- 1Escola de Química, Universidade Federal do Rio de Janeiro, Rio de Janeiro, Brazil
- 2Instituto de Química, Universidade Federal do Rio de Janeiro, Rio de Janeiro, Brazil
- 3Campus Duque de Caxias, Universidade Federal do Rio de Janeiro, Rio de Janeiro, Brazil
Global warming, fossil fuel depletion, climate change, as well as a sudden increase in fuel price have motivated scientists to search for methods of storage and reduction of greenhouse gases, especially CO2. Therefore, the conversion of CO2 by hydrogenation into higher hydrocarbons through the modified Fischer–Tropsch Synthesis (FTS) has become an important topic of current research and will be discussed in this review. In this process, CO2 is converted into carbon monoxide by the reverse water-gas-shift reaction, which subsequently follows the regular FTS pathway for hydrocarbon formation. Generally, the nature of the catalyst is the main factor significantly influencing product selectivity and activity. Thus, a detailed discussion will focus on recent developments in Fe-based, Co-based, and bimetallic catalysts in this review. Moreover, the effects of adding promoters such as K, Na, or Mn on the performance of catalysts concerning the selectivity of olefins and higher hydrocarbons are assessed.
Introduction
Due to the growing world demand for fuels and, at the same time, the need to reduce the greenhouse gas concentration in the atmosphere, the conversion of CO2 into hydrocarbons via hydrogenation have gained considerable importance among scientists.
The hydrogenation of CO2 into hydrocarbons in the liquid fuel range can be obtained via a methanol-mediated process or a modified Fischer-Tropsch Synthesis (FTS). In the first route, CO2 is converted to methanol, then transformed into hydrocarbons through the commercially available methanol-to-gasoline (MTG) process based on zeolite catalysts (Wang et al., 2016; Gao et al., 2017; Sedighi and Mohammadi, 2020). The modified FTS also proceeds by a two-step process with the initial conversion of CO2 into CO by the reverse water gas shift (RWGS), followed by hydrogenation of carbon monoxide into hydrocarbons by regular FTS (Geng et al., 2016), as follows.
In the RWGS reaction, CO is obtained through CO2 conversion to form *CO precursor molecules adsorbed on the catalyst surface. The product distribution can be broad, and changes depending on the catalysts’ structure and composition. Thus, it is necessary to prevent high selectivity to methane and light-saturated hydrocarbons (Martinelli et al., 2014; Visconti et al., 2016).
Like the regular FTS, Fe-based, Co-based, and bimetallic catalysts have been extensively used to catalyze the CO2 hydrogenation to C2+ hydrocarbons (Khodakov et al., 2007; Díaz et al., 2014). The iron catalysts have activity for both the RWGS and FTS reactions. Cobalt is already widely used in the industry for the regular FTS, and bimetallic catalysts like Fe-Co combine the properties of each metal with improving the catalytic performance. To improve the catalysts’ hydrocarbon selectivity and reaction activity, the use of specific supports and the addition of promoters are common approaches. Therefore, a detailed discussion will focus on recent developments in catalyst design and catalytic performance, such as the effects of promoters and supports on yields and selectivity of hydrocarbons.
Catalysts
Currently, several metals have activity for the production of hydrocarbons, as shown in Table 1, among which we can mention: cobalt, iron, and bimetallic catalysts. Despite several studies employing iron-based catalysts, cobalt-based catalysts are more resistant to deactivation by water. There is a less significant effect of water on the carbon monoxide conversion rate (Khodakov et al., 2007; Díaz et al., 2014). In contrast, the presence of iron in copper catalysts can favor the catalytic activity of the hydrogenation reaction and the thermal stability of copper particles in high-temperature reactions. Furthermore, iron tends to promote the dispersion of copper, thus resulting in high surface areas.
Regarding the catalyst parameters that can influence the catalytic behavior, we can mention the particle size (Bezemer et al., 2006; Rane et al., 2012), the phase composition, the type of support and its texture (Borg et al., 2007; Cheng et al., 2015). Thus, all must be carefully controlled to obtain efficient catalysts. The main objective of any catalyst preparation for the synthesis of modified FTS is to produce a significant concentration of stable metal sites (Khodakov, 2009). For possible industrial applications, in addition to high conversions and desired product selectivity, the time for which the catalyst can keep these parameters stable is a critical factor. It is essential to note that the stability of the catalysts must also be evaluated. Some studies, such as Liu et al., Zhang et al., and Iglesias et al. (Iglesias et al., 2015; Zhang et al., 2015; Liu et al., 2018a) obtained stabilities above 100 h for iron-based catalysts with the aid of promoters.
Iron catalysts are used to synthesize hydrocarbons due to their high selectivity for the formation of primary olefins (Zhang et al., 2015). Iron-based catalysts stand out in CO2 hydrogenation due to their activity for the RWGS and FTS reaction (Visconti et al., 2017). In CO2 hydrogenation over Fe-based catalysts, the Fe3O4 phase catalyzes the RWGS reaction, and the Hägg iron carbide (χ-Fe5C2) phase (reduced form) provides active sites for CO hydrogenation and chain-growth (Kim et al., 2020b). Therefore, preparation methods and promoter addition have been extensively studied and impact the catalytic activity.
Cobalt catalysts have been used in industry to convert syngas into hydrocarbons and alcohols via FTS. However, due to the strong hydrogenation ability and lack of RWGS activity, the product selectivity is dominated by methane. Chakrabarti et al. (2015) explored the behavior of CO2 on the CoPt/Al2O3 catalyst system under FTS conditions. The only products formed from CO2 over the CoPt/Al2O3 catalyst were C1−C3 hydrocarbons, with methane being the dominant product. Visconti et al. (2016) also observed that, over an un-promoted Co/γ-Al2O3 catalyst, CO2 is effectively hydrogenated, forming mainly methane among the products.
However, using different catalyst preparation techniques and promoters and supports, one can manage to bypass the methanation process and form other products. Selective hydrogenation of CO2 to ethanol was reported by Wang et al. (Wang L. et al., 2018) with cobalt catalysts derived from a Co-Al layered double hydroxide (LDH), which were subjected to calcination and reduction to form alumina-supported cobalt particles (CoAlOx). The catalyst reduced at 600°C showed the best catalytic performance with an ethanol selectivity of 92.1% at 140°C. The authors indicated that this improved performance is likely due to the optimized presence of surface oxide species with coexisting Co–CoO phases, which enhance the production of *CHx for converting formate into acetate by insertion, an essential intermediate for ethanol production.
Furthermore, Wang et al. (2019) indicate that methanation can be avoided by incorporating nickel into the cobalt catalyst, which would favor the formation of the stable intermediate *CHx. The *CHx intermediary is inserted into the *HCOO, which is easily formed to favor the formation of oxygenated C2, according to Figure 1. According to the authors, the Co0,52Ni0,48AlOx catalyst boosts ethanol production to outperform the noble-metal-containing catalysts. The two works developed by Wang (Wang L. et al., 2018; Wang et al., 2019) expand the application of cobalt as a catalyst by shifting methane production as the primary product and increasing the selectivity of ethanol. The works developed present different modifications of the catalysts. However, they favor the production of ethanol with the formation of *CHx species. Therefore, this is an alternative that draws attention to exploitation.
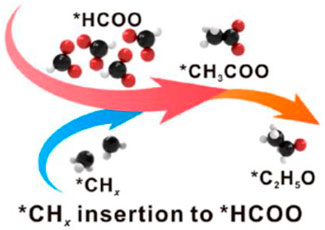
FIGURE 1. *CHx insertion to *HCOO in C2-oxygenates formation on the CoAlOx catalyst (Wang et al., 2019).
Additionally, ethanol hydrocarbons C5+ production can also be achieved from cobalt catalysts. There is an increase in the chemisorption of CO2 and reduction in the adsorption of H2, with the introduction of alkali metals. Then it suppresses the formation of CH4 and increases the selectivity of C5+ at the expense of CO2 conversion levels. Shi et al. (2018a) investigated the impact of promoting alkali metals on catalytic activity and physicochemical properties of CoCu/TiO2 catalysts. Due to the presentation of stronger basicity and lower desorption of H2 among the promoters used in the study, the Na‐modified CoCu/TiO2 catalyst showed the highest yield of C5+ (5.4% and selectivity of 42.1%) with a CO2 conversion of 18.4%.
Tarasov et al. (2018b) evaluated the effect of the nature of the metal (Au, Pt, Fe, and Co) on the activity and selectivity of catalysts in the hydrogenation of CO2. The authors indicate that the metals Fe and Co present activity and selectivity to C2+ hydrocarbons. The highest selectivity and yield of С5+ hydrocarbons are thus observed for 10% Co/MIL-53(Al) catalyst at 300°С and iron-containing catalyst at 400°С (= 30.8 and 4.1%, respectively). In another study, carbon dioxide conversion into liquid hydrocarbons was carried out over Co nanoparticles also embedded in the MIL-53(Al) matrix. Tarasov et al. (2018a) evaluated the 10% Co/MIL-53(Al) catalyst and observed good activity in CO2 hydrogenation. Associated with high chemical and thermal stability, the microporous structure derived from Al3+ MIL-53(Al) was defined as a matrix for Co nanoparticles. The observed increase in CO2 conversion compared to the thermodynamically possible conversion is assumed to be due to the expected shift from equilibrium to CO formation due to its rapid conversion to hydrocarbons by the Fischer-Tropsch reaction.
The CO2 hydrogenation to liquid hydrocarbons typically occurs through RWGS reaction, followed by the hydrogenation of CO to hydrocarbons via FTS. According to He et al. (2019), Co catalyst promoted with Mn could successfully avoid the CO route. In the course of the reaction, CO2 adsorbed on the catalyst was reduced to CH2 monomer and CH3 species via CO2δ−, HCOO−, −CH2OH, and/or CH3O− intermediates by H atoms. The liquid hydrocarbons were produced through chain growth steps similar to Co0 catalyzed FTS reaction. The liquid product, C5 to C26 hydrocarbons (mostly n-paraffin), could achieve selectivity of 53.2 C-mol %.
Thus, these studies show that, despite their limitations, there is ample Co-based catalysts field research in which parameters such as particle size, pretreatment, promoters, and preparation method, among others, can be explored for modified FTS.
Considering that the RWGS is a reversible reaction, the driving force for the RWGS reaction can be increased by consuming the CO intermediate to form a hydrocarbon or by decreasing the steam content in the reactor. Therefore, bimetallic catalysts are recommended since monometallic iron does not favor the increase of CO2 conversion. Including a second metal such as cobalt has attracted much attention. The cobalt is highly active in converting CO to hydrocarbons and inactive in the WGS reaction (Geng et al., 2016), favoring high reaction rates (Satthawong et al., 2013; Gnanamani et al., 2016).
Generally, the intimacy between active sites plays a vital role in determining the catalytic performance of bifunctional catalysts. One is the Fe3O4 active site for RWGS, and the other is the FT active site, such as Co or iron carbide. The close contact between the Fe and Co sites favors a higher concentration of CO in the cobalt sites, which is attributed to the easy overflow of the intermediate CO from the Fe3O4 sites to Co and, thus, favors the production of C2+ hydrocarbons through the FTS reaction. Nevertheless, the distance between the Fe and Co sites leads to selectivity to methane, associated with a lower concentration of CO on the Co sites (Jiang et al., 2018).
Guo et al. (2018a) also studied the behavior of the Fe-Co bimetallic catalyst performance in the two-stage reactor system. However, considering the detrimental impact of H2O formed by the RWGS reaction, an ex-situ water removal between the stages was applied, aiming at removing this product formed in the first reactor. The authors indicate that ex-situ water removal plays an important role in decreasing selectivity to undesired CO by-products and improving catalytic activity. Moreover, a two-stage reactor system enhanced liquid fuel yield to 300 gfuels/(kgcath) and promoted CO2 conversion to 69,9%.
Kim et al. (2020b) synthesized, for CO2 hydrogenation, monodisperse CoFe2O4 nanoparticles (NPs). The excellent performance of the bimetallic catalyst can be credited to the formation of bimetallic alloy carbide (Fe1−xCox)5C2, which led to better CO2 conversion, selectivity of C2+ hydrocarbons and light olefins. Figure 2 shows the evolution of CoFe2O4 in the Na-CoFe2O4/CNT catalyst. CoFe2O4 was converted into a single Co-Fe alloy phase during the initial reduction of H2 and posteriorly into the bimetallic carbide alloy (Fe1-xCox)5C2 with the Hägg carbide structure.
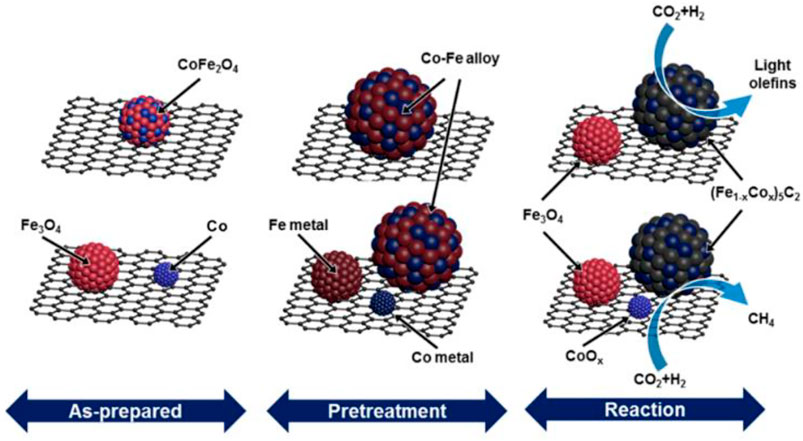
FIGURE 2. Phase transformations of as-prepared spinel CoFe2O4 NPs (top row) or (Fe3O4 + Co) NPs (bottom row) on CNT supports during the pre-reduction and reaction steps. (Kim et al., 2020b).
The hydrogenation capability of cobalt metal is higher than that of the iron catalyst; therefore, there is a benefit in the conversion of CO2 as the cobalt charge increases. However, a higher selectivity to CH4 is observed. This was confirmed by using the 15% Co/SiO2 catalyst, over which CH4 was mainly produced with a selectivity higher than 90% at different temperatures (Geng et al., 2016). Gnanamani et al. (2016) also explored the possibility of keeping iron and cobalt in proximity in a carburized form. The effect of temperature and the fraction of Fe in Co-Fe catalysts were investigated for the activity and selectivity. According to the authors, the increasing Fe fraction and the selectivity to C2−C4 for Co-Fe catalyst increased under all operating conditions. Among the activation conditions, the CO pretreated Co-Fe (50Co50Fe) catalyst presented a much lower methane selectivity. The bimetallic CoFe alloy is the only phase identified with the 50Co50Fe catalyst after reduction in H2 at 350°C. The CO carburized CoFe samples contained smaller particles than those of syngas or H2 pretreated Co−Fe models. The 55Fe Mössbauer study suggests that cobalt was present in proximity to iron which is in both oxide and metallic phases. Despite this, there is the possibility of the presence of cobalt in any of the existing iron carbides. Further ongoing studies aim at establishing the structure and location of cobalt in iron carbides at lower concentrations. The CO2 hydrogenation activity of the CO pretreated Co-Fe catalyst decreased with increasing Fe content from 0 to 50%. The 50Co50Fe catalyst exhibited fairly high selectivity toward C2−C4 hydrocarbons (20–25%) and oxygenates (4.0–5.0%) and a lower selectivity to methane (50–60%) in comparison to the CO-pretreated monometallic catalysts (i.e., Fe and Co). Further increases in Fe content of the catalyst did not significantly alter CO2 hydrogenation activity and selectivity. The CO pretreated 50Co50Fe sample exhibited a low selectivity for methane (47.1%) in comparison to syngas or H2 pretreated 50Co50Fe catalysts (69.2 and 58.5%). An even better selectivity to oxygenates (5.1 or 2.2%) and lower selectivity to methane (30.6 or 14.4%) from CO2 was found for the 1% sodium (Na) or 1.7% potassium (K) promoted 50Co50Fe catalyst. K may be involved in the catalytic cycle for forming acetic acid, acetaldehyde, and ethanol from CO2.
These studies show that the efficiency of Fe-Co bimetallic catalysts can be explained by improving the Fe activity Co. The cobalt has a high power to convert the intermediate CO formed in the RWGS reaction, increasing the driving force of the process and promoting hydrocarbons formation.
In contrast to other metals such as Co, Ni, and Ru, the Cu-based catalyst is well-known for not promoting CO2 methanation (Wang et al., 2011) and for its activity in RWGS (Centi and Perathoner, 2009). In bimetallic catalysts with Cu and Fe, Cu can catalyze RWGS to produce CO, and Fe can enhance chain growth in CO hydrogenation. Thus, the work of Wang et al. (Wang W. et al., 2018) focuses on the effect of combining Fe and Cu on CO2 hydrogenation. A series of Fe-Cu/Al2O3 catalysts with a wide range of Cu/(Fe + Cu) atomic ratios were prepared, and it was observed that the CO2 conversion increases with the increasing Cu content. The maximum conversion was achieved with a ratio of 0.17, however, higher Cu ratios decreased CO2 conversion.
Liu et al. (2018b) also studied the outcome of doping Cu into Fe-based supported catalysts. Catalysts with different Fe and Cu percentages were prepared by various impregnation methods to observe the effect of Cu on the product distribution. This effect over Fe-supported catalyst was different from that obtained using the other usual promoters (e.g., K, Mn, Zn, etc.). The selectivity of light olefins (C2=−C4=) decreased, but a significant improvement was obtained for hydrocarbons C5+. The intense interaction between Cu and Fe facilitated the reduction of Fe, and CO2 adsorption was enhanced on Cu-supported catalysts. Soon, was contributed to the improvement of CO2 conversion, a decrease in the selectivity of CH4, and an increase in selectivity of C5+. Further, the enhanced adsorption of primarily formed olefins on Cu-promoted catalysts favored the secondary conversion of the produced olefins. Hydrogenation of olefins increased the selectivity to paraffin, and the oligomerization of olefins increased the selectivity to C5+.
Supports
In general, studies have focused on using oxides as supports. The application of various supports allows the modification of the metal dispersion, particle size, and metal-support interactions due to the physicochemical properties of the supports. Metal or non-metal oxide supports such as silica (SiO2), alumina (Al2O3), titania (TiO2) as well as carbon materials have been widely used in CO2-FTS catalysts with alkali promoters to produce olefin-rich hydrocarbon mixtures (Hwang et al., 2020).
Owen et al. (2016) studied the effect of inorganic oxide supports (SiO2, CeO2, TiO2 Al2O3, MgO and ZrO2, and ZSM-5) Co-Na-Mo-based catalysts on the direct conversion of CO2 into hydrocarbons. Catalysts supported on SiO2 and ZSM-5 present the highest CO2 conversion values and similar CO and hydrocarbon selectivity. The Co-Na-Mo catalysts supported on CeO2, TiO2, Al2O3, and ZrO2 displayed a similar CO2 conversion under the studied conditions, approximately 15%. However, the hydrocarbon selectivity/CO ratio decreases in the order of ZrO2 < Al2O3 < TiO2 < CeO2. Liu et al. (2018c) also tested a series of supports, in this case, K-modified in a physical mixture with pure Fe5C2 catalyst. The authors observed that the alkaline Al2O3 favored light olefins and C5+ hydrocarbons production since, during the reaction, the potassium migrated into Fe5C2, improving the C2-4= olefins and C5+ value-added hydrocarbons formation.
As seen in these two studies and among others, alumina is widely used as a support for CO2 conversion. In this line, investigating how the surface Al–OH groups in Al2O3 supported catalysts can affect the FeK catalyst performance, Ding et al. (2014) conducted a study. The authors found that, in addition to favoring the CO adsorption, basic hydroxyls improve the iron particles’ dispersion with a smaller particle size of iron and higher catalyst activity. Also, Piriyasurawong et al. (2021) synthesized a Fe/Ce-Al2O3 catalysts by one-step flame spray pyrolysis (FSP). This study observed that Ce increased the carburization process and the formation of iron carbide, improving the catalytic activity and hydrocarbon selectivity.
Kattel et al. (2016) observed that CeO2 and ZrO2 supported PtCo catalysts showed high CH4 selectivity, while CO is preferentially formed with TiO2 support. An AP-XPS analysis of model surfaces and the FTIR spectra of the powder catalysts revealed that the difference in selectivity is probably associated with different dominant reaction pathways. HCOO/*HOCO and *CH3O were observed on PtCo/CeO2 and PtCo/ZrO2 as reaction intermediates, while *HCOO/*HOCO was observed on PtCo/TiO2 catalyst.
In addition to these parameters, the pore size distribution of supports is also significant in determining the catalytic performance and product distribution. It can profoundly impact the dispersion of metals, reducibility, and mass transport performance. In this line, the increasing catalyst pore sizes lead to the higher olefins/paraffins ratios due to the enhancement of product diffusion, alleviating the hydrogenation of re-adsorbed olefins to paraffins. A too-small pore size distribution is unfavorable for the reaction as it leads to a minimum particle size that is not adequate for C–C bond growth (Xie C. et al., 2017; Numpilai et al., 2019).
To provide insights into the role of the metal-support interaction on the CO2 hydrogenation system, Torrente-Murciano et al. (2016), synthesized a series of nanostructured ceria materials. The study demonstrates that the morphology of the ceria support on Fe/CeO2 catalyst plays a vital role in CO2 conversion, methane to hydrocarbon selectivity, and olefin to paraffin ratio. The modification of the iron reactivity is related to the high metal-support interaction shown by the shift of the reduction temperatures of the Fe/CeO2 catalysts concerning their corresponding supports, associated with the selective exposure of different crystal planes in the different ceria morphologies.
Carbon-based supports are an excellent alternative to oxides. Carbon nanotubes, carbon spheres, vitreous carbons, and activated carbon have a good surface area; their pore structures can be adjusted, thus favoring a lower interaction with the active metallic catalyst due to its inert characteristic. Hwang et al. (2020) fabricated well-defined mesoporous carbon (MPC) and used it as a support material for Fe catalysts to produce liquid fuels. The MPC support promoted the formation of Fe7C3, which is an active phase for FTS, due to weak metal-support interaction. The mesoporous structure provided the benefits of fast mass transfer of hydrocarbon molecules, which enhanced CO2 conversion and C5+ hydrocarbon selectivity.
Regarding iron nanoparticles supported on oxygen- and nitrogen-functionalized multi-walled carbon nanotubes (OCNTs and NCNTs) and SiO2, Chew et al. (2014b) observed that the iron oxide nanoparticles have a lower reduction rate on SiO2 than on CNTs support. This behavior can be explained by the strong metal–support (Fe/SiO2) interactions that make it challenging to reduce Fe and consequently interfere with the catalytic activity. Between OCNTs and NCNTs, the NCNTs promotes the iron oxide nanoparticles reduction at lower temperatures and produce the highest olefin selectivity (C2-5=).
Wang et al. (Wang S. et al., 2020) also evaluated carbon materials. The authors fabricated iron−potassium catalysts supported by single- and multi-walled carbon nanotubes, FeK/SWNTs, and FeK/MWNTs. The study finds that the FeK/MWNTs catalyst favors the selectivity of C2-4 olefins (30.7%), whereas the FeK/SWNTs catalyst favors the selectivity of C5+ olefins (39.8%). Besides high productivity of heavy olefins, the FeK/SWNTs catalyst also provides much lower selectivities to unwanted CO and CH4. Its excellent catalytic performance is tentatively explained by the high inclination of SWNTs with large curvature to donate electrons that facilitate the dissociation of the C–O bond. It incites the formation of carbon monomers and ameliorates the carbon/hydrogen surface ratio, helpful to the formation of olefins.
Dong et al. (2020) explored the phase change, particle size, and CO2 hydrogenation performance of a Na-promoted Fe and Co catalyst derived from ZIF-67. According to the authors, there was an excellent selectivity to olefins associated with the formation of the NC layer, which anchored and dispersed the nanoparticles, promoting the reduction and carburization of the metal. However, the pyrolysis temperature influences the degree of carbonization of the support, metallic charge, and particle size. For temperatures above 500°C, ZIF-67 is fully pyrolyzed, and the nanoparticles are well incorporated into the generated carbon support.
Promoters
The alkali metals, principally Na and K, are the most efficacious promoters (Figure 3), limiting methane formation and improving selectivity to C2+ products (Figure 4). According to Table 2, there has been an increase in studies using bimetallic catalysts in recent years, mainly using promoters such as K and Na. When evaluating the selectivity to CH4, there is an average reduction for Co and Fe catalysts of 23 and 42%, respectively. For bimetallic catalysts, such as CoFe (without the addition of promoters), selectivity ranges for CH4 and C2-C4 are at 45 and 20%, respectively. On the other hand, with the addition of K and Na, there is a reduction in CH4 and C2-C4 paraffinic selectivity. However, the formation of C2-C4 olefins is favored. Regarding C5+ selectivity, the addition of promoters is more pronounced in Fe catalysts when compared to Co and bimetallic catalysts, with an average increase above 100%.
K favors CO and CO2 adsorption, increases the chain growth probability, inhibits secondary reactions, and enhances olefins production. Also, it can stabilize the texture of Fe-Al-O spinel, increase the surface Fe5C2 content and strengthen CO2 adsorption (Iglesias et al., 2015). Ramirez et al. (2018) studied the promotion of the resulting Fe (41 wt%)-carbon composites with Cu, Mo, Li, Na, K, Mg, Ca, Zn, Ni, Co, Mn, Fe, Pt, and Rh), and observed that only K can increase the olefins selectivity. Thus, iron oxide and iron carbides favor the absorption of CO2 and CO while reducing the affinity of H2, increasing the selectivity to olefins. However, its effects on the product selectivity and the catalyst activity are strongly dependent on its concentration and the reaction conditions, including the nature of the reacting mixture containing CO or CO2 (Visconti et al., 2017).
Given this, Satthawong et al. (2015) explored the effect of K on FeCo-catalyzed CO2 hydrogenation. The authors found that the addition of potassium enhanced the activity and stability of iron carbide for the hydrogenation of carbon dioxide. Still, the hydrogenation function of the catalyst is suppressed with increasing K levels in the catalyst, resulting in higher selectivity for CO and oxygenates. The authors proposed a model for the hydrogen absorption states on catalysts with and without K. The selectivity to oxygenates increases (to a maximum of 18 C %, CO free) with the increasing K content. Besides, the potassium shifts the product distribution of C2 oxygenates from CO2 towards acetic acid. So, with the addition of potassium, the weakly adsorbed hydrogen on the catalyst surface suppresses the hydrogenation of the produced olefins, resulting in the high olefin content in the hydrocarbon product. Moreover, at the same reaction condition, Fe–Co(0.17)/K (1.0)/Al2O3 catalyst also showed higher CO2 conversion and higher yield of light olefins than the K-promoted Fe–Ce and Fe-Mn/Al2O3 which are known as promising catalysts for hydrocarbon production from CO2 hydrogenation.
The critical parameter to be observed is the different loading levels of K in the iron-based CO2 hydrogenation. Higher K loadings increased the chain growth probability. Further, the K promotion directly enhances the formation of χ-Fe5C2 under reaction conditions but, at higher concentrations, has a detrimental impact on the FT activity without directly influencing the RWGS reaction (Fischer et al., 2016). There is an improvement in CO2 hydrogenation to light olefins over Fe-based catalyst by changing the content of the K promotor and, hence, setting the bonding strengths of adsorbed species. The K/Fe atomic ratio of 2.5 exhibited the maximum C2-4 olefins distribution (46.7%) and O/P ratio of 7.6. This is because the K increases the bonding strength of adsorbed CO2 and H2 species, reducing the hydrogenation of olefins to paraffins. However, the catalyst that obtained the highest yield of light olefins (16.4%) was with 0.5 KFe catalyst. This is justified by the K enriched surface in K/Fe of 2.5, drastically reducing the surface area and creating a hydrogen-lean environment, ultimately diminishing catalytic activity (Numpilai et al., 2020). Visconti et al. (2017) have found that careful control of the calcination process allows achieving high surface area and avoids transforming the spinel structure (Fe3O4/γ-Fe2O3) into the more stable corundum structure of γ-Fe2O3. The authors prepared a high surface area K-promoted iron-based catalyst. The catalyst was synthetized The catalyst was synthetized by thermal decomposition of ammonium glycolate complexes and then by impregnating an aqueous solution of K carbonate, drying, and calcination. The prepared catalyst performs better than two reference model samples (K-α-Fe2O3 and K-Fe3O4) in CO2 hydrogenation. In turn, this results in a better catalytic activity because of the maximization of type II sites (active in CO activation and C–C bond formation) compared to type I sites (involved in the WGS/RWGS process).
Geng et al. (2016) aimed at suppressing the formation of CO and CH4, thus investigated K-promoted supported and unsupported iron catalysts and different reaction conditions, reactor systems, and zeolites for the catalytic conversion of CO2. The iron supported on different supports by K-promotion displayed undesirably high selectivities to CO ranging from 23.2 to 54.5% and selectivities to CH4 ranging from 22.4 to 41.2% at similar conversions of approximately 40%. In contrast, the precipitated iron 92.6Fe7.4K catalyst showed significantly higher activity (41.7%), very low selectivity to CO (6%) and CH4 (10.3%), and high olefins (C2–C4) selectivity (77.7%) under the same reaction conditions. The low selectivity to CO might be ascribed to a larger active iron carbide surface, which increases the chances of CO-FTS. In addition, to produce gasoline-range isoparaffins, the catalyst 92.6Fe7.4K was mixed with 0.5 Pd or HZSM-5 zeolite. The HZSM-5 showed a higher isoparaffin selectivity (70%) due to hydrocracking and hydroisomerization of FT olefin primary products. Figure 5 shows the reaction path of CO2 hydrogenation through iron catalyst and zeolite.
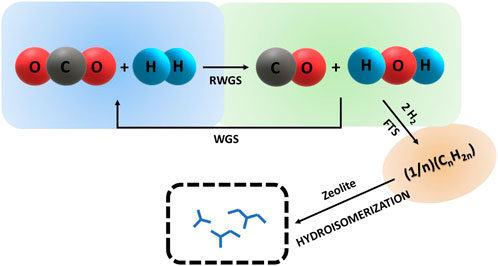
FIGURE 5. Reaction network for isoparaffin synthesis by CO2-based FTS followed by hydroisomerization of the FT products (Geng et al., 2016).
K is the most used promoter, as shown in Table 2. However, studies simultaneously use K and lanthanum (La) promoters. The combination of La and K can adjust the H and C coverage on the catalyst surface, which is essential in changing product distribution in CO2 hydrogenation. Boreriboon et al. (2018) reported the outcomes of K and La promoting in Fe–Cu/TiO2 catalyst on hydrocarbon production from CO2 hydrogenation. The promoted catalyst gave higher C5+ hydrocarbons selectivity (29 C-mol%) than the unpromoted catalyst (21 C-mol%). And the characterization showed that the K addition could significantly reduce the amount of chemisorbed H2 species and increase chemisorbed CO2 species on the catalyst surface. Furthermore, La addition is able to promote the moderately adsorbed CO2 species (principally monodentate carbonate species), which leads to increasing C5–C7 selectivity.
In addition to K and La, Na has also been proposed to enhance both the activity and the selectivity to heavy hydrocarbons and olefins in FT synthesis (da Silva and Mota, 2019). Na was frequently introduced to catalysts employing a precipitating agent on the account that it is difficult to thoroughly remove the residual Na during washing of the precipitates (Huang et al., 2010). Therefore, it is crucial to discuss this effect on the catalyst’s physico-chemical properties and catalytic performance. Based on this, Wei et al. (2016) prepared a series of Fe3O4-based nanocatalysts with different residual Na contents. The FeNa (1.18) catalyst exhibited the maximum olefin/paraffin ratio and the largest total C2–C4 olefins and C5+ yield with a higher CO2 conversion among the FeNa(x) series. The authors observed that increasing the residual Na promotes the surface basicity and dramatically improves the carburization degrees of the iron catalysts. Likewise, Liang et al. (2019a) also indicated that adding the Na promoter favors the increase of CO2 adsorption, the formation and stability of Fe2C2, and inhibits the secondary hydrogenation of alkenes. By increasing the amount of Na, both CO2 conversion and selectivity to alkenes are favored, the highest being 36.8 and 64.3%, respectively, while the corresponding methane production decreases to 7.2%. In situ Raman spectra and temperature-programmed profiles performed by Wei et al. (2020) indicated that the presence of the Na promoter also favors carbon deposits by CO dissociation and inhibits surface carbon reduction by H2. As a result, the intermediate carbon species hydrogenation is limited, favoring enhanced of olefins.
Manganese (Mn) was also the subject of studies for Fe-based catalysts to favor the high selectivity of light olefins. Thus, Liu et al. (Liu B. et al., 2018) investigated the effects of promoting Na and Mn in Fe3O4-based catalysts in CO2 hydrogenation to produce light α-olefins. The authors observed that both Mn and Na promoters can increase the reducibility of Fe3O4. However, the promotional mechanism for Na and Mn in the reduction behavior is different. The authors proposed a mechanism for the promotional reducibility of iron oxides by the presence of oxygen vacancies in MnO and by the donation of electrons from Na. In this case, Na favors the reduction by electron donation to Fe as the oxygen vacancy formation energy is expressively diminished in the presence of Na. In contrast, the oxygen vacancy in MgO promotes reduction as the oxygen in Fe oxide can spontaneously overflow into the vacancy in MnO. In addition, Jiang et al. (2020) synthesized Mn well-dispersed on Fe3O4 microsphere (Mn−Fe3O4) catalyst and investigated the mechanism of the Mn-modulation effect on the C=O band activation and C−C chain growth. The study indicated that adding Mn to Fe-based catalysts could facilitate the C=O bond activation in the RWGS reaction of CO2 hydrogenation and inhibit C−C chain growth and the hydrogenation improving the selectivity and yield of olefins.
In opposition, the results obtained by Zhang et al. (Zhang Z. et al., 2020) show that the selectivity of light olefins decreases from 38.73 to 30.58%. In comparison, the ratio olefin/paraffin decreases from 4.97 to 3.56, with the content of Mn promoters in FeNa catalyst increasing from 0.5 to 10.0%. With the increasing content of the Mn, the strong adsorption of CO2 is confirmed on surface Fe species near the Mn promoters, which possibly decreases the conversion of CO2. Furthermore, the increasing content of Mn increases the adsorption ability of H2 on the surface of iron carbides, which is associated with the secondary hydrogenation of intermediates to paraffins and consequently decreases the ratio of olefins to paraffins. The electronic effect of Mn promoters on the nature of Fe-based active species and the catalytic consequences during CO2 hydrogenation is a very controversial topic. The same debate is open to the electronic effects of zinc during CO and CO2 hydrogenation processes. With the scope of identifying the most influential promoter, Falbo et al. (2017) studied the outcomes of Zn or Mn association in the structure of a Fe-based catalyst. The authors found that the two catalysts have resemblant textural and morphological properties, with elevated surface areas, a predominance of the crystalline Fe2O3 phase, and the presence of ZnFe2O4 and MnFe2O4. However, different from Mn, the stronger CO2 adsorptions keep the H/C ratio on the surface low in the Zn-promoted sample. As a result, the products shift toward long-chain and unsaturated hydrocarbons. Likewise, Zhang et al. (2015) prepared a series of K-promoted Fe–Zn catalysts with varied Fe/Zn molar ratios and applied them to CO2 hydrogenation reaction via FTS for the selective production of C2–C4 olefins. Results showed that the addition of zinc to the iron matrix formed the ZnFe2O4 spinel phase and ZnO phase, caused an increase in the surface areas, enhanced the interaction between iron and zinc, and altered the reduction and CO2 adsorption behaviors. In contrast to the results obtained by Falbo et al. (2017), the appropriate interactions between Fe and Zn demonstrate to be advantageous in repressing the C5+ hydrocarbons production and promoting the production of light olefins. The 1Fe–1Zn–K catalyst with H2/CO reduction exhibited a higher performance with the CO2 conversion of 51%. The selectivity of C2–C4 olefins in overall hydrocarbons and the olefin ratio to paraffin in the C2–C4 fraction reached 53.5 and 6.8%, respectively. Therefore, Zn serves as a structural promoter to suppress the growth of Fe particles. In contrast, Na serves as an electronic promoter to modify the catalytic activity and selectivity of the Fe particles. The combination of Zn and Na with Fe increases the CO2 adsorption properties. It promotes the in situ formation of Hägg iron carbide (χ-Fe5C2), the active phase for forming heavy hydrocarbons in CO2 hydrogenation (Choi et al., 2017b).
Although iron-based catalysts are a feasible route to olefins production from CO2, one challenge is their vulnerability to water produced during the reaction. An additional route to increasing a catalyst’s resistance to water is to add elements that are more resistant to oxidation. to get around this effects of water on iron-based catalysts, Bradley et al. (2017b) added copper (10–30%) to the catalyst blend to improve catalyst activity and durability. The authors showed that adding 10% copper decreases the selectivity of CH4 and CO and considerably increases the CO2 conversion. Moreover, copper does not influence the catalyst’s crystallinity or dispersion but generates some phase separation effects if the quantity of copper is too high. According to the authors, the FeCu/K catalyst creates an inverse spinel crystal phase, independent of the amount of metal. Furthermore, the formation of this phase is favored with the loading of Cu (>10%).
Conclusion
The use of CO2 and H2 as clean hydrocarbons sources remains a challenge, gaining emphasis in the last years due to the potential for reducing CO2 emissions and introducing renewable energy in the chemical industry and automotive market.
Several routes have been evaluated for hydrogenating CO2 into hydrocarbons. This review summarizes the FTS pathway catalytic hydrogenation progress of CO2, which consists of a modified FT reaction, where CO2 reacts instead of CO.
The reaction occurs in two steps. Firstly, CO2 reacts with H2 in the RWGS to form CO, and then CO reacts in the traditional FT reactions to form hydrocarbons. The reaction coupling of RWGS and carbon chain growth should proceed synergistically to achieve high selectivity towards the desired heavy hydrocarbons instead of a more undesirable CO product. Therefore, the reaction requires a catalyst that has to be active in both the RWGS and the FT reactions. Because of their higher activity in RWGS, Fe-based catalysts are preferred (usually doped with alkali metals) over Co-based catalysts.
This review shows that alkali metals, especially K, are essential as promoters for obtaining high selectivity to olefins. K is an excellent promoter increasing the catalyst carburization process and decreasing H2 adsorption. As a result, a higher activity in the chain growth process and an inhibition of the secondary hydrogenation of olefins is obtained. Following potassium, Na has been the most used promoter because it favors the formation and stability of Fe2C2, increases the CO2 conversion by improving surface adsorption, and inhibits the hydrogenation, enhancing olefins selectivity.
The most common support used in modified FTS is γ-Al2O3. Their modification with K and Ce enhanced light olefins and C5+ hydrocarbons formation and increased the carburization process and the formation of iron carbide, respectively. SiO2 supports difficult iron oxide nanoparticle reduction by strongly interacting with the metal and the support. Carbon-based supports have a lower interaction with the active metallic catalyst due to their inert characteristic. Nontraditional supports, such as MOFs and porous carbons, need further research to increase stability and lower olefin yields.
Many technological and economic impediments still have to be overcome. Economically feasible technical and catalytic advances are still required for the large-scale conversion of CO2 to value-added hydrocarbons. However, economic and social incentives can encourage investigations that aim at developing more efficient catalysts.
Author contributions
JA, PR, and ES-A contributed to conception and design of the study. MT and GW organized the database. MT wrote the first draft of the manuscript. GW, JA, PR, and ES-A wrote sections of the manuscript. All authors contributed to manuscript revision, read, and approved the submitted version.
Conflict of interest
The authors declare that the research was conducted in the absence of any commercial or financial relationships that could be construed as a potential conflict of interest.
Publisher’s note
All claims expressed in this article are solely those of the authors and do not necessarily represent those of their affiliated organizations, or those of the publisher, the editors and the reviewers. Any product that may be evaluated in this article, or claim that may be made by its manufacturer, is not guaranteed or endorsed by the publisher.
References
Al-Dossary, M., Ismail, A. A., Fierro, J. L. G., Bouzid, H., and Al-Sayari, S. A. (2015). Effect of Mn loading onto MnFeO nanocomposites for the CO2 hydrogenation reaction. Appl. Catal. B Environ. 165, 651–660. doi:10.1016/j.apcatb.2014.10.064
Albrecht, M., Rodemerck, U., Schneider, M., Bröring, M., Baabe, D., and Kondratenko, E. V. (2017). Unexpectedly efficient CO2 hydrogenation to higher hydrocarbons over non-doped Fe2O3. Appl. Catal. B Environ. 204, 119–126. doi:10.1016/j.apcatb.2016.11.017
Ando, H. (2016). Selective alkene production by the hydrogenation of carbon dioxide over Fe-Cu catalyst. Energy Procedia 89, 421–427. doi:10.1016/j.egypro.2016.05.056
Bashiri, N., Omidkhah, M. R., and Godini, H. R. (2021). Direct conversion of CO2 to light olefins over FeCo/XK-ϒAL2O3 (X = La, Mn, Zn) catalyst via hydrogenation reaction. Res. Chem. Intermed. 47 (12), 5267–5289. doi:10.1007/s11164-021-04562-z
Bashiri, N., Royaee, S. J., and Sohrabi, M. (2018). The catalytic performance of different promoted iron catalysts on combined supports Al2O3 for carbon dioxide hydrogenation. Res. Chem. Intermed. 44 (1), 217–229. doi:10.1007/s11164-017-3099-9
Bezemer, G. L., Bitter, J. H., Kuipers, H. P. C. E., Oosterbeek, H., Holewijn, J. E., Xu, X., et al. (2006). Cobalt particle size effects in the Fischer-Tropsch reaction studied with carbon nanofiber supported catalysts. J. Am. Chem. Soc. 128 (12), 3956–3964. doi:10.1021/ja058282w
Boreriboon, N., Jiang, X., Song, C., and Prasassarakich, P. (2018). Higher hydrocarbons synthesis from CO2 hydrogenation over K- and La-promoted Fe–Cu/TiO2 catalysts. Top. Catal. 61 (15–17), 1551–1562. doi:10.1007/s11244-018-1023-1
Borg, O., Eri, S., Blekkan, E. A., Storsaeter, S., Wigum, H., Rytter, E., et al. (2007). Fischer-Tropsch Synthesis over alpha-alumina-supported cobalt catalysts: Effect of support variables. J. Catal. 248 (1), 89–100. doi:10.1016/j.jcat.2007.03.008
Bradley, M. J., Ananth, R., Willauer, H. D., Baldwin, J. W., Hardy, D. R., DiMascio, F., et al. (2017a). The role of catalyst environment on CO2 hydrogenation in a fixed-bed reactor. J. CO2 Util. 17, 1–9. doi:10.1016/j.jcou.2016.10.014
Bradley, M. J., Ananth, R., Willauer, H. D., Baldwin, J. W., Hardy, D. R., and Williams, F. W. (2017b). The effect of copper addition on the activity and stability of iron-based CO2 hydrogenation catalysts. Molecules 22 (9), 1579. doi:10.3390/molecules22091579
Centi, G., and Perathoner, S. (2009). Opportunities and prospects in the chemical recycling of carbon dioxide to fuels. Catal. Today 148 (3–4), 191–205. doi:10.1016/j.cattod.2009.07.075
Chaipraditgul, N., Numpilai, T., Kui Cheng, C., Siri-Nguan, N., Sornchamni, T., Wattanakit, C., et al. (2021). Tuning interaction of surface-adsorbed species over Fe/K-Al2O3 modified with transition metals (Cu, Mn, V, Zn or Co) on light olefins production from CO2 hydrogenation. Fuel 283, 119248. doi:10.1016/j.fuel.2020.119248
Chakrabarti, D., De Klerk, A., Prasad, V., Gnanamani, M. K., Shafer, W. D., Jacobs, G., et al. (2015). Conversion of CO2 over a co-based Fischer-Tropsch catalyst. Ind. Eng. Chem. Res. 54 (4), 1189–1196. doi:10.1021/ie503496m
Chen, T., Jiang, W., Sun, X., Ning, W., Liu, Y., Xu, G., et al. (2020). Size-controlled synthesis of hematite α-Fe2O3 nanodisks closed with (0001) basal facets and {11-20} side facets and their catalytic performance for CO2 hydrogenation. ChemistrySelect 5 (1), 430–437. doi:10.1002/slct.201904490
Chen, Y., Choi, S., and Thompson, L. T. (2016). Low temperature CO2 hydrogenation to alcohols and hydrocarbons over Mo2C supported metal catalysts. J. Catal. 343, 147–156. doi:10.1016/j.jcat.2016.01.016
Cheng, K., Virginie, M., Ordomsky, V. V., Cordier, C., Chernavskii, P. A., Ivantsov, M. I., et al. (2015). Pore size effects in high-temperature Fischer–Tropsch Synthesis over supported iron catalysts. J. Catal. 328, 139–150. doi:10.1016/j.jcat.2014.12.007
Chernyak, S. A., Ivanov, A. S., Stolbov, D. N., Maksimov, S. V., Maslakov, K. I., Chernavskii, P. A., et al. (2020). Sintered Fe/CNT framework catalysts for CO2 hydrogenation into hydrocarbons. Carbon N. Y. 168, 475–484. doi:10.1016/j.carbon.2020.06.067
Chew, L. M., Kangvansura, P., Ruland, H., Schulte, H. J., Somsen, C., Xia, W., et al. (2014b). Effect of nitrogen doping on the reducibility, activity and selectivity of carbon nanotube-supported iron catalysts applied in CO2 hydrogenation. Appl. Catal. A General 482, 163–170. doi:10.1016/j.apcata.2014.05.037
Chew, L. M., Ruland, H., Schulte, H. J., Xia, W., and Muhler, M. (2014a). CO2 hydrogenation to hydrocarbons over iron nanoparticles supported on oxygen-functionalized carbon nanotubes. J. Chem. Sci. 126 (2), 481–486. doi:10.1007/s12039-014-0591-2
Choi, Y. H., Jang, Y. J., Park, H., Kim, W. Y., Lee, Y. H., Choi, S. H., et al. (2017a). Carbon dioxide fischer-tropsch synthesis: A new path to carbon-neutral fuels. Appl. Catal. B Environ. 202, 605–610. doi:10.1016/j.apcatb.2016.09.072
Choi, Y. H., Ra, E. C., Kim, E. H., Kim, K. Y., Jang, Y. J., Kang, K. N., et al. (2017b). Sodium-containing spinel zinc ferrite as a catalyst precursor for the selective synthesis of liquid hydrocarbon fuels. ChemSusChem 10 (23), 4764–4770. doi:10.1002/cssc.201701437
Cui, X., Gao, P., Li, S., Yang, C., Liu, Z., Wang, H., et al. (2019). Selective production of aromatics directly from carbon dioxide hydrogenation. ACS Catal. 9 (5), 3866–3876. doi:10.1021/acscatal.9b00640
Cui, Y., Guo, L., Gao, W., Wang, K., Zhao, H., He, Y., et al. (2021). From single metal to bimetallic sites: Enhanced higher hydrocarbons yield of CO2 hydrogenation over bimetallic catalysts. ChemistrySelect 6 (21), 5241–5247. doi:10.1002/slct.202101072
da Silva, I. A., and Mota, C. J. A. (2019). Conversion of CO2 to light olefins over iron-based catalysts supported on niobium oxide. Front. Energy Res. 7, 1–8. doi:10.3389/fenrg.2019.00049
Dai, C., Zhao, X., Hu, B., Zhang, X., Luo, Q., Guo, X., et al. (2021). Effect of EDTA-2Na modification on Fe-Co/Al2O3for hydrogenation of carbon dioxide to lower olefins and gasoline. J. CO2 Util. 43, 101369. doi:10.1016/j.jcou.2020.101369
Das, T., and Deo, G. (2012a). Effects of metal loading and support for supported cobalt catalyst. Catal. Today 198 (1), 116–124. doi:10.1016/j.cattod.2012.04.028
Das, T., and Deo, G. (2012b). Promotion of alumina supported cobalt catalysts by iron. J. Phys. Chem. C 116 (39), 20812–20819. doi:10.1021/jp3007206
Díaz, J. A., Calvo-Serrano, M., De La Osa, A. R., García-Minguillán, A. M., Romero, A., Giroir-Fendler, A., et al. (2014). β-Silicon carbide as a catalyst support in the Fischer-Tropsch Synthesis: Influence of the modification of the support by a pore agent and acidic treatment. Appl. Catal. A General 475, 82–89. doi:10.1016/j.apcata.2014.01.021
Ding, F., Zhang, A., Liu, M., Zuo, Y., Li, K., Guo, X., et al. (2014). CO2 hydrogenation to hydrocarbons over iron-based catalyst: Effects of physicochemical properties of Al2O3 supports. Ind. Eng. Chem. Res. 53 (45), 17563–17569. doi:10.1021/ie5031166
Ding, J., Huang, L., Gong, W., Fan, M., Zhong, Q., Russell, A. G., et al. (2019). CO2 hydrogenation to light olefins with high-performance Fe0.30Co0.15Zr0.45K0.10O1.63. J. Catal. 377, 224–232. doi:10.1016/j.jcat.2019.07.036
Ding, J., Liu, Q., Ye, R. P., Gong, W., Zhang, F., He, X., et al. (2021). Metal-support interactions in Fe-Cu-K admixed with SAPO-34 catalysts for highly selective transformation of CO2 and H2 into lower olefins. J. Mat. Chem. A Mat. 9 (38), 21877–21887. doi:10.1039/d1ta03327a
Dong, Z., Zhao, J., Tian, Y., Zhang, B., and Wu, Y. (2020). Preparation and performances of ZIF-67-derived FeCo bimetallic catalysts for CO2 hydrogenation to light olefins. Catalysts 10 (4), 455. doi:10.3390/catal10040455
Drab, D. M., Willauer, H. D., Olsen, M. T., Ananth, R., Mushrush, G. W., Baldwin, J. W., et al. (2013). Hydrocarbon synthesis from carbon dioxide and hydrogen: A two-step process. Energy fuels. 27 (11), 6348–6354. doi:10.1021/ef4011115
Elishav, O., Shener, Y., Beilin, V., Landau, M. V., Herskowitz, M., Shter, G. E., et al. (2020). Electrospun Fe-Al-O nanobelts for selective CO2 hydrogenation to light olefins. ACS Appl. Mat. Interfaces 12 (22), 24855–24867. doi:10.1021/acsami.0c05765
Falbo, L., Martinelli, M., Visconti, C. G., Lietti, L., Forzatti, P., Bassano, C., et al. (2017). Effects of Zn and Mn promotion in Fe-based catalysts used for COx hydrogenation to long-chain hydrocarbons. Ind. Eng. Chem. Res. 56 (45), 13146–13156. doi:10.1021/acs.iecr.7b01494
Fischer, N., Henkel, R., Hettel, B., Iglesias, M., Schaub, G., and Claeys, M. (2016). Hydrocarbons via CO2 hydrogenation over iron catalysts: The effect of potassium on structure and performance. Catal. Lett. 146 (2), 509–517. doi:10.1007/s10562-015-1670-9
Gao, P., Li, S., Bu, X., Dang, S., Liu, Z., Wang, H., et al. (2017). Direct conversion of CO2 into liquid fuels with high selectivity over a bifunctional catalyst. Nat. Chem. 9 (10), 1019–1024. doi:10.1038/nchem.2794
Garbarino, G., Cavattoni, T., Riani, P., and Busca, G. (2020). Support effects in metal catalysis: A study of the behavior of unsupported and silica-supported cobalt catalysts in the hydrogenation of CO2 at atmospheric pressure. Catal. Today 345, 213–219. doi:10.1016/j.cattod.2019.10.016
Geng, S., Jiang, F., Xu, Y., and Liu, X. (2016). Iron-based fischer-tropsch synthesis for the efficient conversion of carbon dioxide into isoparaffins. ChemCatChem 8 (7), 1303–1307. doi:10.1002/cctc.201600058
Gnanamani, M. K., Hamdeh, H. H., Jacobs, G., Shafer, W. D., Hopps, S. D., Thomas, G. A., et al. (2017). Hydrogenation of carbon dioxide over K-promoted FeCo bimetallic catalysts prepared from mixed metal oxalates. ChemCatChem 9 (7), 1303–1312. doi:10.1002/cctc.201601337
Gnanamani, M. K., Hamdeh, H. H., Shafer, W. D., Hopps, S. D., and Davis, B. H. (2018). Hydrogenation of carbon dioxide over iron carbide prepared from alkali metal promoted iron oxalate. Appl. Catal. A General 564, 243–249. doi:10.1016/j.apcata.2018.07.034
Gnanamani, M. K., Jacobs, G., Hamdeh, H. H., Shafer, W. D., and Davis, B. H. (2013). Fischer-Tropsch synthesis: Mössbauer investigation of iron containing catalysts for hydrogenation of carbon dioxide. Catal. Today 207, 50–56. doi:10.1016/j.cattod.2012.02.059
Gnanamani, M. K., Jacobs, G., Hamdeh, H. H., Shafer, W. D., Liu, F., Hopps, S. D., et al. (2016). Hydrogenation of carbon dioxide over Co-Fe bimetallic catalysts. ACS Catal. 6 (2), 913–927. doi:10.1021/acscatal.5b01346
Gnanamani, M. K., Jacobs, G., Keogh, R. A., Shafer, W. D., Sparks, D. E., Hopps, S. D., et al. (2015). Fischer-Tropsch synthesis: Effect of pretreatment conditions of cobalt on activity and selectivity for hydrogenation of carbon dioxide. Appl. Catal. A General 499, 39–46. doi:10.1016/j.apcata.2015.03.046
Guo, L., Cui, Y., Zhang, P., Peng, X., Yoneyama, Y., Yang, G., et al. (2018a). Enhanced liquid fuel production from CO2 hydrogenation: Catalytic performance of bimetallic catalysts over a two-stage reactor system. ChemistrySelect 3 (48), 13705–13711. doi:10.1002/slct.201803335
Guo, L., Li, J., Zeng, Y., Kosol, R., Cui, Y., Kodama, N., et al. (2020). Heteroatom doped iron-based catalysts prepared by urea self-combustion method for efficient CO2 hydrogenation. Fuel 276, 118102. doi:10.1016/j.fuel.2020.118102
Guo, L., Sun, J., Ji, X., Wei, J., Wen, Z., Yao, R., et al. (2018b). Directly converting carbon dioxide to linear α-olefins on bio-promoted catalysts. Commun. Chem. 1 (1), 11–18. doi:10.1038/s42004-018-0012-4
Guo, L., Sun, S., Li, J., Gao, W., Zhao, H., Zhang, B., et al. (2021). Boosting liquid hydrocarbons selectivity from CO2 hydrogenation by facilely tailoring surface acid properties of zeolite via a modified Fischer-Tropsch synthesis. Fuel 306, 121684. doi:10.1016/j.fuel.2021.121684
Guo, L., Zhang, P., Cui, Y., Liu, G., Wu, J., Yang, G., et al. (2019). One-pot hydrothermal synthesis of nitrogen functionalized carbonaceous material catalysts with embedded iron nanoparticles for CO2 hydrogenation. ACS Sustain. Chem. Eng. 7 (9), 8331–8339. doi:10.1021/acssuschemeng.8b06795
Gupta, S., Jain, V. K., and Jagadeesan, D. (2016). Fine tuning the composition and nanostructure of Fe-based core–shell nanocatalyst for efficient CO2 hydrogenation. ChemNanoMat 2 (10), 989–996. doi:10.1002/cnma.201600234
He, Z., Cui, M., Qian, Q., Zhang, J., Liu, H., and Han, B. (2019). Synthesis of liquid fuel via direct hydrogenation of CO2. Proc. Natl. Acad. Sci. U. S. A. 116 (26), 12654–12659. doi:10.1073/pnas.1821231116
Huang, L., Qin, Z., Wang, G., Du, M., Ge, H., Li, X., et al. (2010). A detailed study on the negative effect of residual sodium on the performance of Ni/ZnO adsorbent for diesel fuel desulfurization. Ind. Eng. Chem. Res. 49 (10), 4670–4675. doi:10.1021/ie100293h
Hwang, S. M., Han, S. J., Min, J. E., Park, H. G., Jun, K. W., and Kim, S. K. (2019). Mechanistic insights into Cu and K promoted Fe-catalyzed production of liquid hydrocarbons via CO2 hydrogenation. J. CO2 Util. 34, 522–532. doi:10.1016/j.jcou.2019.08.004
Hwang, S. M., Zhang, C., Han, S. J., Park, H. G., Kim, Y. T., Yang, S., et al. (2020). Mesoporous carbon as an effective support for Fe catalyst for CO2 hydrogenation to liquid hydrocarbons. J. CO2 Util. 37, 65–73. doi:10.1016/j.jcou.2019.11.025
Iglesias, G. M., De Vries, C., Claeys, M., and Schaub, G. (2015). Chemical energy storage in gaseous hydrocarbons via iron Fischer-Tropsch synthesis from H2/CO2 - kinetics, selectivity and process considerations. Catal. Today 242, 184–192. doi:10.1016/j.cattod.2014.05.020
Jiang, F., Liu, B., Geng, S., Xu, Y., and Liu, X. (2018). Hydrogenation of CO2 into hydrocarbons: Enhanced catalytic activity over Fe-based Fischer-Tropsch catalysts. Catal. Sci. Technol. 8 (16), 4097–4107. doi:10.1039/c8cy00850g
Jiang, J., Wen, C., Tian, Z., Wang, Y., Zhai, Y., Chen, L., et al. (2020). Manganese-promoted Fe3O4 microsphere for efficient conversion of CO2 to light olefins. Ind. Eng. Chem. Res. 59 (5), 2155–2162. doi:10.1021/acs.iecr.9b05342
Jimenez, J. D., Wen, C., and Lauterbach, J. (2019). Design of highly active cobalt catalysts for CO2 hydrogenation: Via the tailoring of surface orientation of nanostructures. Catal. Sci. Technol. 9 (8), 1970–1978. doi:10.1039/c9cy00402e
Jimenez, J. D., Wen, C., Royko, M. M., Kropf, A. J., Segre, C., and Lauterbach, J. (2020). Influence of coordination environment of anchored single-site cobalt catalyst on CO2 hydrogenation. ChemCatChem 12 (3), 846–854. doi:10.1002/cctc.201901676
Kangvansura, P., Chew, L. M., Kongmark, C., Santawaja, P., Ruland, H., Xia, W., et al. (2017). Effects of potassium and manganese promoters on nitrogen-doped carbon nanotube-supported iron catalysts for CO2 hydrogenation. Engineering 3 (3), 385–392. doi:10.1016/J.ENG.2017.03.013
Kattel, S., Yu, W., Yang, X., Yan, B., Huang, Y., Wan, W., et al. (2016). CO2 hydrogenation over oxide-supported PtCo catalysts: The role of the oxide support in determining the product selectivity. Angew. Chem. Int. Ed. Engl. 55 (28), 8100–8105. doi:10.1002/ange.201601661
Khodakov, A. Y., Chu, W., and Fongarland, P. (2007). Advances in the development of novel cobalt Fischer − Tropsch catalysts for synthesis of long-chain hydrocarbons and clean fuels. Chem. Rev. 107 (5), 1692–1744. doi:10.1021/cr050972v
Khodakov, A. Y. (2009). Fischer-Tropsch Synthesis: Relations between structure of cobalt catalysts and their catalytic performance. Catal. Today 144 (3–4), 251–257. doi:10.1016/j.cattod.2008.10.036
Kim, K. Y., Lee, H., Noh, W. Y., Shin, J., Han, S. J., Kim, S. K., et al. (2020b). Cobalt ferrite nanoparticles to form a catalytic Co-Fe alloy carbide phase for selective CO2 hydrogenation to light olefins. ACS Catal. 10 (15), 8660–8671. doi:10.1021/acscatal.0c01417
Kim, Y., Kwon, S., Song, Y., and Na, K. (2020a). Catalytic CO2 hydrogenation using mesoporous bimetallic spinel oxides as active heterogeneous base catalysts with long lifetime. J. CO2 Util. 36, 145–152. doi:10.1016/j.jcou.2019.11.005
Kosol, R., Guo, L., Kodama, N., Zhang, P., Reubroycharoen, P., Vitidsant, T., et al. (2021). Iron catalysts supported on nitrogen functionalized carbon for improved CO2 hydrogenation performance. Catal. Commun. 149, 106216. doi:10.1016/j.catcom.2020.106216
Landau, M. V., Meiri, N., Utsis, N., Vidruk Nehemya, R., and Herskowitz, M. (2017). Conversion of CO2, CO, and H2 in CO2 hydrogenation to fungible liquid fuels on Fe-based catalysts. Ind. Eng. Chem. Res. 56 (45), 13334–13355. doi:10.1021/acs.iecr.7b01817
Landau, M. V., Vidruk, R., and Herskowitz, M. (2014). Sustainable production of green feed from carbon dioxide and hydrogen. ChemSusChem 7 (3), 785–794. doi:10.1002/cssc.201301181
Li, W., Zhang, A., Jiang, X., Janik, M. J., Qiu, J., Liu, Z., et al. (2018). The anti-sintering catalysts: Fe–Co–Zr polymetallic fibers for CO2 hydrogenation to C2 = –C4 = –rich hydrocarbons. J. CO2 Util. 23, 219–225. doi:10.1016/j.jcou.2017.07.005
Li, Z., Liu, J., Shi, R., Waterhouse, G. I. N., Wen, X. D., and Zhang, T. (2021). Fe-based catalysts for the direct photohydrogenation of CO2 to value-added hydrocarbons. Adv. Energy Mat. 11 (12), 2002783–2002789. doi:10.1002/aenm.202002783
Liang, B., Duan, H., Sun, T., Ma, J., Liu, X., Xu, J., et al. (2019a). Effect of Na promoter on Fe-based catalyst for CO2 hydrogenation to alkenes. ACS Sustain. Chem. Eng. 7 (1), 925–932. doi:10.1021/acssuschemeng.8b04538
Liang, B., Sun, T., Ma, J., Duan, H., Li, L., Yang, X., et al. (2019b). Mn decorated Na/Fe catalysts for CO2 hydrogenation to light olefins. Catal. Sci. Technol. 9 (2), 456–464. doi:10.1039/c8cy02275e
Lino, A. V. P., Assaf, E. M., and Assaf, J. M. (2022). Production of light hydrocarbons at atmospheric pressure from CO2 hydrogenation using CexZr(1-x)O2 iron-based catalysts. J. CO2 Util. 55 (2021), 101805. doi:10.1016/j.jcou.2021.101805
Liu, B., Geng, S., Zheng, J., Jia, X., Jiang, F., and Liu, X. (2018). Unravelling the new roles of Na and Mn promoter in CO2 hydrogenation over Fe3O4-based catalysts for enhanced selectivity to light α-olefins. ChemCatChem 10 (20), 4718–4732. doi:10.1002/cctc.201800782
Liu, J., Li, K., Song, Y., Song, C., and Guo, X. (2021a). Selective hydrogenation of CO2to hydrocarbons: Effects of Fe3O4Particle size on reduction, carburization, and catalytic performance. Energy fuels. 35 (13), 10703–10709. doi:10.1021/acs.energyfuels.1c01265
Liu, J., Sun, Y., Jiang, X., Zhang, A., Song, C., and Guo, X. (2018a). Pyrolyzing ZIF-8 to N-doped porous carbon facilitated by iron and potassium for CO2 hydrogenation to value-added hydrocarbons. J. CO2 Util. 25, 120–127. doi:10.1016/j.jcou.2018.03.015
Liu, J., Zhang, A., Jiang, X., Liu, M., Sun, Y., Song, C., et al. (2018b). Selective CO2 hydrogenation to hydrocarbons on Cu-promoted Fe-based catalysts: Dependence on Cu-Fe interaction. ACS Sustain. Chem. Eng. 6 (8), 10182–10190. doi:10.1021/acssuschemeng.8b01491
Liu, J., Zhang, A., Jiang, X., Liu, M., Zhu, J., Song, C., et al. (2018c). Direct transformation of carbon dioxide to value-added hydrocarbons by physical mixtures of Fe5C2 and K-modified Al2O3. Ind. Eng. Chem. Res. 57 (28), 9120–9126. doi:10.1021/acs.iecr.8b02017
Liu, J., Zhang, A., Jiang, X., Zhang, G., Sun, Y., Liu, M., et al. (2019). Overcoating the surface of Fe-based catalyst with ZnO and nitrogen-doped carbon toward high selectivity of light olefins in CO2 hydrogenation. Ind. Eng. Chem. Res. 58 (10), 4017–4023. doi:10.1021/acs.iecr.8b05478
Liu, J., Zhang, A., Liu, M., Hu, S., Ding, F., Song, C., et al. (2017). Fe-MOF-derived highly active catalysts for carbon dioxide hydrogenation to valuable hydrocarbons. J. CO2 Util. 21, 100–107. doi:10.1016/j.jcou.2017.06.011
Liu, J., Zhang, G., Jiang, X., Wang, J., Song, C., and Guo, X. (2021b). Insight into the role of Fe5C2 in CO2 catalytic hydrogenation to hydrocarbons. Catal. Today 371, 162–170. doi:10.1016/j.cattod.2020.07.032
Liu, X., Zhang, C., Tian, P., Xu, M., Cao, C., Yang, Z., et al. (2021). Revealing the effect of sodium on iron-based catalysts for CO2 hydrogenation: Insights from calculation and experiment. J. Phys. Chem. C 125 (14), 7637–7646. doi:10.1021/acs.jpcc.0c11123
Martinelli, M., Visconti, C. G., Lietti, L., Forzatti, P., Bassano, C., and Deiana, P. (2014). CO2 reactivity on Fe-Zn-Cu-K Fischer-Tropsch synthesis catalysts with different K-loadings. Catal. Today 228, 77–88. doi:10.1016/j.cattod.2013.11.018
Mattia, D., Jones, M. D., O’Byrne, J. P., Griffiths, O. G., Owen, R. E., Sackville, E., et al. (2015). Towards carbon-neutral CO2 conversion to hydrocarbons. ChemSusChem 8 (23), 4064–4072. doi:10.1002/cssc.201500739
Minett, D. R., O’Byrne, J. P., Pascu, S. I., Plucinski, P. K., Owen, R. E., Jones, M. D., et al. (2014). Fe@CNT-monoliths for the conversion of carbon dioxide to hydrocarbons: Structural characterisation and Fischer-Tropsch reactivity investigations. Catal. Sci. Technol. 4 (9), 3351–3358. doi:10.1039/c4cy00616j
Mokou, C., Beisswenger, L., Vogel, H., Klemenz, S., and Albert, B. (2015). “Iron-catalyzed hydrogenation of carbon dioxide to hydrocarbons/fuels in condensed phase,” in 2015 5th International Youth Conference on Energy (IYCE), (Pisa, Italy: IEEE), 1–6.
Nasriddinov, K., Min, J-E., Park, H-G., Han, S. J., Chen, J., Jun, K-W., et al. (2022). Effect of Co, Cu, and Zn on FeAlK catalysts in CO2 hydrogenation to C 5+ hydrocarbons. Catal. Sci. Technol. 12 (3), 906–915. doi:10.1039/d1cy01980e
Ning, W., Wang, T., Chen, H., Yang, X., and Jin, Y. (2014). The effect of Fe2O3 crystal phases on CO2 hydrogenation. Acta Crystallogr. Sect. A Found. Adv. 70 (1), C49. doi:10.1371/journal.pone.0182955
Numpilai, T., Chanlek, N., Poo-Arporn, Y., Cheng, C. K., Siri-Nguan, N., Sornchamni, T., et al. (2020). Tuning interactions of surface-adsorbed species over Fe−Co/K−Al2O3 catalyst by different K contents: Selective CO2 hydrogenation to light olefins. ChemCatChem 12 (12), 3306–3320. doi:10.1002/cctc.202000347
Numpilai, T., Chanlek, N., Poo-Arporn, Y., Wannapaiboon, S., Cheng, C. K., Siri-Nguan, N., et al. (2019). Pore size effects on physicochemical properties of Fe-Co/K-Al 2 O 3 catalysts and their catalytic activity in CO2 hydrogenation to light olefins. Appl. Surf. Sci. 483, 581–592. doi:10.1016/j.apsusc.2019.03.331
Numpilai, T., Witoon, T., Chanlek, N., Limphirat, W., Bonura, G., Chareonpanich, M., et al. (2017). Structure–activity relationships of Fe-Co/K-Al2O3 catalysts calcined at different temperatures for CO2 hydrogenation to light olefins. Appl. Catal. A General 547, 219–229. doi:10.1016/j.apcata.2017.09.006
Ouyang, B., Xiong, S., Zhang, Y., Liu, B., and Li, J. (2017). The study of morphology effect of Pt/Co3O4 catalysts for higher alcohol synthesis from CO2 hydrogenation. Appl. Catal. A General 543, 189–195. doi:10.1016/j.apcata.2017.06.031
Owen, R. E., Cortezon-Tamarit, F., Calatayud, D. G., Evans, E. A., Mitchell, S. I. J., Mao, B., et al. (2020). Shedding light onto the nature of iron decorated graphene and graphite oxide nanohybrids for CO2 conversion at atmospheric pressure. ChemistryOpen 9 (2), 242–252. doi:10.1002/open.201900368
Owen, R. E., O’Byrne, J. P., Mattia, D., Plucinski, P., Pascu, S. I., and Jones, M. D. (2013b). Cobalt catalysts for the conversion of CO2 to light hydrocarbons at atmospheric pressure. Chem. Commun. 49 (99), 11683–11685. doi:10.1039/c3cc46791k
Owen, R. E., O’Byrne, J. P., Mattia, D., Plucinski, P., Pascu, S. I., and Jones, M. D. (2013a). Promoter effects on iron-silica fischer-tropsch nanocatalysts: Conversion of carbon dioxide to lower olefins and hydrocarbons at atmospheric pressure. Chempluschem 78 (12), 1536–1544. doi:10.1002/cplu.201300263
Owen, R. E., Plucinski, P., Mattia, D., Torrente-Murciano, L., Ting, V. P., and Jones, M. D. (2016). Effect of support of Co-Na-Mo catalysts on the direct conversion of CO2 to hydrocarbons. J. CO2 Util. 16, 97–103. doi:10.1016/j.jcou.2016.06.009
Piriyasurawong, K., Panpranot, J., Mekasuwandumrong, O., and Praserthdam, P. (2021). CO2 hydrogenation over FSP-made iron supported on cerium modified alumina catalyst. Catal. Today 375, 307–313. doi:10.1016/j.cattod.2020.03.007
Pokusaeva, Y. A., Koklin, A. E., Eliseev, O. L., Kazantsev, R. V., and Bogdan, V. I. (2020). Hydrogenation of carbon oxides over the Fe-based catalysts on the carbon support. Russ. Chem. Bull. 69 (2), 237–240. doi:10.1007/s11172-020-2751-5
Pour, A. N., Housaindokht, M. R., and Asil, A. G. (2018). Production of higher hydrocarbons from CO2 over nanosized iron catalysts. Chem. Eng. Technol. 41 (3), 479–488. doi:10.1002/ceat.201700490
Puga, A. V., and Corma, A. (2018). Hydrogenation of CO2 on nickel–iron nanoparticles under sunlight irradiation. Top. Catal. 61 (18–19), 1810–1819. doi:10.1007/s11244-018-1030-2
Qadir, M. I., Bernardi, F., Scholten, J. D., Baptista, D. L., and Dupont, J. (2019). Synergistic CO2 hydrogenation over bimetallic Ru/Ni nanoparticles in ionic liquids. Appl. Catal. B Environ. 252, 10–17. doi:10.1016/j.apcatb.2019.04.005
Qadir, M. I., Weilhard, A., Fernandes, J. A., De Pedro, I., Vieira, B. J. C., Waerenborgh, J. C., et al. (2018). Selective carbon dioxide hydrogenation driven by ferromagnetic RuFe nanoparticles in ionic liquids. ACS Catal. 8 (2), 1621–1627. doi:10.1021/acscatal.7b03804
Ramirez, A., Dutta Chowdhury, A., Dokania, A., Cnudde, P., Caglayan, M., Yarulina, I., et al. (2019b). Effect of zeolite topology and reactor configuration on the direct conversion of CO2 to light olefins and aromatics. ACS Catal. 9 (7), 6320–6334. doi:10.1021/acscatal.9b01466
Ramirez, A., Gevers, L., Bavykina, A., Ould-Chikh, S., and Gascon, J. (2018). Metal organic framework-derived iron catalysts for the direct hydrogenation of CO2 to short chain olefins. ACS Catal. 8 (10), 9174–9182. doi:10.1021/acscatal.8b02892
Ramirez, A., Gong, X., Caglayan, M., Nastase, S. A. F., Abou-Hamad, E., Gevers, L., et al. (2021). Selectivity descriptors for the direct hydrogenation of CO2 to hydrocarbons during zeolite-mediated bifunctional catalysis. Nat. Commun. 12 (1), 5914. doi:10.1038/s41467-021-26090-5
Ramirez, A., Ould-Chikh, S., Gevers, L., Chowdhury, A. D., Abou-Hamad, E., Aguilar-Tapia, A., et al. (2019a). Tandem conversion of CO2 to valuable hydrocarbons in highly concentrated potassium iron catalysts. ChemCatChem 11 (12), 2879–2886. doi:10.1002/cctc.201900762
Rane, S., Borg, O., Rytter, E., and Holmen, A. (2012). Relation between hydrocarbon selectivity and cobalt particle size for alumina supported cobalt Fischer-Tropsch catalysts. Appl. Catal. A General 437–438, 10–17. doi:10.1016/j.apcata.2012.06.005
Rodemerck, U., Holeňa, M., Wagner, E., Smejkal, Q., Barkschat, A., and Baerns, M. (2013). Catalyst development for CO2 hydrogenation to fuels. ChemCatChem 5 (7), 1948–1955. doi:10.1002/cctc.201200879
Samanta, A., Landau, M. V., Vidruk-Nehemya, R., and Herskowitz, M. (2017). CO2 hydrogenation to higher hydrocarbons on K/Fe-Al-O spinel catalysts promoted with Si, Ti, Zr, Hf, Mn and Ce. Catal. Sci. Technol. 7 (18), 4048–4063. doi:10.1039/c7cy01118k
Satthawong, R., Koizumi, N., Song, C., and Prasassarakich, P. (2013). Bimetallic Fe-Co catalysts for CO2 hydrogenation to higher hydrocarbons. J. CO2 Util. 3–4, 102–106. doi:10.1016/j.jcou.2013.10.002
Satthawong, R., Koizumi, N., Song, C., and Prasassarakich, P. (2014). Comparative study on CO2 hydrogenation to higher hydrocarbons over Fe-based bimetallic catalysts. Top. Catal. 57 (6–9), 588–594. doi:10.1007/s11244-013-0215-y
Satthawong, R., Koizumi, N., Song, C., and Prasassarakich, P. (2015). Light olefin synthesis from CO2 hydrogenation over K-promoted Fe-Co bimetallic catalysts. Catal. Today 251, 34–40. doi:10.1016/j.cattod.2015.01.011
Sedighi, M., and Mohammadi, M. (2020). CO2 hydrogenation to light olefins over Cu-CeO2/SAPO-34 catalysts: Product distribution and optimization. J. CO2 Util. 35, 236–244. doi:10.1016/j.jcou.2019.10.002
Shafer, W. D., Jacobs, G., Graham, U. M., Hamdeh, H. H., and Davis, B. H. (2019). Increased CO2 hydrogenation to liquid products using promoted iron catalysts. J. Catal. 369, 239–248. doi:10.1016/j.jcat.2018.11.001
Shi, Z., Yang, H., Gao, P., Chen, X., Liu, H., Zhong, L., et al. (2018a). Effect of alkali metals on the performance of CoCu/TiO2 catalysts for CO2 hydrogenation to long-chain hydrocar-bons. Chin. J. Catal. 39 (8), 1294–1302. doi:10.1016/S1872-2067(18)63086-4
Shi, Z., Yang, H., Gao, P., Li, X., Zhong, L., Wang, H., et al. (2018b). Direct conversion of CO2 to long-chain hydrocarbon fuels over K-promoted CoCu/TiO2 catalysts. Catal. Today 311, 65–73. doi:10.1016/j.cattod.2017.09.053
Skrypnik, A. S., Yang, Q., Matvienko, A. A., Bychkov, V. Y., Tulenin, Y. P., Lund, H., et al. (2021). Understanding reaction-induced restructuring of well-defined FexOyCz compositions and its effect on CO2 hydrogenation. Appl. Catal. B Environ. 291, 120121. doi:10.1016/j.apcatb.2021.120121
Srisawad, N., Chaitree, W., Mekasuwandumrong, O., Shotipruk, A., Jongsomjit, B., and Panpranot, J. (2012). CO2 hydrogenation over Co/Al 2O 3 catalysts prepared via a solid-state reaction of fine gibbsite and cobalt precursors. Reac. Kinet. Mech. Cat. 107 (1), 179–188. doi:10.1007/s11144-012-0459-8
Tarasov, A. L., Isaeva, V. I., Tkachenko, O. P., Chernyshev, V. V., and Kustov, L. M. (2018a). Conversion of CO2 into liquid hydrocarbons in the presence of a Co-containing catalyst based on the microporous metal-organic framework MIL-53(Al). Fuel Process. Technol. 176, 101–106. doi:10.1016/j.fuproc.2018.03.016
Tarasov, A. L., Redina, E. A., and Isaeva, V. I. (2018b). Effect of the nature of catalysts on their properties in the hydrogenation of carbon dioxide. Russ. J. Phys. Chem. 92 (10), 1889–1892. doi:10.1134/s0036024418100345
Torrente-Murciano, L., Chapman, R. S. L., Narvaez-Dinamarca, A., Mattia, D., and Jones, M. D. (2016). Effect of nanostructured ceria as support for the iron catalysed hydrogenation of CO2 into hydrocarbons. Phys. Chem. Chem. Phys. 18 (23), 15496–15500. doi:10.1039/c5cp07788e
Tursunov, O., and Tilyabaev, Z. (2019). Hydrogenation of CO2 over Co supported on carbon nanotube, carbon nanotube-Nb2O5, carbon nanofiber, low-layered graphite fragments and Nb2O5. J. Energy Inst. 92 (1), 18–26. doi:10.1016/j.joei.2017.12.004
Visconti, C. G., Martinelli, M., Falbo, L., Fratalocchi, L., and Lietti, L. (2016). CO2 hydrogenation to hydrocarbons over Co and Fe-based Fischer-Tropsch catalysts. Catal. Today 277, 161–170. doi:10.1016/j.cattod.2016.04.010
Visconti, C. G., Martinelli, M., Falbo, L., Infantes-Molina, A., Lietti, L., Forzatti, P., et al. (2017). CO2 hydrogenation to lower olefins on a high surface area K-promoted bulk Fe-catalyst. Appl. Catal. B Environ. 200, 530–542. doi:10.1016/j.apcatb.2016.07.047
Wang, H., Hodgson, J., Shrestha, T. B., Thapa, P. S., Moore, D., Wu, X., et al. (2014). Carbon dioxide hydrogenation to aromatic hydrocarbons by using an iron/iron oxide nanocatalyst. Beilstein J. Nanotechnol. 5 (1), 760–769. doi:10.3762/bjnano.5.88
Wang, J., You, Z., Zhang, Q., Deng, W., and Wang, Y. (2013). Synthesis of lower olefins by hydrogenation of carbon dioxide over supported iron catalysts. Catal. Today 215, 186–193. doi:10.1016/j.cattod.2013.03.031
Wang, L., He, S., Wang, L., Lei, Y., Meng, X., and Xiao, F. S. (2019). Cobalt-nickel catalysts for selective hydrogenation of carbon dioxide into ethanol. ACS Catal. 9 (12), 11335–11340. doi:10.1021/acscatal.9b04187
Wang, L., Wang, L., Zhang, J., Liu, X., Wang, H., Zhang, W., et al. (2018). Selective hydrogenation of CO2 to ethanol over cobalt catalysts. Angew. Chem. Int. Ed. 57 (21), 6104–6108. doi:10.1002/anie.201800729
Wang, S., Wu, T., Lin, J., Ji, Y., Yan, S., Pei, Y., et al. (2020). Iron-potassium on single-walled carbon nanotubes as efficient catalyst for CO2 hydrogenation to heavy olefins. ACS Catal. 10 (11), 6389–6401. doi:10.1021/acscatal.0c00810
Wang, W., Jiang, X., Wang, X., and Song, C. (2018). Fe-Cu bimetallic catalysts for selective CO2 hydrogenation to olefin-rich C2+ hydrocarbons. Ind. Eng. Chem. Res. 57 (13), 4535–4542. doi:10.1021/acs.iecr.8b00016
Wang, W., Wang, S., Ma, X., and Gong, J. (2011). Recent advances in catalytic hydrogenation of carbon dioxide. Chem. Soc. Rev. 40 (7), 3703–3727. doi:10.1039/c1cs15008a
Wang, X., Yang, G., Zhang, J., Chen, S., Wu, Y., Zhang, Q., et al. (2016). Synthesis of isoalkanes over a core (Fe-Zn-Zr)-shell (zeolite) catalyst by CO2 hydrogenation. Chem. Commun. 52 (46), 7352–7355. doi:10.1039/c6cc01965j
Wang, X., Zeng, C. Y., Gong, N., Zhang, T., Wu, Y., Zhang, J., et al. (2021). Effective suppression of CO selectivity for CO2 hydrogenation to high-quality gasoline. ACS Catal. 11 (3), 1528–1547. doi:10.1021/acscatal.0c04155
Wang, Y., Y., Kazumi, S., Gao, W., Gao, X., Li, H., Guo, X., et al. (2020). Direct conversion of CO2 to aromatics with high yield via a modified Fischer-Tropsch synthesis pathway. Appl. Catal. B Environ. 269, 118792. doi:10.1016/j.apcatb.2020.118792
Wei, C., Tu, W., Jia, L., Liu, Y., Lian, H., Wang, P., et al. (2020). The evolutions of carbon and iron species modified by Na and their tuning effect on the hydrogenation of CO2 to olefins. Appl. Surf. Sci. 525, 146622. doi:10.1016/j.apsusc.2020.146622
Wei, J., Ge, Q., Yao, R., Wen, Z., Fang, C., Guo, L., et al. (2017). Directly converting CO2 into a gasoline fuel. Nat. Commun. 8, 15174–15178. doi:10.1038/ncomms15174
Wei, J., Sun, J., Wen, Z., Fang, C., Ge, Q., and Xu, H. (2016). New insights into the effect of sodium on Fe3O4- based nanocatalysts for CO2 hydrogenation to light olefins. Catal. Sci. Technol. 6 (13), 4786–4793. doi:10.1039/c6cy00160b
Willauer, H. D., Ananth, R., Olsen, M. T., Drab, D. M., Hardy, D. R., and Williams, F. W. (2013). Modeling and kinetic analysis of CO2 hydrogenation using a Mn and K-promoted Fe catalyst in a fixed-bed reactor. J. CO2 Util. 3–4, 56–64. doi:10.1016/j.jcou.2013.10.003
Wu, T., Lin, J., Cheng, Y., Tian, J., Wang, S., Xie, S., et al. (2018). Porous graphene-confined Fe-K as highly efficient catalyst for CO2 direct hydrogenation to light olefins. ACS Appl. Mat. Interfaces 10 (28), 23439–23443. doi:10.1021/acsami.8b05411
Xie, C., Chen, C., Yu, Y., Su, J., Li, Y., Somorjai, G. A., et al. (2017). Tandem catalysis for CO2 hydrogenation to C2-C4 hydrocarbons. Nano Lett. 17 (6), 3798–3802. doi:10.1021/acs.nanolett.7b01139
Xie, T., Wang, J., Ding, F., Zhang, A., Li, W., Guo, X., et al. (2017). CO2 hydrogenation to hydrocarbons over alumina-supported iron catalyst: Effect of support pore size. J. CO2 Util. 19, 202–208. doi:10.1016/j.jcou.2017.03.022
Xu, Q., Xu, X., Fan, G., Yang, L., and Li, F. (2021). Unveiling the roles of Fe-Co interactions over ternary spinel-type ZnCoxFe2-xO4 catalysts for highly efficient CO2 hydrogenation to produce light olefins. J. Catal. 400, 355–366. doi:10.1016/j.jcat.2021.07.002
Xu, Y., Shi, C., Liu, B., Wang, T., Zheng, J., Li, W., et al. (2019). Selective production of aromatics from CO2. Catal. Sci. Technol. 9 (3), 593–610. doi:10.1039/c8cy02024h
Yao, R., Wei, J., Ge, Q., Xu, J., Han, Y., Xu, H., et al. (2021). Structure sensitivity of iron oxide catalyst for CO2 hydrogenation. Catal. Today 371, 134–141. doi:10.1016/j.cattod.2020.07.073
Yao, Y., Liu, X., Hildebrandt, D., and Glasser, D. (2012). The effect of CO2 on a cobalt-based catalyst for low temperature Fischer-Tropsch synthesis. Chem. Eng. J. 193–194, 318–327. doi:10.1016/j.cej.2012.04.045
You, Z., Deng, W., Zhang, Q., and Wang, Y. (2013). Hydrogenation of carbon dioxide to light olefins over non-supported iron catalyst. Chin. J. Catal. 34 (5), 956–963. doi:10.1016/S1872-2067(12)60559-2
Yuan, F., Zhang, G., Zhu, J., Ding, F., Zhang, A., Song, C., et al. (2021). Boosting light olefin selectivity in CO2 hydrogenation by adding Co to Fe catalysts within close proximity. Catal. Today 371, 142–149. doi:10.1016/j.cattod.2020.07.072
Zhang, J., Lu, S., Su, X., Fan, S., Ma, Q., and Zhao, T. (2015). Selective formation of light olefins from CO2 hydrogenation over Fe-Zn-K catalysts. J. CO2 Util. 12, 95–100. doi:10.1016/j.jcou.2015.05.004
Zhang, J., Su, X., Wang, X., Ma, Q., Fan, S., and Zhao, T. S. (2018). Promotion effects of Ce added Fe–Zr–K on CO2 hydrogenation to light olefins. Reac. Kinet. Mech. Cat. 124 (2), 575–585. doi:10.1007/s11144-018-1377-1
Zhang, S., Liu, X., Shao, Z., Wang, H., and Sun, Y. (2020). Direct CO2 hydrogenation to ethanol over supported Co2C catalysts: Studies on support effects and mechanism. J. Catal. 382, 86–96. doi:10.1016/j.jcat.2019.11.038
Zhang, Z., Huang, G., Tang, X., Yin, H., Kang, J., Zhang, Q., et al. (2022a). Zn and Na promoted Fe catalysts for sustainable production of high-valued olefins by CO2 hydrogenation. Fuel 309, 122105. doi:10.1016/j.fuel.2021.122105
Zhang, Z., Liu, Y., Jia, L., Sun, C., Chen, B., Liu, R., et al. (2022b). Effects of the reducing gas atmosphere on performance of FeCeNa catalyst for the hydrogenation of CO2 to olefins. Chem. Eng. J. 428, 131388. doi:10.1016/j.cej.2021.131388
Zhang, Z., Wei, C., Jia, L., Liu, Y., Sun, C., Wang, P., et al. (2020). Insights into the regulation of FeNa catalysts modified by Mn promoter and their tuning effect on the hydrogenation of CO2 to light olefins. J. Catal. 390, 12–22. doi:10.1016/j.jcat.2020.07.020
Zhao, H., Guo, L., Gao, W., Chen, F., Wu, X., Wang, K., et al. (2021). Multi-promoters regulated iron catalyst with well-matching reverse water-gas shift and chain propagation for boosting CO2 hydrogenation. J. CO2 Util. 52, 101700. doi:10.1016/j.jcou.2021.101700
Keywords: modified fischer-tropsch synthesis, carbon dioxide, reverse water-gas-shift reaction, olefins, higher hydrocarbons
Citation: Tavares M, Westphalen G, Araujo Ribeiro de Almeida JM, Romano PN and Sousa-Aguiar EF (2022) Modified fischer-tropsch synthesis: A review of highly selective catalysts for yielding olefins and higher hydrocarbons. Front. Nanotechnol. 4:978358. doi: 10.3389/fnano.2022.978358
Received: 25 June 2022; Accepted: 01 August 2022;
Published: 24 August 2022.
Edited by:
Marco Aurélio Suller Garcia, Federal University of Maranhão, BrazilReviewed by:
Yuechang Wei, China University of Petroleum, Beijing, ChinaDedong He, Kunming University of Science and Technology, China
Copyright © 2022 Tavares, Westphalen, Araujo Ribeiro de Almeida, Romano and Sousa-Aguiar. This is an open-access article distributed under the terms of the Creative Commons Attribution License (CC BY). The use, distribution or reproduction in other forums is permitted, provided the original author(s) and the copyright owner(s) are credited and that the original publication in this journal is cited, in accordance with accepted academic practice. No use, distribution or reproduction is permitted which does not comply with these terms.
*Correspondence: Eduardo Falabella Sousa-Aguiar, ZWZhbGFiZWxsYUBlcS51ZnJqLmJy