CoV-2 assembly and egress. Nat. Commun. 12, 4629. doi:10.1038/s41467-021-24887-y
Monsel, A., Zhu, Y. G., Gennai, S., Hao, Q., Hu, S., Rouby, J. J., et al. (2015). Therapeutic effects of human mesenchymal stem cell-derived microvesicles in severe pneumonia in mice. Am. J. Respir. Crit. Care Med. 192, 324–336. doi:10.1164/RCCM.201410-1765OC
Morel, P. A., Falkner, D., Plowey, J., Larregina, A. T., and Falo, L. D. (2004). DNA immunisation: Altering the cellular localisation of expressed protein and the immunisation route allows manipulation of the immune response. Vaccine 22, 447–456. doi:10.1016/J.VACCINE.2003.07.012
Morrison, T. J., Jackson, M. V., Cunningham, E. K., Kissenpfennig, A., McAuley, D. F., O’Kane, C. M., et al. (2017). Mesenchymal stromal cells modulate macrophages in clinically relevant lung injury models by extracellular vesicle mitochondrial transfer. Am. J. Respir. Crit. Care Med. 196, 1275–1286. doi:10.1164/RCCM.201701-0170OC
Müller, C., Hardt, M., Schwudke, D., Neuman, B. W., Pleschka, S., and Ziebuhr, J. (2018). Inhibition of cytosolic phospholipase A 2 α impairs an early step of coronavirus replication in cell culture. J. Virol. 92, e01463. doi:10.1128/JVI.01463-17
Nagashima, S., Jirintai, S., Takahashi, M., Kobayashi, T., Tanggis, , , Nishizawa, T., et al. (2014). Hepatitis E virus egress depends on the exosomal pathway, with secretory exosomes derived from multivesicular bodies. J. Gen. Virol. 95 (Pt 10), 2166–2175. doi:10.1099/VIR.0.066910-0
Nguyen, V. V. T., Witwer, K. W., Verhaar, M. C., Strunk, D., and van Balkom, B. W. M. (2020). Functional assays to assess the therapeutic potential of extracellular vesicles. J. Extracell. Vesicles 10, e12033. doi:10.1002/JEV2.12033
Nikfarjam, S., Rezaie, J., Zolbanin, N. M., and Jafari, R. (2020). Mesenchymal stem cell derived-exosomes: A modern approach in translational medicine. J. Transl. Med. 18, 449–521. doi:10.1186/S12967-020-02622-3
Noronha Nc, N. D. C., Mizukami, A., Caliári-Oliveira, C., Cominal, J. G., Rocha, J. L. M., Covas, D. T., et al. (2019). Priming approaches to improve the efficacy of mesenchymal stromal cell-based therapies. Stem Cell Res. Ther. 10, 131–221. doi:10.1186/S13287-019-1224-Y
Obita, T., Saksena, S., Ghazi-Tabatabai, S., Gill, D. J., Perisic, O., Emr, S. D., et al. (2007). Structural basis for selective recognition of ESCRT-III by the AAA ATPase Vps4. Nature 449, 735–739. doi:10.1038/nature06171
Pérez, P. S., Romaniuk, M. A., Duette, G. A., Zhao, Z., Huang, Y., Martin-Jaular, L., et al. (2019). Extracellular vesicles and chronic inflammation during HIV infection J. Extracell. Vesicles 8. 1687275. doi:10.1080/20013078.2019.1687275
Pleet, M. L., Branscome, H., DeMarino, C., Pinto, D. O., Zadeh, M. A., Rodriguez, M., et al. (2018). Autophagy, EVs, and infections: A perfect question for a perfect time. Front. Cell. Infect. Microbiol. 8, 362. doi:10.3389/FCIMB.2018.00362
Popowski, K. D., Dinh, P.-U. C., George, A., Lutz, H., and Cheng, K. (2021). Exosome therapeutics for COVID-19 and respiratory viruses. View 2, 20200186. doi:10.1002/VIW.20200186
Rai, A., Fang, H., Claridge, B., Simpson, R. J., and Greening, D. W. (2021). Proteomic dissection of large extracellular vesicle surfaceome unravels interactive surface platform. J. Extracell. Vesicles 10, e12164. doi:10.1002/JEV2.12164
Raiborg, C., Grønvold Bache, K., Mehlum, A., Stang, E., and Stenmark, H. (2001). Hrs recruits clathrin to early endosomes. EMBO J. 20, 5008–5021. doi:10.1093/EMBOJ/20.17.5008
Robbins, P. D., and Morelli, A. E. (2014). Regulation of immune responses by extracellular vesicles. Nat. Rev. Immunol. 14, 195–208. doi:10.1038/nri3622
Rocha, N., Kuijl, C., van der Kant, R., Janssen, L., Houben, D., Janssen, H., et al. (2009). Cholesterol sensor ORP1L contacts the ER protein VAP to control Rab7-RILP-p150 Glued and late endosome positioning. J. Cell Biol. 185 (7), 1209–1225. doi:10.1083/jcb.200811005
Romagnoli, G. G., Zelante, B. B., Toniolo, P. A., Migliori, I. K., and Barbuto, J. A. M. (2015). Dendritic cell-derived exosomes may be a tool for cancer immunotherapy by converting tumor cells into immunogenic targets. Front. Immunol. 6, 692. doi:10.3389/FIMMU.2014.00692
Sadri Nahand, J., Moghoofei, M., Salmaninejad, A., Bahmanpour, Z., Karimzadeh, M., Nasiri, M., et al. (2020). Pathogenic role of exosomes and microRNAs in HPV-mediated inflammation and cervical cancer: A review. Int. J. Cancer 146, 305–320. doi:10.1002/IJC.32688
Saraste, J., and Prydz, K. (2021). Assembly and cellular exit of coronaviruses: Hijacking an unconventional secretory pathway from the pre-golgi intermediate compartment via the Golgi ribbon to the extracellular space. Cells 10, 503. doi:10.3390/CELLS10030503
Satija, N. K., Singh, V. K., Verma, Y. K., Gupta, P., Sharma, S., Afrin, F., et al. (2009). Mesenchymal stem cell-based therapy: A new paradigm in regenerative medicine. J. Cell. Mol. Med. 13, 4385–4402. doi:10.1111/J.1582-4934.2009.00857.X
Schorey, J. S., Cheng, Y., Singh, P. P., and Smith, V. L. (2015). Exosomes and other extracellular vesicles in host–pathogen interactions. EMBO Rep. 16, 24–43. doi:10.15252/EMBR.201439363
Sengupta, V., Sengupta, S., Lazo, A., Woods, P., Nolan, A., and Bremer, N. (2020). Exosomes derived from bone marrow mesenchymal stem cells as treatment for severe COVID-19. Stem cells Dev. 29 (12), 747–754. doi:10.1089/scd.2020.0080
Sicco, C. L., Reverberi, D., Balbi, C., Ulivi, V., Principi, E., Pascucci, L., et al. (2017). Mesenchymal stem cell-derived extracellular vesicles as mediators of anti-inflammatory effects: Endorsement of macrophage polarization. Stem Cells Transl. Med. 6, 1018–1028. doi:10.1002/SCTM.16-0363
Sin, J., McIntyre, L., Stotland, A., Feuer, R., and Gottlieb, R. A. (2017). Coxsackievirus B escapes the infected cell in ejected mitophagosomes. J. Virol. 91, e01347-17. doi:10.1128/jvi.01347-17
Snijder, E. J., Decroly, E., and Ziebuhr, J. (2016). The nonstructural proteins directing coronavirus RNA synthesis and processing. Adv. Virus Res. 96, 59–126. doi:10.1016/BS.AIVIR.2016.08.008
Snijder, E. J., Limpens, R. W. A. L., de Wilde, A. H., de Jong, A. W. M., Zevenhoven-Dobbe, J. C., Maier, H. J., et al. (2020). A unifying structural and functional model of the coronavirus replication organelle: Tracking down RNA synthesis. PLoS Biol. 18, e3000715. doi:10.1371/JOURNAL.PBIO.3000715
Somiya, M., Yoshioka, Y., Ochiya, T., Somiya, M., Yoshioka, Y., and Ochiya, T. (2017). Drug delivery application of extracellular vesicles; insight into production, drug loading, targeting, and pharmacokinetics. AIMS Bioeng. 173 (4), 73–92. doi:10.3934/BIOENG.2017.1.73
Song, J. W., Lam, S. M., Fan, X., Cao, W. J., Wang, S. Y., Tian, H., et al. (2020). Omics-driven systems interrogation of metabolic dysregulation in COVID-19 pathogenesis. Cell Metab. 32, 188–202.e5. doi:10.1016/J.CMET.2020.06.016
Tai, W., He, L., Zhang, X., Pu, J., Voronin, D., Jiang, S., et al. (2020). Characterization of the receptor-binding domain (RBD) of 2019 novel coronavirus: Implication for development of RBD protein as a viral attachment inhibitor and vaccine. Cell. Mol. Immunol. 17, 613–620. doi:10.1038/s41423-020-0400-4
Tenoever, B. R. (2013). RNA viruses and the host microRNA machinery. Nat. Rev. Microbiol. 11, 169–180. doi:10.1038/nrmicro2971
Théry, C., Witwer, K. W., Aikawa, E., Alcaraz, M. J., Anderson, J. D., Andriantsitohaina, R., et al. (2018). Minimal information for studies of extracellular vesicles 2018 (MISEV2018): A position statement of the international society for extracellular vesicles and update of the MISEV2014 guidelines. J. Extracell. vesicles 7 (1), 1535750. doi:10.1080/20013078.2018.1535750
Thoms, M., Buschauer, R., Ameismeier, M., Koepke, L., Denk, T., Hirschenberger, M., et al. (2020). Structural basis for translational shutdown and immune evasion by the Nsp1 protein of SARS-CoV-2. Science 369, 1249–1255. doi:10.1126/SCIENCE.ABC8665
Tregoning, J. S., Flight, K. E., Higham, S. L., Wang, Z., and Pierce, B. F. (2021). Progress of the COVID-19 vaccine effort: Viruses, vaccines and variants versus efficacy, effectiveness and escape. Nat. Rev. Immunol. 21 (10), 626–636. doi:10.1038/s41577-021-00592-1
Tricarico, C., Clancy, J., and D’Souza-Schorey, C. (2016). Biology and biogenesis of shed microvesicles. Small GTPases 8, 220–232. doi:10.1080/21541248.2016.1215283
Tsai, S. J., Guo, C., Sedgwick, A., Kanagavelu, S., Nice, J., Shetty, S., et al. (2021). Exosome-mediated mRNA delivery for SARS-CoV-2 vaccination. bioRxiv, 2020.371419. doi:10.1101/2020.11.06.371419
Urbanelli, L., Buratta, S., Tancini, B., Sagini, K., Delo, F., Porcellati, S., et al. (2019). The role of extracellular vesicles in viral infection and transmission. Vaccines 7, 102. doi:10.3390/VACCINES7030102
Urciuoli, E., and Peruzzi, B. (2020). Inhibiting extracellular vesicle trafficking as antiviral approach to corona virus disease 2019 infection. Front. Pharmacol. 11, 580505. doi:10.3389/FPHAR.2020.580505
Vader, P., Mol, E. A., Pasterkamp, G., and Schiffelers, R. M. (2016). Extracellular vesicles for drug delivery. Adv. Drug Deliv. Rev. 106, 148–156. doi:10.1016/J.ADDR.2016.02.006
Valadi, H., Ekström, K., Bossios, A., Sjöstrand, M., Lee, J. J., and Lötvall, J. O. (2007). Exosome-mediated transfer of mRNAs and microRNAs is a novel mechanism of genetic exchange between cells. Nat. Cell Biol. 96 (9), 654–659. doi:10.1038/ncb1596
van der Grein, S. G., Defourny, K. A. Y., Slot, E. F. J., and Nolte-‘t Hoen, E. N. M. (2018). Intricate relationships between naked viruses and extracellular vesicles in the crosstalk between pathogen and host. Semin. Immunopathol. 40, 491–504. doi:10.1007/S00281-018-0678-9
van Dongen, H. M., Masoumi, N., Witwer, K. W., and Pegtel, D. M. (2016). Extracellular vesicles exploit viral entry routes for cargo delivery. Microbiol. Mol. Biol. Rev. 80, 369–386. doi:10.1128/MMBR.00063-15
van Doremalen, N., Lambe, T., Spencer, A., Belij-Rammerstorfer, S., Purushotham, J. N., Port, J. R., et al. (2020). ChAdOx1 nCoV-19 vaccine prevents SARS-CoV-2 pneumonia in rhesus macaques. Nature 586, 578–582. doi:10.1038/s41586-020-2608-y
Van Niel, G., D’Angelo, G., and Raposo, G. (2018). Shedding light on the cell biology of extracellular vesicles. Nat. Rev. Mol. Cell Biol. 19, 213–228. doi:10.1038/nrm.2017.125
Velandia-Romero, M. L., Caldern-Pelaez, M. A., Balbas-Tepedino, A., Alejandro Marquez-Ortiz, R., Madroñero, L. J., Prieto, A. B., et al. (2020). Extracellular vesicles of U937 macrophage cell line infected with DENV-2 induce activation in endothelial cells EA.hy926. PLoS One 15, e0227030. doi:10.1371/JOURNAL.PONE.0227030
Vella, L. J., Sharples, R. A., Nisbet, R. M., Cappai, R., and Hill, A. F. (2008). The role of exosomes in the processing of proteins associated with neurodegenerative diseases. Eur. Biophys. J. 37, 323–332. doi:10.1007/S00249-007-0246-Z
V’kovski, P., Gerber, M., Kelly, J., Pfaender, S., Ebert, N., Lagache, S. B., et al. (2019). Determination of host proteins composing the microenvironment of coronavirus replicase complexes by proximity-labeling. Elife 8, e42037. doi:10.7554/ELIFE.42037
V’kovski, P., Kratzel, A., Steiner, S., Stalder, H., and Thiel, V. (2021). Coronavirus biology and replication: Implications for SARS-CoV-2. Nat. Rev. Microbiol. 19, 155–170. doi:10.1038/s41579-020-00468-6
Vogel, A. B., Kanevsky, I., Che, Y., Swanson, K. A., Muik, A., Vormehr, M., et al. (2020). A prefusion SARS-CoV-2 spike RNA vaccine is highly immunogenic and prevents lung infection in non-human primates. bioRxiv. 2020 doi:10.1101/2020.09.08.280818
Wang, J., Chen, S., and Bihl, J. (2020). Exosome-mediated transfer of ACE2 (Angiotensin-Converting enzyme 2) from endothelial progenitor cells promotes survival and function of endothelial cell. Oxid. Med. Cell. Longev., 2020 1–11. doi:10.1155/2020/4213541
Wang, M., Yuan, Q., and Xie, L. (2018). Mesenchymal stem cell-based immunomodulation: Properties and clinical application. Stem Cells Int., 2018 1–12. doi:10.1155/2018/3057624
Wang, T., Gilkes, D. M., Takano, N., Xiang, L., Luo, W., Bishop, C. J., et al. (2014). Hypoxia-inducible factors and RAB22A mediate formation of microvesicles that stimulate breast cancer invasion and metastasis. Proc. Natl. Acad. Sci. U. S. A. 111 (31), E3234–E3242. doi:10.1073/pnas.1410041111
Witwer, K. W., Goberdhan, D. C., O'Driscoll, L., Théry, C., Welsh, J. A., Blenkiron, C., et al. (2021). Updating MISEV: Evolving the minimal requirements for studies of extracellular vesicles. J. Extracell. Vesicles 10 (14), e12182. doi:10.1002/jev2.12182
Wolff, G., Melia, C. E., Snijder, E. J., and Bárcena, M. (2020). Double-membrane vesicles as platforms for viral replication. Trends Microbiol. 28, 1022–1033. doi:10.1016/J.TIM.2020.05.009
Xia, X., Wang, Y., Huang, Y., Zhang, H., Lu, H., and Zheng, J. C. (2019). Exosomal miRNAs in central nervous system diseases: Biomarkers, pathological mediators, protective factors and therapeutic agents. Prog. Neurobiol. 183, 101694. doi:10.1016/J.PNEUROBIO.2019.101694
Xiao, Y., Wang, S. K., Zhang, Y., Rostami, A., Kenkare, A., Casella, G., et al. (2021). Role of extracellular vesicles in neurodegenerative diseases. Prog. Neurobiol. 201, 102022. doi:10.1016/J.PNEUROBIO.2021.102022
Xu, A. L., Rodriguez, L. A., Walker, K. P., Mohammadipoor, A., Kamucheka, R. M., Cancio, L. C., et al. (2019). Mesenchymal stem cells reconditioned in their own serum exhibit augmented therapeutic properties in the setting of acute respiratory distress syndrome. Stem Cells Transl. Med. 8, 1092–1106. doi:10.1002/SCTM.18-0236
Yao, Z., Qiao, Y., Li, X., Chen, J., Ding, J., Bai, L., et al. (2018). Exosomes exploit the virus entry machinery and pathway to transmit alpha interferon-induced antiviral activity. J. Virol. 92, e01578. doi:10.1128/JVI.01578-18
Yue, B., Yang, H., Wang, J., Ru, W., Wu, J., Huang, Y., et al. (2020). Exosome biogenesis, secretion and function of exosomal miRNAs in skeletal muscle myogenesis. Cell Prolif. 53, e12857. doi:10.1111/CPR.12857
Zhang, S., Liu, Y., Wang, X., Yang, L., Li, H., Wang, Y., et al. (2020). SARS-CoV-2 binds platelet ACE2 to enhance thrombosis in COVID-19. J. Hematol. Oncol. 13 (1), 120. doi:10.1186/s13045-020-00954-7
Zhao, T., Sun, F., Liu, J., Ding, T., She, J., Mao, F., et al. (2019). Emerging role of mesenchymal stem cell-derived exosomes in regenerative medicine. Curr. Stem Cell Res. Ther. 14, 482–494. doi:10.2174/1574888X14666190228103230
Zhao, Y. G., Codogno, P., and Zhang, H. (2021). Machinery, regulation and pathophysiological implications of autophagosome maturation. Nat. Rev. Mol. Cell Biol. 22 (11), 733–750. doi:10.1038/s41580-021-00392-4
Keywords: extracellular vesicles, SARS–CoV–2, COVID-19, EVs-based vaccines, COVID-19 vaccination, drug delivery
Citation: Sbarigia C, Vardanyan D, Buccini L, Tacconi S and Dini L (2022) SARS-CoV-2 and extracellular vesicles: An intricate interplay in pathogenesis, diagnosis and treatment. Front. Nanotechnol. 4:987034. doi: 10.3389/fnano.2022.987034
Received: 05 July 2022; Accepted: 11 August 2022;
Published: 31 August 2022.
Edited by:
Sima Umrao, Indian Institute of Science (IISc), IndiaReviewed by:
Shahnaz Majid Qadri, Texas A&M University, United StatesCopyright © 2022 Sbarigia, Vardanyan, Buccini, Tacconi and Dini. This is an open-access article distributed under the terms of the Creative Commons Attribution License (CC BY). The use, distribution or reproduction in other forums is permitted, provided the original author(s) and the copyright owner(s) are credited and that the original publication in this journal is cited, in accordance with accepted academic practice. No use, distribution or reproduction is permitted which does not comply with these terms.
*Correspondence: L. Dini, bHVjaWFuYS5kaW5pQHVuaXJvbWExLml0
†These authors share first authorship
Disclaimer: All claims expressed in this article are solely those of the authors and do not necessarily represent those of their affiliated organizations, or those of the publisher, the editors and the reviewers. Any product that may be evaluated in this article or claim that may be made by its manufacturer is not guaranteed or endorsed by the publisher.
Research integrity at Frontiers
Learn more about the work of our research integrity team to safeguard the quality of each article we publish.