- 1Department of Chemical and Biological Engineering, University of Colorado Boulder, Boulder, CO, United States
- 2Department of Chemistry, University of Colorado Boulder, Boulder, CO, United States
- 3Department of Chemistry, Stanford University, Stanford, CA, United States
- 4Department of Materials Science and Engineering, Stanford University, Stanford, CA, United States
- 5BioFrontiers Institute, University of Colorado Boulder, Boulder, CO, United States
- 6Department of Physics, University of Colorado Boulder, Boulder, CO, United States
- 7Materials Science and Engineering Program, University of Colorado Boulder, Boulder, CO, United States
- 8National Renewable Energy Laboratory, Golden, CO, United States
Dynamic windows based on reversible metal electrodeposition are an attractive way to enhance the energy efficiency of buildings and show great commercial potential. Dynamic windows that rely on liquid electrolytes are at risk of short circuiting when two electrodes contact, especially at larger-scale. Here we developed a poly (vinyl alcohol) (PVA) gel polymer electrolyte (GPE) with 85% transmittance, that is, sufficiently stiff to act as a separator. The GPE is implemented into windows that exhibit comparable electrochemical and optical properties to windows using a liquid electrolyte. Furthermore, the GPE enables the fabrication of windows with dual-working electrodes (WE) and a metal mesh counter electrode in the center without short-circuiting. Our dual-WE PVA GPE window reaches the 0.1% transmittance state in 101 s, more than twice the speed of liquid windows with one working electrode (207 s). Additionally, each side of the dual-WE GPE window can be tinted individually to demonstrate varied optical effects (i.e., more reflective, or more absorptive), providing users and intelligent building systems with greater control over the appearance and performance of the windows in a single device architecture.
1 Introduction
Dynamic windows tune visible light and heat flow while maintaining views, thereby saving 10%–20% of energy for buildings and vehicles over static low emissivity coatings (Eleanor et al., 2004; Sbar et al., 2012). In addition to energy savings, a study has shown that occupants in office buildings with dynamic windows will be healthier, happier and more productive (Hedge and Nou, 2018). By reducing glare and optimizing both the temperature and light flow in an indoor work environment, employee productivity can increase by up to 2% (Prnewswire, 2018). Currently there are many technologies using electrochromic oxides and organic molecules to tune the color and transmission of the window (Barile et al., 2017). Compared with these technologies, dynamic windows based on reversible metal deposition (RME) have the advantage of potential low cost, neutral color, and great contrast ratio (Barile et al., 2017; Jeong et al., 2017; Hernandez et al., 2018; Strand et al., 2018; Hernandez et al., 2020; Eh et al., 2020; Kimura et al., 2020; Poh et al., 2021; Guo et al., 2022; Kimura et al., 2022; Song et al., 2022; Tao et al., 2022). These windows operate by the reversible electrodeposition of metals ions, e.g., Bi3+ and Cu2+, to their metallic form on a Pt-coated indium tin oxide (ITO) transparent electrode. A copper mesh works as a counter electrode to balance the metal ions in the electrolyte (Hernandez et al., 2018; Strand et al., 2018). Recently, we reported poly (vinyl) alcohol as a growth inhibitor to reversibly deposit metal films with more uniform morphology (Hernandez et al., 2020). Previously, the electrolyte implemented in these windows consisted of Bi3+ and Cu2+ ions in an liquid, aqueous ClO4− based electrolyte (Hernandez et al., 2020).
Though aqueous liquid electrolytes often have higher ionic conductivities than ones with high polymer content (Alesanco et al., 2018), dynamic windows with liquid electrolytes face problems including leakage and short circuiting, especially for large-scale windows in fenestration applications. Polymer gel electrolytes are attractive due to their ability to act as a physical separator between the two electrodes to avoid short-circuiting (Luo et al., 2015; Alipoori et al., 2020). For this study, a crosslinked porous polyvinyl alcohol (PVA) gel polymer electrolyte (GPE) is synthesized as a physical separator where the polymer backbone provides mechanical structure. The pores are filled with BiCu ClO4 liquid electrolyte which dictates ionic conductivity based on concentration. To build strong three-dimensional porous PVA frameworks to infill with aqueous electrolyte, physical crosslinking is induced by “freeze” (5°C) -thaw (25°C) phase separation cycles to form PVA gel crystallites (Hassan and Peppas, 2000; Lozinsky, 1998), (Hyon et al., 1989). As previously demonstrated, dimethyl sulfoxide (DMSO) is an attractive choice as a cosolvent with H2O to synthesize highly-transparent PVA gels due to its miscibility with water and distinct hydrogen bonding properties (Hassan and Peppas, 2000; Hou et al., 2015). When the weight ratio of DMSO: H2O is over 2:1, hydrogen bonds formed between DMSO and PVA limit the growth of PVA crystalline regions, resulting in a small volume of crystallites and thus a very high transparency in gels (Kanaya et al., 2012). As such, the optimized ratio used in our GPE windows was 4:1 DMSO: H2O (weight percent ratio) which results in the highest transparency gel (98%) (Supplementary Figure S1). This high-transparent physically cross-linked PVA gel was implemented into RME dynamic windows (PVA GPE window), and the PVA GPE window can be tinted below 0.1% visible light transmittance, exhibits color-neutral transmittance, and has a comparable coloration efficiency to that of liquid windows (mean value: 18.4 cm2/C for PVA GPE window, 18.8 cm2/C for liquid window, to 0.1% transmission).
Importantly, the use of PVA GPE in windows provides opportunities for dual-working electrode (dual-WE) windows without short circuiting. Dual-WE windows are made by incorporating a second piece of ITO-glass and integrating the Cu mesh counter electrode within the electrolytic matrix to the center layer of the window. Compared with single-WE windows, dual-WE windows are more susceptible to short circuits because there is a higher potential for the middle free-standing mesh to touch the working electrodes, as shown in Figure 1A. Substituting the liquid electrolyte with GPE as the physical separator prevents short-circuiting without losing significant transmittance in this dual-WE device architecture (Figure 1B). With this new device architecture, we can plate metal on either sheet of ITO separately or tint both sheets of ITO simultaneously. Metal viewed through the ITO appears mirrorlike as the light encounters a smooth ITO-metal interface. If instead it is viewed from the other side, the light interacts with the rough top surface of the metal film, causing strong absorption and a matte appearance (Strand et al., 2021). Thus, occupants can choose to tint either side of the dual-WE PVA GPE window to the same transmittance but get an absorptive or reflective visual effect according to their needs. Further, by tinting both sides of ITOs at the same time, dual-WE PVA GPE dynamic windows exhibit fast switching speed.
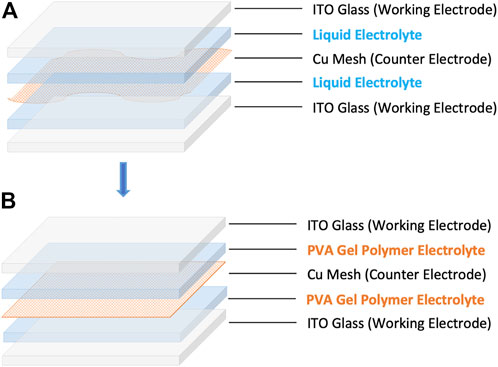
FIGURE 1. Schematic diagram of dual-working electrode windows with liquid electrolyte (A) and PVA gel polymer electrolyte (B).
2 Materials and methods
2.1 Liquid electrolyte preparation
Chemicals were bought and used without purification. The electrolyte used for RME consisted of 10 mM Cu(ClO4)2 • 6H2O (ACROS Organics), 10 mM BiOClO4 • H2O (GFS Chemicals), 10 mM HClO4 (Alfa Aesar), 1 M LiClO4 • 3H2O (ACROS Organics) with 0.1 w/v% PVA (31,000–50,000 g/mol, 87% hydrolyzed, Aldrich) additive. PVA was added last and stirred at 1200 rpm and 60–70°C until dissolved, about 1 h.
2.2 PVA gel polymer electrolyte preparation
10 wt% 89–98 k PVA (99% hydrolyzed) was added to wt. 80:20 DMSO: H2O solvent, dissolved by stirring at 90°C for 2 h, and processed under the two freeze-thaw cycles under vacuum to make PVA gels. After the freeze-thaw cycling, PVA gels are stirred in three separate fresh DI water baths for 1h, and then soaked in DI water for another 24 h to remove extra DMSO to form PVA hydrogels. Then they were directly soaked in baths of BiCu ClO4 with 0.1wt% PVA additive electrolyte for 24 h to replace the water in the hydrogel with enough liquid electrolyte to get PVA gel polymer electrolyte (GPE).
2.3 Pt-modified ITO working electrode preparation
Indium tin oxide (ITO) on glass substrates (Xinyan Technology Ltd., sheet resistance of 10 Ω □−1) were cleaned by sonication in 10% Extran in DI water solution, pure DI water, acetone, then isopropyl alcohol for 15 min each. After, the substrates were dried with N2 then cleaned in a UV-Ozone cleaner for 15 min. The ITO was then placed in a 10 mM 3-mercaptopropionic acid in ethanol solution and placed on a shaker for 24 h. The ITO substrates were then rinsed with ethanol then water before being placed in a Pt-nanoparticle solution (Sigma Aldrich) diluted 1:19 with DI water and placed on a shaker for 24–72 h. The substrates were then rinsed with DI water, dried with N2, then annealed at 250°C for 25 min before use.
2.4 RME dynamic window fabrication
PVA Additive Liquid Window: Two-electrode devices used Pt-modified ITO (5 cm2 × 5 cm2) on glass substrates (Pt-ITO) as a working electrode and a Cu metal counter electrode. Butyl rubber edge seal (Quanex: Solargain edge tape LP03, 1.5 mm thickness) separated the two electrodes and encapsulated the electrolyte between the Pt-ITO and the back piece of glass. Conductive tape (Conducty Z22, ElectricMosaic) was used to make electrical contact with the working electrode.
PVA GPE Window (Sandwiched for Testing): First, the “free-standing” PVA gel is made in a mold (made by rubber edge seal on a glass substrate) and is peeled off after freeze-thaw cycling and then soaked in DI water and PVA additive liquid electrolyte in this order. The Cu mesh is adhered to the backside glass using 3 M tapes. Then the Cu mesh-glass with “free-standing GPE” and the ITO-glass (5 cm2 × 5 cm2) are “sandwiched” with small clamps. The pressure provided by the clamps avoids potential optical problems as the PVA GPE is compressed evenly in each direction, and the contact between PVA GPE and ITO-glass (5 cm2 × 5 cm2) will be more uniform (transmittance of the whole device −70%). Furthermore, this method provides a convenient way to make windows. PVA gels can be cut into any size and shape fitting for different applications.
PVA GPE Window (Device with Sealings for Durability Testing): First, the PVA gel is made in the half-device architecture with Cu mesh, rubber edge seals, and the backside glass through freeze-thaw cycling. And the half device is soaked in DI water and PVA additive liquid electrolyte in this order. The ITO-glass is then compressed onto the half device to seal by rubber edge seals. Silicone seals are used for additional secure sealing.
2.5 Electrochemical characterization
Electrochemical experiments were run using a BioLogic SP-50 or SP-150 potentiostat. Two-electrode devices were cycled at −0.7 V until the privacy state was reached for window tinting and +0.7 V until transparency was restored for window bleaching.
2.6 Optical characterization
Ocean Optics OCEAN FX Miniature and Flame Miniature spectrometers were used in a standard configuration with an Ocean Optics halogen light source (HL-2000) for most transmission and specular reflection measurements.
Total Reflection measurements were conducted using a 135 mm 819C Series Spectralon Collimated Beam integrating sphere. The remaining transmission measurements (total and diffuse transmission) were conducted using a Varian CARY 500 UV-Vis-NIR Spectrophotometer (Labsphere DRA-CA-5500) equipped with a 150 mm integrating sphere. The haze coefficient values, quantifying the amounts of scattered light, were calculated based on the total and diffused transmission measurements using the integrating sphere following the ASTM D1003 (Standard Test Method for Haze and Luminous Transmittance), commonly used for haze measurements in windows applications.
2.7 Mechanical characterization
Hydrogels and GPEs were formed at a thickness of 5 mm between glass slides and a plug was cut to a diameter of 5 mm with a biopsy punch. The diameter and height of each hydrogel/GPE plug were measured using calipers. Hydrogels/GPEs were compressed to 30% strain at a rate of 10% strain/min (MTS Synergie 100). The compressive modulus (n = 3) was measured from the slope of the linear region of the stress-strain curve from 10% to 15% strain.
2.8 Other characterization
Scanning Electron Microscopy-Energy Dispersive Spectroscopy (SEM-EDS) was run using a HITACHI SU3500 scanning electron microscope operated at an accelerating voltage of 5/15 kV and equipped with an EDS detector.
3 Results
3.1 Single-working electrode PVA gel polymer electrolyte window’s performance
To synthesize a gel polymer electrolyte with acceptable haze, proper mechanical strength and ionic conductivity, we prepared nine PVA gels with varied PVA precursor molecular weights (31–50 k, 89–98 k, and 146–186 k) and varied PVA mass fractions in the DMSO: H2O solutions (5 wt%, 10 wt%, and 20 wt%). Considering the solubility limit of PVA precursors in DMSO: H2O solvent and gel formation, we chose three PVA gels for the exploration: 5 wt% 146–186 k, 10 wt%, 89–98 k, 20 wt% 31–50 k PVA gels (Supplementary Table S1).
To systematically evaluate the gels in windows, we assessed the optical and mechanical properties as well as ionic conductivity for 5 wt% 146–186 k, 10 wt% 89–98 k, 20 wt% 31–50 k PVA GPE. Considering 10 wt% 89–98 k PVA GPE has the largest Young’s modulus (148 kPa), low haze (2.0%), and high ionic conductivity (liquid electrolyte filling 91%), it was chosen as the suitable material to be implemented into our windows. Details are noted in SI GPE choice section, including Supplementary Figures S2–S9.
After selecting the 10 wt% 89–98 k PVA GPE as the most suitable material, we implemented it into our RME dynamic window in a “sandwiched” way where freestanding PVA GPE is clamped between the two electrodes for testing.
3.1.1 Comparable optical and electrochemical performance with liquid windows
To evaluate the selected GPE’s performance, its optical and electrochemical behaviors were characterized and compared with liquid windows. The transmission and specular reflection spectra show the comparable optical behaviors of single-WE PVA GPE window and 0.1 wt% PVA additive liquid window (Figures 2A,B, in each left as GPE windows and right as liquid windows). Coloration efficiency, current and charge density vs. time curves for the single-WE PVA GPE window are plotted in Figures 2C–E. Single-WE PVA GPE window has similar coloration efficiency as the 0.1 wt% PVA additive liquid window depending on the desired contrast (Figure 2C), a further testament to the suitability of this gel in an RME dynamic window. The charge density vs. time curve (Figure 2E) shows that the tinting speed of single-WE PVA GPE windows is slower than PVA-additive liquid windows, which can be explained by the lower ionic conductivity of GPE compared with pure liquid electrolyte.
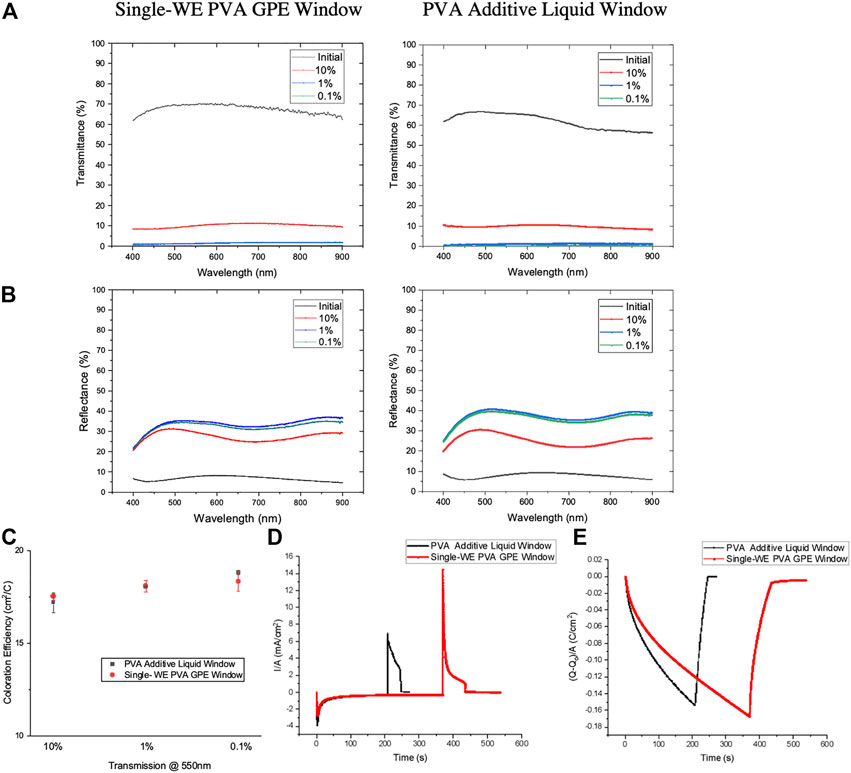
FIGURE 2. Comparison between liquid electrolyte window and single working electrode PVA GPE window. (A), Transmission spectra of single-WE PVA GPE window (left) and liquid window (right). (B), Specular reflection spectra single WE PVA GPE window (left) and liquid window (right). (C–E), Coloration efficiency at various window transmittance states (C), current density vs. time curve (D) and charge density vs. time curve (E) for windows using liquid electrolyte (black) and PVA GPE (red).
3.1.2 Morphologies of metal deposits
We used SEM to characterize the metal film morphology in a PVA GPE window at different tinting states. Figure 3 shows SEM images of Bi-Cu metal deposition on ITO at the state of 10%, 1%, 0.1% transmittance of a single-WE PVA GPE window. The images show that the diameter of metal deposit particles gradually increases with increasing tinting time. The morphology of the metal films is similar with and without the polymer gel (Supplementary Figure S10). Therefore, we infer that there is probably a thin layer of electrolyte between the GPE and the ITO-glass electrode, which may be caused by the strong hydrophilicity of the PVA gel framework. If the metal only grows where the aperture of the GPE is in contact with the ITO-glass, the metal deposition layer would not be as shown in the SEM image in Figure 3.
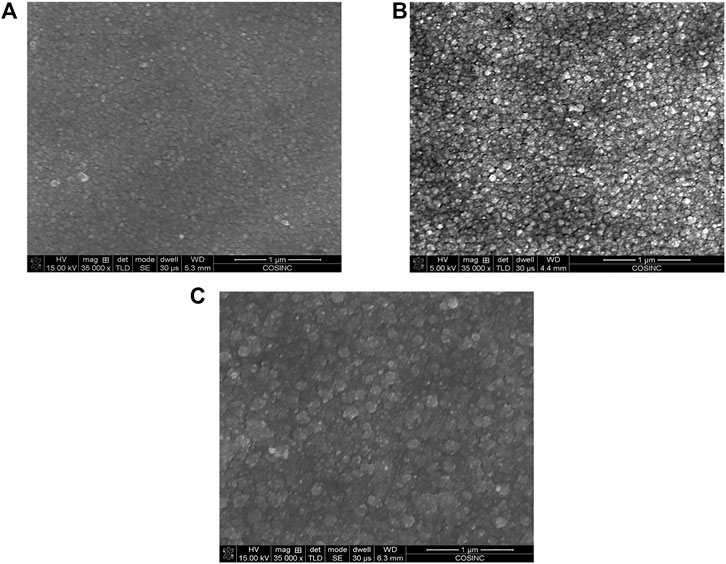
FIGURE 3. SEM images of Bi-Cu depositions on Pt-ITO at 10% (A), 1% (B), 0.1% (C) transmittance states for PVA GPE windows.
3.2 Dual-working electrode PVA gel polymer electrolyte window’s performance
3.2.1 Different optical performance by tinting one side of the GPE window
As stated above, substituting liquid electrolytes with GPE as the physical separator prevents short-circuiting in the dual-WE device architecture. Figure 4A shows a fully assembled dual-WE PVA GPE window with two pieces of ITO-glass working electrodes and one Cu mesh counter electrode in the middle. With this new device architecture, we can tint either side of ITO separately or tint both sides of ITO at the same time. In fact, the side of ITO tinted has a strong impact on the resulting visual effect. If the front side ITO is tinted, the front side of our window will appear more reflective (Figure 4B) (looking directly at the plated film), while the back side of the device is more absorptive (Figure 4C). If only the back side ITO is tinted, these visual effects will be switched. Figures 4D,E show the specular and diffuse reflectance of a dual-WE PVA GPE window from the front and the back side, respectively, with the front side ITO-glass tinted. The higher proportion of diffuse reflectance in the total reflectance implies a more absorptive film.
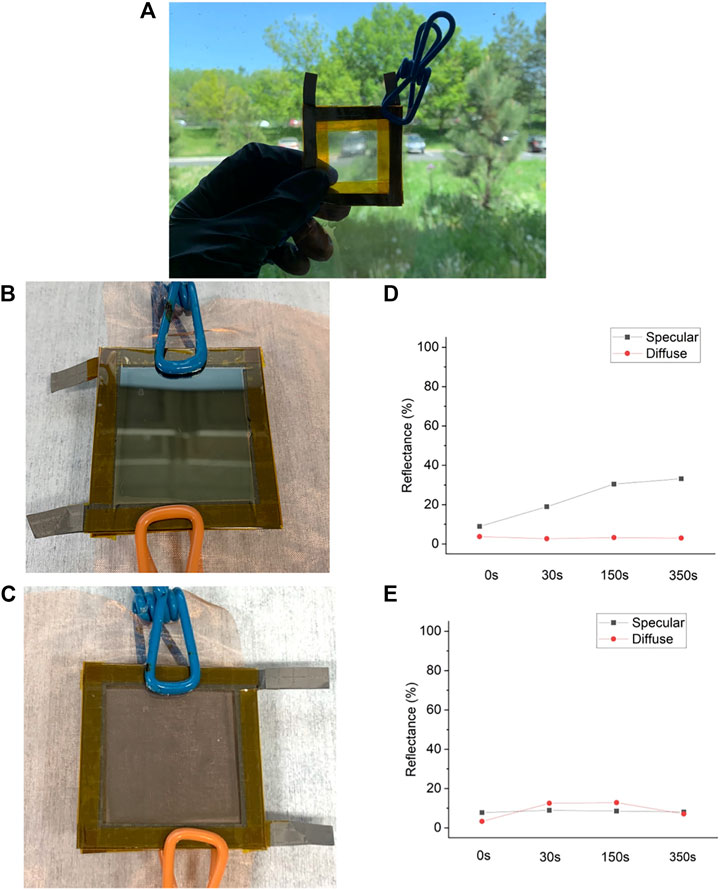
FIGURE 4. (A) Photo of a real dual-WE PVA GPE window. (B,C) If only one side is tinted, the tinted side is more reflective and the untinted side is more absorptive. Photograph of the window when it has 1% transmittance at 550 nm from the tinted (B) and untinted side (C). Specular and diffuse reflectance from the tinted (D) and untinted side (E) at initial, 10%, 1%, 0.1% transmission states.
3.2.2 Faster switching speed by tinting both sides of the GPE window and durability test
PVA additive liquid windows, single-WE PVA GPE windows, and dual-WE PVA GPE windows (both sides tinted) were tinted to privacy state (0.1% transmittance at 550 nm) to compare their switching speeds. Figure 5A shows the transmittance of these three windows at 550 nm vs. time curves of a privacy cycle, where it is clear the dual-WE GPE window switch the fastest. On average it takes liquid and single- WE PVA GPE windows 205 and 355 s, respectively, to reach privacy states. The single-WE PVA GPE window is 73% slower, due to the lower ionic conductivity in the gel polymer system. However, for the dual-WE PVA GPE window it takes only 106 s on average to reach the privacy state, which is double the tinting speed of liquid windows. Detailed transmission and reflection spectra of the dual-WE PVA GPE window at initial, 10%, 1%, and 0.1% transmittance states at 550 nm are illustrated in Supplementary Figure S11.
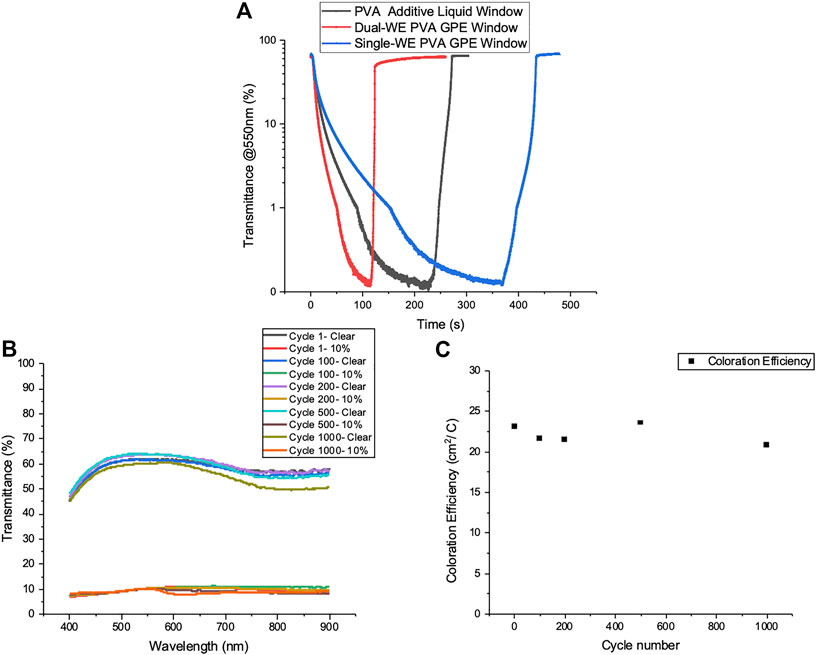
FIGURE 5. (A) Transmittance at 550 nm versus time curves for PVA additive liquid window, single-WE PVA GPE window, and dual-WE PVA GPE window with symmetric logarithmic scales. (B,C) Performance of a sealed dual-WE PVA GPE window over 1000 cycles. Transmission spectra of clear and 10% transmission states (B) and coloration efficiency (C).
To test the durability of PVA GPE windows, a dual-WE PVA GPE window was sealed with rubber and silicone and cycled to 10% transmittance at 550 nm. Transmission spectra and coloration efficiency are plotted over cycling, as shown in Figures 5B,C, exhibiting the consistent electrochemical behaviors of these windows over 1000 cycles.
4 Discussion
In this manuscript we have shown that a physically cross-linked poly (vinyl alcohol) polymer gel can be used to improve dynamic windows based on reversible metal electrodeposition. Using the gel polymer instead of an aqueous electrolyte causes a minimal increase in haze and only slows down the window by a factor of approximately 1.7 due to the reduced conductivity of the metal ions in the polymer gel matrix compared to its fully liquid counterpart. In addition, the gel circumvents failure due to hydrostatic pressure and leakage. We also showed that gels can be used as a separator to prevent short-circuiting for dual-working electrode windows with a copper mesh counter electrode in the middle. The dual working electrode windows switch approximately twice as fast. Furthermore, having two working electrodes enable a tinted window to achieve different optical properties: one with higher specular reflection or one with higher absorption and some diffuse reflection. Finally, we observed 1000 transmission cycles with a dual working electrode PVA gel polymer electrolyte window.
Data availability statement
The original contributions presented in the study are included in the article/Supplementary Material, further inquiries can be directed to the corresponding author.
Author contributions
YC: Writing–original draft, writing–review and editing, validation, conceptualization, investigation, methodology. TH: Writing–review and editing, validation, conceptualization, investigation, methodology. AY: investigation, validation, methodology, writing–review and editing. MS: Validation, methodology, writing–review and editing. FY: Investigation, writing–review and editing. EA: Investigation, writing–review and editing. MM: Writing–review and editing, conceptualization, funding acquisition, supervision, project administration.
Acknowledgments
The authors would like to thank Lori Postak from Quanex for providing the Solargain butyl rubber edge tape. The authors would like to thank Isaac Gould and Jay Patel for assisting with the diffuse reflection experiments that are shown in Figures 4D,E. This research was funded by the National Science Foundation (NSF) under award number 2127308. This research was supported in part by the Colorado Shared Instrumentation in Nanofabrication and Characterization (COSINC): the COSINC-CHR (Characterization) and/or CONSINC-FAB (Fabrication), College of Engineering and Applied Science, University of Colorado Boulder. The authors would like to acknowledge the support of the staff (Tomoko Borsa) and the facility that have made this work possible.
Conflict of interest
Authors TH, MS, and MM are cofounders of Tynt Technologies.
The remaining authors declare that the research was conducted in the absence of any commercial or financial relationships that could be construed as a potential conflict of interest.
Publisher’s note
All claims expressed in this article are solely those of the authors and do not necessarily represent those of their affiliated organizations, or those of the publisher, the editors and the reviewers. Any product that may be evaluated in this article, or claim that may be made by its manufacturer, is not guaranteed or endorsed by the publisher.
Supplementary material
The Supplementary Material for this article can be found online at: https://www.frontiersin.org/articles/10.3389/fnano.2022.1083247/full#supplementary-material
References
Alesanco, Y. A-O., Viñuales, A., Rodriguez, J., and Tena-Zaera, R. (2018). All-in-One gel-based electrochromic devices: Strengths and recent developments. Materials (Basel). 11 (3), 414. doi:10.3390/ma11030414
Alipoori, S., Mazinani, S., Aboutalebi, S. H., and Sharif, F. (2020). Review of PVA-based gel polymer electrolytes in flexible solid-state supercapacitors: Opportunities and challenges. J. Energy Storage 27, 101072. doi:10.1016/j.est.2019.101072
Barile, C. J., Slotcavage, D. J., Hou, J., Strand, M. T., Hernandez, T. S., and McGehee, M. D. (2017). Dynamic windows with neutral color, high contrast, and excellent durability using reversible metal electrodeposition. Joule 1 (1), 133–145. doi:10.1016/j.joule.2017.06.001
Eh, A. L-S., Chen, J., Yu, S. H., Thangavel, G., Zhou, X., Cai, G., et al. (2020). Reversible electrochemical mirror devices: A quasi-solid-state tristate reversible electrochemical mirror device with enhanced stability (adv. Sci. 13/2020). Adv. Sci. (Weinh). 7 (13), 2070073. doi:10.1002/advs.202070073
Eleanor, S. L., Mehry, Y., and Stephen, E. S. (2004). The energy-savings potential of electrochromic windows in the US commercial buildings sector. Berkeley, CA, United States: Lawrence Berkeley National Laboratory.
Guo, X., Chen, J., Eh, A. L-S., Poh, W. C., Jiang, F., Jiang, F., et al. (2022). Heat-insulating black electrochromic device enabled by reversible nickel–copper electrodeposition. ACS Appl. Mat. Interfaces 14 (17), 20237–20246. doi:10.1021/acsami.2c02626
Hassan, C. M., and Peppas, N. A. (2000). Structure and applications of poly(vinyl alcohol) hydrogels produced by conventional crosslinking or by freezing/thawing methods. Biopolymers · PVA hydrogels, anionic polymerisation nanocomposites. Berlin, Heidelberg: Springer Berlin Heidelberg, 37–65.
Hedge, A., and Nou, D. (2018). Effects of electrochromic glass on computer vision syndrome. Proc. Hum. Factors Ergonomics Soc. Annu. Meet. 62 (1), 378–382. doi:10.1177/1541931218621087
Hernandez, T., Alshurafa, M., Strand, M., Yeang, A., Danner, M., Barile, C., et al. (2020). Electrolyte for improved durability of dynamic windows based on reversible metal electrodeposition. Joule 4, 1501–1513. doi:10.1016/j.joule.2020.05.008
Hernandez, T. S., Barile, C. J., Strand, M. T., Dayrit, T. E., Slotcavage, D. J., and McGehee, M. D. (2018). Bistable black electrochromic windows based on the reversible metal electrodeposition of Bi and Cu. ACS Energy Lett. 3 (1), 104–111. doi:10.1021/acsenergylett.7b01072
Hou, Y., Chen, C., Liu, K., Tu, Y., Zhang, L., and Li, Y. (2015). Preparation of PVA hydrogel with high-transparence and investigations of its transparent mechanism. RSC Adv. 5 (31), 24023–24030. doi:10.1039/c5ra01280e
Hyon, S. H., Cha, W. I., and Ikada, Y. (1989). Preparation of transparent poly(vinyl alcohol) hydrogel. Polym. Bull. 22 (2), 119–122. doi:10.1007/bf00255200
Jeong, K. R., Lee, I., Park, J. Y., Choi, C. S., Cho, S-H., and Lee, J-L. (2017). Enhanced black state induced by spatial silver nanoparticles in an electrochromic device. NPG Asia Mat. 9 (3), e362–e. doi:10.1038/am.2017.25
Kanaya, T., Takahashi, N., Takeshita, H., Ohkura, M., Nishida, K., and Kaji, K. (2012). Structure and dynamics of poly(vinyl alcohol) gels in mixtures of dimethyl sulfoxide and water. Polym. J. 44 (1), 83–94. doi:10.1038/pj.2011.88
Kimura, S., Nakamura, K., and Kobayashi, N. (2020). Fabrication of complementary electrochromic device based on Ag deposition/prussian blue: Its optical modulating properties and memory functions. Meet. Abstr. MA2020-02 (32), 2070. doi:10.1149/ma2020-02322070mtgabs
Kimura, S., Wakatsuki, H., Nakamura, K., and Kobayashi, N. (2022). Compensative electrochromic device utilizing electro-deposited plasmonic silver nanoparticles and manganese oxide to achieve retention of chromatic color. Electrochemistry 90 (4), 22-00006–047002. doi:10.5796/electrochemistry.22-00006
Lozinsky, V. I. (1998). Cryotropic gelation of poly(vinyl alcohol) solutions. Russ. Chem. Rev. 67 (7), 573–586. doi:10.1070/rc1998v067n07abeh000399
Luo, W-B., Chou, S-L., Wang, J-Z., Kang, Y-M., Zhai, Y-C., and Liu, H-K. (2015). A hybrid gel–solid-state polymer electrolyte for long-life lithium oxygen batteries. Chem. Commun. 51 (39), 8269–8272. doi:10.1039/c5cc01857a
Poh, W. C., Gong, X., Yu, F., and Lee, P. S. (2021). Electropolymerized 1D growth coordination polymer for hybrid electrochromic aqueous zinc battery. Adv. Sci. 8 (21), 2101944. doi:10.1002/advs.202101944
Prnewswire (2018). Study: Natural light is the best medicine for the office. Available at: https://www.prnewswire.com/news-releases/study-natural-light-is-the-best-medicine-for-the-office-300590905.html.
Sbar, N. L., Podbelski, L., Yang, H. M., and Pease, B. (2012). Electrochromic dynamic windows for office buildings. Int. J. Sustain. Built Environ. 1 (1), 125–139. doi:10.1016/j.ijsbe.2012.09.001
Song, J., Park, J. Y., Kim, K., and Lee, J-L. (2022). High-vacuum annealed Al-doped ZnO nanorods for fast-switching electrochromic windows with high-optical contrast and long-term stability. ACS Appl. Nano Mat. 5 (3), 3946–3956. doi:10.1021/acsanm.1c04528
Strand, M. T., Barile, C. J., Hernandez, T. S., Dayrit, T. E., Bertoluzzi, L., Slotcavage, D. J., et al. (2018). Factors that determine the length scale for uniform tinting in dynamic windows based on reversible metal electrodeposition. ACS Energy Lett. 3 (11), 2823–2828. doi:10.1021/acsenergylett.8b01781
Strand, M. T., Hernandez, T. S., Danner, M. G., Yeang, A. L., Jarvey, N., Barile, C. J., et al. (2021). Polymer inhibitors enable >900 cm2 dynamic windows based on reversible metal electrodeposition with high solar modulation. Nat. Energy 6 (5), 546–554. doi:10.1038/s41560-021-00816-7
Keywords: dynamic window, reversible metal electrodeposition, gel polymer electrolyte, electrochromic, dual-working electrode, poly(vinyl alcohol)
Citation: Cai Y, Hernandez TS, Yeang AL, Strand MT, Yavitt FM, Abraham E and McGehee MD (2022) Gel polymer electrolyte for reversible metal electrodeposition dynamic windows enables dual-working electrodes for faster switching and reflectivity control. Front. Nanotechnol. 4:1083247. doi: 10.3389/fnano.2022.1083247
Received: 28 October 2022; Accepted: 14 November 2022;
Published: 28 November 2022.
Edited by:
Zhen Wang, Hainan University, ChinaReviewed by:
Wu Zhang, University of Alberta, CanadaXueqing Tang, Suzhou Institute of Nano-Tech and Nano-Bionics (CAS), China
Copyright © 2022 Cai, Hernandez, Yeang, Strand, Yavitt, Abraham and McGehee. This is an open-access article distributed under the terms of the Creative Commons Attribution License (CC BY). The use, distribution or reproduction in other forums is permitted, provided the original author(s) and the copyright owner(s) are credited and that the original publication in this journal is cited, in accordance with accepted academic practice. No use, distribution or reproduction is permitted which does not comply with these terms.
*Correspondence: Michael D. McGehee, bWljaGFlbC5tY2dlaGVlQGNvbG9yYWRvLmVkdQ==