- 1Semiochemical and lipid laboratory, Department of Life Science, Presidency University, Kolkata, West Bengal, India
- 2Entomology Laboratory, Department of Zoology, University of Calcutta, Kolkata, West Bengal, India
- 3Department of Pharmacology, School of Pharmacy, Anurag Group of Institutions (Formerly Lalitha College of Pharmacy), Hyderabad, Telangana, India
- 4Department of Biomedical Engineering, New Jersey Institute of Technology, Newark, NJ, United States
With the popularity of nanotechnology, the use of nanoparticles in pest management has become widespread. Nanoformulated pesticides have several advantages over conventional pesticide formulations, including improved environmental stability, controlled release of active ingredients, increased permeability, targeted delivery, etc. Despite these advantages, recent research shows that several nanoparticles used in conventional nanopesticide formulations can be toxic to crops and beneficial organisms due to bioaccumulation and trophic transfer. Therefore, traditional nanopesticides are thought to be non-advantageous for “green agriculture”. In assessing the current situation, developing “all-organic” nanopesticides could be the next-generation weapon for reducing the adverse impact of traditional nanopesticides. However, their formulation and application knowledge is remarkably limited. The green synthesis of “all-organic” nanoparticles makes them more environmentally friendly than conventional nanopesticides due to their minimal residual and hazardous effects. This review focuses on the current development scenario of “all-organic” nanopesticides, their advantages, and potential effects on target organisms compared to traditional nanopesticides.
Introduction
Agricultural insect pest management has been a challenging job for a long time. At the same time, as it is necessary to kill harmful insects, it is also essential to take care of the environment. Various synthetic chemicals have been used to eradicate the notorious pests at various times, but none have been effective. Over time, the misuse, uncontrolled use, and insensitive use of pesticides have always been controversial. Environmental health protection has always been neglected on the pretext of food security. The use of broad-spectrum conventional insecticides, like organochlorines, organophosphates, carbamates, and pyrethroids, has been widespread in agricultural croplands over the past five decades for their immediate effects (Sparks, 2013). However, the indiscriminate and excessive use of these chemicals resulted in a wide range of negative consequences, including eco-framework irregularity (Schäfer et al., 2019; Vašíčková et al., 2019), toxicity to non-target organisms (Singh and Leppanen, 2020; Teng et al., 2020), and the development of insecticide-resistant pests (Kariyanna et al., 2020; Sparks et al., 2020). In addition to synthetic-chemical pesticides, some organic insecticides have also been recommended to combat insect pests (Lengai et al., 2020; Stankovic et al., 2020). However, such organic’s efficacy for environmental sustainability remains questionable (Pavela, 2014). Under these circumstances, “precision agricultural crop protection” approaches are essential for crop management and environmental health protection (Stankovic et al., 2020).
In the last two decades, the expansion of nanotechnology has resulted in the development of nanopesticides as a new and promising armament to encounter pests in agriculture. These nanopesticides can diminish the undiscriminating use of chemical pesticides and are assumed to be an environmentally safer option (Djiwanti and Kaushik, 2019). In nanopesticides, the nanoparticles (NPs) can be used directly as an active ingredient (AI) (the principal component present in pesticides), or they can be used as a delivery agent for AI (as a carrier molecule). The carrier molecule facilitates the uniform spread of AI over the foliar surfaces of the targeted crop plants. As a result, they are quickly taken up by chewing insects (Rai and Ingle, 2012). Several unique properties of nanoparticles, such as small size (1–100 nm), high surface-to-volume ratio, a strong affinity for the target organism, permeability, crystallinity, and thermal stability, enable nanopesticides to be used for increased pesticidal effect, targeted delivery, environmental stability, and controlled release (Perlatti et al., 2013; Kah, 2015). In general, nanopesticides are divided into two categories. Firstly, the pesticides with nanoscale active components, which are often a nano dispersant emulsion of active pesticides. Secondly, a formulation where the regular pesticides are, encapsulated, doped, or coated with nanomaterials (Shekhar et al., 2021). Most of the typical commercial nanoinsecticides are composed of an appropriate combination of chemical and organic nanoparticles. Among these, organophosphorus (chlorpyrifos, malathion, parathion), carbamates (carbofuran), pyrethrin and pyrethroid derivatives (bifenthrin, deltamethrin, cypermethrin, gamma/delta/lambda-cyhalothrin, pyrethrin) are predominately used during commercial formulation. However, in recent times, the production of organic-pesticide derived nanoinsecticides instead of synthetic chemicals has gained momentum. Among these, the development of abamectin, avermectin, azadirachtin, and rotenone-based nanoinsecticide formulations is prominent (Hwang et al., 2011; Cui et al., 2015; Kilani-Morakchi et al., 2021).
Several reviews have summarised the classification and prospect of nanopesticides in agriculture (Kah et al., 2018; 2013; Kah and Hofmann, 2014). Even if there are some promising applications of nanopesticides in agriculture, the possible adverse effects of nanomaterials on the environment, living organisms, and humans are unknown comprehensively. Several studies reveal that nanomaterials can generate toxicological effects on lettuce, tomatoes, wheat, and cucumbers when used in high concentrations (Wang C et al., 2019; Paramo et al., 2020; Pelegrino et al., 2020). Therefore, environmental and non-target toxicity due to existing nanopesticides is also under screening (Grillo et al., 2021). Furthermore, concerns are being raised about using existing nanoparticles for their safety, reliability, and health insecurity (Sarkar et al., 2012; Kah et al., 2021). Deka et al. (2021) mentioned that these nanoparticles can enter human or animal body via dermal contact or inhalation and the chronic exposure can cause sub-acute toxicity and sometimes can lead to severe health risk (Deka et al., 2021).
After considering the facts and realities, the concept of “all-green” nanopesticides has been proposed to overcome the shortcomings that could arise from the use of conventional chemical based nanopesticides. The concept of “all-green” nanopesticides is a view where both components of nanopesticides come from biological sources (Ball, 2018; Liang et al., 2018; Wang et al., 2018). In last two decades multiple independent research area has been developed and strengthened such as nanotechnology, green synthesis of biogenic nanoparticles, natural products research, material biology, biopolymer synthesis and characterization, which can converge together to develop a new class of nanopesticide where both the A and the carrier molecule are biological in origin, which we mentioned in this literature as “all-green” nanopesticide. As the concept and idea of “all-green” nanopesticides are novel, much information is not available on their sustainable formulation and future development. However, few literatures have been published in last decade and we have attempted to explore the available information of recent advancements in “all-green” nanopesticides and summarize the idea in this comprehensive review. More specifically, in this present review we have concentrated our focus on nanoinsecticides, present advancement of nanoinsecticides in insect pest management and the future prospects of nanoinsecticide as “all-green” nanoinsecticide.
Nanoinsecticides: An overview
An ideal insecticide should fulfil specific toxicity criteria, including the ability to remain active without physical degradation in the face of environmental calamities. It should be taken up by the target organism effectively; it should have the ability to invade the pest’s defensive barriers and remain benign to plants, humans, and other mammals. An ideal insecticide should provide economic security to agrarians through its unparalleled mode of action. Nanoinsecticides almost cover all of the criteria fulfilled, and henceforth, they are regarded as the epitome of emerging scientific development. Furthermore, in addition to insecticidal applications, nanoinsecticides may provide a variety of additional benefits such as increased target efficacy (Kah et al., 2018; Kaziem et al., 2018; Ahmed, 2019; Gao et al., 2019; Sun et al., 2020), durability and environmental half-life (Liu et al., 2008; Shakil et al., 2010; Kaziem et al., 2018; Gao et al., 2019), and a required minimum amount of active ingredient (AI) (Vašíčková et al., 2019; Wang Y et al., 2019). For these reasons, conventional chemical insecticides are being reformulated as nanoinsecticides to become more efficient and effective (Kah et al., 2018; Pires-Oliveira et al., 2020). Physically, nanoinsecticides are small-sized particles at the nanoscale of AIs or other engineered nanoparticles with potential insecticidal properties (Bergeson, 2010). Several commercial nanoinsecticides have been developed to date, including Banner MAXX, Subdue MAXX, Bifender FC, AZeroid, and Fenstro (Kah et al., 2013; Walker et al., 2018), but none can be considered truly environmentally friendly. These nanoinsecticides are formulated as nanocarriers combined with registered AIs having insecticidal properties (Walker et al., 2018). Nevertheless, the most frequently used AIs are chemical or inorganic compounds. However, there is some evidence that plant-derived botanicals are also used as AIs.
Compared to regularly applied pesticides, nanopesticides display valuable characteristics such as stiffness, permeability, crystallinity, thermal stability, and biodegradability (Lade, 2017). In particular, these nanoformulations allow a slower release of AI into the environment, resulting in the retention of pest control efficacy over a more extended period than conventional insecticides (Liu et al., 2008; Ishaque et al., 2013). In addition, nano-formulated insecticides provide enhanced apparent solubility and enhanced uptake efficacy of AI, which ultimately leads to a lower requirement of insecticides for pest control (Anjali et al., 2010; Kookana et al., 2014; Cui et al., 2020). Reports also suggest that nanoinsecticides are less toxic than conventional chemical pesticides (Wan-Jun et al., 2010).
Advantages of nanoinsecticides
Using various techniques, reforming conventional insecticides into different nanoforms (Figure 1) brings an array of favourable advantages for agricultural pest management programs (Kookana et al., 2014; Camara et al., 2019; Kumar et al., 2019). In general, these nanoinsecticides are formulated either through manipulations of nanocapsules, nanospheres, nanomicelles, nanoemulsion, nanosuspension, liposomes, or solid or lipid nanoparticles (Nuruzzaman et al., 2016). Nanoencapsulation is possibly the most popular nanoinsecticide formulation technique. In this technique, active ingredients or the insecticides are enclosed within a polymer or matrix of nanoscale range. The encapsulation protects the AI from environmental degradation, rapid environmental loss and allows accurate targeting. Nanoemulsion is the kinetically stable colloidal dispersion of nano sized AIs (1–100 nm), which have enhanced functional property and enhanced bioavailability in compared to conventional AIs. Nanosuspensions can be defined as a colloidal, biphasic dispersions of AIs of submicron size that are stabilized by surfactants. Nanosphere is a nanoscale homogenous sphere that either carry AIs on their surface or entrap the AI within the polymeric matrix. Solid lipid nanoparticles are nothing but nanosized sold lipid suspensions which are attracting wide attentions from researchers nowadays as an alternative carrier for lipophilic AIs.
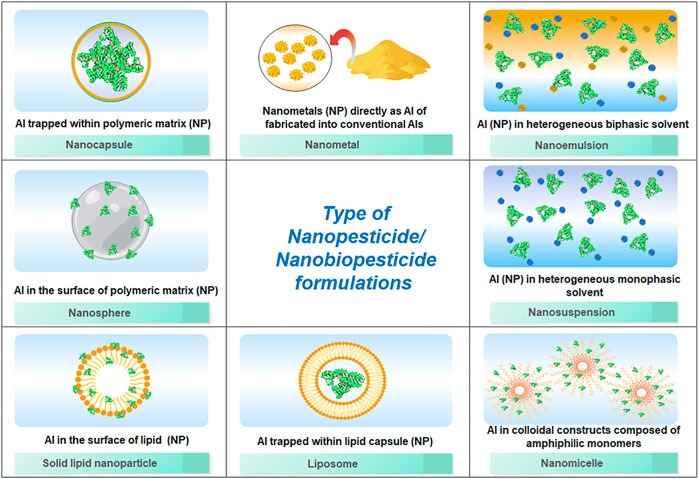
FIGURE 1. Illustration on different ways of nanopesticide formulations (active ingredients with carrier molecule and/or matrix).
Each of these types of nanoformulation has its unique advantages regarding pest control efficacy and sustained release. It is claimed that nanocapsules deliver the best pest control efficiency due to their nanoguard property in their external shells. Nanoparticle loading of active substances permits the controlled release of AIs into the environment, leading to extended pest control efficacy compared to commercially available formulations (Liu et al., 2008; Ishaque et al., 2013). Nanoemulsions are another good form of nanoinsecticide formulation that enables enhanced apparent solubility, bioavailability, and AI uptake efficacy of AIs (Anjali et al., 2010; Kookana et al., 2014). In addition, nanoemulsions are considered a prospecting insecticide delivery system with better kinetic stability, smaller size, low viscosity, and optical transparency (Mustafa and Hussein, 2020; Sabry et al., 2021). Nanodispersion and nanosuspension are kinds of nanoformulations that may enhance the toxicity of AIs to the target organism, even at a suboptimal lower dose (Frederiksen et al., 2003; Chen et al., 2018; Wang C et al., 2019; Wang Y et al., 2019). For example, aqueous nanodispersions of triclosan (an antibacterial and antifungal agent) display greater efficacy than organic or aqueous solutions of triclosan (Zhang et al., 2008). In addition, nanometals or metal-oxide nanoparticles are also reported to be used in pest management directly as AIs or as delivery vehicles or adjuvants indirectly. Thus, the active constituents of nanoinsecticide provide advantages for pest management in different ways (Figure 2), and these are as follows:
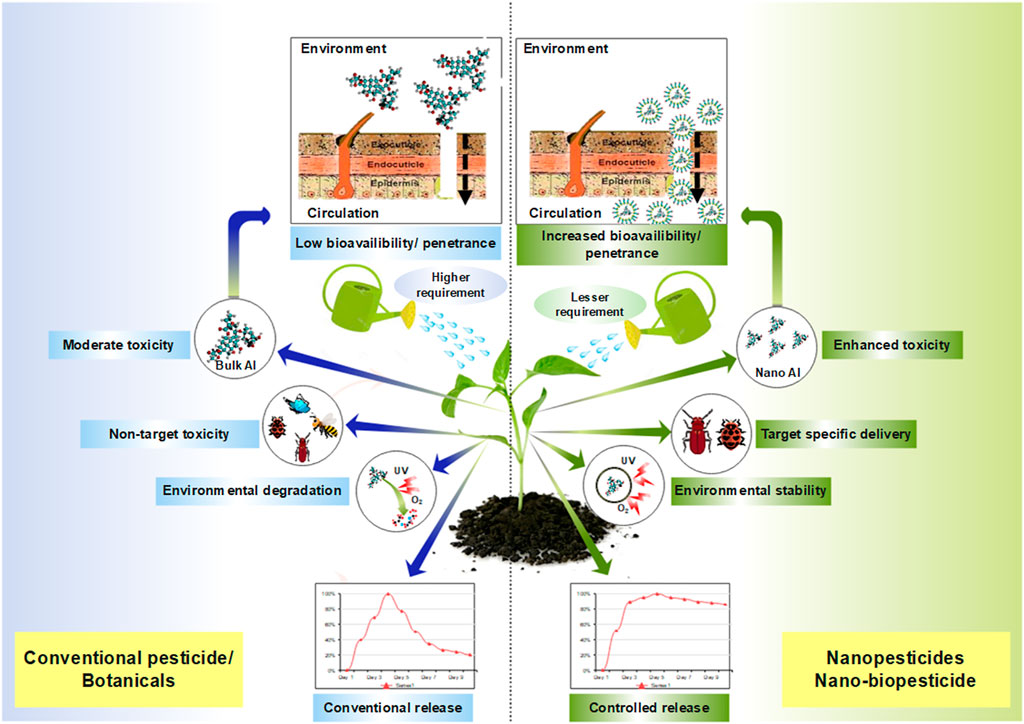
FIGURE 2. An outline depicting superiority of nanopesticides over traditionally used chemical pesticides.
Active ingredient
Some investigations reveal that metal nanoparticles can directly act as AIs for nanoinsecticides. For example, ZnO is used as a fungicide against multiple pathogenic fungi (Alternaria mali, Botryosphaeria dothidea, Diplodia seriata) in fruit orchards to defend against fruit blotches, plant cankers, and bot cankers (I. Ahmad I et al., 2020; Jameel et al., 2020). ZnO nanoparticles enhance thiamethoxam’s (a systemic insecticide) insecticidal activity against the tobacco cutworm, Spodoptera litura larvae (Jameel et al., 2020). Likewise, SiO2 also has a broad spectrum of insecticidal properties against a bunch of notorious insect pests like cotton leafworm (Spodoptera littoralis), rice weevil (Sitophilus oryzae), wheat weevil (Rhizopertha dominica), red flour beetle (Tribolium castaneum), and grain beetle (Orizaephilus surinamenisis) (Debnath et al., 2011; Ayoub et al., 2017; El-Naggar et al., 2020). Similarly, the effect of nanostructured alumina on the leaf-cutting ant Acromyrmex lobicornis, which is a major pest of agricultural and forest plants, has been studied. According to the report, adult mortality increases with increasing exposure time and dosage. Furthermore, it was discovered that nano-formulated alumina has increased cuticular attachment, which increases the probability of cytotoxicity (Buteler et al., 2018). Some other metallic nanoparticles such as MnO, TiO2, Ag, and Fe3O4 have also shown a variety of antifungal activities on a diverse group of fungal pathogens from crops (Chen J et al., 2020; Panova et al., 2019; Paramo et al., 2020; Wang et al., 2017). Besides metallic AIs, some non-metallic AIs like graphene oxide (C140H42O20) (Wang et al., 2017; Wang C et al., 2019) and silicon (Rastogi et al., 2019) are also reported to have an insecticidal response to several pathogens.
Enhanced toxicity
Nanoformulations of conventional insecticides can potentially improve the toxicity levels of their target insect pests by up to 10-fold (Kah et al., 2018). Triclosan nanopesticides, formulated as aqueous nanodispersions, show more significant activity than organic or aqueous solutions of Triclosan (Zhang et al., 2008). The larvicidal effects of nano-permethrins (C21H20Cl2O3) (an atopic antidermatitis for mosquitoes, scabies, and lice) on Culex quinquefasciatus were recorded almost six times higher than bulk permethrin (Anjali et al., 2010). Similarly, nano-formulated chlorantraniliprole and thiocyclam were 3.86-fold and 2.06-fold more effective on black cutworms (Agrotis ipsilon) than their conventional forms. These insecticide’s nanoformulations can successfully reduce egg-hatching rates and alter larval growth periods (Awad et al., 2022). Similarly, the insecticidal activity of pyridalyl (C18H14Cl4F3NO3) nanosuspension (a selectively cytotoxic compound for Lepidoptera and thrips) is more effective (LC50: 40 μg/L) than its bulk use for the treatment (LC50: 90 μg/L) of cotton bollworm, Helicoverpa armigera (Saini et al., 2014). Sabry et al. (2021) demonstrated that nanoparticles from oxadiazine larvicide, indoxacarb (C22H17CIF3N3O7) (a neurotoxic insecticide for lepidopteran larvae), and the neonicotinoid insecticide, imidacloprid (C9H10ClN5O2) (a neurotoxic substance that arrests insect CNS) are respectively 12 to 4 times more effective than their conventional formulations against the cotton leafworm, S. littoralis. Rahwanudin et al., 2022 found that Spinetoram nano-suspension (a neurotoxic constituent from Saccharopolyspora spinosa) had a greater efficiency (33%) in controlling diamondback moth, Plutella xylostella than the commercial form. Metallic oxides, such as CuO and ZnO, when used as nanocarriers, can facilitate the uptake of bifenthrin (C23H22ClF3O2) (a pyrethroid neurotoxic) in the earthworm, Eisenia fetida. ZnO nanoparticles could augment the insecticidal action of thiamethoxam (C8H10ClN5O3S) against S. litura larvae (Jameel et al., 2020; Sabry et al., 2021).
Enhanced solubility and uptake efficiency
Enhanced apparent solubility is the phenomenon where the solubility of conventional pesticides is drastically increased, which are in general less soluble in water or organic solvents. In addition to increased solubility, nano-formulated pesticides allow increased transfer of AI from the treated surface to the target pest. The toxicity of insecticides can be enhanced on target organisms even at suboptimal lower doses by reformulating the bulk molecule into nanoinsecticides (Kookana et al., 2014). For example, aqueous nano-dispersions of antibacterial/fungal Triclosan (C12H7Cl3O2) showed more significant activity than organic or aqueous solutions of an equivalent amount of Triclosan (Zhang et al., 2013). Similarly, nanoencapsulation of nicotine-mimicking commercial systemic insecticide imidacloprid (C9H10ClN5O2) is equally effective as bulk imidacloprid, even at a lower dose (Memarizadeh et al., 2014). Therefore, it is assumed that the exceptional properties of nanoinsecticides permit a uniform spread of AI over the foliar and soil surfaces, and thus, they are easily taken up by chewing insects. Additionally, nanocarrier-based AIs are absorbed by the cuticular wax (lipid) layers of insects and break down the water protection barrier (Nuruzzaman et al., 2016; Rastogi et al., 2019). Therefore, in short, the large surface area of nano insecticides favours increased affinity for the target pest and, therefore, reduces the amount of insecticide required for controlling the enemy (Boehm et al., 2003).
Targeted delivery and controlled release
In the 21st century, several nanoinsecticide formulations have been conceptualized and designed to efficiently deliver optimum amounts of AIs. Porous materials have shown great potential for targeted delivery and controlled release of AIs in practical applications. Materials like mesoporous silica nanoparticles have become a great choice of interest as they showed multiple benefits such as improved efficacy, efficient delivery and reduced requirement of AI than conventional dose (Sharma et al., 2021a). For example, abamectin loaded in mesoporous silica nanoparticles showed release rate of 30 μg/h for 25 h which eventually dropped to 10 μg/h for next 200 h (Wang et al., 2014). In last decade, with advancement of nanotechnology, a smart insecticide delivery system has been developed to minimize the usages of AIs, which is known as “stimuli-responsive-nanoinsecticides”. In such nanoinsecticide formulation, the AI releases from the formulation only after the onset of pest infestation. Alternation of pH, temperature, redox system, light irradiation and even some specific enzymes could serve as stimulus (Kumar et al., 2015; Kaziem et al., 2018; Wang et al., 2018; Xiang et al., 2018; Gao et al., 2019; Zhang et al., 2019).
A number of pH responsive nanoinsecticide formulations have been developed experimentally to control insect pests that are optimized to release AI in presence of a wide range of pH conditions (acidic or alkaline) present in the insect intestine (Kaziem et al., 2018; Wang et al., 2018). One such example is the nanoformulation of cypermethrin with alginate nanocarrier. The pH of the nanoformulation system is maintained towards acidic side where alginate forms crosslinking polymer mesh and make the interior of the nanoparticle hydrophobic, resulting into reduced release of AIs from the system. After triggering with alkaline pH, the crosslinking polymer starts to disintegrate owing to the loss of electrostatic interactions and resulting into release of insecticide (Patel et al., 2018). The pH of the system not always interferes with the electrostatic interaction or polymer crosslinking. Wang et al. (2018) reported that enhanced release of avermectin from poly-(succinate) nanocarrier system at higher pH was due to collapse of nanoparticles in presence of alkaline condition. Some other nanoinsecticide formulations also exhibit the same kind of pH-dependent active ingredient’s release prototype. Abamectin-silica, coated with polystyrene and trimethoxysilyl-propyl methacrylate nanoinsecticide formulation released around 15% of insecticide at pH 5 after 15 days of application but at pH 10 the loss of abamectin reached up to 87% (Gao et al., 2019). Few other nanoformulations, for example, cyclodextrin-SiO2 NP containing avermectin (Kaziem et al., 2018), alginate-chitosan nanocarrier system containing acetamiprid (Kumar et al., 2015) have been developed which are activated in presence of alkaline pH. Photo-responsive nanoinsecticide formulations are another example of nanotechnological advancement. Formulation of fipronil—a broad spectrum phenylpyrazole insecticide, and coumarin—a phytochemical belonging to flavonoid group, is one of the well-recognized evidence of photo-responsive insecticide (Gao et al., 2019). It was found that in dark, the insecticide exhibits low insecticidal activity in Aedes mosquitoes, but in presence of sunlight their insecticidal activity significantly amplified. A similar observation was later found by Xu et al. (2018) in case of spirotetramat enol-coumarin insecticide formulation.
As an example of further technological advancement Sharma et al. (2017) have developed graphene oxide (modified with copper and selenium NP) nanocomposite to deliver chlorpyrifos to cabbage white butterfly, Pieris rapae. The nanoformulation showed both pH sensitive and photo-sensitive release of AI. The composite showed 25%–30% release of AI in presence of extreme PH, which is typical of insect digestive tract, in compare to ‘release’ (17%) at neutral pH. Furthermore, the release rate of AI from the formulation was calculated to be four times higher in presence of light irradiation in compared to control. These specific release pattern of AI could be appropriate specifically for the diurnal insects and could reduce the usage of AIs.
Enzyme responsive nanoinsecticide formulations have also been thoroughly investigated. Enzymes found in herbivorous insect’s salivary glands or in mid-gut, such as alkaline phosphatases, alpha-amylase, carboxylases, and others, promote specific reactions during feeding and therefter trigger the process of AI-release from nano-based insecticides (Kaziem et al., 2018; Camara et al., 2019). Guo et al. (2015) have developed epichlorohydrin-modified carboxymethylcellulose microcapsule containing emmamectin benzoate insecticide, crosslinked with silica nanoparticles. In absence of insect feeding the carboxy-methylcellulose capsule remains intact, hence restrict the release of emmamectin benzoate (20% loss in 30 h). However, in presence of cellulase in insect saliva, the cellulose wall of the capsule cleaved into smaller fragments causing the release of AIs from the formulation (80% loss in 30 h) (Guo et al., 2015). Similarly, α-amylase-responsive α-cyclodextrin anchored insecticide formulation has been developed containing silica loaded avermectin. In absence and presence of larval feeding, around 95% and 60% of AIs retained within the formulation after 17 days of application, indicating the stimulus responsive insecticide release pattern of the nanoformulation (Kaziem et al., 2018).
Furthermore, change in ambient temperature acts as a stimulus for temperature responsive nanoinsecticides. In most cases high temperature causes enhanced release rate of AIs from the formulations, which is due to the enhanced thermodynamic movement of the active molecules that facilitate the diffusion of the insecticides through the carrier material (Liang et al., 2018). SiO2 nanoparticle-coated temperature-responsive chitosan containing avermectin (Liang et al., 2018), and mixed micelle nanomycetes loaded with pyrethrin (Zhang et al., 2019) are some examples of smart thermal responsive nanoformulation that have been developed for experimental purposes.
Environmental stability
Most of the AI commonly used in insect pest management is vulnerable to environmental degradation due to oxidation, UV exposure, leaching, etc. At the same time, it is also suggested that AIs can be sustained in the environment for a longer duration in presence of nanocarriers without losing their insecticidal ability (Kumar et al., 2019). Researchers showed that the neurotoxic nano-bifenthrin slowly degrades the environment following a first-order model (Kah et al., 2016). Similarly, compared to bulk counterparts, the organophosphate pesticide, chlorpyrifos (C9H11Cl3NO3PS) with lipid nanocarrier and the fungicide, tebuconazole (C16H22ClN3O) with polymeric nanocarrier had longer soil half-lives (Fojtová et al., 2019). Clay and LDHs (layered double hydroxides) containing nanoformulations prevent volatilization and photodegradation of pesticides (Chaud et al., 2021). Using the co-solvent approach, nanoliposomes were synthesized by encapsulating emamectin benzoate with 1,2-distearoyl-sn-glycero-3-phosphoethanolamine-N-[amino(polyethylene glycol)-2000]. The resulting nanoformulation not only has considerable larvicidal activity against the fall armyworm, Spodoptera frugiperda (LC50: 0.046 mg/L) but also has improved leaf adherence and outstanding sustained release properties (Chen et al., 2022). Sharma et al. (2021b) have developed nanoformulations using copper and selenium modified graphene oxide nanocomposite containing captan. The 2D morphology of graphene oxide enhanced the charge-assisted-binding of nanoformulation with the foliar surface and it was found that leaching of AI is significantly less (26%–35%) from the nanoformulation in compared to bulk captan emulsion (70%). Patil and Bendre (2022) used in situ polymerization to synthesize a phenol-urea-formaldehyde (PUF) terpolymer, which was then used to encapsulate a ‘neem’ oil-based bioinsecticide. Because of their stability, the resulting microcapsules (30 um) worked remarkably well even at higher ambient temperatures (up to 45°C). The microcapsules demonstrated first-order release kinetics, indicating a slow environmental release feature (Patil and Bendre, 2022).
Reduced toxicity
One such experiment is being carried out in a human model. Powered nanostructured alumina, which is being studied as an alternative to chemical insecticides, is less harmful in humans than commercially available chemical pesticides. Experimental evidence suggests that nanostructured alumina causes considerably less DNA damage, chromosomal breakage, and cell viability in human peripheral blood lymphocytes than routinely used organophosphates (Vineela et al., 2017).
Disadvantages of nanoinsecticides
As stated before, the application of nano-formulated insecticides increases the effectiveness of typical insecticides, though commercially available nanoparticles are not always safe (Kah, 2015). Hence, there is a need to assess the possible undesired outcomes of applying nanoparticles in agriculture. While, on the one hand, these nanomaterials can provide nutrients to plants and protection against pests, on the other hand, they can induce stress on other non-pest species of the ecosystem, causing ecological risks (Bourguet and Guillemaud, 2016). Thus, during the last few years, a new genre of toxicology has been developed called “nanotoxicology,” where the toxic effects of nanomaterials are under the scanner. Nanomaterials seem to exhibit toxic effects that are uncommon and not seen with larger particles, and these smaller particles can pose more of a threat to living organisms due to their ability to move with a much higher level of freedom (Sukhanova et al., 2018). There remains much evidence of the harmful impacts of nanoparticles becoming an obstacle to existing nanoinsecticides in recent times (Côa et al., 2020; Paramo et al., 2020). Therefore, along with their good merits, the nanoinsecticides also have some shortcomings in functionality, as follows:
Cellular stress
Oxidative stress is a phenomenon caused by an unevenness between the production and accumulation of reactive oxygen species (ROS) in cells and tissues and the capacity of a biological system to detoxify ROS (Betteridge, 2000). Upon treatment, the interactions between cells and nanomaterials are evident due to fabricated and engineered nanomaterials designed with unique features to attain specific targets (Kovacic and Somanathan, 2013). However, the scientific basis for the cytotoxicity and genotoxicity of most manufactured nanomaterials is not well understood. Excessive synthesis of ROS can induce oxidative stress, resulting in cell’s failing to maintain normal physiological redox-regulated functions, which results in DNA damage, unregulated cell signalling, changes in cell motility, and cytotoxicity (Rajeshwari et al., 2016; Samhadaneh et al., 2019). Copper [Cu(OH)2] nanopesticides, for example, have been shown to harm spinach by lowering antioxidant molecules such as ascorbic acid, alpha-tocopherol, threonic acid, 4-hydroxybutyric acid, ferulic acid, and total phenolic compounds by 29%–85% (Zhao et al., 2017). Another study found that Cu(OH)2 based nanopesticides cause significant oxidative stress in lettuce by lowering antioxidant levels (cis-caffeic acid, chlorogenic acid, 3,4-dihydroxycinnamic acid, and dehydroascorbic acid) in comparison to the control (Zhao et al., 2016). In the onion, Allium cepa, gold nanoparticle-dependent generation of reactive oxygen species (ROS) was observed along with enhanced lipid-peroxidation and chromosome aberrations in root hair cells (Rajeshwari et al., 2016).
Reduction of plant growth and seed germination
Nanoinsecticides are known to hinder plant growth and thereby reduce agricultural yield. Literature suggests that nanoparticles present in nanoformulation interfere with plant growth by disturbing water homeostasis and disrupting concentrations of other small molecules in plants (Qian et al., 2013). Moreover, nanoinsecticides can manipulate plasma membrane K+ efflux and Ca2+ influx, which eventually cause membrane breakdown (Sosan et al., 2016). For example, ZnO and CuO nanoparticles affect crop yield by interfering with root and shoot growth (Wang C et al., 2019; Paramo et al., 2020; Pelegrino et al., 2020). Nanoparticles may further interfere with plant growth by disrupting the thylakoid membrane, which eventually decreases chlorophyll content and the photosynthetic rate of the plants (Qian et al., 2013; Fayez et al., 2017). Other metallic nanoparticles like TiO2 and Ag are known to reduce host plant’s early growth and chlorophyll content (Qian et al., 2013; Gao et al., 2019; Wang Y et al., 2019; Paramo et al., 2020). Lee et al., 2008 demonstrated that Cu-nanoparticles have the potential to reduce the growth rate of mung bean (Phaseolus radiates) and wheat (Triticum aestivum). Seed germination is also affected by exposure to nanoparticles (Lee et al., 2008). For example, Ag-based nanoparticles can reduce carrot seed germination by 20% (Park and Ahn, 2016). Inhibition in lettuce seed germination and radicle growth is reported on exposure of CuO-nanoparticles due to ROS or RNS (reactive oxygen or nitrogen species) accumulations in the seed (Pelegrino et al., 2020).
Ecological impact
Apart from toxic costs, there is evidence that nanopesticides can be accumulated and transferred through the food chain, causing long-term effects on the ecosystem (Dang et al., 2019; Xiao et al., 2019; Côa et al., 2020). However, only a few reports have focused on the toxicity and bioaccumulation of nanomaterials in agricultural lands. The majority of research has been conducted on planktons (algae and daphnids), where metal oxides are primarily tested as nanoparticles (Tangaa et al., 2016). Trophic transfer of bio-accumulated silver nanoparticles is demonstrated in different algae-daphnids (McTeer et al., 2014; Chen et al., 2015), algae-fish (Skjolding et al., 2014), algae-bivalve (Renault et al., 2008), algae-amphipod (Jackson et al., 2012), and algae-daphnids-fish (Chae and An, 2016). Kalman et al (2015) show the bioaccumulation and trophic transfer of silver nanoparticles in the green alga, Chlorella vulgaris, and in the crustacean, Daphnia magna, resulting from the Ag-nanoparticle assimilations (Kalman et al., 2015).
Nanoparticles can suffer environmental physical and chemical modifications such as changes in aggregation and oxidation state, colloidal behaviour, dissolution, sulfidation, and sorption of inorganic and organic species that result in a transient pattern of dissolution or stability of nanoparticles (Santaella and Plancot, 2020). All of these can alter the toxicological profiles of nanopesticides (Côa et al., 2020). In addition, the physiochemical transformation of nanoparticles favours the sedimentation rate, which eventually extends their persistence in the environment and thus prolongs the toxicity period (Deng et al., 2017; Côa et al., 2020). Deng et al. (2017) suggest that nanoparticles can change the bioavailability of existing environmental contaminants and heavy metal ions, enhancing their accumulation and, thereby, distribution within biota. Bio-accumulation of contaminants depends primarily on the organism’s ability to accrue the sorptive nano-contaminant complexes. The readily accumulated nanoparticles can act as carriers for the transport and bioaccumulation of co-contaminants (Deng et al., 2017).
Global scenario of bio-nanoinsecticides
Scientific laboratories and leading agrochemical manufacturing companies (Novartis AG, Bayers, Monsanto, Indigo, DOW agro-sciences, BASF, Symrise, and Syngenta) have repeatedly attempted to develop nanoinsecticides, and many of these formulations have been patented. More than 3,600 nano-encapsulated insecticides have been patented during the last 20 years (Pires-Oliveira et al., 2020). However, most of these nanoinsecticides have been developed through nanoparticles from commercially available chemical insecticides like carbamate, organophosphate, chlorinated cyclodiene, etc. (Table 1). Therefore, considering the adverse effects of chemicals, the utilization of biological molecules is now being considered to formulate nanoinsecticides termed “bio-nanoinsecticides” (Jampílek and Kráľová, 2019; Medina-Pérez et al., 2019). In formulating such bio-nanoinsecticides, biological molecules are often obtained from microbial, botanical, or animal origins and are usually used as carriers or/and AI (Choi et al., 2011; Riyajan, 2011; Feng and Peng, 2012; Chen et al., 2013; Jia et al., 2014; Pacheco-Aguirre et al., 2016; Ball, 2018; Liang et al., 2018). Upon considering several advantages of bio-nanoinsecticides, they are now becoming popular worldwide to boost organic farming. The growing demand for organic nanoencapsulated bioinsecticides increases from agricultural croplands to fruit orchards and vegetable grounds to tea plantations (Rastogi et al., 2019). In the formulation and development of bio-nanoinsecticides, the organics that are being utilized are as follows:

TABLE 1. List of experimentally successful nanoinsecticide formulations (active ingredients and carriers) and their functional superiority to conventional chemical insecticides (Abbreviations; HNM, Hybrid nano metal; NE, Nano encapsulation; NG, Nano gel; NM, Nano micelle; NN, Nano emulsion; NS, Nano suspension; SLN, Solid lipid nanoparticle; M-NP, Metal nanoparticle).
Botanicals as active ingredients
Azadirachtin (C35H44O16), the neem (source: Azadirachta indica, Meliaceae) alkaloid, is the biological AI typically used to develop nanoinsecticides. The biological AI can be loaded with nanoparticles from organic and inorganic sources (Feng and Peng, 2012). Rotenone (C23H22O6) is another organic AI (source: Derris elliptica, Fabaceae) widely used in the formulation of nanoinsecticides and has a strong paralysis effect (knockdown) on poikilotherms (Othman et al., 2016). Likewise, allicin (C6H10OS2)—an organosulfur compound obtained from garlic (Allium sativum, Alliaceae) and garlicin—the product of garlic (Yang et al., 2009; Ali et al., 2014), and aloin (C21H22O9)—the dried latex from the leaves of several Aloe sp (Asphodelaceae) have also been explored for the development of various nanoinsecticides (Lade, 2017). In addition to these, capsaicin (C18H27NO3) from capsicum (Capsicum sp; Solanaceae), abamectin [C48H72O14 (B1a); C47H70O14 (B1b)] from the soil-dwelling actinomycete, Streptomyces avermitilis, act as highly efficient AI against insect pests (Table 1).
Microbes as the active ingredient
The concept of nanoencapsulation of living organisms like bacteria and fungi is a recent trend in research. In this process, such biological microorganisms that are commonly used for the biological control of pests are encapsulated in a specialized matrix to provide a suitable microenvironment (Chen et al., 2013; Pacheco-Aguirre et al., 2016; Locatelli et al., 2018; Pires-Oliveira et al., 2020). This concept of nanoinsecticide formulation is novel for agricultural pest management and could be very promising for the upcoming years (Pires-Oliveira et al., 2020). Additionally, co-encapsulating microorganisms with botanicals or chemical AIs can increase the effectiveness of the formulation constituents even at a reduced dose (Shang et al., 2019). Besides using whole organisms, some nanoinsecticides have been developed by milling microbial toxins into the nanoscale. The best example of this method is the Bt-based nanoinsecticides. Nanoscale derivatives of Bt (2–5 um), made by top-down processes, are now being investigated for insect pest management efficiency (Murthy et al., 2014; Vineela et al., 2017).
“Biologicals” as carrier or matrix
Besides the role of biological molecules (“biologicals”) as AIs in nanoinsecticide formulations, the use of “biologicals” as a carrier of AI for nanoinsecticides has also been observed. It has been demonstrated that tannic acid (as a carrier)-based nanoinsecticides have a far better foliar adhesive property and, thus, can be retained on the leaf surface for a more extended period (Yu et al., 2019). Chitosan, the linear N-acetyl derivative of chitin (C8H13O5N)n obtained from the arthropod exoskeleton, serves best in this category. Chitosan nanoparticles have been tested as an efficient carrier for several commercial insecticides (Maruyama et al., 2016; Sharma et al., 2019) and have been found to have no adverse effects on living organisms. As a result, it has been approved as a non-toxic biogenic nanomaterial (Wang et al., 2011). Besides chitosan, alginates (C6H8O6)n—a multifunctional anionic biopolymer that occurs naturally in the brown algae cell wall (Phaeophyceae), and its derivatives, have gradually drawn attention as attractive carrier compounds for AIs (Szekalska et al., 2016). Polydopamine (PDA) is another bio-adhesive nanoparticle derived from mussels (Lynge et al., 2011) that exhibits exceptional adhesive performance on crop foliage and thus enhances the retention time of insecticides (Jia et al., 2014; Ball, 2018; Liang et al., 2018). PDA is the final oxidation product of dopamine or other catecholamines that coat the “biological element” at an adjustable thickness ranging from a few to about 100 nm. These PDA layers can be modified with molecules carrying nucleophilic groups or metallic nanoparticles from solutions containing metallic cations. However, during deposition of PDA on the surface, reaction products obtained from the oxidation of catecholamines precipitate on the foliage (Ball, 2018).
Scope and limitation
Though nanoinsecticides are the promising future of modern agronomy, some scientists have already raised questions regarding the biosafety of nanoinsecticides. Therefore, the short-to long-term toxicological effects on the environment have become alarming (Chaud et al., 2021). Moreover, conventional chemical or metallic-nanoparticle production techniques mentioned in Figure 3A involve volatile organic solvents and hazardous chemicals, which sometimes become noxious for human and environmental health (Küünal et al., 2018). In addition, these techniques sometimes are very complex and require more financial aid. Therefore, to overcome the adverse health effects and complexity associated with conventional nanoparticle synthesis process, the development and formulation of biogenic nanomaterials have been conceptualized (Figure 3A) (Ahmad I et al., 2020; Jadoun et al., 2021). Though the exploration of biogenic nanoparticles is quite old (Lippert and Zachos, 2007), the development of microscopic and sub-microscopic nanomaterials using living organisms has gained pace in recent times towards its commercialization for agricultural pest management (Gour and Jain, 2019). It is substantially acknowledged that applying biologically derived nanomaterials, otherwise called “green pesticides,” would be environmentally friendly and, therefore, sustainable (Gour and Jain, 2019).
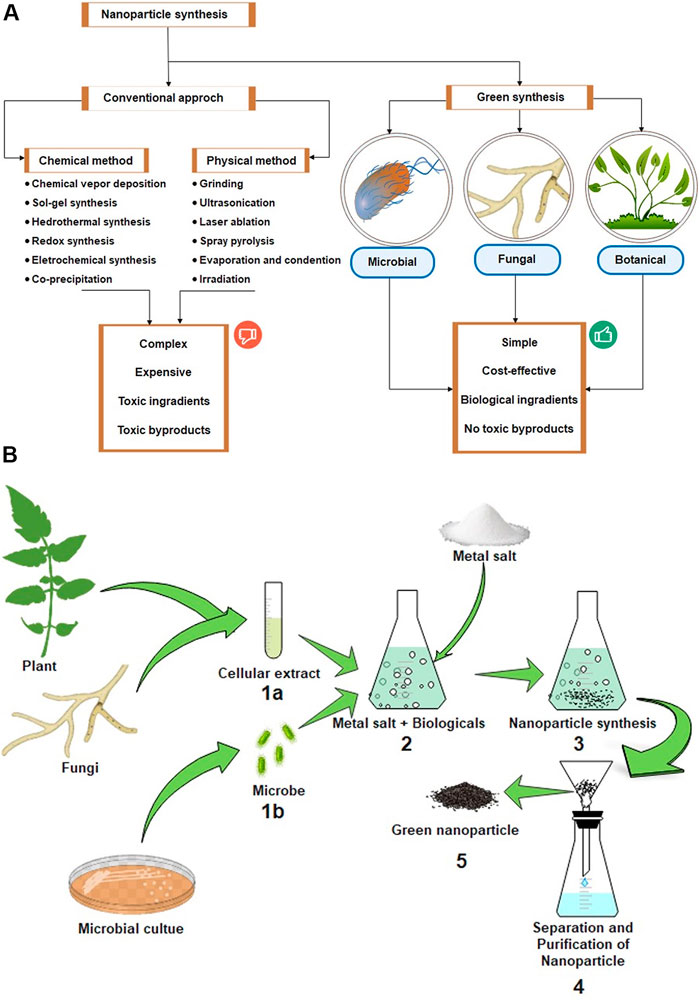
FIGURE 3. (A) Schematic representation of different approaches (conventional and green synthesis) of nanoparticle synthesis and advantages of green synthesis of nanoparticles. (B) Schematic representation of green biogenic nanoparticle synthesis, separation and purification process.
Biogenic nanoparticles can be assorted according to different functional groups with specific functionalities. They may be classified as either intracellular or extracellular performances or organic or inorganic arrangements (Stanley, 2014; Kaushal, 2018). For example, the membranous intracellular organelles like the magnetosome (present in magnetotactic bacteria) and the extracellular assemblies such as lipoproteins (droplets of fat surrounded by a single layer of phospholipid molecules) are significant (Stanley, 2014; Kaushal, 2018). Though the natural sources of such biogenic nanomaterials are plants, bacteria, fungi, or insects, they can be transformed into “green pesticides” to minimize environmental risks (Jadoun et al., 2021). However, one of the significant barriers to developing such nanoinsecticides is the availability of functional ingredients for nanoinsecticide formulation. Most common nanoinsecticides are synthetic products derived from chemicals, though some are hybrids. Hybrid nanoinsecticides are developed with combinations of biological and synthetic chemical nanoparticles. It is seemingly due to the limited choice of biologically active substances or biologically compatible AI-conveyance materials employed during formulation. Exact bio-originated nanoinsecticides where both the AI and nanocarrier come from biological sources are extremely rare. However, research has gained momentum recently, considering its immense prospects for environmental safety and insecticidal efficacy. It could help overcome the hazardous effects of chemical insecticides and inorganic nanoparticles. Some attempts have already been made to develop fully bio-origin nanoinsecticides (Table 1), though there remain many opportunities to explore more. While agreeing with the advantages of bio-nanoinsecticides, it is essential to discover diverse “biologicals” with insecticidal properties to enrich the available biological inventory. At the same time, it is equally important to explore biological sources to screen out “biologicals” that can be manufactured as nanoparticles with AI conveyance properties. In the last few years, many biopolymers like alendronate functionalized gelatin, dipalmitoylphosphatidylcholine, fucoidan, carrageenan, and porphyrin have been used in nanoformulations, and their potential as bio-nanocarriers has been investigated (Mekhail et al., 2016; Manivasagan et al., 2017; Etman et al., 2020). Nevertheless, the application of these potential bio nanocarriers has been restricted to pharmaceutical, drug delivery, and gene therapy purposes.
Green synthesis of biogenic nanoparticles
Since nanoparticles used in agriculture are primarily synthesized through chemical routes, they often cause toxicity (Nath and Banerjee, 2013). However, green synthesis of nanoparticles using living organisms is more helpful in producing highly stable, well-characterized, and safer nanoparticles than chemical methods, which are usually not environmentally friendly, less stable, and not easy to scale up (Pacheco-Aguirre et al., 2016). As biocompatible green synthesis involves living organisms, the use of bio-nanoinsecticides ensures the excellent potential for sustainable practice for pest management (Clark and Macquarrie, 2008). The three leading conditions for the green development of nanoparticles are the choice of a green or environmentally less harmful solvent, an efficient reducing agent, and an eco-friendly stabilization material (Jadoun et al., 2021). All living organism-mediated nanoparticle biosynthesis follows the principle of bottom-up biosynthesis, in which atoms of a specific metal assemble in the presence of reducing agents and ultimately develop nanoscale compounds (Gour and Jain, 2019). It involves using diverse living organisms like bacteria, fungi, actinomycetes, yeast, algae, and plant materials, which have metal-tolerant abilities and flourishes under the utmost environmental conditions (Figure 3A) (Kuppusamy et al., 2016; Phanjom and Ahmed, 2017; Patil and Chandrasekaran, 2020; Jadoun et al., 2021).
Green synthesis using botanicals
Plants are regarded as the chemical factories of nature. Phytochemicals in plant extracts such as polyols, terpenoids, and polyphenols are responsible for metallic ion bioreduction (Kuppusamy et al., 2016; Ovais et al., 2018). Plant-derived metabolites such as phenolics (Moulton et al., 2010), proteins (Sanghi and Verma, 2009), polysaccharides (Wei and Qian, 2008), flavonoids, and tannins (Nam et al., 2008) are synthesized into nanoparticles through eco-friendly methods. Polyphenolic compounds such as rutin, curcumin, ellagic acid and gallic acid have been used to synthesize Ag-NPs (Swilam and Nematallah 2020). Like polyphenols, another secondary metabolite of plants, i.e., flavonoids, a group polyhydroxylated secondary metabolites, are also successfully investigated for the purpose of green synthesis of nanoparticles. Hesperidin, naringin and diosmin like flavonoids that are found in citrus plants have been reported in bio-reduction of silver salts to Ag-NPs of varying sizes (5–80 nm). It is claimed that the hydroxyl groups present in the molecule play the pivotal role in the conversion process (Sahu et al., 2016). In another trial Jain and Mehata (2017) used another flavonoid, quercetin (found in Ocimum sanctum) to develop silver nanoparticles of 11–14 nm size (Jain and Mehata 2017). Tannic acid, which is a representative of tannins, has been well explored for their potential to convert metallic salts into Ag-NPs and Au-NPs (Ahmad, 2014). Polysaccharides are also documented to produce nano metallic compounds from respective salts. Transparent nanoporous gels produced from cellulose, a major component of plant cell wall, is reported to synthesize Ag-NPs and Au-NPs from AgNO3 and HAuCl4.3H2O respectively (Cai et al., 2009). Similarly, another abundant plant polysaccharide, like starch, which is a fusion of a-amylose and amylopectin, can synthesize Ag-NPs of 5.3 nm size from silver salt in presence of glucose (Raveendran et al., 2003).
The reduction of metallic salts to nanoparticles using phytochemicals or plant extracts is considered as an eco-friendly and cost-effective method. In addition, the use of universal solvent, water, as a reducing medium enhances its biocompatibility and reduces the usage or toxic organic solvents. For the synthesis process, specific plant parts that have high phytochemical contents such as dried barks, leaves or roots are also used for extraction, which are then purified by filtration steps. In the succeeding step, various quantities of plant extracts are mixed with the solution of metal salts in water and the mixture is incubated to convert the metal salts into metallic nanoparticles (Figure 3B). The conversion is usually monitored either by visual colour change or by using UV-Vis spectrophotometry.
Plants responsible for accumulating, detoxifying, and phytoremediating toxic metals are mostly used as reducing agents during bottom-up synthesis (Carolin et al., 2017). Medicinal plants are also extensively used in the process because of the high phytochemical contents. There are plenty of evidences that extracts from different plant parts can be used for the biological synthesis of nanoparticles (Kuppusamy et al., 2016). Botanicals are sometimes more advantageous than microorganism-mediated green synthesis, as microbes can only be propagated through complex actions of preserving culture (Hulkoti and Taranath, 2014). Thus, plant-based nanoparticle synthesis has proven to be a better method due to its slower kinetics and better manipulative control over crystal growth and stabilization (Prasad, 2014). Using green technology, Prosopis juliflora (Fabaceae) leaf extract was utilised to synthesize Cu/Zn-bimetallic nanoparticles ranging in size from 74.33 nm to 59.46 nm. Using Cu/Zn solution (100 ppm) and aqueous P. juliflora extracts as controls, this bimetallic nanoparticle was applied to the cotton mealy bug, Phenacoccus solenopsis, and found near about 30% mortality for the pest (Mendez-Trujillo et al., 2019).
Metallic nanomaterials such as gold, copper, silver, zinc oxide, etc., are commonly used as nano insecticidal ingredients through green synthesis (H. Ahmad H et al., 2020; Jadoun et al., 2021; Santhosh et al., 2020; Sharma et al., 2019; Solgi and Taghizadeh, 2020). The insecticidal ability of a phyto-nanoparticle made from zinc oxide and Zingiber officinale rhizome extract was tested on tobacco cutworm, Spodoptera litura and potato aphids, Macrosiphum euphorbiae. This green nanoparticle offers more potential in terms of insecticidal action and environment-friendly nature. At 500 ppm concentration, particular stages of relevant pests revealed nearly 100% death after being exposed for 144 h (Thakur et al., 2022).
Green synthesis using microbes
During the microbe-based synthesis method, microbial culture filtrates (extracellular and intracellular) are used as reducing agents for the green synthesis of nanoparticles (Bahrulolum et al., 2021). The ability of microbes to tolerate, accumulate, and convert metallic mass into individual nanoparticles is studied first in the Gram-positive catalase bacterium Bacillus subtilis (Southam and Beveridge, 1994). Metals are reduced into metallic nanoparticles by bacterial intracellular or extracellular redox reactions.
Bacterial cells contain specific polysaccharide on their cell wall surface known as exopolymeric substances (EPS) which play some crucial role in the formation of nanoparticles. The bacterial EPS is a polyanionic structural component with high abundance of negatively charged hydroxyl and carboxyl group that can reduce metallic salts into respective nanoparticles in a metabolism-independent manner (Sathiyanarayanan et al., 2017; Saha et al., 2022). The EPS of certain species of electroactive bacteria like Shewanella oneidensis, Aeromonas hydrophila, and Pseudomonas putida have been used to synthesize nano silver. Further investigation revealed that the Cytochrome-C present in the EPS is the key contributing component behind the reduction of silver salt to silver nanoparticle (Li et al., 2016). Besides, multiple lactic acid bacteria like Lactobacillus sp., Pediococcus pentosaceus and Enterococcus faecium also have the potential to reduce silver ions to Ag-NPs. Researchers demonstrated that the EPS aids in the process by promoting redox reaction (Saravanan et al., 2017). Additionally, enzymatic conversion of metal salts to metal nanoparticles is also a common bacterial physiology. Plenty of extracellular microbial enzymes that rely on NADP or NADPH can effectively reduce metal ions by transferring electrons (Bose and Chatterjee, 2016). For example, Rhodopseudomonas capsulate secrets extracellular NADH dependent enzymes that catalyses electron transfer to Au3+, which in turn eventually become Au-NP (He et al., 2007). In addition to enzymes, other components of electron transport chain like anthraquinone, hydroquinone and naphthoquinones are also reported to transfer electron to metal salts (Patra et al., 2014). Nanoparticles can also be synthesized intracellularly with the help of multiple reducing enzymes and accumulated in the periplasmic space, cell membrane and wall (Ovais et al., 2018). Extremophiles are the mostly investigated bacterial group that has been studied for nanoparticle biosynthesis. The potential of extremophiles like Rhodococcus sp. (Ahmad et al., 2003a) and Thermomonospora sp. (Ahmad et al., 2003b) have been investigated and it was found that these organisms can promote intracellular gold nanoparticle synthesis of 8–12 nm and 8–40 nm respectively. Few species of Gram-negative bacteria, such as Pseudomonas stutzeri (Klaus et al., 1999) and Shewanella algae (Konishi et al., 2007) can bio-reduce salts to Ag-NP (200 nm) and Au-NP (10–20 nm) respectively and accumulate at periplasmic place.
Using microorganisms, nanoparticles can be synthesized in two ways like, extracellular and intracellular approaches. Firstly, the desired microorganisms are cultured under optimum temperature, media ingredients and pH. In the subsequent steps, the culture is centrifuged to obtain the supernatant which is required to synthesize nanoparticles from sterilized metal salt solution. The supernatant and metal salt solution is incubated together for the bio-reduction of metal salts and the synthesis of nanoparticles is monitored by the appearance of specific coloration of the culturing media like deep brown for Ag-NPs or red to deep purple for Au-NPs. Finally, the media-content is centrifuged with density-gradient to obtain the nanoparticles from the bottom pellet. In intracellular approach, the microbial biomass is collected instead of supernatant in the first step, which is then dissolved in filter sterilized solution of desired metallic salts. After sufficient incubation, the microbial biomass is collected by centrifugation and subject to repeated steps of ultrasonication which eventually breaks the cell wall of the organism. The cell wall rupture causes the release of nanoparticles from the biomass, which is then collected following centrifugation (Figure 3B).
Examples of microbe-containing green synthesis of nanoparticles are generous; in most studies, silver nanoparticles are frequently used. During the last two decades, researchers have synthesized silver nanoparticles using several bacterial strains like Bacillus licheniformis, Bacillus cereus, Pseudomonas proteolytica, Bacillus cecembensis, Lactobacillus casei, Klebsiella pneumonia, Escherichia coli, Enterobacter cloacae, and Bacillus indicus (Kalishwaralal et al., 2008; Shivaji et al., 2011; Korbekandi et al., 2012; Sunkar and Nachiyar, 2012). However, besides silver-based nanoparticles, gold (Au) and iron (Fe2O3) nanoparticles have also been produced from other bacterial strains like Plectonema boryanum 485, Bacillus subtilis 168, Shewanella alga, Bacillus megaterium D01, and Magnetospirillum magnetotacticum (Southam and Beveridge, 1994; Philipse and Maas, 2002; Lengke et al., 2006; Konishi et al., 2007).
Green synthesis using fungi
Nanoparticle biosynthesis using different fungi has been documented so far, suggesting a competent fungal role as a biological agent for producing metallic nanoparticles. Fungi can produce larger quantities of nanoparticles than bacteria (Singh et al., 2018), and diverse groups of intracellular fungal enzymes, proteins, and reducing components facilitate the reduction of metal salts into metal nanoparticles (Chen et al., 2009; Singh et al., 2018). According to some authors mycosynthesis is more straightforward approach than bacterial synthesis as fungal cells have more bioaccumulation capacity and higher tolerance to metals (Gade et al., 2008). Alghuthaymi et al. (2015), mentioned that the reducing enzymes like α-NADPH-dependent reductases, nitrate-dependent reductases are the major cellular constituents that aid in bio-reduction processes. In addition, few extracellular electron transporters like quinone play equally important role in the process.
Similar to bacterial extracts, fungal extracts are used in the mycosynthesis process and the mostly investigated fungal group is filamentous fungi. Different species of filamentous fungi like Aureobasidium pullulans and Fusarium oxysporum has been used to synthesize gold nanoparticles of 29 nm and 128 nm respectively (Zhang et al., 2011). Silver nanoparticle crystal has been synthesised extracellularly using the extracts of black mold (Aspergillus niger), kozi mold (A. oryzae), soil mold (Fusarium solani, F. oxysporum), fibre mold (Phoma glomerata), and cotton mold (Pleurotus Sajor Caju) and yeasts (Ahmad et al., 2003a; Gade et al., 2008; Ingle et al., 2008; Birla et al., 2009; Binupriya et al., 2010; Thakkar et al., 2010; Singh et al., 2018). Apart from these, gold and zinc oxide nanoparticles have also been developed from ascomycete species like filamentous mitosporic fungus (Trichothecium sp) and soil fungus (F. oxysporum or A. terreus) (Ahmad et al., 2005; Senapati et al., 2005; Raliya and Tarafdar, 2014).
Exploration of new “biologicals”
A total of 496 active substances, irrespective of chemical or organic origin, have been registered in the European Commission database to date. Almost 450 of them have insecticidal properties (https://ec.europa.eu/food/plants/pesticides/eu-pesticides-database_en). On the other hand, more than 600 insect pests have developed resistance against conventionally used chemical insecticides (Hawkins et al., 2019) (www.pesticidestewardship.org/resistance). According to EPA United States of America, the use of “biologicals” is the only way to combat the growing tendency of insecticidal resistance for agricultural pests (https://www.epa.gov/pesticides/biopesticides), and hence, in organic farming, the use of bioinsecticides is being popularized instead of synthetic chemicals (Röös et al., 2018; Awasthi, 2021). Unfortunately, only a few of the registered active substances are of biological origin, and therefore, it is imperative to discover more “biologicals” with insecticidal properties to enrich the available biological inventory.
Currently, some biological AIs are used for nano-bioinsecticides. Some of the notable ones are Bt toxins (source: Bacillus thuringiensis), azadirachtin (source: Azadirachta indica), pyrethrin (source: Chrysanthemum cinerariaefolium), rotenone (source: Derris sp, Lonchocarpussp, Tephrosia sp.), curcumin (source: Curcuma longa), allicin (source: Allium cepa), garlicin (source: Allium sativa) and aloin (source: Aloe vera). Nevertheless, numerous microbes, fungi, and plants remain rich in phytochemicals with insecticidal properties. Plants from different families, including Apiaceae, Apocynaceae, Asteraceae, Caesalpinaceae, Cupressaceae, Lamiaceae, Lauraceae, Liliaceae, Myrtaceae, Piperaceae, Poaceae, Rutaceae, Sapotaceae, Solanaceae, Zingiberaceae, have been reported to have bioactive compounds with biocidal activities against agricultural crop pests (Okwute, 2012; Baskar et al., 2017; Khan et al., 2017; Lengai et al., 2020).
Among phytochemicals, the most common bioactive compounds are primarily secondary metabolites such as alkaloids, terpenes, phenolics, flavonoids, etc., that possess multiple biocidal properties, including insecticidal effects (Oskoueian et al., 2011; Singh et al., 2017). For example, Ryanodine (C25H35NO9)—a toxic diterpenoid produced by the South American plant Ryania speciosa (Salicaceae), exhibits species-specific insecticidal activity. The molecule affects muscles by binding to the sarcoplasmic reticulum calcium channels (Feher and Lipford, 1985). This molecule also acts on mitochondria and peroxisomes and eventually interferes with the respiratory chain (Hamilton et al., 2018). Nicotine (C10H14N2) is an anxiolytic chiral alkaloid obtained from the tobacco plant Nicotiana tabacum (Solanaceae) that causes continuous uncontrolled nerve firing by binding with acetylcholine receptors at nerve synapses and executes insecticidal effects on selected insects (Kimura-Kuroda et al., 2012). Further, plant-derived essential oils, such as limonene (C10H16) (a cyclic monoterpene found in citrus fruit peels), eugenol (C10H12O2) (an allyl chain-substituted guaiacol obtained from clove, nutmeg, cinnamon, basil, and bay leaf), eucalyptol (C10H18O) (a bicyclic monoterpenoid ether produced from blue gum, Eucalyptus globules, Myrtaceae) also have potential insecticidal activities (Campolo et al., 2017; Ainane et al., 2019; Ahmed et al., 2021). Annonin (C37H66O7), a complex acetogenin derived from the sugar apple Annona sp. (Annonaceae), is neurotoxic to insects and inhibits NADH cytochrome C-reductase and complex-I of insect mitochondria (Londershausen et al., 1991; Lümmen, 1998). Besides these bioactive substances, several plant species with insecticidal qualities have not yet been explored to identify bioactive substances (Campolo et al., 2018; Ebadollahi et al., 2020). For example, crude extracts of the pantropical nicker bean Caesalpinia bonduc (Caesalpinieae) were tested for their larvicidal and pupicidal activities against the cotton bollworm Dicladispa armigera (Baskar et al., 2017). Similarly, crude essential oil from rock-samphire, Crithmum maritimum (Apiaceae), has been tested on beet armyworm larva, Spodoptera exigua, and stored grain pests like Sitophilus granarius, Sitophilus oryzae, Tribolium castaneum, Tribolium confusum, Rhyzopertha dominica, and Oryzaephilus surinamensis (Polatoğlu et al., 2016). Crude secondary metabolites isolated from Indian birthwort, Aristolochia tagala (Aristolochiaceae) (Baskar et al., 2011), Patagonian slipperwort, Calceolaria talcana (Calceolariaceae) (Muñoz et al., 2013), foetid herb, Cassia tora (Fabaceae) (Baskar and Ignacimuthu, 2012), lesser round weed, Hyptis brevipes (Lamiaceae) (Hamed Sakr et al., 2013), orange climber, Toddalia asiatica (Rutaceae) (Duraipandiyan et al., 2015), African mahogany, Trichilia americana (Meliaceae) (Wheeler and Isman, 2001) have antifeedant, larvicidal, pupicidal, or growth-interrupting roles against different species of lepidopteran pests, including cotton bollworm and fall armyworm.
Concerning other botanicals tested against different lepidopteran pests, solvent extracts from Mexican fireweed, Kochia scoparia (Chenopodiaceae); pokeweed, Phytolacca Americana (Phytolaccaceae); golden larch, Pseudolarix kaempferi (Pinaceae) and black false hellebore, Veratrum nigrum (Melanthiaceae) showed larvicidal activity against P. xyllostella. Plant extracts, like chaste berry, Vitex agnus-castus (Lamiaceae); common rue, Ruta graveolens (Rutaceae); pomegranate, Punica granatum (Lythraceae); olibanum, Boswellia carterii (Burseraceae); wild rue, Peganum harmala (Rutaceae); common myrtle, Myrtus communis (Myrtaceae); squaw mint, Mentha pulegium (Lamiaceae); colocynth, Citrullus colocynthis (Cucurbitaceae); asafoetida, Ferula asafetida (Apiaceae); common wormwood, Artemisia absinthium (Asteraceae); bae laurel, Laurus nobilis (Lauraceae); marjoram, Origanum majorana (Lamiaceae); basil, Ocimum basilicum (Lamiaceae); and oleander, Nerium oleander (Apocynaceae) have also documented to have insecticidal properties (Cheraghi Niroumand et al., 2016; Khan et al., 2017). Out of various floral resources, solanaceous plants mainly produce an array of alkaloids that causes gut injury, metabolic arrest, growth disruption, and reproductive abnormality to a wide range of lepidopteran pests (Chowański et al., 2016); hence, comprehensive explorations of AIs could provide sustenance for expansion of nanoinsecticides. Apart from these, some experimental evidence unfolding possibilities of new botanicals in the formulation of insecticides have been proposed in recent times (Ahmed et al., 2020; Lengai et al., 2020).
Molecular administrations for nanoinsecticide development
Double-stranded RNA (dsRNA) is a new approach to nanoinsecticide development. Biological administration of dsRNA delays specific gene expression of crop pests and restricts pests from infestation (Katoch et al., 2013; Castellanos et al., 2019; Christiaens et al., 2020; Liu et al., 2020; Yan et al., 2020). RNA interference (RNAi) is a gene silencing method caused by double-stranded RNA (dsRNA) that, when ingested by insects, results in the death of the target pest. The RNAi mechanism in a sequence-dependent mode has high target specificity, allowing agrarians to target insects more precisely than conventional agrochemicals and “biologicals”. Despite having substantial advantages, the RNAi-mediated pest management strategy has its limitations when researchers opt for the SIGS (spray-induced gene silencing) approach to trigger RNAi in targeted pests. Firstly, externally applied dsRNA is unstable, and secondly, dsRNA has a feeble penetration ability across the insect cuticle. However, developing nanoinsecticides by encapsulating the desired dsRNA with compatible nanocarriers seems to be a superior alternative to the usually practised nanoinsecticides, as dsDNA’s nanoencapsulation increases penetration efficiency and environmental stability (Ghormade et al., 2011; Liu et al., 2020); however, available literature in this regard is still inadequate.
This idea emerged after Zhang and others (2010) attempted to silence the chitin synthase genes AgCHS1 and AgCHS2 in the African malaria mosquito (Anopheles gambiae) using chitosan/AgCHS dsRNA-based nanoparticles. A 30%–60% reduction of chitin biosynthesis was observed, and as a consequence, susceptibility towards diflubenzuron, calcofluor white (CF), and dithiothreitol had taken place (Zhang et al., 2010). Since then, many researchers have shown interest in this route and have tried different approaches to interfere with insect’s genetic expression. Researchers have optimized the approach of the SIGS-based strategy using different conveyance materials like chitosan and chitosan derivatives, nanoliposomes, cationic dendrimers, quantum dots (QDs), and branch amphiphilic peptide capsules (BAPC) (Yan et al., 2020). After finding initial success with chitosan-encapsulated dsDNA delivery, Zhang et al. (2010) modified the strategy and delivered chitin synthase in A. gambiae using chitosan with smaller particle sizes. The mortality of A. gambiae was increased compared to the previous study due to the smaller-sized chitosan. Later on, delivery approaches were modified using several cross-linking agents like sodium tripolyphosphate, folate, polyethylene glycol, polyethylenimine, and dextran sulfate to increase the transfection and gene silencing efficiency of dsRNA-nanoformulations. Another convenient vehicle to deliver dsRNA to target pests is dendrimers, an artificial polymer with a branched peripheral side chain and a nanoscale internal core. He et al. (2013) developed a CHT10 dsRNA containing dendrimer with a cationic core-shell, which upon application, efficiently diminished the body mass and interfered with the moulting of Ostrinia furnacalis (He et al., 2013). Similarly, spraying a 555 bp double-stranded hemocytin RNA-dendrimer nanoformulation has competently interfered with the target gene expression of Aphis glycines (95%) and effectively suppressed population growth (80%) of the species (Zheng et al., 2019). The use of quantum dots (QDs), which discharge narrow symmetric bands under a broad excitation range, is another exciting approach to dsRNA delivery. Carbon QD-mediated SNF7 and G3PDH gene silencing has been verified as a practical approach to insect pest management (Neng et al., 2019). Other nanoformulations like dsDNA encapsulated in nanoliposomes and branched amphiphilic peptide capsules are also considered practical and convenient. Nano-pGPMA (guanidinium-functionalized inter polyelectrolyte complexes)—an analog of arginine-rich cell-penetrating peptides, can enable RNAi in resistant insect pests. This biomimetic pGMPA encapsulated sequence-specific dsRNA, synthesized by reversible addition-fragmentation chain transfer (α-RAFT) polymerization, and was found to trigger target gene knockdown and subsequent larval mortality in Spodoptera frugiperda (Parsons et al., 2018). Biogenic nanocarrier-like liposome-encapsulated dsRNA formulation with essential gene targeting increases oral RNAi-caused mortality in the neotropical stink bug, Euschistus heros (Castellanos et al., 2019). Thairu et al. (2017) attempted to aerosolize siRNA-nano formulations, which can travel through the spiracular routes of Acyrthosiphon pisum, Aphis glycines, and Schizaphis graminum and target carotene dehydrogenase (tor) and branched-chain amino acid transaminase (b-cat) genes. In this experiment, the efficacy differed based on the target species, but the outcome was satisfactory (Thairu et al., 2017).
In the execution of RNAi manipulations, most of the carriers and matrices used in nanoinsecticides are synthetic polymers or polyester, but there is space for using natural polymers and biomolecules like chitosan alginate or their derivatives as green alternatives. It is expected that biopolymers could have better compatibility with biological AIs, and with this understanding, many biopolymers are under screening for inventories (Sun et al., 2020). For example, non-conventional biomolecules, like tannic acid (polyphenol) (Yu et al., 2019), catecholamines (polydopamine) (Jia et al., 2014), myristic acid (saturated fatty acid) (Ziaee et al., 2014), and cashew gum (cellulose) (Kalia et al., 2011; Abreu et al., 2012), Gelator (Bhagat et al., 2013) are used for AI delivery. Likewise, solid lipids (beeswax) and essential natural oils (corn) are also successfully examined as biological matrixes for formulating nanosuspensions (Nguyen et al., 2012a; H. M; Nguyen et al., 2012b). Zein is a water-insoluble maize-derived prolamine protein and has shown the potential to formulate AI’s nanosuspension (Bidyarani and Kumar, 2019). Non-etheless, bio-nano-cellulose polymers (Kalia et al., 2011), citric acid-glycerol nanopolymers, and citric acid-glycerol-oleic acid nanopolymers (Shiri et al., 2019) have also been developed on different occasions, but their applications are restricted to medicinal purposes only. As the concept of RNAi-mediated dsRNA technology for nanoinsecticide formulation is utterly novel for plant protection, the choice of target sequences and sequence-specific dsRNAs is limited, and further investigation and improvisation are needed to expand the knowledge of the subject.
Biogenic nanoinsecticides: New directions
Fully biogenic or all-green nanoinsecticide formulations have been given attention recently to address environmental issues and combat currently used non-green nanoinsecticides. The exploration of new biological AIs, biogenic nanoparticles, green synthesis technology development, compatible “biologicals”, and bio-nanocarriers has been hypothesized (Figure 4). Although researchers have attempted to develop such bio-nanoinsecticides in the last decade, there remains ample opportunity to go further. For example, Lao et al. (2010) formulated rotenone with an amphiphilic chitosan derivative [N-(octadecanol-1-glycidyl ether)-O-sulfate chitosan (NOSCS)] to increase rotenone’s controlled release ability (Lao et al., 2010). Similarly, nano-micelle formulations from azadirachtin and carboxymethyl chitosan have been developed to increase AI’s bioavailability (Feng and Peng, 2012). Plant essential oils infused in cashew gum (Abreu et al., 2012) or myristic acids (Ziaee et al., 2014) in developing nanogel-based nanoinsecticides were also experimentally proven. Entomopathogenic microbes have also been encapsulated to intensify their efficacy and environmental stability (Chen et al., 2013; Pacheco-Aguirre et al., 2016; Maghsoudi and Jalali, 2017). Furthermore, RNA interference technology has recently been incorporated to develop efficient target-specific activity (Castellanos et al., 2019). Other pieces of evidence are listed in Table 1.
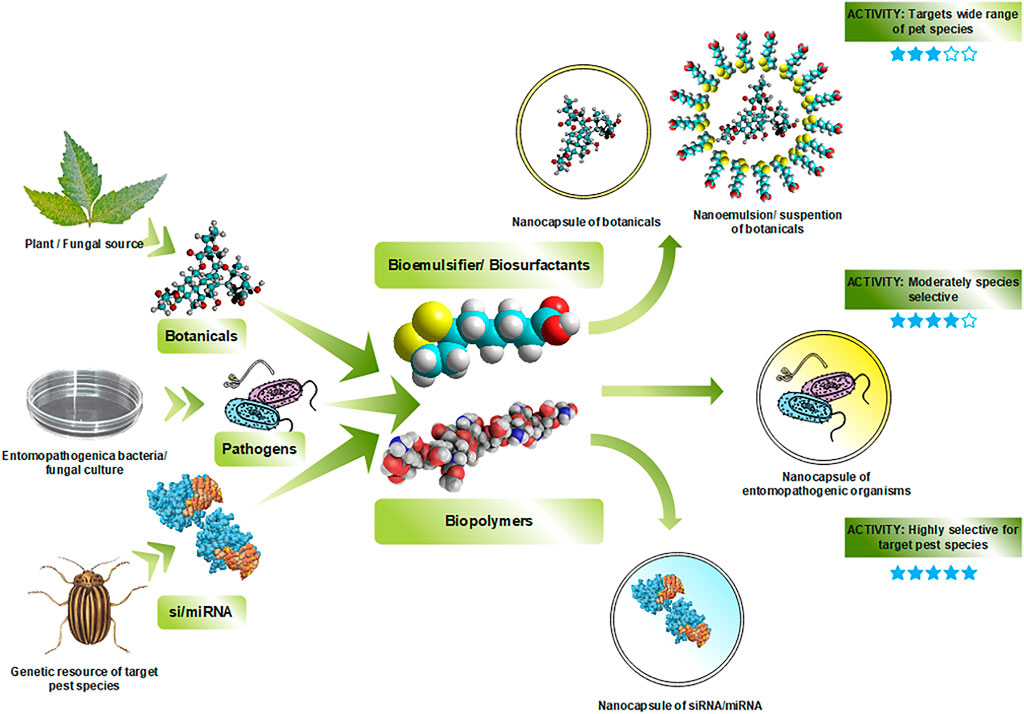
FIGURE 4. Schematic representation of synthesizing fully bio-nanoinsecticides employing both bioactive components/active ingredients (plant-based AI, microorganisms, and siRNA) and carrier/matrix materials (biopolymer) derived from natural sources.
Besides environmental benefits, a fully bio-based nanoinsecticides is efficient for other rewards. They are environmentally stable, have controlled release properties, and are equally effective as conventional nanoinsecticides (Riyajan and Sakdapipanich, 2009; Riyajan, 2011; Choupanian et al., 2017; Khoobdel et al., 2017). For example, R-CM-chitosan/Aza-nanomicelles have demonstrated environmental stability, controlled release properties, and efficacy comparable to many conventional nanoinsecticides (Feng and Peng, 2012). Azadirachtin was also reported to be stable for 11 days during an experimental trial (Riyajan, 2011). In another experiment, azadirachtin formulated in a sodium alginate matrix cross-linked by glutaraldehyde and coated with natural rubber reported 50% remaining AI even after 41 days of experimental trial (Riyajan and Sakdapipanich, 2009). These experimental results can be compared with the usual conventional nanoformulations like PEG-Aza, which have approximately 24 days of environmental sustainability (Kumar et al., 2010). On the question of efficacy, the fully bio-nanoinsecticides have shown challenging insecticidal activity compared with conventional nanoinsecticides. For example, traditionally used bio-nanoinsecticides have more or less >80% insecticidal activity on stored grain pests (Yang et al., 2009; Werdin González et al., 2014; Choupanian et al., 2017; Khoobdel et al., 2017). Similarly, complete natural bio-nanoinsecticides like PMS (Plantago major seed extract) loaded nanoliposomes also showed approximately 70% insecticidal activity on stored grain pests upon application (Khoshraftar et al., 2020). Natural nanogel formulations of cumin essential oil (Cuminum cyminum) and ajwain oil (Carum copticum) have also shown 80%–100% insecticidal activity against the target stored grain pests (Ziaee et al., 2014). Exclusive biogenic nanoformulated avermectin obtained from a soil-dwelling actinomycete, Streptomyces avermitilis, and semi-bio-nanoformulation have also shown more than 80% insecticidal effects on the cabbage moth, Plutella xylostella (Wang et al., 2018).
Another entirely organic nanoinsecticide is tried against cotton leafworm, Spodoptera littoralis, by encapsulating citronella essential oil with chitosan nanoparticle-cellulose nanofiber systems (CSNPs/CNF). By encapsulating the bioactive ingredient, it is protected from environmental degradation. However, in absence of encapsulations, the active compounds were completely lost on or after 6 hours, but in presence of encapsulation, its functionality lasts for 2 weeks. Furthermore, both nanoformulations, particularly the CSNPs/CNF encapsulated formulation, are more effective and delay larval and pupal development, adult longevity, and fecundity (Ibrahim et al., 2022)
Therefore, it can be presumed that an entirely natural bio-nanoinsecticide can be as effective as conventional hybrid bio-nanoinsecticides in terms of their controlled release ability, environmental stability, and insecticidal efficacy. Moreover, the entirely natural bio-nanoinsecticide is more target-specific and thereby causes the least negligible residual effect and environmental toxicity. Though there are only a few reports on fully bio-nanoinsecticides at present, the increase in their number is just a matter of time.
Conclusion
Analyzing the described publications and considering the facts and figures, it can be concluded that the fully organic bio-nanoinsecticides in which the ingredients of nanoinsecticides (AI and carrier molecule) come from biological sources are the most effective nanoinsecticides for pest management. It is environmentally friendly and hence more sustainable than others. Nevertheless, as of now, our choices of “biologicals” and bio-nanocarriers are restricted due to the available known resources, which, in turn, confine the number of compatible combinations of prolific bio-bio-nanoinsecticide formulations. Therefore, it is essential to amplify our exploration of “biologicals”, biogenic nanoparticles, and biopolymers and inventory them. Appropriate identification and characterization of these compounds are needed to assess the potential of these compounds to be a future contributor to bio-nanoinsecticides. Further research and investigation into the biogenic nanoparticles in agricultural croplands is also relatively new and thus demands further research and investigation.
Comprehensive exploration is therefore needed in the next few years and should incorporate:
1. Identification and characterization of “biologicals” and bioactivity screening,
2. Identification of new biogenic nanoparticles of natural origin
3. Technological advancement for the quantitative generation of biogenic nanoparticles
4. Fine optimal compatibility screening for active “biologicals” and greenly synthesized nanoparticles/biogenic nanoparticles/bio-nanopolymers, and
5. Toxicity evaluation of plants and human health
6. Sustainable environmental risk assessment.
Author contributions
SM: Conceptualization, Investigation, Writing—Original Draft. SR: Writing—Reviewing and Editing. AD: Writing—Reviewing and Editing. AR: Supervision. VP: Supervision, Reviewing and Editing. AD: Conceptualization, Investigation, Supervision, Writing—Reviewing and Editing.
Acknowledgments
We acknowledge the Head, Department of Zoology, University of Calcutta, Kolkata, West Bengal, India for providing the necessary laboratory facilities to execute this work.
Conflict of interest
The authors declare that the research was conducted in the absence of any commercial or financial relationships that could be construed as a potential conflict of interest.
Publisher’s note
All claims expressed in this article are solely those of the authors and do not necessarily represent those of their affiliated organizations, or those of the publisher, the editors and the reviewers. Any product that may be evaluated in this article, or claim that may be made by its manufacturer, is not guaranteed or endorsed by the publisher.
References
Abo-Arab, R. B., Amal, M. H., and Hashem, A. S. (2014). Comparative bioactivity of aluminum oxide (Al2O3), titanium dioxide (TiO2) nanoparticles and malathion on Sitophilus oryzae L. And Sitophilus zeamais (motsch.). Glob. J. Agric. Food Saf. Sci. 1, 25–37.
Abreu, F. O. M. S., Oliveira, E. F., Paula, H. C. B., and de Paula, R. C. M. (2012). Chitosan/cashew gum nanogels for essential oil encapsulation. Carbohydr. Polym. 89, 1277–1282. doi:10.1016/j.carbpol.2012.04.048
Adak, T., Kumar, J., Dey, D., Shakil, N. A., and Walia, S. (2012a). Residue and bio-efficacy evaluation of controlled release formulations of imidacloprid against pests in soybean (Glycine max). J. Environ. Sci. Health Part B 47, 226–231. doi:10.1080/03601234.2012.634368
Adak, T., Kumar, J., Shakil, N. A., and Walia, S. (2012b). Development of controlled release formulations of imidacloprid employing novel nano-ranged amphiphilic polymers. J. Environ. Sci. Health B 47, 217–225. doi:10.1080/03601234.2012.634365
Ahmad, A., Mukherjee, P., Senapati, S., Mandal, D., Khan, M. I., Kumar, R., et al. (2003a). Extracellular biosynthesis of silver nanoparticles using the fungus Fusarium oxysporum. Colloids Surf. B Biointerfaces 28, 313–318. doi:10.1016/S0927-7765(02)00174-1
Ahmad, A., Senapati, S., Khan, M. I., Kumar, R., and Sastry, M. (2005). Extra-/Intracellular biosynthesis of gold nanoparticles by an alkalotolerant fungus, <I>Trichothecium</I> sp. J. Biomed. Nanotechnol. 1, 47–53. doi:10.1166/jbn.2005.012
Ahmad, A., Senapati, S., Khan, M. I., Kumar, R., and Sastry, M. (2003b). Extracellular biosynthesis of monodisperse gold nanoparticles by a novel extremophilic actinomycete, Thermomonospora sp. Langmuir 19, 3550–3553. doi:10.1021/la026772l
Ahmad, H., Venugopal, K., Rajagopal, K., De Britto, S., Nandini, B., Pushpalatha, H. G., et al. (2020). Green synthesis and characterization of zinc oxide nanoparticles using Eucalyptus globules and their fungicidal ability against pathogenic fungi of apple orchards. Biomolecules 10, 425. doi:10.3390/biom10030425
Ahmad, I., Irfan, S., Dera, A. A., Zaman, G. S., Chandramoorthy, H. C., Mir, M. A., et al. (2020). GC-MS analysis of ethanol extract from areal parts of Nepeta deflersiana and its anticancer and antimicrobial efficacies. Biol. (Bratisl.) 1–12, 1739–1750. doi:10.2478/s11756-020-00473-3
Ahmad, T. (2014). Reviewing the tannic acid mediated synthesis of metal nanoparticles. J. Nanotechnol. 2014, 1–11. doi:10.1155/2014/954206
Ahmed, K. (2019). Effect of lambda-cyahalothrin as nanopesticide on cotton leafworm, Spodoptera littoralis (boisd.). J. Chem. 0, 0. doi:10.21608/ejchem.2019.6871.1581
Ahmed, M., Peiwen, Q., Gu, Z., Liu, Y., Sikandar, A., Hussain, D., et al. (2020). Insecticidal activity and biochemical composition of Citrullus colocynthis , Cannabis indica and Artemisia argyi extracts against cabbage aphid ( Brevicoryne brassicae L.). Sci. Rep. 10, 522. doi:10.1038/s41598-019-57092-5
Ahmed, Q., Agarwal, M., Al-Obaidi, R., Wang, P., and Ren, Y. (2021). Evaluation of Aphicidal effect of essential oils and their synergistic effect against Myzus persicae (Sulzer)(Hemiptera: Aphididae). Molecules 26, 3055. doi:10.3390/molecules26103055
Ainane, A., Khammour, F., Charaf, S., Elabboubi, M., Elkouali, M., Talbi, M., et al. (2019). Chemical composition and insecticidal activity of five essential oils: Cedrus atlantica, Citrus limonum, Rosmarinus officinalis, Syzygium aromaticum and Eucalyptus globules. Mat. Today Proc. 13, 474–485. doi:10.1016/j.matpr.2019.04.004
Alghuthaymi, M. A., Almoammar, H., Rai, M., Said-Galiev, E., and Abd-Elsalam, K. A. (2015). Myconanoparticles: Synthesis and their role in phytopathogens management. Biotechnol. Biotechnol. Equip. 29, 221–236. doi:10.1080/13102818.2015.1008194
Ali, S., Sagheer, M., Ul, M., Abbas, M., Hafeez, F., Farooq, M., et al. (2014). Insecticidal activity of turmeric (Curcuma longa) and garlic (Allium sativum) extracts against red flour beetle, Tribolium castaneum: A safe alternative to insecticides in stored commodities. J. Entomol. Zool. Stud. 201, 1.
Anjali, C. H., Sudheer Khan, S., Margulis-Goshen, K., Magdassi, S., Mukherjee, A., and Chandrasekaran, N. (2010). Formulation of water-dispersible nanopermethrin for larvicidal applications. Ecotoxicol. Environ. Saf. 73, 1932–1936. doi:10.1016/j.ecoenv.2010.08.039
Awad, M., Ibrahim, E.-D. S., Osman, E. I., Elmenofy, W. H., Mahmoud, A. W. M., Atia, M. A., et al. (2022). Nano-insecticides against the black cutworm Agrotis ipsilon (Lepidoptera: Noctuidae): Toxicity, development, enzyme activity, and DNA mutagenicity. Plos One 17, e0254285. doi:10.1371/journal.pone.0254285
Ayoub, H. A., Khairy, M., Rashwan, F. A., and Abdel-Hafez, H. F. (2017). Synthesis and characterization of silica nanostructures for cotton leaf worm control. J. Nanostructure Chem. 7, 91–100. doi:10.1007/s40097-017-0229-2
Bahrulolum, H., Nooraei, S., Javanshir, N., Tarrahimofrad, H., Mirbagheri, V. S., Easton, A. J., et al. (2021). Green synthesis of metal nanoparticles using microorganisms and their application in the agrifood sector. J. Nanobiotechnology 19, 86–26. doi:10.1186/s12951-021-00834-3
Ball, V. (2018). Polydopamine nanomaterials: Recent advances in synthesis methods and applications. Front. Bioeng. Biotechnol. 6, 109. doi:10.3389/fbioe.2018.00109
Baskar, K., and Ignacimuthu, S. (2012). Antifeedant, larvicidal and growth inhibitory effects of ononitol monohydrate isolated from Cassia tora L. against Helicoverpa armigera (Hub.) and Spodoptera litura (Fab.) (Lepidoptera: Noctuidae). Chemosphere 88, 384–388. doi:10.1016/j.chemosphere.2012.02.051
Baskar, K., Pavunraj, M., Packiam, S., Ignacimuthu, S., Duraipandiyan, V., and Benelli, G. (2017). Toxicity and antifeedant activity of Caesalpinia bonduc (L.) Roxb. (Caesalpiniaceae) extracts and fractions against the cotton bollworm Helicoverpa armigera Hub. (Lepidoptera: Noctuidae). Physiol. Mol. Plant Pathol. 101, 69–74. doi:10.1016/j.pmpp.2017.01.006
Baskar, K., Sasikumar, S., Muthu, C., Kingsley, S., and Ignacimuthu, S. (2011). Bioefficacy of Aristolochia tagala cham. Against Spodoptera litura fab. (Lepidoptera: Noctuidae). Saudi J. Biol. Sci. 18, 23–27. doi:10.1016/j.sjbs.2010.09.004
Bergeson, L. L. (2010). Nanosilver: US EPA’s pesticide office considers how best to proceed. Environ. Qual. Manag. 19, 79–85. doi:10.1002/tqem.20255
Betteridge, D. J. (2000). What is oxidative stress? Metabolism 49, 3–8. doi:10.1016/s0026-0495(00)80077-3
Bhagat, D., Samanta, S. K., and Bhattacharya, S. (2013). Efficient management of fruit pests by pheromone nanogels. Sci. Rep. 3, 1294. doi:10.1038/srep01294
Bhan, S., Mohan, L., and Srivastava, C. N. (2014). Relative larvicidal potentiality of nano-encapsulated Temephos and Imidacloprid against Culex quinquefasciatus. J. Asia-Pac. Entomol. 17, 787–791. doi:10.1016/j.aspen.2014.07.006
Bidyarani, N., and Kumar, U. (2019). Synthesis of rotenone loaded zein nano-formulation for plant protection against pathogenic microbes. RSC Adv. 9, 40819–40826. doi:10.1039/C9RA08739G
Binupriya, A. R., Sathishkumar, M., and Yun, S.-I. (2010). Myco-crystallization of silver ions to nanosized particles by live and dead cell filtrates of Aspergillus oryzae var. viridis and its bactericidal activity toward Staphylococcus aureus KCCM 12256. Ind. Eng. Chem. Res. 49, 852–858. doi:10.1021/ie9014183
Birla, S. S., Tiwari, V. V., Gade, A. K., Ingle, A. P., Yadav, A. P., and Rai, M. K. (2009). Fabrication of silver nanoparticles by Phoma glomerata and its combined effect against Escherichia coli, Pseudomonas aeruginosa and Staphylococcus aureus. Lett. Appl. Microbiol. 48, 173–179. doi:10.1111/j.1472-765X.2008.02510.x
Boehm, A. L., Martinon, I., Zerrouk, R., Rump, E., and Fessi, H. (2003). Nanoprecipitation technique for the encapsulation of agrochemical active ingredients. J. Microencapsul. 20, 433–441. doi:10.1080/0265204021000058410
Bose, D., and Chatterjee, S. (2016). Biogenic synthesis of silver nanoparticles using guava (Psidium guajava) leaf extract and its antibacterial activity against Pseudomonas aeruginosa. Appl. Nanosci. 6, 895–901. doi:10.1007/s13204-015-0496-5
Bourguet, D., and Guillemaud, T. (2016). “The hidden and external costs of pesticide use,” in Sustainable agriculture reviews: Volume 19, sustainable agriculture reviews. Editor E. Lichtfouse (Cham: Springer International Publishing), 35–120.
Buteler, M., Lopez Garcia, G., and Stadler, T. (2018). Potential of nanostructured alumina for leaf-cutting ants Acromyrmex lobicornis (Hymenoptera: Formicidae) management. Austral Entomol. 57, 292–296. doi:10.1111/aen.12277
Cai, J., Kimura, S., Wada, M., and Kuga, S. (2009). Nanoporous cellulose as metal nanoparticles support. Biomacromolecules 10, 87–94. doi:10.1021/bm800919e
Camara, M. C., Campos, E. V. R., Monteiro, R. A., do Espirito Santo Pereira, A., de Freitas Proença, P. L., and Fraceto, L. F. (2019). Development of stimuli-responsive nano-based pesticides: Emerging opportunities for agriculture. J. Nanobiotechnology 17, 100. doi:10.1186/s12951-019-0533-8
Campolo, O., Cherif, A., Ricupero, M., Siscaro, G., Grissa-Lebdi, K., Russo, A., et al. (2017). Citrus peel essential oil nanoformulations to control the tomato borer, tuta absoluta : Chemical properties and biological activity. Sci. Rep. 7, 13036. doi:10.1038/s41598-017-13413-0
Campolo, O., Giunti, G., Russo, A., Palmeri, V., and Zappalà, L. (2018). Essential oils in stored product insect pest control. J. Food Qual. 2018, 1–18. doi:10.1155/2018/6906105
Carbone, K., Santangelo, E., Angelis, A., Micheli, L., Frosinini, R., Gargani, E., et al. (2020). “Green synthesis of silver nanoparticles from hyperpigmented tomato skins and preliminary evaluation of the insecticidal activity,” in 28th European Biomass Conference and Exhibition.
Carolin, C. F., Kumar, P. S., Saravanan, A., Joshiba, G. J., and Naushad, Mu. (2017). Efficient techniques for the removal of toxic heavy metals from aquatic environment: A review. J. Environ. Chem. Eng. 5, 2782–2799. doi:10.1016/j.jece.2017.05.029
Castellanos, N. L., Smagghe, G., Sharma, R., Oliveira, E. E., and Christiaens, O. (2019). Liposome encapsulation and EDTA formulation of dsRNA targeting essential genes increase oral RNAi-caused mortality in the Neotropical stink bug Euschistus heros. Pest Manag. Sci. 75, 537–548. doi:10.1002/ps.5167
Chae, Y., and An, Y.-J. (2016). Toxicity and transfer of polyvinylpyrrolidone-coated silver nanowires in an aquatic food chain consisting of algae, water fleas, and zebrafish. Aquat. Toxicol. 173, 94–104. doi:10.1016/j.aquatox.2016.01.011
Chaud, M., Souto, E. B., Zielinska, A., Severino, P., Batain, F., Oliveira-Junior, J., et al. (2021). Nanopesticides in agriculture: Benefits and challenge in agricultural productivity, toxicological risks to human health and environment. Toxics 9, 131. doi:10.3390/toxics9060131
Chen, J., Li, H., Han, X., and Wei, X. (2015). Transmission and accumulation of nano-TiO 2 in a 2-step food chain (Scenedesmus obliquus to Daphnia magna). Bull. Environ. Contam. Toxicol. 95, 145–149. doi:10.1007/s00128-015-1580-y
Chen, J., Wu, L., Lu, M., Lu, S., Li, Z., and Ding, W. (2020). Comparative study on the fungicidal activity of metallic MgO nanoparticles and macroscale MgO against soilborne fungal phytopathogens. Front. Microbiol. 11, 365. doi:10.3389/fmicb.2020.00365
Chen, K.-N., Chen, C.-Y., Lin, Y.-C., and Chen, M.-J. (2013). Formulation of a novel antagonistic bacterium based biopesticide using microencapsulated techniques in fungal disease control. J. Agric. Sci. 5, p153. doi:10.5539/jas.v5n3p153
Chen, K., Fu, Z., Wang, M., Lv, Y., Wang, C., Shen, Y., et al. (2018). Preparation and characterization of size-controlled nanoparticles for high-loading λ-cyhalothrin delivery through flash nanoprecipitation. J. Agric. Food Chem. 66, 8246–8252. doi:10.1021/acs.jafc.8b02851
Chen, K., Wang, Y., Cui, H., Wei, Z., Jia, X., Liu, Z., et al. (2020). Difunctional fluorescence nanoparticles for accurate tracing of nanopesticide fate and crop protection prepared by flash nanoprecipitation. J. Agric. Food Chem. 68, 735–741. doi:10.1021/acs.jafc.9b06744
Chen, X., Qiu, L., Liu, Q., and He, Y. (2022). Preparation of an environmentally friendly nano-insecticide through encapsulation in polymeric liposomes and its insecticidal activities against the fall armyworm, Spodoptera frugiperda. Insects 13, 625. doi:10.3390/insects13070625
Chen, Y.-L., Tuan, H.-Y., Tien, C.-W., Lo, W.-H., Liang, H.-C., and Hu, Y.-C. (2009). Augmented biosynthesis of cadmium sulfide nanoparticles by genetically engineered Escherichia coli. Biotechnol. Prog. 25, 1260–1266. doi:10.1002/btpr.199
Cheraghi Niroumand, M., Farzaei, M. H., Karimpour Razkenari, E., Amin, G., Khanavi, M., Akbarzadeh, T., et al. (2016). An evidence-based review on medicinal plants used as insecticide and insect repellent in traditional Iranian medicine. Red. Crescent Med. J. 18, e22361. doi:10.5812/ircmj.22361
Choi, A.-J., Kim, C.-J., Cho, Y.-J., Hwang, J.-K., and Kim, C.-T. (2011). Characterization of capsaicin-loaded nanoemulsions stabilized with alginate and chitosan by self-assembly. Food Bioprocess Technol. 4, 1119–1126. doi:10.1007/s11947-011-0568-9
Choupanian, M., Omar, D., Basri, M., and Asib, N. (2017). Preparation and characterization of neem oil nanoemulsion formulations against <i>Sitophilus oryzae</i> and <i>Tribolium castaneum</i> adults. J. Pestic. Sci. 42, 158–165. doi:10.1584/jpestics.D17-032
Chowański, S., Adamski, Z., Marciniak, P., Rosiński, G., Büyükgüzel, E., Büyükgüzel, K., et al. (2016). A review of bioinsecticidal activity of Solanaceae alkaloids. Toxins 8, 60. doi:10.3390/toxins8030060
Christiaens, O., Whyard, S., Vélez, A. M., and Smagghe, G. (2020). Double-stranded RNA technology to control insect pests: Current status and challenges. Front. Plant Sci. 11, 451. doi:10.3389/fpls.2020.00451
Clark, J. H., and Macquarrie, D. J. (2008). Handbook of green chemistry and technology. John Wiley & Sons.
Côa, F., Bortolozzo, L. S., Petry, R., Da Silva, G. H., Martins, C. H. Z., de Medeiros, A. M. Z., et al. (2020). “Environmental toxicity of nanopesticides against non-target organisms: The state of the art,” in Nanopesticides: From research and development to mechanisms of action and sustainable use in agriculture. Editors L. F. Fraceto, S. S. de Castro, R. Grillo, D. Ávila, H. Caixeta Oliveiraet al. (Cham: Springer International Publishing), 227–279.
Cui, B., Feng, L., Pan, Z., Yu, M., Zeng, Z., Sun, C., et al. (2015). Evaluation of stability and biological activity of solid nanodispersion of lambda-cyhalothrin. PLOS ONE 10, e0135953. doi:10.1371/journal.pone.0135953
Cui, B., Gao, F., Zeng, Z., Wang, C., Wang, Y., Sun, C., et al. (2020). Construction and characterization of avermectin B 2 solid nanodispersion. Sci. Rep. 10, 9096–9099. doi:10.1038/s41598-020-66098-3
Dang, F., Chen, Y.-Z., Huang, Y.-N., Hintelmann, H., Si, Y.-B., and Zhou, D.-M. (2019). Discerning the sources of silver nanoparticle in a terrestrial food chain by stable isotope tracer technique. Environ. Sci. Technol. 53, 3802–3810. doi:10.1021/acs.est.8b06135
Debnath, N., Das, S., Seth, D., Chandra, R., Bhattacharya, S. Ch., and Goswami, A. (2011). Entomotoxic effect of silica nanoparticles against Sitophilus oryzae (L.). J. Pest Sci. 84, 99–105. doi:10.1007/s10340-010-0332-3
Deka, B., Babu, A., Baruah, C., and Barthakur, M. (2021). Nanopesticides: A systematic review of their prospects with special reference to tea pest management. Front. Nutr. 8, 1. doi:10.3389/fnut.2021.686131
Deng, R., Lin, D., Zhu, L., Majumdar, S., White, J. C., Gardea-Torresdey, J. L., et al. (2017). Nanoparticle interactions with co-existing contaminants: Joint toxicity, bioaccumulation and risk. Nanotoxicology 11, 591–612. doi:10.1080/17435390.2017.1343404
Desheesh, M. A., El-Masry, D. M., Fargand, M. M., and Youssef, H. M. (2019). Larvicidal activity of nano- encapsulated lambda – cyhalothrin against susceptible mosquito larvae (Culex pipiens) in comparison with conventional form. Alex. Sci. Exch. J. 40, 568–573. doi:10.21608/asejaiqjsae.2019.58477
Djiwanti, S. R., and Kaushik, S. (2019). “Nanopesticide: Future application of nanomaterials in plant protection,” in Plant nanobionics: Volume 2, approaches in nanoparticles, biosynthesis, and toxicity, nanotechnology in the life sciences. Editor R. Prasad (Cham: Springer International Publishing), 255–298.
Duraipandiyan, V., et al. Duraipandiyan, V., Baskar, K., Muthu, C., Ignacimuthu, S., and Al-Dhabi, N. A. (2015). Bioefficacy of flindersine against Helicoverpa armigera hübner, Spodoptera litura fabricius, Anopheles stephensis liston. And Culex quinquefasciatus say. Braz. Arch. Biol. Technol. 58, 595–604. doi:10.1590/S1516-8913201500282
Ebadollahi, A., Ziaee, M., and Palla, F. (2020). Essential oils extracted from different species of the Lamiaceae plant family as prospective bioagents against several detrimental pests. Molecules 25, 1556. doi:10.3390/molecules25071556
El Borady, O. (2016). Remakable enhancement of cyhalothrin upon loading into silver nanoparticles as larvicidal, 252–264.
El-Naggar, M. E., Abdelsalam, N. R., Fouda, M. M. G., Mackled, M. I., Al-Jaddadi, M. A. M., Ali, H. M., et al. (2020). Soil application of nano silica on maize yield and its insecticidal activity against some stored insects after the post-harvest. Nanomaterials 10, 739. doi:10.3390/nano10040739
El-Samahy, M., El-Ghobary, A., and Khafagy, I. (2014). Using silica nanoparticles and neemoil extract as new approaches to control tuta absoluta (meyrick) in tomato under field conditions. Int. J. Plant Soil Sci. 3, 1355–1365. doi:10.9734/IJPSS/2014/8435
Elek, N., Hoffman, R., Raviv, U., Resh, R., Ishaaya, I., and Magdassi, S. (2010). Novaluron nanoparticles: Formation and potential use in controlling agricultural insect pests. Colloids Surf. Physicochem. Eng. Asp. 372, 66–72. doi:10.1016/j.colsurfa.2010.09.034
Etman, S. M., Elnaggar, Y. S., and Abdallah, O. Y. (2020). Fucoidan, a natural biopolymer in cancer combating: From edible algae to nanocarrier tailoring. Int. J. Biol. Macromol. 147, 799–808. doi:10.1016/j.ijbiomac.2019.11.191
Fayez, K. A., El-Deeb, B. A., and Mostafa, N. Y. (2017). Toxicity of biosynthetic silver nanoparticles on the growth, cell ultrastructure and physiological activities of barley plant. Acta Physiol. Plant. 39, 155. doi:10.1007/s11738-017-2452-3
Feher, J. J., and Lipford, G. B. (1985). Mechanism of action of ryanodine on cardiac sarcoplasmic reticulum. Biochim. Biophys. Acta BBA-Biomembr. 813, 77–86. doi:10.1016/0005-2736(85)90347-5
Feng, B.-H., and Peng, L.-F. (2012). Synthesis and characterization of carboxymethyl chitosan carrying ricinoleic functions as an emulsifier for azadirachtin. Carbohydr. Polym. 88, 576–582. doi:10.1016/j.carbpol.2012.01.002
Flores-Castañeda, M., Campos González, E., Camps, E., Ruiz-Aguilar, I., Cruces, M. P., Pimentel, E., et al. (2019). Insecticide activity of bifenthrin nanoparticles synthesized by laser ablation of solids in liquids. Superf. Vacío 32, 27–30. doi:10.47566/2019_syv32_1-010027
Fojtová, D., Vašíčková, J., Grillo, R., Bílková, Z., Šimek, Z., Neuwirthová, N., et al. (2019). Nanoformulations can significantly affect pesticide degradation and uptake by earthworms and plants. Environ. Chem. 16, 470–481. doi:10.1071/EN19057
Frederiksen, H. K., Kristensen, H. G., and Pedersen, M. (2003). Solid lipid microparticle formulations of the pyrethroid gamma-cyhalothrin-incompatibility of the lipid and the pyrethroid and biological properties of the formulations. J. Control. Release Off. J. Control. Release Soc. 86, 243–252. doi:10.1016/s0168-3659(02)00406-6
Gade, A. K., Bonde, P., Ingle, A. P., Marcato, P. D., Durán, N., and Rai, M. K. (2008). Exploitation of <I>Aspergillus Niger</I> for synthesis of silver nanoparticles. J. Biobased Mat. Bioenergy 2, 243–247. doi:10.1166/jbmb.2008.401
Gao, Y., Zhang, Y., He, S., Xiao, Y., Qin, X., Zhang, Y., et al. (2019). Fabrication of a hollow mesoporous silica hybrid to improve the targeting of a pesticide. Chem. Eng. J. 364, 361–369. doi:10.1016/j.cej.2019.01.105
Ghormade, V., Deshpande, M. V., and Paknikar, K. M. (2011). Perspectives for nano-biotechnology enabled protection and nutrition of plants. Biotechnol. Adv. 29, 792–803. doi:10.1016/j.biotechadv.2011.06.007
Gour, A., and Jain, N. K. (2019). Advances in green synthesis of nanoparticles. Artif. Cells Nanomedicine Biotechnol. 47, 844–851. doi:10.1080/21691401.2019.1577878
Grillo, R., Fraceto, L. F., Amorim, M. J. B., Scott-Fordsmand, J. J., Schoonjans, R., and Chaudhry, Q. (2021). Ecotoxicological and regulatory aspects of environmental sustainability of nanopesticides. J. Hazard. Mat. 404, 124148. doi:10.1016/j.jhazmat.2020.124148
Guan, H., Chi, D., Yu, J., and Li, X. (2008). A novel photodegradable insecticide: Preparation, characterization and properties evaluation of nano-Imidacloprid. Pestic. Biochem. Physiol. 92, 83–91. doi:10.1016/j.pestbp.2008.06.008
Guo, M., Zhang, W., Ding, G., Guo, D., Zhu, J., Wang, B., et al. (2015). Preparation and characterization of enzyme-responsive emamectin benzoate microcapsules based on a copolymer matrix of silica–epichlorohydrin–carboxymethylcellulose. RSC Adv. 5, 93170–93179. doi:10.1039/C5RA17901G
Hamed Sakr, H., Roshdy, S., and El-Seedi, H. (2013). Hyptis brevipes (Lamiaceae) extracts strongly inhibit the growth and development of Spodoptera littoralis (boisd.) larvae (Lepidoptera: Noctuidae) ARTICLE INFO ABSTRACT. J. Appl. Pharm. Sci. 3, 83–88. doi:10.7324/JAPS.2013.31014
Hamilton, S., Terentyeva, R., Kim, T. Y., Bronk, P., Clements, R. T., Csordás, G., et al. (2018). Pharmacological modulation of mitochondrial Ca2+ content regulates sarcoplasmic reticulum Ca2+ release via oxidation of the ryanodine receptor by mitochondria-derived reactive oxygen species. Front. Physiol. 9, 1831. doi:10.3389/fphys.2018.01831
Hawkins, N. J., Bass, C., Dixon, A., and Neve, P. (2019). The evolutionary origins of pesticide resistance. Biol. Rev. 94, 135–155. doi:10.1111/brv.12440
He, B., Chu, Y., Yin, M., Müllen, K., An, C., and Shen, J. (2013). Fluorescent nanoparticle delivered dsRNA toward genetic control of insect pests. Adv. Mat. 25, 4580–4584. doi:10.1002/adma.201301201
He, S., Guo, Z., Zhang, Y., Zhang, S., Wang, J., and Gu, N. (2007). Biosynthesis of gold nanoparticles using the bacteria Rhodopseudomonascapsulata. Mater. Lett. 61, 3984–3987. doi:10.1016/j.matlet.2007.01.018
Hulkoti, N. I., and Taranath, T. C. (2014). Biosynthesis of nanoparticles using microbes—a review. Colloids Surf. B Biointerfaces 121, 474–483. doi:10.1016/j.colsurfb.2014.05.027
Hwang, I. C., Kim, T. H., Bang, S. H., Kim, K. S., Kwon, H. R., Seo, M. J., et al. (2011). Insecticidal effect of controlled release formulations of etofenprox based on nano-bio technique. J. Fac. Agric. Kyushu Univ. 56, 33–40. doi:10.5109/19633
Ibrahim, S. S., Abou-Elseoud, W. S., Elbehery, H. H., and Hassan, M. L. (2022). Chitosan-cellulose nanoencapsulation systems for enhancing the insecticidal activity of citronella essential oil against the cotton leafworm Spodoptera littoralis. Ind. Crops Prod. 184, 115089. doi:10.1016/j.indcrop.2022.115089
Ingle, A., Rai, M., Gade, A., and Bawaskar, M. (2008). Fusarium solani: A novel biological agent for the extracellular synthesis of silver nanoparticles. J. Nanoparticle Res. 11, 2079–2085. doi:10.1007/s11051-008-9573-y
Ishaque, M., Schnabel, G., and Anspaugh, D. D. (2013). Agrochemical formulations comprising a pesticide, an organic UV-photoprotective filter and coated metal-oxide nanoparticles. US8404263B2.
Jackson, B. P., Bugge, D., Ranville, J. F., and Chen, C. Y. (2012). Bioavailability, toxicity, and bioaccumulation of quantum dot nanoparticles to the amphipod Leptocheirus plumulosus. Environ. Sci. Technol. 46, 5550–5556. doi:10.1021/es202864r
Jadoun, S., Arif, R., Jangid, N. K., and Meena, R. K. (2021). Green synthesis of nanoparticles using plant extracts: A review. Environ. Chem. Lett. 19, 355–374. doi:10.1007/s10311-020-01074-x
Jain, S., and Mehata, M. S. (2017). Medicinal plant leaf extract and pure flavonoid mediated green synthesis of silver nanoparticles and their enhanced antibacterial property. Sci. Rep. 7, 15867. doi:10.1038/s41598-017-15724-8
Jameel, M., Shoeb, M., Khan, M. T., Ullah, R., Mobin, M., Farooqi, M. K., et al. (2020). Enhanced insecticidal activity of thiamethoxam by zinc oxide nanoparticles: A novel nanotechnology approach for pest control. ACS Omega 5, 1607–1615. doi:10.1021/acsomega.9b03680
Jampílek, J., and Kráľová, K. (2019). Nanobiopesticides in agriculture: State of the art and future opportunities. Nano-Biopestic. Today Future Perspect. 1, 397–447.
Jampilek, J., Zaruba, K., Oravec, M., Kunes, M., Babula, P., Ulbrich, P., et al. (2015). Preparation of silica nanoparticles loaded with nootropics and their in vivo permeation through blood-brain barrier. BioMed Res. Int. 2015, 812673. doi:10.1155/2015/812673
Jerobin, J., Sureshkumar, R. S., Anjali, C. H., Mukherjee, A., and Chandrasekaran, N. (2012). Biodegradable polymer based encapsulation of neem oil nanoemulsion for controlled release of Aza-A. Carbohydr. Polym. 90, 1750–1756. doi:10.1016/j.carbpol.2012.07.064
Jia, X., Sheng, W., Li, W., Tong, Y., Liu, Z., and Zhou, F. (2014). Adhesive polydopamine coated avermectin microcapsules for prolonging foliar pesticide retention. ACS Appl. Mat. Interfaces 6, 19552–19558. doi:10.1021/am506458t
Kah, M., Beulke, S., Tiede, K., and Hofmann, T. (2013). Nanopesticides: State of knowledge, environmental fate, and exposure modeling. Crit. Rev. Environ. Sci. Technol. 43, 1823–1867. doi:10.1080/10643389.2012.671750
Kah, M., and Hofmann, T. (2014). Nanopesticide research: Current trends and future priorities. Environ. Int. 63, 224–235. doi:10.1016/j.envint.2013.11.015
Kah, M., Johnston, L. J., Kookana, R. S., Bruce, W., Haase, A., Ritz, V., et al. (2021). Comprehensive framework for human health risk assessment of nanopesticides. Nat. Nanotechnol. 16, 955–964. doi:10.1038/s41565-021-00964-7
Kah, M., Kookana, R. S., Gogos, A., and Bucheli, T. D. (2018). A critical evaluation of nanopesticides and nanofertilizers against their conventional analogues. Nat. Nanotechnol. 13, 677–684. doi:10.1038/s41565-018-0131-1
Kah, M. (2015). Nanopesticides and nanofertilizers: Emerging contaminants or opportunities for risk mitigation? Front. Chem. 3, 64. doi:10.3389/fchem.2015.00064
Kah, M., Weniger, A.-K., and Hofmann, T. (2016). Impacts of (Nano)formulations on the fate of an insecticide in soil and consequences for environmental exposure assessment. Environ. Sci. Technol. 50, 10960–10967. doi:10.1021/acs.est.6b02477
S. Kalia, B. S. Kaith, and I. Kaur (Editors) (2011). Cellulose fibers: Bio- and nano-polymer composites: Green chemistry and technology (Berlin Heidelberg: Springer-Verlag).
Kalishwaralal, K., Deepak, V., Ramkumarpandian, S., Nellaiah, H., and Sangiliyandi, G. (2008). Extracellular biosynthesis of silver nanoparticles by the culture supernatant of Bacillus licheniformis. Mat. Lett. 62, 4411–4413. doi:10.1016/j.matlet.2008.06.051
Kalman, J., Paul, K. B., Khan, F. R., Stone, V., and Fernandes, T. F. (2015). Characterisation of bioaccumulation dynamics of three differently coated silver nanoparticles and aqueous silver in a simple freshwater food chain. Environ. Chem. 12, 662–672. doi:10.1071/en15035
Kang, M. A., Seo, M. J., Hwang, I. C., Jang, C., Park, H. J., Yu, Y. M., et al. (2012). Insecticidal activity and feeding behavior of the green peach aphid, Myzuspersicae, after treatment with nano types of pyrifluquinazon. J. Asia-Pacific Entomology 15, 533–541. doi:10.1016/j.aspen.2012.05.015
Kango, S., Kalia, S., Celli, A., Njuguna, J., Habibi, Y., and Kumar, R. (2013). Surface modification of inorganic nanoparticles for development of organic–inorganic nanocomposites—a review. Prog. Polym. Sci. 38, 1232–1261. doi:10.1016/j.progpolymsci.2013.02.003
Kantrao, S., Ravindra, M. A., Akbar, S. M. D., Kamala Jayanthi, P. D., and Venkataraman, A. (2017). Effect of biosynthesized Silver nanoparticles on growth and development of Helicoverpa armigera (Lepidoptera: Noctuidae): Interaction with midgut protease. J. Asia-Pacific Entomology 20, 583–589. doi:10.1016/j.aspen.2017.03.018
Kariyanna, B., Prabhuraj, A., Asokan, R., Agrawal, A., Gandhi Gracy, R., Jyoti, P., et al. (2020). Genome mining and expression analysis of carboxylesterase and glutathione S-transferase genes involved in insecticide resistance in eggplant shoot and fruit borer, leucinodes orbonalis (Lepidoptera: Crambidae). Front. Physiol. 11, 594845. doi:10.3389/fphys.2020.594845
Katoch, R., Sethi, A., Thakur, N., and Murdock, L. L. (2013). RNAi for insect control: Current perspective and future challenges. Appl. Biochem. Biotechnol. 171, 847–873. doi:10.1007/s12010-013-0399-4
Kaushal, M. (2018). “Role of microbes in plant protection using intersection of nanotechnology and biology,” in Nanobiotechnology applications in plant protection (Springer), 111–135.
Kaziem, A. E., Gao, Y., Zhang, Y., Qin, X., Xiao, Y., Zhang, Y., et al. (2018). α-Amylase triggered carriers based on cyclodextrin anchored hollow mesoporous silica for enhancing insecticidal activity of avermectin against Plutella xylostella. J. Hazard. Mat. 359, 213–221. doi:10.1016/j.jhazmat.2018.07.059
Khan, S., Taning, C. N. T., Bonneure, E., Mangelinckx, S., Smagghe, G., and Shah, M. M. (2017). Insecticidal activity of plant-derived extracts against different economically important pest insects. Phytoparasitica 45, 113–124. doi:10.1007/s12600-017-0569-y
Khoobdel, M., Ahsaei, S. M., and Farzaneh, M. (2017). Insecticidal activity of polycaprolactone nanocapsules loaded with Rosmarinus officinalis essential oil in Tribolium castaneum (Herbst). Entomol. Res. 47, 175–184. doi:10.1111/1748-5967.12212
Khoshraftar, Z., Shamel, A., Safekordi, A. A., Ardjmand, M., and Zaefizadeh, M. (2020). Natural nanopesticides with origin of Plantago major seeds extract for Tribolium castaneum control. J. Nanostructure Chem. 10, 255–264. doi:10.1007/s40097-020-00346-w
Kilani-Morakchi, S., Morakchi-Goudjil, H., and Sifi, K. (2021). Azadirachtin-based insecticide: Overview, risk assessments and future directions. Front. Agron. 3, 32. doi:10.3389/fagro.2021.676208
Kim, J. S., and Je, Y. H. (2012). Milling effect on the control efficacy of spray-dried Bacillus thuringiensis technical powder against diamondback moths. Pest Manag. Sci. 68, 321–323. doi:10.1002/ps.2330
Kimura-Kuroda, J., Komuta, Y., Kuroda, Y., Hayashi, M., and Kawano, H. (2012). Nicotine-like effects of the neonicotinoid insecticides acetamiprid and imidacloprid on cerebellar neurons from neonatal rats. PLoS One 7, e32432. doi:10.1371/journal.pone.0032432
Klaus, T., Joerger, R., Olsson, E., and Granqvist, C.-G. (1999). Silver-based crystalline nanoparticles, microbially fabricated. Proc. Natl. Acad. Sci. 96, 13611–13614. doi:10.1073/pnas.96.24.13611
Konishi, Y., Ohno, K., Saitoh, N., Nomura, T., Nagamine, S., Hishida, H., et al. (2007). Bioreductive deposition of platinum nanoparticles on the bacterium Shewanella algae. J. Biotechnol. 128, 648–653. doi:10.1016/j.jbiotec.2006.11.014
Kookana, R. S., Boxall, A. B. A., Reeves, P. T., Ashauer, R., Beulke, S., Chaudhry, Q., et al. (2014). Nanopesticides: Guiding principles for regulatory evaluation of environmental risks. J. Agric. Food Chem. 62, 4227–4240. doi:10.1021/jf500232f
Korbekandi, H., Iravani, S., and Abbasi, S. (2012). Optimization of biological synthesis of silver nanoparticles using Lactobacillus casei subsp. casei. J. Chem. Technol. Biotechnol. 87, 932–937. doi:10.1002/jctb.3702
Kovacic, P., and Somanathan, R. (2013). Nanoparticles: Toxicity, radicals, electron transfer, and antioxidants. Oxidative Stress Nanotechnol. 1028, 15–35. doi:10.1007/978-1-62703-475-3_2
Kumar, J., Shakil, N. A., Singh, Manish K., PankajSingh, Mukesh K., Pandey, A., Pandey, R. P., et al. (2010). Development of controlled release formulations of azadirachtin-A employing poly(ethylene glycol) based amphiphilic copolymers. J. Environ. Sci. Health Part B 45, 310–314. doi:10.1080/03601231003704457
Kumar, S., Chauhan, N., Gopal, M., Kumar, R., and Dilbaghi, N. (2015). Development and evaluation of alginate–chitosan nanocapsules for controlled release of acetamiprid. Int. J. Biol. Macromol. 81, 631–637. doi:10.1016/j.ijbiomac.2015.08.062
Kumar, S., Nehra, M., Dilbaghi, N., Marrazza, G., Hassan, A. A., and Kim, K.-H. (2019). Nano-based smart pesticide formulations: Emerging opportunities for agriculture. J. Control. Release Off. J. Control. Release Soc. 294, 131–153. doi:10.1016/j.jconrel.2018.12.012
Kuppusamy, P., Yusoff, M. M., Maniam, G. P., and Govindan, N. (2016). Biosynthesis of metallic nanoparticles using plant derivatives and their new avenues in pharmacological applications–An updated report. Saudi Pharm. J. 24, 473–484. doi:10.1016/j.jsps.2014.11.013
Küünal, S., Rauwel, P., and Rauwel, E. (2018). “Plant extract mediated synthesis of nanoparticles,” in Emerging applications of nanoparticles and architecture nanostructures (Elsevier), 411–446.
Lade, B. (2017). Nano bio pesticide to constraint plant destructive pests. J. Nanomedicine Res. 6, 1. doi:10.15406/jnmr.2017.06.00158
Lai, F., Wissing, S. A., Müller, R. H., and Fadda, A. M. (2006). Artemisia arborescens L essential oil-loaded solid lipid nanoparticles for potential agricultural application: Preparation and characterization. AAPS PharmSciTech 7, E10–E18. doi:10.1208/pt070102
Lao, S.-B., Zhang, Z.-X., Xu, H.-H., and Jiang, G.-B. (2010). Novel amphiphilic chitosan derivatives: Synthesis, characterization and micellar solubilization of rotenone. Carbohydr. Polym. 82, 1136–1142. doi:10.1016/j.carbpol.2010.06.044
Lee, W.-M., An, Y.-J., Yoon, H., and Kweon, H.-S. (2008). Toxicity and bioavailability of copper nanoparticles to the terrestrial plants mung bean (Phaseolus radiatus) and wheat (Triticum aestivum): Plant agar test for water-insoluble nanoparticles. Environ. Toxicol. Chem. 27, 1915–1921. doi:10.1897/07-481.1
Lengai, G. M. W., Muthomi, J. W., and Mbega, E. R. (2020). Phytochemical activity and role of botanical pesticides in pest management for sustainable agricultural crop production. Sci. Afr. 7, e00239. doi:10.1016/j.sciaf.2019.e00239
Lengke, M. F., Ravel, B., Fleet, M. E., Wanger, G., Gordon, R. A., and Southam, G. (2006). Mechanisms of gold bioaccumulation by filamentous cyanobacteria from gold(III)−Chloride complex. Environ. Sci. Technol. 40, 6304–6309. doi:10.1021/es061040r
Li, M., Huang, Q., and Wu, Y. (2011). A novel chitosan-poly(lactide) copolymer and its submicron particles as imidacloprid carriers. Pest Manag. Sci. 67, 831–836. doi:10.1002/ps.2120
Li, M., Xu, G., Yang, X., Zeng, Y., and Yu, Y. (2020). Metal oxide nanoparticles facilitate the accumulation of bifenthrin in earthworms by causing damage to body cavity. Environ. Pollut. 263, 114629. doi:10.1016/j.envpol.2020.114629
Li, S.-W., Zhang, X., and Sheng, G.-P. (2016). Silver nanoparticles formation by extracellular polymeric substances (EPS) from electroactive bacteria. Environ. Sci. Pollut. Res. 23, 8627–8633. doi:10.1007/s11356-016-6105-7
Li, Z., Wang, H., An, S., and Yin, X. (2021). Nanochitin whisker enhances insecticidal activity of chemical pesticide for pest insect control and toxicity. J. Nanobiotechnology 19, 49. doi:10.1186/s12951-021-00792-w
Liang, J., Yu, M., Guo, L., Cui, B., Zhao, X., Sun, C., et al. (2018). Bioinspired development of P(St-MAA)-Avermectin nanoparticles with high affinity for foliage to enhance folia retention. J. Agric. Food Chem. 66, 6578–6584. doi:10.1021/acs.jafc.7b01998
Lippert, P. C., and Zachos, J. C. (2007). A biogenic origin for anomalous fine-grained magnetic material at the Paleocene-Eocene boundary at Wilson Lake, New Jersey. Paleoceanography 22, 1. doi:10.1029/2007PA001471
Liu, S., Jaouannet, M., Dempsey, D. A., Imani, J., Coustau, C., and Kogel, K.-H. (2020). RNA-based technologies for insect control in plant production. Biotechnol. Adv. 39, 107463. doi:10.1016/j.biotechadv.2019.107463
Liu, Y., Tong, Z., and Prud’homme, R. K. (2008). Stabilized polymeric nanoparticles for controlled and efficient release of bifenthrin. Pest Manag. Sci. 64, 808–812. doi:10.1002/ps.1566
Locatelli, G. O., dos Santos, G. F., Botelho, P. S., Finkler, C. L. L., and Bueno, L. A. (2018). Development of Trichoderma sp. formulations in encapsulated granules (CG) and evaluation of conidia shelf-life. Biol. Control 117, 21–29. doi:10.1016/j.biocontrol.2017.08.020
Loha, K. M., Shakil, N. A., Kumar, J., Singh, M. K., and Srivastava, C. (2012). Bio-efficacy evaluation of nanoformulations of β-cyfluthrin against Callosobruchus maculatus (Coleoptera: Bruchidae). J. Environ. Sci. Health, Part B 47, 687–691. doi:10.1080/03601234.2012.669254
Londershausen, M., Leicht, W., Lieb, F., Moeschler, H., and Weiss, H. (1991). Molecular mode of action of annonins. Pestic. Sci. 33, 427–438. doi:10.1002/ps.2780330405
López-García, G. P., Buteler, M., and Stadler, T. (2018). Testing the insecticidal activity of nanostructured alumina on Sitophilus oryzae (L.) (Coleoptera: Curculionidae) under laboratory conditions using galvanized steel containers. Insects 9, 87. doi:10.3390/insects9030087
Louni, M., Shakarami, J., and Negahban, M. (2018). Insecticidal efficacy of nanoemulsion containing Mentha longifolia essential oil against ephestia kuehniella (Lepidoptera: Pyralidae). J. Crop Prot. 7, 171–182.
Lümmen, P. (1998). Complex I inhibitors as insecticides and acaricides1Dedicated to the memory of Dr. Gerhard Salbeck.1. Biochim. Biophys. Acta BBA - Bioenerg. 1364, 287–296. doi:10.1016/S0005-2728(98)00034-6
Lynge, M. E., Westen, R. V. D., Postma, A., and Städler, B. (2011). Polydopamine—A nature-inspired polymer coating for biomedical science. Nanoscale 3, 4916–4928. doi:10.1039/C1NR10969C
Maghsoudi, S., and Jalali, E. (2017). Noble UV protective agent for Bacillus thuringiensis based on a combination of graphene oxide and olive oil. Sci. Rep. 7, 11019. doi:10.1038/s41598-017-11080-9
Malaikozhundan, B., Vaseeharan, B., Vijayakumar, S., and Thangaraj, M. P. (2017). Bacillus thuringiensis coated zinc oxide nanoparticle and its biopesticidal effects on the pulse beetle, Callosobruchus maculatus. J. Photochem Photobiol. B 174, 306–314. doi:10.1016/j.jphotobiol.2017.08.014
Malathi, S., Rameshkumar, G., Rengarajan, R. L., Rajagopal, T., Muniasamy, S., Ponmanickam, P., et al. (2019). Phytofabrication of silver nanoparticles using Annona reticulata and assessment of insecticidal and bactericidal activities. J. Environ. Biol. 40, 626–633. doi:10.22438/jeb/40/4/mrn-934
Manivasagan, P., Bharathiraja, S., Moorthy, M. S., Oh, Y.-O., Seo, H., and Oh, J. (2017). Marine biopolymer-based nanomaterials as a novel platform for theranostic applications. Polym. Rev. 57, 631–667. doi:10.1080/15583724.2017.1311914
Maruyama, C. R., Guilger, M., Pascoli, M., Bileshy-José, N., Abhilash, P. C., Fraceto, L. F., et al. (2016). Nanoparticles based on chitosan as carriers for the combined herbicides imazapic and imazapyr. Sci. Rep. 6, 19768. doi:10.1038/srep19768
McTeer, J., Dean, A. P., White, K. N., and Pittman, J. K. (2014). Bioaccumulation of silver nanoparticles into Daphnia magna from a freshwater algal diet and the impact of phosphate availability. Nanotoxicology 8, 305–316. doi:10.3109/17435390.2013.778346
Medina-Pérez, G., Fernández-Luqueño, F., Campos-Montiel, R. G., Sánchez-López, K. B., Afanador-Barajas, L. N., and Prince, L. (2019). “Nanotechnology in crop protection: Status and future trends,” in Nano-biopesticides today and future perspectives (Elsevier), 17–45.
Mekhail, G. M., Kamel, A. O., Awad, G. A., Mortada, N. D., Rodrigo, R. L., Spagnuolo, P. A., et al. (2016). Synthesis and evaluation of alendronate-modified gelatin biopolymer as a novel osteotropic nanocarrier for gene therapy. Nanomed 11, 2251–2273. doi:10.2217/nnm-2016-0151
Memarizadeh, N., Ghadamyari, M., Adeli, M., and Talebi, K. (2014). Preparation, characterization and efficiency of nanoencapsulated imidacloprid under laboratory conditions. Ecotoxicol. Environ. Saf. 107, 77–83. doi:10.1016/j.ecoenv.2014.05.009
Mendez-Trujillo, V., Valdez-Salas, B., Carrillo-Beltran, M., Curiel-Alvarez, M., Tzintzun-Camacho, O., Ceceña-Duran, C., et al. (2019). Green synthesis of bimetallic nanoparticles from Prosopis juliflora (Sw) DC., and its effect against cotton mealybug, Phenacoccus solenopsis (Hemiptera: Pseudococcidae). Phyton 88, 269–275. doi:10.32604/phyton.2019.07316
Moulton, M. C., Braydich-Stolle, L. K., Nadagouda, M. N., Kunzelman, S., Hussain, S. M., and Varma, R. S. (2010). Synthesis, characterization and biocompatibility of “green” synthesized silver nanoparticles using tea polyphenols. Nanoscale 2, 763–770. doi:10.1039/c0nr00046a
Muñoz, E., Lamilla, C., Marin, J. C., Alarcon, J., and Cespedes, C. L. (2013). Antifeedant, insect growth regulatory and insecticidal effects of Calceolaria talcana (Calceolariaceae) on Drosophila melanogaster and Spodoptera frugiperda. Ind. Crops Prod. 42, 137–144. doi:10.1016/j.indcrop.2012.05.014
Murthy, K. S., Vineela, V., and Devi, P. S. V. (2014). Generation of nanoparticles from technical powder of the insecticidal bacterium Bacillus thuringiensis var. kurstaki for improving efficacy. Int. J. Biomed. Nanosci. Nanotechnol. 3, 236. doi:10.1504/IJBNN.2014.065470
Mustafa, I. F., and Hussein, M. Z. (2020). Synthesis and technology of nanoemulsion-based pesticide formulation. Nanomaterials 10, 1608. doi:10.3390/nano10081608
Nam, K. T., Lee, Y. J., Krauland, E. M., Kottmann, S. T., and Belcher, A. M. (2008). Peptide-mediated reduction of silver ions on engineered biological scaffolds. Acs Nano 2, 1480–1486. doi:10.1021/nn800018n
Nath, D., and Banerjee, P. (2013). Green nanotechnology – a new hope for medical biology. Environ. Toxicol. Pharmacol. 36, 997–1014. doi:10.1016/j.etap.2013.09.002
Neng, W., Cheng, X., Li, N., Wang, H., and Chen, H. (2019). Nanocarriers and their loading strategies. Adv. Healthc. Mat. 8, 1801002. doi:10.1002/adhm.201801002
Nguyen, H. M., Hwang, I.-C., Park, J.-W., and Park, H.-J. (2012a). Photoprotection for deltamethrin using chitosan-coated beeswax solid lipid nanoparticles. Pest Manag. Sci. 68, 1062–1068. doi:10.1002/ps.3268
Nguyen, H. M., Hwang, I. C., Park, J. W., and Park, H. J. (2012b). Enhanced payload and photo-protection for pesticides using nanostructured lipid carriers with corn oil as liquid lipid. J. Microencapsul. 29, 596–604. doi:10.3109/02652048.2012.668960
Nuruzzaman, Md., Rahman, M. M., Liu, Y., and Naidu, R. (2016). Nanoencapsulation, nano-guard for pesticides: A new window for safe application. J. Agric. Food Chem. 64, 1447–1483. doi:10.1021/acs.jafc.5b05214
Okwute, S. K. (2012). Plants as potential sources of pesticidal agents: A review. Pestic. Chem. Bot. Pestic. 10, 208–232.
Oskoueian, E., Abdullah, N., Ahmad, S., Saad, W. Z., Omar, A. R., and Ho, Y. W. (2011). Bioactive compounds and biological activities of Jatropha curcas L. kernel meal extract. Int. J. Mol. Sci. 12, 5955–5970. doi:10.3390/ijms12095955
Othman, Z., Zubairi, Ts.Dr.S., Sarmidi, M., and Aziz, R. (2016). Environmental friendly bio-pesticide rotenone extracted from Derris sp. A review on the extraction method, toxicity and field effectiveness. J. Teknol. 78, 47–69. doi:10.11113/jt.v78.5942
Ovais, M., Khalil, A. T., Islam, N. U., Ahmad, I., Ayaz, M., Saravanan, M., et al. (2018). Role of plant phytochemicals and microbial enzymes in biosynthesis of metallic nanoparticles. Appl. Microbiol. Biotechnol. 102, 6799–6814. doi:10.1007/s00253-018-9146-7
Pacheco-Aguirre, J., Borges-Gómez, L., Tún-Suárez, J. M., Cristóbal-Alejo, J., Reyes-Ramírez, A., and Ruiz-Sánchez, E. (2016). Polymer-based encapsulation of Bacillus subtilis and its effect on Meloidogyne incognita in tomato. Aires: Phyton B.
Pankaj, S., Shakil, N. A., Kumar, J., Singh, M. K., and Singh, K. (2012). Bioefficacy evaluation of controlled release formulations based on amphiphilic nano-polymer of carbofuran against Meloidogyne incognita infecting tomato. J. Environ. Sci. Health, Part B 47, 520–528. doi:10.1080/03601234.2012.665667
Panova, G. G., Serebryakov, E. B., Semenov, K. N., Charykov, N. A., Shemchuk, O. S., Andrusenko, E. V., et al. (2019). Bioactivity study of the C60-L-Threonine derivative for potential application in agriculture. J. Nanomater. 2019, 2306518. doi:10.1155/2019/2306518
Paramo, L. A., Feregrino-Pérez, A. A., Guevara, R., Mendoza, S., and Esquivel, K. (2020). Nanoparticles in agroindustry: Applications, toxicity, challenges, and trends. Nanomaterials 10, 1654. doi:10.3390/nano10091654
Park, M., Lee, C.-I., Seo, Y. J., Woo, S. R., Shin, D., and Choi, J. (2010). Hybridization of the natural antibiotic, cinnamic acid, with layered double hydroxides (LDH) as green pesticide. Environ. Sci. Pollut. Res. 17, 203–209. doi:10.1007/s11356-009-0235-0
Park, S., and Ahn, Y.-J. (2016). Multi-walled carbon nanotubes and silver nanoparticles differentially affect seed germination, chlorophyll content, and hydrogen peroxide accumulation in carrot (Daucus carota L.). Biocatal. Agric. Biotechnol. 8, 257–262. doi:10.1016/j.bcab.2016.09.012
Parsons, K. H., Mondal, M. H., McCormick, C. L., and Flynt, A. S. (2018). Guanidinium-functionalized interpolyelectrolyte complexes enabling RNAi in resistant insect pests. Biomacromolecules 19, 1111–1117. doi:10.1021/acs.biomac.7b01717
Pascoli, M., Jacques, M. T., Agarrayua, D. A., Avila, D. S., Lima, R., and Fraceto, L. F. (2019). Neem oil based nanopesticide as an environmentally-friendly formulation for applications in sustainable agriculture: An ecotoxicological perspective. Sci. Total Environ. 677, 57–67. doi:10.1016/j.scitotenv.2019.04.345
Patel, S., Bajpai, J., Saini, R., Bajpai, A. K., and Acharya, S. (2018). Sustained release of pesticide (Cypermethrin) from nanocarriers: An effective technique for environmental and crop protection. Process Saf. Environ. Prot. 117, 315–325. doi:10.1016/j.psep.2018.05.012
Patil, R. D., and Bendre, R. S. (2022). Encapsulation of bioinsecticide oil in phenol-urea-formaldehyde terpolymer. J. Macromol. Sci. Part A 1, 1–13.
Patil, S., and Chandrasekaran, R. (2020). Biogenic nanoparticles: A comprehensive perspective in synthesis, characterization, application and its challenges. J. Genet. Eng. Biotechnol. 18, 67. doi:10.1186/s43141-020-00081-3
Patra, C. R., Mukherjee, S., and Kotcherlakota, R. (2014). Biosynthesized silver nanoparticles: A step forward for cancer theranostics? Nanomedicine 9, 1445–1448. doi:10.2217/nnm.14.89
Pavela, R. (2014). Limitation of plant biopesticides. Adv. Plant Biopestic. 1, 347–359. doi:10.1007/978-81-322-2006-0_17
Pelegrino, M. T., Kohatsu, M. Y., Seabra, A. B., Monteiro, L. R., Gomes, D. G., Oliveira, H. C., et al. (2020). Effects of copper oxide nanoparticles on growth of lettuce (Lactuca sativa L.) seedlings and possible implications of nitric oxide in their antioxidative defense. Environ. Monit. Assess. 192, 232. doi:10.1007/s10661-020-8188-3
Perlatti, B., Bergo, P. L., Silva, M. F., das, G. F., Fernandes, J. B., and Forim, M. R. (2013). Polymeric nanoparticle-based insecticides: A controlled release purpose for agrochemicals. Insectic. - Dev. Safer More Eff. Technol. 1, 1. doi:10.5772/53355
Phanjom, P., and Ahmed, G. (2017). Effect of different physicochemical conditions on the synthesis of silver nanoparticles using fungal cell filtrate of Aspergillus oryzae (MTCC No. 1846) and their antibacterial effect. Adv. Nat. Sci. Nanosci. Nanotechnol. 8, 045016. doi:10.1088/2043-6254/aa92bc
Philipse, A. P., and Maas, D. (2002). Magnetic colloids from magnetotactic bacteria: Chain formation and colloidal stability. Langmuir 18, 9977–9984. doi:10.1021/la0205811
Pires-Oliveira, R., Kfouri, M. S., Mendonça, B., and Cardoso-Gustavson, P. (2020). “Nanopesticides: From the bench to the market,” in Nanopesticides: From research and development to mechanisms of action and sustainable use in agriculture. Editors L. F. Fraceto, S. S. de Castro, R. Grillo, D. Ávila, H. Caixeta Oliveiraet al. (Cham: Springer International Publishing), 317–348.
Polatoğlu, K., Karakoç, Ö. C., Yücel, Y. Y., Gücel, S., Demirci, B., Başer, K. H. C., et al. (2016). Insecticidal activity of edible Crithmum maritimum L. essential oil against Coleopteran and Lepidopteran insects. Ind. Crops Prod. 89, 383–389. doi:10.1016/j.indcrop.2016.05.032
Pradhan, S., Roy, I., Lodh, G., Patra, P., Choudhury, S. R., Samanta, A., et al. (2013). Entomotoxicity and biosafety assessment of PEGylated acephate nanoparticles: A biologically safe alternative to neurotoxic pesticides. J. Environ. Sci. Health B 48, 559–569. doi:10.1080/03601234.2013.774891
Prasad, R. (2014). Synthesis of silver nanoparticles in photosynthetic plants. J. Nanoparticles 2014, 1.
Qian, H., Peng, X., Han, X., Ren, J., Sun, L., and Fu, Z. (2013). Comparison of the toxicity of silver nanoparticles and silver ions on the growth of terrestrial plant model Arabidopsis thaliana. J. Environ. Sci. 25, 1947–1956. doi:10.1016/S1001-0742(12)60301-5
Rahwanudin, I., Susanto, A., Panatarani, C., Zainuddin, A., and Setiawati, W. (2022). Study of spinetoram nano suspension for environmentally friendly control of Plutella xylostella L.(Lepidoptera: Plutellidae). Biocatal. Agric. Biotechnol. 44, 102456. doi:10.1016/j.bcab.2022.102456
Rai, M., and Ingle, A. (2012). Role of nanotechnology in agriculture with special reference to management of insect pests. Appl. Microbiol. Biotechnol. 94, 287–293. doi:10.1007/s00253-012-3969-4
Rajeshwari, A., Suresh, S., Chandrasekaran, N., and Mukherjee, A. (2016). Toxicity evaluation of gold nanoparticles using an Allium cepa bioassay. RSC Adv. 6, 24000–24009. doi:10.1039/C6RA04712B
Raliya, R., and Tarafdar, J. C. (2014). Biosynthesis and characterization of zinc, magnesium and titanium nanoparticles: An eco-friendly approach. Int. Nano Lett. 4, 93. doi:10.1007/s40089-014-0093-8
Rashwan, R. S., and Abu-Zaid, A. A. (2018). Evaluation of nutritive values and microbial content of wheat grains treated with some types of nano particles to control Sitophilus granaries (L.). Arab Univ. J. Agric. Sci. 26, 293–301. doi:10.21608/ajs.2018.14006
Rastogi, A., Tripathi, D. K., Yadav, S., Chauhan, D. K., Živčák, M., Ghorbanpour, M., et al. (2019). Application of silicon nanoparticles in agriculture. 3 Biotech. 9, 90. doi:10.1007/s13205-019-1626-7
Raveendran, P., Fu, J., and Wallen, S. L. (2003). Completely “green” synthesis and stabilization of metal nanoparticles. J. Am. Chem. Soc. 125, 13940–13941. doi:10.1021/ja029267j
Renault, S., Baudrimont, M., Mesmer-Dudons, N., Gonzalez, P., Mornet, S., and Brisson, A. (2008). Impacts of gold nanoparticle exposure on two freshwater species: A phytoplanktonic alga (scenedesmus subspicatus) and a benthic bivalve (corbicula fluminea). Gold Bull. 41, 116–126. doi:10.1007/bf03216589
Riyajan, S.-A. (2011). Development of neem capsule via biopolymer and natural rubber for its controlled release.
Riyajan, S.-A., and Sakdapipanich, J. (2009). Development of a controlled release neem capsule with a sodium alginate matrix, crosslinked by glutaraldehyde and coated with natural rubber. Polym. Bull. 63, 609–622. doi:10.1007/s00289-009-0126-z
Röös, E., Mie, A., Wivstad, M., Salomon, E., Johansson, B., Gunnarsson, S., et al. (2018). Risks and opportunities of increasing yields in organic farming. A review. A Rev. Agron. sustain. Dev. 38, 14–21. doi:10.1007/s13593-018-0489-3
Sabry, A. H., Salem, H. A.-N., and Metwally, H. M. (2021). Development of imidacloprid and indoxacarb formulations to nanoformulations and their efficacy against Spodoptera littoralis (Boisd). Bull. Natl. Res. Cent. 45, 16. doi:10.1186/s42269-020-00477-8
Saha, R., Banerjee, D. B., Manna, S., and Banerjee, S. (2022). “Chapter 12 - microbial bioremediation: A promising approach to withstand heavy metal contamination in soil and its future possibilities,” in Synergistic Approaches for Bioremediation of environmental pollutants : Recent Advances and challenges developments in applied microbiology and biotechnology. Editors R. T. Kapoor, and M. P. Shah (Academic Press), 227–262. doi:10.1016/B978-0-323-91860-2.00018-X
Sahab, A., Waly, A. I., Sabbour, M., and Nawar, L. S. (2015). Synthesis, antifungal and insecticidal potential of chitosan (CS)-g-poly (acrylic acid) (PAA) nanoparticles against some seed borne fungi and insects of soybean. Int. J. ChemTech Res. 8, 589–598.
Sahu, N., Soni, D., Chandrashekhar, B., Satpute, D. B., Saravanadevi, S., Sarangi, B. K., et al. (2016). Synthesis of silver nanoparticles using flavonoids: Hesperidin, naringin and diosmin, and their antibacterial effects and cytotoxicity. Int. Nano Lett. 6, 173–181. doi:10.1007/s40089-016-0184-9
Saini, P., Gopal, M., Kumar, R., and Srivastava, C. (2014). Development of pyridalyl nanocapsule suspension for efficient management of tomato fruit and shoot borer (Helicoverpa armigera). J. Environ. Sci. Health Part B 49, 344–351. doi:10.1080/03601234.2014.882168
Samhadaneh, D. M., Alqarni, K. A., Smart, A., Kuang, M., Moujaber, O., Maysinger, D., et al. (2019). Gold nanourchins induce cellular stress, impair proteostasis and damage RNA. Nanomedicine Nanotechnol. Biol. Med. 22, 102083. doi:10.1016/j.nano.2019.102083
Sanghi, R., and Verma, P. (2009). Biomimetic synthesis and characterisation of protein capped silver nanoparticles. Bioresour. Technol. 100, 501–504. doi:10.1016/j.biortech.2008.05.048
Santaella, C., and Plancot, B. (2020). “Interactions of nanoenabled agrochemicals with soil microbiome,” in Nanopesticides (Springer), 137–163.
Santhosh, A., Theertha, V., Prakash, P., and Chandran, S. (2020). From waste to a value added product: Green synthesis of silver nanoparticles from onion peels together with its diverse applications. Mat. Today Proc. 46, 4460–4463. doi:10.1016/j.matpr.2020.09.680
Saravanan, C., Rajesh, R., Kaviarasan, T., Muthukumar, K., Kavitake, D., and Shetty, P. H. (2017). Synthesis of silver nanoparticles using bacterial exopolysaccharide and its application for degradation of azo-dyes. Biotechnol. Rep. 15, 33–40. doi:10.1016/j.btre.2017.02.006
Sarkar, D. J., Kumar, J., Shakil, N., and Walia, S. (2012). Release kinetics of controlled release formulations of thiamethoxam employing nano-ranged amphiphilic PEG and diacid based block polymers in soil. J. Environ. Sci. Health Part A 47, 1701–1712. doi:10.1080/10934529.2012.687294
Sathiyanarayanan, G., Dineshkumar, K., and Yang, Y.-H. (2017). Microbial exopolysaccharide-mediated synthesis and stabilization of metal nanoparticles. Crit. Rev. Microbiol. 43, 731–752. doi:10.1080/1040841X.2017.1306689
Schäfer, R. B., Liess, M., Altenburger, R., Filser, J., Hollert, H., Roß-Nickoll, M., et al. (2019). Future pesticide risk assessment: Narrowing the gap between intention and reality. Environ. Sci. Eur. 31, 21. doi:10.1186/s12302-019-0203-3
Selvaraj, P., Christina, A. P., and Princy Rathnamala, J. (2019). Insecticidal and antibacterial potential of Syzygium aromaticum (L.) merrill and perry. J. Biopestic. 12, 191–196.
Senapati, S., Ahmad, A., Khan, M. I., Sastry, M., and Kumar, R. (2005). Extracellular biosynthesis of bimetallic Au–Ag alloy nanoparticles. Small 1, 517–520. doi:10.1002/smll.200400053
Shahzadi, K., Bashir, F., Nazir, K., Seemab, S., Afsar, M., Shaker, A., et al. (2019). Impacts of green synthesized silver-nanoparticles against Tribolium castaneum (Coleoptera: Tenebrionidae).
Shakiba, S., Astete, E., Paudel, S., Sabliov, M., Rodrigues, C., F., and Louie, D., M. S. (2020). Emerging investigator series: Polymeric nanocarriers for agricultural applications: Synthesis, characterization, and environmental and biological interactions. Environ. Sci. Nano 7, 37–67. doi:10.1039/C9EN01127G
Shakil, N. A., Singh, M. K., Pandey, A., Kumar, J., PankajParmar, V. S., Singh, M. K., et al. (2010). Development of poly(ethylene glycol) based amphiphilic copolymers for controlled release delivery of carbofuran. J. Macromol. Sci. Part A 47, 241–247. doi:10.1080/10601320903527038
Shang, Q., Shi, Y., Zhang, Y., Zheng, T., and Shi, H. (2013). Pesticide-conjugated polyacrylate nanoparticles: Novel opportunities for improving the photostability of emamectin benzoate. Polym. Adv. Technol. 24, 137–143. doi:10.1002/pat.3060
Shang, Y., Hasan, M. K., Ahammed, G. J., Li, M., Yin, H., and Zhou, J. (2019). Applications of nanotechnology in plant growth and crop protection: A review. Molecules 24, 2558. doi:10.3390/molecules24142558
Sharma, S., Singh, S., Ganguli, A. K., and Shanmugam, V. (2017). Anti-drift nano-stickers made of graphene oxide for targeted pesticide delivery and crop pest control. Carbon 115, 781–790. doi:10.1016/j.carbon.2017.01.075
Sharma, A., Sood, K., Kaur, J., and Khatri, M. (2019). Agrochemical loaded biocompatible chitosan nanoparticles for insect pest management. Biocatal. Agric. Biotechnol. 18, 101079. doi:10.1016/j.bcab.2019.101079
Sharma, S., Sahu, B. K., Cao, L., Bindra, P., Kaur, K., Chandel, M., et al. (2021a). Porous nanomaterials: Main vein of agricultural nanotechnology. Prog. Mater. Sci. 121, 100812. doi:10.1016/j.pmatsci.2021.100812
Sharma, S., Singh, B., Bindra, P., Panneerselvam, P., Dwivedi, N., Senapati, A., et al. (2021b). Triple-smart eco-friendly chili anthracnose control agro-nanocarrier. ACS Appl. Mat. Interfaces 13, 9143–9155. doi:10.1021/acsami.0c18797
Shekhar, S., Sharma, S., Kumar, A., Taneja, A., and Sharma, B. (2021). The framework of nanopesticides: A paradigm in biodiversity. Mat. Adv. 2, 6569–6588. doi:10.1039/D1MA00329A
Shiri, S., Abbasi, N., Alizadeh, K., and Karimi, E. (2019). Novel and green synthesis of a nanopolymer and its use as a drug delivery system of silibinin and silymarin extracts in the olfactory ensheathing cells of rats in normal and high-glucose conditions. RSC Adv. 9, 38912–38927. doi:10.1039/C9RA05608D
Shivaji, S., Madhu, S., and Singh, S. (2011). Extracellular synthesis of antibacterial silver nanoparticles using psychrophilic bacteria. Process Biochem. 46, 1800–1807. doi:10.1016/j.procbio.2011.06.008
Siddique, M. A., Hasan, M. U., Sagheer, M., and Sahi, S. T. (2022). Comparative toxic effects of Eucalyptus globulus L. (Myrtales: Myrtaceae) and its green synthesized zinc oxide nanoparticles (ZnONPs) against Rhyzopertha Dominica (F.) (Coleoptera: Bostrichidae). Int. J. Trop. Insect Sci. 42, 1697–1706. doi:10.1007/s42690-021-00691-5
Singh, A., and Leppanen, C. (2020). Known target and nontarget effects of the novel neonicotinoid cycloxaprid to arthropods: A systematic review. Integr. Environ. Assess. Manag. 16, 831–840. doi:10.1002/ieam.4305
Singh, J., Dutta, T., Kim, K.-H., Rawat, M., Samddar, P., and Kumar, P. (2018). ‘Green’ synthesis of metals and their oxide nanoparticles: Applications for environmental remediation. J. Nanobiotechnology 16, 84. doi:10.1186/s12951-018-0408-4
Singh, S., Kumar, S., and Correspondence, W. (2017). Phytochemical Screening and antimicrobial study of Euphorbia hirta extracts. J. Med. Plants Stud. 5, 183–186.
Skjolding, L. M., Winther-Nielsen, M., and Baun, A. (2014). Trophic transfer of differently functionalized zinc oxide nanoparticles from crustaceans (Daphnia magna) to zebrafish (Danio rerio). Aquat. Toxicol. 157, 101–108. doi:10.1016/j.aquatox.2014.10.005
Solgi, M., and Taghizadeh, M. (2020). “Biogenic synthesis of metal nanoparticles by plants,” in Biogenic nano-particles and their use in agro-ecosystems. Editors M. Ghorbanpour, P. Bhargava, A. Varma, and D. K. Choudhary (Singapore: Springer), 593–606.
Song, S., Liu, X., Jiang, J., Qian, Y., Zhang, N., and Wu, Q. (2009). Stability of triazophos in self-nanoemulsifying pesticide delivery system. Colloids Surfaces A Physicochem. Eng. Aspects 350, 57–62. doi:10.1016/j.colsurfa.2009.08.034
Sooresh, A., Kwon, H., Taylor, R., Pietrantonio, P., Pine, M., and Sayes, C. M. (2011). Surface functionalization of silver nanoparticles: Novel applications for insect vector control. ACS Appl. Mat. Interfaces 3, 3779–3787. doi:10.1021/am201167v
Sosan, A., Svistunenko, D., Straltsova, D., Tsiurkina, K., Smolich, I., Lawson, T., et al. (2016). Engineered silver nanoparticles are sensed at the plasma membrane and dramatically modify the physiology of Arabidopsis thaliana plants. Plant J. Cell Mol. Biol. 85, 245–257. doi:10.1111/tpj.13105
Southam, G., and Beveridge, T. J. (1994). The in vitro formation of placer gold by bacteria. Geochim. Cosmochim. Acta 58, 4527–4530. doi:10.1016/0016-7037(94)90355-7
Sparks, M. E., Bansal, R., Benoit, J. B., Blackburn, M. B., Chao, H., Chen, M., et al. (2020). Brown marmorated stink bug, halyomorpha halys (stål), genome: Putative underpinnings of polyphagy, insecticide resistance potential and biology of a top worldwide pest. BMC Genomics 21, 227. doi:10.1186/s12864-020-6510-7
Sparks, T. C. (2013). Insecticide discovery: An evaluation and analysis. Pestic. Biochem. Physiol. 107, 8–17. doi:10.1016/j.pestbp.2013.05.012
Stankovic, S., Kostic, M., Kostic, I., and Krnjajic, S. (2020). “Practical approaches to pest control,” in Pests, weeds and diseases in agricultural crop and animal husbandry production. Editors D. Kontogiannatos, A. Kourti, and K. F. Mendes (London: IntechOpen).
Stanley, S. (2014). Biological nanoparticles and their influence on organisms. Curr. Opin. Biotechnol. Nanobiotechnology • Syst. Biol. 28, 69–74. doi:10.1016/j.copbio.2013.11.014
Suganya, P., Vaseeharan, B., Vijayakumar, S., Balan, B., Govindarajan, M., Alharbi, N. S., et al. (2017). Biopolymer zein-coated gold nanoparticles: Synthesis, antibacterial potential, toxicity and histopathological effects against the Zika virus vector Aedes aegypti. J. Photochem. Photobiol. B Biol. 173, 404–411. doi:10.1016/j.jphotobiol.2017.06.004
Sugumar, S., Clarke, S. K., Nirmala, M. J., Tyagi, B. K., Mukherjee, A., and Chandrasekaran, N. (2014). Nanoemulsion of eucalyptus oil and its larvicidal activity against Culex quinquefasciatus. Bull. Entomol. Res. 104, 393–402. doi:10.1017/S0007485313000710
Sukhanova, A., Bozrova, S., Sokolov, P., Berestovoy, M., Karaulov, A., and Nabiev, I. (2018). Dependence of nanoparticle toxicity on their physical and chemical properties. Nanoscale Res. Lett. 13, 44–21. doi:10.1186/s11671-018-2457-x
Sun, C., Shu, K., Wang, W., Ye, Z., Liu, T., Gao, Y., et al. (2014). Encapsulation and controlled release of hydrophilic pesticide in shell cross-linked nanocapsules containing aqueous core. Int. J. Pharm. 463, 108–114. doi:10.1016/j.ijpharm.2013.12.050
Sun, C., Yu, M., Zeng, Z., Francis, F., Cui, H., and Verheggen, F. (2020). Biocidal activity of polylactic acid-based nano-formulated abamectin on Acyrthosiphon pisum (Hemiptera: Aphididae) and the aphid predator Adalia bipunctata (Coleoptera: Coccinellidae). PLOS ONE 15, e0228817. doi:10.1371/journal.pone.0228817
Sundararajan, B., and Ranjitha Kumari, B. D. (2017). Novel synthesis of gold nanoparticles using Artemisia vulgaris L. leaf extract and their efficacy of larvicidal activity against dengue fever vector Aedes aegypti L. J. Trace Elem. Med. Biol. 43, 187–196. doi:10.1016/j.jtemb.2017.03.008
Sunkar, S., and Nachiyar, C. V. (2012). Biogenesis of antibacterial silver nanoparticles using the endophytic bacterium Bacillus cereus isolated from Garcinia xanthochymus. Asian pac. J. Trop. Biomed. 2, 953–959. doi:10.1016/S2221-1691(13)60006-4
Suresh Kumar, R. S., Shiny, P. J., Anjali, C. H., Jerobin, J., Goshen, K. M., Magdassi, S., et al. (2013). Distinctive effects of nano-sized permethrin in the environment. Environ. Sci. Pollut. Res. 20, 2593–2602. doi:10.1007/s11356-012-1161-0
Swilam, N., and Nematallah, K. A. (2020). Polyphenols profile of pomegranate leaves and their role in green synthesis of silver nanoparticles. Sci. Rep. 10, 14851. doi:10.1038/s41598-020-71847-5
Szekalska, M., Puciłowska, A., Szymańska, E., Ciosek, P., and Winnicka, K. (2016). Alginate: Current use and future perspectives in pharmaceutical and biomedical applications. Int. J. Polym. Sci. 2016, 76970311–e7697117. doi:10.1155/2016/7697031
Tangaa, S. R., Selck, H., Winther-Nielsen, M., and Khan, F. R. (2016). Trophic transfer of metal-based nanoparticles in aquatic environments: A review and recommendations for future research focus. Environ. Sci. Nano 3, 966–981. doi:10.1039/c5en00280j
Teng, H., Yuan, Y., Zhang, T., Chang, X., and Wang, D. (2020). Evaluation of the sublethal effect of tetrachlorantraniliprole on Spodoptera exigua and its potential toxicity to two non-target organisms. PLOS ONE 15, e0242052. doi:10.1371/journal.pone.0242052
Thairu, M. W., Skidmore, I. H., Bansal, R., Nováková, E., Hansen, T. E., Li-Byarlay, H., et al. (2017). Efficacy of RNA interference knockdown using aerosolized short interfering RNAs bound to nanoparticles in three diverse aphid species. Insect Mol. Biol. 26, 356–368. doi:10.1111/imb.12301
Thakkar, K. N., Mhatre, S. S., and Parikh, R. Y. (2010). Biological synthesis of metallic nanoparticles. Nanomedicine Nanotechnol. Biol. Med. 6, 257–262. doi:10.1016/j.nano.2009.07.002
Thakur, P., Thakur, S., Kumari, P., Shandilya, M., Sharma, S., Poczai, P., et al. (2022). Nano-insecticide: Synthesis, characterization, and evaluation of insecticidal activity of ZnO NPs against Spodoptera litura and Macrosiphum euphorbiae. Appl. Nanosci. 12, 3835–3850. doi:10.1007/s13204-022-02530-6
Tippayawat, P., Phromviyo, N., Boueroy, P., and Chompoosor, A. (2016). Green synthesis of silver nanoparticles in aloe vera plant extract prepared by a hydrothermal method and their synergistic antibacterial activity. PeerJ 4, e2589. doi:10.7717/peerj.2589
Vašíčková, J., Hvězdová, M., Kosubová, P., and Hofman, J. (2019). Ecological risk assessment of pesticide residues in arable soils of the Czech Republic. Chemosphere 216, 479–487. doi:10.1016/j.chemosphere.2018.10.158
Vineela, V., Nataraj, T., Reddy, G., and Devi, P. S. V. (2017). Enhanced bioefficacy of Bacillus thuringiensis var. kurstaki against Spodoptera litura (Lepidoptera: Noctuidae) through particle size reduction and formulation as a suspension concentrate. Biocontrol Sci. Technol. 27, 58–69. doi:10.1080/09583157.2016.1247433
Walker, G. W., Kookana, R. S., Smith, N. E., Kah, M., Doolette, C. L., Reeves, P. T., et al. (2018). Ecological risk assessment of nano-enabled pesticides: A perspective on problem formulation. J. Agric. Food Chem. 66, 6480–6486. doi:10.1021/acs.jafc.7b02373
Wan-Jun, S., Wei-Wei, S., Sai-Yan, G., Yi-Tong, L., Yong-Song, C., and Pei, Z. (2010). Effects of nanopesticide chlorfenapyr on mice. Toxicol. Env. Chem. 92, 1901–1907. doi:10.1080/02772248.2010.483758
Wang, C., Cui, B., Zhao, X., Wang, Y., Zeng, Z., Sun, C., et al. (2019). Optimization and characterization of lambda-cyhalothrin solid nanodispersion by self-dispersing method. Pest Manag. Sci. 75, 380–389. doi:10.1002/ps.5122
Wang, G., Xiao, Y., Xu, H., Hu, P., Liang, W., Xie, L., et al. (2018). Development of multifunctional avermectin poly(succinimide) nanoparticles to improve bioactivity and transportation in rice. J. Agric. Food Chem. 66, 11244–11253. doi:10.1021/acs.jafc.8b03295
Wang, J. J., Zeng, Z. W., Xiao, R. Z., Xie, T., Zhou, G. L., Zhan, X. R., et al. (2011). Recent advances of chitosan nanoparticles as drug carriers. Int. J. Nanomedicine 6, 765–774. doi:10.2147/IJN.S17296
Wang, L., Li, X., Zhang, G., Dong, J., and Eastoe, J. (2007). Oil-in-water nanoemulsions for pesticide formulations. J. Colloid Interface Sci. 314, 230–235. doi:10.1016/j.jcis.2007.04.079
Wang, X., Cai, A., Wen, X., Jing, D., Qi, H., and Yuan, H. (2017). Graphene oxide-Fe3O4 nanocomposites as high-performance antifungal agents against Plasmopara viticola. Sci. China Mat. 60, 258–268. doi:10.1007/s40843-016-9005-9
Wang, Y., Cui, H., Sun, C., Zhao, X., and Cui, B. (2014). Construction and evaluation of controlled-release delivery system of Abamectin using porous silica nanoparticles as carriers. Nanoscale Res. Lett. 9, 655. doi:10.1186/1556-276X-9-655
Wang, Y., Jiang, F., Ma, C., Rui, Y., Tsang, D. C. W., and Xing, B. (2019). Effect of metal oxide nanoparticles on amino acids in wheat grains (Triticum aestivum) in a life cycle study. J. Environ. Manage. 241, 319–327. doi:10.1016/j.jenvman.2019.04.041
Wei, D., and Qian, W. (2008). Facile synthesis of Ag and Au nanoparticles utilizing chitosan as a mediator agent. Colloids Surf. B Biointerfaces 62, 136–142. doi:10.1016/j.colsurfb.2007.09.030
Werdin González, J. O., Gutiérrez, M. M., Ferrero, A. A., and Fernandez Band, B. (2014). Essential oils nanoformulations for stored-product pest control – characterization and biological properties. Chemosphere 100, 130–138. doi:10.1016/j.chemosphere.2013.11.056
Wheeler, D. A., and Isman, M. B. (2001). Antifeedant and toxic activity of Trichilia americana extract against the larvae of Spodoptera litura. Entomol. Exp. Appl. 98, 9–16. doi:10.1046/j.1570-7458.2001.00751.x
Xiang, Y., Zhang, G., Chen, C., Liu, B., Cai, D., and Wu, Z. (2018). Fabrication of a pH-responsively controlled-release pesticide using an attapulgite-based hydrogel. ACS Sustain. Chem. Eng. 6, 1192–1201. doi:10.1021/acssuschemeng.7b03469
Xiao, B., Zhang, Y., Wang, X., Chen, M., Sun, B., Zhang, T., et al. (2019). Occurrence and trophic transfer of nanoparticulate Ag and Ti in the natural aquatic food web of Taihu Lake, China. Environ. Sci. Nano 6, 3431–3441. doi:10.1039/C9EN00797K
Xu, Z., Gao, Z., and Shao, X. (2018). Light-triggered release of insecticidally active spirotetramat-enol. Chin. Chem. Lett. 29, 1648–1650. doi:10.1016/j.cclet.2018.01.025
Yan, S., Ren, B., Zeng, B., and Shen, J. (2020). Improving RNAi efficiency for pest control in crop species. BioTechniques 68, 283–290. doi:10.2144/btn-2019-0171
Yang, F.-L., Li, X.-G., Zhu, F., and Lei, C.-L. (2009). Structural characterization of nanoparticles loaded with garlic essential oil and their insecticidal activity against Tribolium castaneum (herbst) (Coleoptera: Tenebrionidae). J. Agric. Food Chem. 57, 10156–10162. doi:10.1021/jf9023118
Yin, Y., Xu, S., Chang, D., Zheng, H., Li, J., Liu, X., et al. (2010). One-pot synthesis of biopolymeric hollow nanospheres by photocrosslinking. Chem. Commun. 46, 8222–8224. doi:10.1039/C0CC03129A
Yu, M., Sun, C., Xue, Y., Liu, C., Qiu, D., Cui, B., et al. (2019). Tannic acid-based nanopesticides coating with highly improved foliage adhesion to enhance foliar retention. RSC Adv. 9, 27096–27104. doi:10.1039/C9RA05843E
Zhang, H., Wang, D., Butler, R., Campbell, N. L., Long, J., Tan, B., et al. (2008). Formation and enhanced biocidal activity of water-dispersable organic nanoparticles. Nat. Nanotechnol. 3, 506–511. doi:10.1038/nnano.2008.188
Zhang, J., Li, M., Fan, T., Xu, Q., Wu, Y., Chen, C., et al. (2013). Construction of novel amphiphilic chitosan copolymer nanoparticles for chlorpyrifos delivery. J. Polym. Res. 20, 107. doi:10.1007/s10965-013-0107-7
Zhang, X., He, X., Wang, K., and Yang, X. (2011). Different active biomolecules involved in biosynthesis of gold nanoparticles by three fungus species. J. Biomed. Nanotechnol. 7, 245–254. doi:10.1166/jbn.2011.1285
Zhang, X., Zhang, J., and Zhu, K. Y. (2010). Chitosan/double-stranded RNA nanoparticle-mediated RNA interference to silence chitin synthase genes through larval feeding in the African malaria mosquito (Anopheles gambiae). Insect Mol. Biol. 19, 683–693. doi:10.1111/j.1365-2583.2010.01029.x
Zhang, Y., Chen, W., Jing, M., Liu, S., Feng, J., Wu, H., et al. (2019). Self-assembled mixed micelle loaded with natural pyrethrins as an intelligent nano-insecticide with a novel temperature-responsive release mode. Chem. Eng. J. 361, 1381–1391. doi:10.1016/j.cej.2018.10.132
Zhao, L., Huang, Y., Adeleye, A. S., and Keller, A. A. (2017). Metabolomics reveals Cu(OH)2 nanopesticide-activated anti-oxidative pathways and decreased beneficial antioxidants in spinach leaves. Environ. Sci. Technol. 51, 10184–10194. doi:10.1021/acs.est.7b02163
Zhao, L., Ortiz, C., Adeleye, A. S., Hu, Q., Zhou, H., Huang, Y., et al. (2016). Metabolomics to detect response of lettuce (Lactuca sativa) to Cu(OH)2 nanopesticides: Oxidative stress response and detoxification mechanisms. Environ. Sci. Technol. 50, 9697–9707. doi:10.1021/acs.est.6b02763
Zheng, Y., Hu, Y., Yan, S., Zhou, H., Song, D., Yin, M., et al. (2019). A polymer/detergent formulation improves dsRNA penetration through the body wall and RNAi-induced mortality in the soybean aphid Aphis glycines. Pest Manag. Sci. 75, 1993–1999. doi:10.1002/ps.5313
Keywords: organic nanopesticide, sustainable agriculture, future prospects, pest management, insect pest control, nanoparticles
Citation: Manna S, Roy S, Dolai A, Ravula AR, Perumal V and Das A (2023) Current and future prospects of “all-organic” nanoinsecticides for agricultural insect pest management. Front. Nanotechnol. 4:1082128. doi: 10.3389/fnano.2022.1082128
Received: 27 October 2022; Accepted: 14 December 2022;
Published: 09 January 2023.
Edited by:
Gaurav Srivastava, Indian Institute of Technology Kanpur, IndiaReviewed by:
Montcharles da Silva Pontes, Federal University of Mato Grosso do Sul (UFMS), BrazilVijaya Kumar Shanmugam, Institute of Nano Science and Technology (INST), India
Copyright © 2023 Manna, Roy, Dolai, Ravula, Perumal and Das. This is an open-access article distributed under the terms of the Creative Commons Attribution License (CC BY). The use, distribution or reproduction in other forums is permitted, provided the original author(s) and the copyright owner(s) are credited and that the original publication in this journal is cited, in accordance with accepted academic practice. No use, distribution or reproduction is permitted which does not comply with these terms.
*Correspondence: Amlan Das, dasamlan@yahoo.co.in