- Centre for Nanobiotechnology, Vellore Institute of Technology, Vellore, Tamil Nadu, India
COVID-19 is one of the serious catastrophes that have a substantial influence on human health and the environment. Diverse preventive actions were implemented globally to limit its spread and transmission. Personnel protective equipment (PPE) was an important part of these control approaches. But unfortunately, these types of PPE mainly comprise plastics, which sparked challenges in the management of plastic waste. Disposable face masks (DFM) are one of the efficient strategies used across the world to ward off disease transmission. DFMs can contribute to micro and nano plastic pollution as the plastic present in the mask may degrade when exposed to certain environmental conditions. Microplastics (MPs) can enter the food chain and devastate human health. Recognizing the possible environmental risks associated with the inappropriate disposal of masks, it is crucial to avert it from becoming the next plastic crisis. To address this environmental threat, titanium dioxide (TiO2)-based photocatalytic degradation (PCD) of MPs is one of the promising approaches. TiO2-based photocatalysts exhibit excellent plastic degradation potential due to their outstanding photocatalytic ability, cost efficiency, chemical, and thermal stability. In this review, we have discussed the reports on COVID-19 waste generation, the limitation of current waste management techniques, and the environmental impact of MPs leachates from DFMs. Mainly, the prominence of TiO2 in the PCD and the applications of TiO2-based photocatalysts in MPs degradation are the prime highlights of this review. Additionally, various synthesis methods to enhance the photocatalytic performance of TiO2 and the mechanism of PCD are also discussed. Furthermore, current challenges and the future research perspective on the improvement of this approach have been proposed.
1 Introduction
Personnel protective equipment (PPE) plays a crucial role in safeguarding individuals during COVID-19 (Benson et al., 2021). PPE comprises disposable face masks (DFMs), gloves, goggles, gowns, face shields, respirators, and aprons made largely of single-use plastics, according to WHO (2020). Wearing DFM is one of the effective strategies against COVID-19 (Vieten, 2020; Du et al., 2022a). These face masks mainly comprise three layers: an inner layer of soft fibers, a middle layer of a melt-blown filter, and an exterior covering of nonwoven fibers that ensures water resistance (Fadare and Okoffo, 2020). Polypropylene (PP), polystyrene (PS), polycarbonate (PC), polyurethane, polyethylene (PE), and polyacrylonitrile (PAN) are the most common polymers that are used in face masks (Akber Abbasi et al., 2020).
Since the commencement of the pandemic, the quantity of litter produced by the COVID-19 outbreak such as DFMs, and other PPE has increased dramatically. Especially, this outbreak has resulted in an astounding increase in the usage of DFMs to prevent disease transmission (Sullivan et al., 2021). The handling of wastes generated by masks is a troublesome reflection of the COVID-19 outbreak, which has devastated worldwide healthcare systems and affected national economies (Herron et al., 2020; Patrício Silva et al., 2020). In early 2020, WHO anticipated that 89 million DFMs will be needed per month for health workers (de Sousa, 2021). China’s daily manufacturing of DFM has increased to 14.8 million during (February) 2020. According to US officials, 3.5 billion masks will be needed by their nation to combat this pandemic (de Sousa, 2021). It was predicted that more than 7 billion masks would be used every day throughout the world (Hantoko et al., 2021). Moreover, it was anticipated that by 2020, 1.56 billion (5,159–6,878 tons) of plastics (only from COVID-19 DFM) might leach into oceans (Shams et al., 2021). In the United Kingdom (66.7 million residents), it was estimated that if every resident wore one mask every day, a minimum of 60,000 tons of plastic trash would be generated (Benson et al., 2021). In Brazil, it is estimated over 85 Million used masks might be discarded each day (Urban and Nakada, 2021). It is projected that 0.15 million–0.39 million tons of mishandled COVID-19 plastic trash might wind up in the global oceans within a year (Chowdhury et al., 2021; Patrício Silva et al., 2021). Even if only 1% of disposable face masks were discarded improperly by the global population, it would result in the release of 10 million (30,000–40,000 kg) masks into the environment (WWF International, 2020). There have been numerous reports of used DFMs cluttering city streets, flowing through sewage lines, and floating in seas right from the onset of the COVID-19 outbreak (Ardusso et al., 2021; Okuku et al., 2021; Torres and De-la-Torre, 2021). The release of microplastics from face masks was reported in the recent literature (Saliu et al., 2021; Sullivan et al., 2021; Wang et al., 2021b). Physiochemical processes such as UV radiation, wind, currents, and other biochemical processes in the environment trigger the disintegration or degradation of these used masks into nanofibers and/or microplastics (MPs) (<5 mm) (Du et al., 2022a). Owing to the remarkable resistance of the plastics, they are inconceivable for complete mineralization and biological breakdown. Consequently, most plastics will remain in the environment for a longer period (Khoo et al., 2021) and endanger the wellness of the ecosystem (Kane et al., 2020; Benson et al., 2021). As the size of the plastics reduces, they are more likely to be ingested and accumulated by the organisms which subsequently creates a high chance of entry into the food chain. Recent studies have demonstrated that certain MPs and nanoplastics can be absorbed by the stomach and passes across the blood-brain barrier, causing neurotoxic injury (Prust et al., 2020). This enormous increase in DFM waste and other PPE may add to the avalanche of plastic pollution (Benson et al., 2021). Particulate matter (PM) is one of the influencing factors which have contributed to the increased spread of COVID-19. PM could generate a condition suitable for spreading the virus over larger distances than those envisaged for intimate contact (Comunian et al., 2020). Apart from the usage of DFMs to combat COVID-19, it is also used to prevent air pollution (Morgana et al., 2021; Chaudhary et al., 2022). This resulted in increased mask waste generation, which makes it crucial to explore all potential environmental consequences. Photocatalysis is one of the remarkable measures for the degradation of MPs leachates that are released from DFMs. This approach is reliable and affordable as it utilizes sunlight. It is a promising approach mainly due to its low cost and great efficiency when compared to other approaches (Mandade, 2021). The underpinnings of photocatalytic degradation (PCD) are photocatalysts with strong redox potential. Titanium dioxide (TiO2) is considered the quintessential photocatalyst because of its unique qualities such as biocompatibility, high stability, availability, low operating temperature, and low cost (Turkten and Bekbolet, 2020). This approach has the potential for the degradation of various organic pollutants including microplastic leachates from DFMs.
2 COVID-19 and the untenable waste management
Plastic pollution has increased as a result of poor plastic waste management (PWM) during the COVID-19 outbreak throughout the world (Patrício Silva et al., 2021). Though PPE may be a lifesaver during COVID-19, the accumulation, mishandling, and dumping of these PPE wastes resulted in a sudden collapse of waste management chains. This leads to catastrophic plastic pollution in the ecosystem. The COVID-19 pandemic has enhanced the complexity of PWM and appears to be impeding the attempts to eliminate plastic pollution.
During COVID-19, there was an unconvincing increase in global medical waste from 200 tons/day to 29,000 tons/day in a short period (February 2020–September 2020). During COVID-19 tests, kits including plastic items such as tips, pipettes, falcon tubes, 96 well plates, Eppendorf tubes, and optical plastics plates were utilized. As a consequence of this increased usage, it was predicted that about 37 g of plastic debris will be left in the environment for each test (Celis et al., 2021). Medical waste surged from 45 to 247 tons (about 6 times higher when compared to pre-COVID) in China; a 30%–50% increase in the most afflicted districts of France; and a 30% surge in the Netherlands (de Sousa, 2021). In China, the Ministry of Ecology and Environment reported a 23% increase in the quantity of biomedical waste produced during the initial phase of the COVID-19 outbreak (Parashar and Hait, 2021).
The increase in DFM wastes is viewed as a new source of pollution that is intimately linked to the COVID-19 outbreak (Sullivan et al., 2021). Even in less populous nations like Ireland (almost 4.5 million people), health personnel utilized 9 million masks every week (Liang et al., 2021). The disposal of used DFMs appears to be substantially greater on rivers and beaches. Beaches in Kenya reported ten times more dumping of used masks than streets (Okuku et al., 2021). It was estimated that around 250 DFMs may enter aquatic systems each day in Jakarta, Indonesia (Cordova et al., 2021). Used masks have been littered in metropolitan areas, with concentrations of about 0.001 items per square meter in Peru and Canada, and, it was less than 0.3 items per square meter in Kenya (Ammendolia et al., 2021; De-la-Torre et al., 2021). The use of DFM surged in most of the developing nations. Population and predicted mask usage rate (per day) in some developing nations as predicted by Badillo-Goicoechea et al. (2021) is depicted in Figure 1. This exorbitant usage of various PPE (especially DFM) and other medical-associated plastics has elevated the complication in managing COVID-19 wastes.
2.1 Limitations of current techniques
All over the world, widely employed approaches in plastic waste management are landfilling, incineration, and mechanical recycling (Alabi et al., 2019). The majority of plastic waste can be disposed of through incineration and landfills. The main issue is that it demands a huge space and energy (Anderson et al., 2021). The incineration of these biohazardous wastes will lead to air pollution, raising the particulate matter in the air and boosting the risk of COVID-19 infection and other respiratory issues (Torres-Agullo et al., 2021). During COVID-19, landfills were overloaded with plastic waste beyond their capacity. This increased landfill dumping might lead to various adverse effects including the leaching of adverse chemicals (Selvaranjan et al., 2021; Shams et al., 2021). The astounding generation of plastic waste combined with the lack of recycling measures during the lockdown resulted in a significant decline in global plastic recycling. The vast majority of plastics can be recycled and reused. Manpower is essential in the collection and sorting of plastics. But, one of the primary challenges with plastic recycling during COVID-19 was the lack of manpower as people were hesitant to collect COVID-19-based wastes (Anderson et al., 2021). The most difficult components of recycling are collecting and sorting plastic trash. Moreover, mechanical recycling is fraught with difficulties, such as inorganic pollutants, additives, and polymer cross-contamination (Anderson et al., 2021). Incineration can emit toxic gases such as dioxins and furans into the atmosphere, which paves way for global warming (Torres and De-la-Torre, 2021). The widely employed approaches in plastic disposal are depicted in Figure 2. In terms of MPs removal, wastewater treatment plants (WTP) are not satisfactory. They may easily be eluded from the collection by WTP due to their smaller size. These MPs may eventually result in the aquatic ecosystem. Studies on WTP from various sites revealed that an excessive quantity of MPs in the treated water ranges between 5.00 × 105 and 1.39 × 1010 particles (Hamd et al., 2022). As per the scientific reports, there is still no distinct approach in any WTP for the elimination of MPs. Ideally, these disadvantages make these processes not suitable for sustainable plastic waste degradation.
3 Environmental impact of microplastics generated from disposable face masks
The nature of deposition sites and composite materials determine the fate of the DFMs (Du et al., 2022a). Environmental factors trigger the transformation of used DFMs into MPs, which are considered as a new form of environmental pollutant. In most cases, used DFMs are often thrown randomly or segregated as plastic waste. These wastes are either incinerated or disposed of in landfills (Du et al., 2022a). Because of the inclusion of plastics in DFMs and its subsequent waste mismanagement, there are numerous negative environmental consequences. In general, terrestrial ecosystems are the primary sources of aquatic plastic pollution, which is mostly due to anthropogenic sources. About 80% of worldwide ocean plastics originate from land, with the remaining 20% ascribed to marine sources (LI et al., 2016). The impact of mask-generated MPs on terrestrial and aquatic ecosystems is depicted in Figure 3.
3.1 Terrestrial ecosystem
3.1.1 Impact on animals
Face masks that have been dumped in the soil can endanger fauna causing entanglement and even mortality (Hiemstra et al., 2021; Patricio Silva et al., 2021; Du et al., 2022a; Yang et al., 2022). Several incidents of animals becoming tangled in discarded facemasks have been documented across the world including entanglement in claws, snouts, necks, legs, and other parts of the body (Hiemstra et al., 2021; Patrício Silva et al., 2021). DFMs are also reported to be used by some birds as nesting material. This may alter the thermal conditions and increase the likelihood of ingestion or entanglement (Hiemstra et al., 2021). Reports suggest that hedgehogs, American robins, swans, bats, mallards, and gulls are at significant risk of being the victims of masks and other PPE entanglement (Yang et al., 2022). Most of the DFMs are disposed of as solid waste, and animals that rely on landfills for food may be particularly vulnerable to plastic uptake. For example, in Spain, Ciconia (white storks) were discovered to feed on landfill trash accounting for 68.8% of their diet (Peris, 2003). Moreover, when animals mistook face masks for food, these plastics can block their intestines, impede food intake, and cause a slew of health issues, including death (Du et al., 2022a). For example, a significant amount of landfill debris was also found in the guts of overwintering gull species like Larus marinus, L. glaucoides, and L. smithsonianus (Seif et al., 2018; Patrício Silva et al., 2021).
3.1.2 Impact on plants
Once dumped on the soil, plastic debris may clog sewage systems and leads to various detrimental effects on the soil. It impacts agricultural water percolation, soil aeration, and reduces soil quality (Prata et al., 2020). Wet-dry cycles, plowing, and other bio-factors, rapidly move MPs into the soil and spoil the soil structure, water-retaining nature, and bulk density of soil (Zhou et al., 2020; Du et al., 2022a). Furthermore, tillage processes transfer MPs and other pollutants from the upper layer of soil to the bottom, and leaching can transport MPs to groundwater. MPs in soil not only restrict the functional diversity of soil microbes, but also influence nutrient absorption by plants and indirectly impacts plant development (Du et al., 2022b). Plant roots were found to be able to adsorb MPs, and these MPs penetrate into the plants via a crack-entry mechanism at root tips. Subsequently, transport from the root system to other tissues may happen primarily through a transpiration pull force (Li et al., 2020). Researchers also found that nano-sized microbeads may enter tobacco cells via endocytosis, implying that smaller plastics can enter the plant through rhizosphere adsorption (Su et al., 2019). As a consequence, through the trophic chain, these MPs will end up in the human body. For instance, MPs have been identified in feces, indicating human intake, and also their occurrence has recently been identified inside the human placenta (Schwabl et al., 2019; Ragusa et al., 2021).
3.2 Impact on the aquatic system
The inappropriate waste management of DFMs is highly menacing to aquatic systems as the rate of decomposition of plastics is comparable to be high in the soil. Temperature differences between these two distinct thermal habitats, partial submergence, water absorption capability, and other properties of the DFMs hampered the degradation of DFMs in aquatic habitats (Du et al., 2022a). DFM and other PPEs are often made of polymers such as latex, nitrile, or polyethylene that require the inclusion of additives such as softeners and stabilizers to improve physical qualities. When PPE kits are widely disseminated across the environment, these additives can be hazardous, and there is a significant risk of leaching into water bodies (Ray et al., 2022). DFMs can be disintegrated into MPs by undergoing weathering, corrosion, and aquatic immersion in natural conditions. Saliu et al. (2021) estimated the release of microfibres from DFM into the marine ecosystem when exposed to UV radiation. According to the findings, one DFM was exposed to 180 h of UV irradiation and severe mixing in artificial saltwater may release up to 173,000 fibers per day (Saliu et al., 2021). They have also discovered a similar chemical and morphological deterioration pattern in surgical masks found on Italian beaches, indicating that comparable processes may occur in the natural sea (Patricio Silva et al., 2021; Saliu et al., 2021). Furthermore, the hydrophobic nature of MPs aids in adsorbing organic compounds, resulting in bacterial colonization and microalgae development (Ray et al., 2022). This biofouling may contribute to the sinking out of immense plastic objects and attribute to a surge in marine pollution. Researchers found that, when NaCl concentration increases, the hydrodynamic diameter (Dh) of particulate plastic also increases which in turn causes PS MPs to aggregate. As a result, PS MPs are predicted to get aggregated in seawater (Cai et al., 2018). Due to microbial interactions, biofilm development can affect water quality by altering the microbiome and accelerating the rate of organic material decomposition. This leads to a reduction in dissolved oxygen (DO) content (Kirstein et al., 2019).
MPs are ubiquitous throughout the aquatic system and are mistaken as feed by many aquatic organisms. Certain properties of MPs such as micro-size, appealing hues, and great buoyancy make these tiny particles easily accessible to fishes and other organisms (Ray et al., 2022). For instance, MPs associated with masks and PPE have been reported in over 20% of marine crustaceans (Jeong et al., 2016). MPs are reported to be consumed by marine mammals such as whales and dolphins (Dharmaraj et al., 2021). Researchers have found that plastics were identified in the guts of 56% of aquatic birds. As a consequence, it was predicted that by 2050 identical gastrointestinal issues are expected to infect 99.9% of birds (Aragaw, 2020). MPs of <1.5 μm in diameter can cause direct cell injury. Recent studies revealed that crabs intake MPs and it was found to get accumulated mostly in the hepatopancreas (Wang T. et al., 2021) and in 67% of shark samples, guts and digestive tracts showed a minimum of one MP (Parton et al., 2020). Aquatic megafauna and apex predators like whales, sharks, turtles, and mammals are at high risk of consuming whole masks (Fernandez and Anastasopoulou, 2019; Du et al., 2022a). MPs cannot be metabolized by organisms, and MPs containing biomolecules and aggregates can induce gastrointestinal or blockage issues. MPs can absorb water contaminants such as dye and hazardous compounds, which might be consumed by aquatic biota and impact the food chain (Binda et al., 2021). Plastic generated from DFM and other PPE can absorb organic and hazardous contaminants in aquatic systems, forming a hazardous film. This approach might harm aquatic biota that consumes plastic-based particles (Ray et al., 2022). These consumed MPs may influence reproduction, survival, and animal growth over time. Nanoplastics, together with genotoxins and oxidative stress have been found as a reservoir of neurotoxins in a wide range of aquatic biota, including corals (Chang et al., 2020). As a result of the bioaccumulation of these generated MPs in the primary food chain, human and animal health becomes intricate. For instance, a study on stool samples of adults and infants confirmed the presence of MPs. Surprisingly, MPs in infants are up to 20-fold higher than in adults (Zhang et al., 2021).
3.3 Impact on the atmosphere
The COVID-19 lockdown measures appear to minimize greenhouse gas emissions and enhance outdoor air quality. However, in the long run, the huge manufacturing of DFM and usage of other PPE causes a hidden problem of global greenhouse gas emissions. It was predicted that DFM has a greenhouse gas footprint of 0.059 kg carbon dioxide equivalents (CO2-eq) (includes transport), whereas fabric masks have a footprint of 0.036 kg CO2-eq/usage (includes rinsing) (Klemes et al., 2020). This implies that DFM use may have a tenfold greater impact on climate change than reusable masks (Yang et al., 2022). Globally, PP, PS, PVC, PE, and other airborne microplastics have been widely identified (Enyoh et al., 2019), which are the major components of DFMs. Airborne MPs flow through the atmosphere, accumulating in the air, water, and soil (Yang et al., 2022). The accumulation of MPs in the air expedites their threat of inhalation by humans. Furthermore, reports have also revealed that MPs in the DFM become a vital source of airborne MP pollution (Chen et al., 2021). Moreover, there is a high risk of MP inhalation, mainly when using low-quality DFM and repeated use of the same mask (Li et al., 2021).
4 Role of photocatalyst in the degradation of plastics
Photocatalysis is one of the important methods in the advanced oxidation process (AOP). As this method utilizes solar energy, it implies minimal cost and an eco-friendly approach (Du et al., 2021). The underpinnings of photocatalytic degradation (PCD) are photocatalysts with strong redox potential. Semiconductor materials such as TiO2, ZnO, WO3, g-C3N4, CdS, SnO2 ZrO2, BiVO4, and ZnS are employed as a photocatalysts in the PCD of organic contaminants (Lee and Li, 2021; Sharma et al., 2021). Once the absorbed energy of the photon (E) exceeds the semiconductor’s band gap energy, electrons (e−) in the valence band (VB) are transported to the conduction band (CB), and hence positive holes (h+) are created in the VB, resulting in the dissociation of electron-hole pairs. Both species (e− and h+) react with O2, H2O, or OH, to form highly reactive oxygen species (ROS) (Nakata and Fujishima, 2012; Du et al., 2021). Such active species eventually disintegrate the organic polymers, causing polymeric chain breakage and even total mineralization (Sharma et al., 2021).
4.1 Titanium dioxide as photocatalyst
TiO2 is an inevitable photocatalyst in plastic degradation. TiO2 has a less bandgap energy between its VB and CB. When exposed to UV radiation, an electron in the VB gets excited and transfers to the CB (Figure 4). Electrons can migrate to or from the adsorbent, resulting in positively or negatively charged species. Anatase TiO2 possesses a large bandgap of 3.2 eV, which relates to the excitation wavelength of 388 nm and enables it to absorb light in the UV range. Rutile TiO2 has a bandgap of 3.0 eV and absorbs visible light with a wavelength of 410 nm (Martinez and Hammer, 2011; Li, 2020; Nabi et al., 2021). For PCD, anatase and rutile forms of TiO2 are often used. Brookite forms are rarely utilized due to their unstable nature (Lee and Li, 2021). Iron oxide semiconductors are unstable because of their rapid photocathodic reactions and the resulting corrosive materials. Binary metal sulfide semiconductors like PbS, CdS, or CdSe are considered to be less stable due to their photoanodic corrosion nature and toxicity (Aziz et al., 2021). Though ZnO possesses a similar band gap to TiO2, it is not much stable towards the pH. This instability leads to the Zn(OH)2 precipitate formation on the surface of the particle, which results in the deactivation of the photocatalyst (Aziz et al., 2021). Furthermore, illumination of UV light upon ZnO leads to frequent photocorrosion (Barnes et al., 2013). In comparison with other photocatalysts, cost efficiency, mass production, doping, surface modifications, and well-established preparation processes are added advantages of TiO2 in PCD (Zhang et al., 2019; Turkten and Bekbolet, 2020; Aziz et al., 2021).
4.2 Synthesis of Titanium dioxide-based photocatalytic nanomaterial/composites
4.2.1 Sol-gel
One of the most important processes in TiO2 preparation is the sol-gel (also referred to as chemical solution deposition) method. The precursor and the reducer are the two main agents of the sol-gel method. (Li et al., 2010). Based on the type of titanium metal precursor, sol-gel synthesis of TiO2 is classified into two types: 1) Aqueous-based approach (starting precursor: inorganic metal salt) and 2) alcohol-based approach (starting precursor: metal oxide). In the aqueous-based method, TiCl4 and TiOSO4 are essential precursors. The hydrolysis and condensation process may also be used to generate aqueous-based sol-gels as depicted in Figure 5A. Precipitation and peptization are two stages in the aqueous-based sol-gel process (Ullattil and Periyat, 2017). In the alcohol-based approach Ti(OC4H9)4, Ti(OC3H7)4, and Ti(OC2H5) are the most prominent metal alkoxide precursors of TiO2. There is a metal-oxygen link in these alkoxides, and because of the notable variation in electro-negativity between Ti and O, the bond turns highly polar and incredibly reactive. As a result of the addition of water, parallel hydrolysis and condensation events occur eventually leading to the development of a gel (Ullattil and Periyat, 2017). It was reported that TTIP (precursor) and ethanol (reducing agent) were employed in sol-gel TiO2 preparation for the degradation of PE film. Researchers have also used Ti(OBu)4 as a precursor and ethanol as a reducing agent. This method was used in the preparation of polypyrrole/TiO2 nanocomposite for the degradation of PE (Thomas et al., 2013).
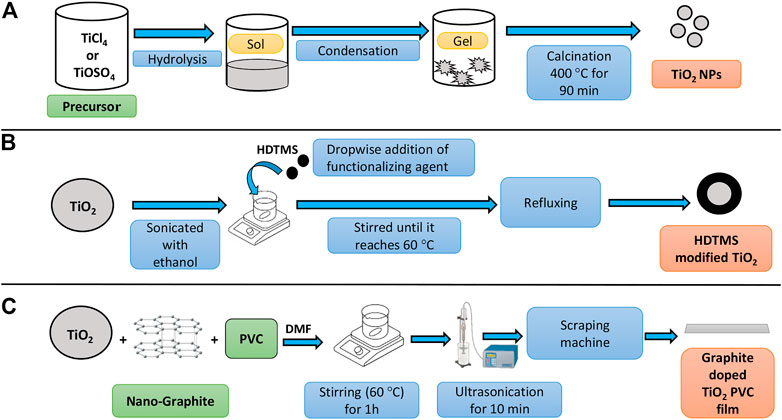
FIGURE 5. Flowchart illustration (A) Sol-gel synthesis of TiO2, (B) Surface modification of HDTMS TiO2 and (C) Graphite doped TiO2 PVC film.
4.2.2 Surface modification
TiO2 has been frequently modified to increase its efficiency and specificity. Modified TiO2 characteristics may vary from pure TiO2 in terms of charge separation, ease of TiO2 particle separation, light, and pollution adsorption. Numerous studies have been conducted on TiO2 modification, particularly for plastics deterioration. To prepare vitamin-C (VC) modified TiO2, VC was dissolved in tetrahydrofuran (THF). The mixture of n-TiO2 powder and THF solution were mixed and ultrasonicated. VC–THF solutions were mixed with n-TiO2 to obtain surface-modified n- TiO2 with VC (Ariza-Tarazona et al., 2019).
Protein-based modified TiO2 was synthesized using an extrapallial fluid of Mytilus edulis. This fluid acts as a pore-forming agent and N2 precursor. This solution was mixed with titanium (IV) butoxide followed by mineralization and thermal treatment. This method was employed in the degradation of HDPE microplastics (Ariza-Tarazona et al., 2019).
Natural ore-based TiO2 modifications were also reported. From leucoxene, ore rutile TiO2 was obtained and prepared through a ball-milling planetary approach. It was mixed (20:80) with (commercial) anatase with the help of a milling machine (Mekprasart et al., 2018). To synthesize surfactant-modified TiO2, P25 TiO2 was ground into fine powder. DI water and Triton X-100 (non-ionic surfactant) were added to the powder (dropwise manner) to obtain a paste. Ethanol was added to this paste (dropwise). This precursor solution was sonicated followed by string and used. This method aid in the degradation of PS microspheres and PE (Nabi et al., 2020).
Surface modification using a functionalizing agent is one of the important approaches in TiO2 modification. TiO2 NPs were added to ethanol and sonicated. Following the sonication, the dispersion was stirred until it reaches a favorable temperature (60°C). To this dispersion hexadecyltrimethoxysilane (HDTMS-functionalizing agent) was added (dropwise) as per the required degree of functionalization. Further, the temperature was enhanced followed by refluxing. Finally, the hexadecyltrimethoxysilane-modified TiO2 (resultant material) was rinsed and dried as depicted in Figure 5B (Alvarado et al., 2016).
Fe(St)3 modified TiO2 was prepared by the addition of TiO2 and ferric stearate to tetrahydrofuran. This combination was mixed with KH550 silicone by ultrasonication. This suspension was coated with PS to obtain the plastic-photocatalyst combination by stirring (12 h). The resulting material was placed on a glass stick and dried. This approach was employed in the degradation of PS (Fa et al., 2013).
4.2.3 Doping
TiO2 doping is a process carried out to enhance the reactivity of TiO2 and results in additional energy to the band structure that can have an effective role in the transmission of charges to the surface (Lee and Li, 2021). To fabricate Graphite doped (GrD) TiO2, PVC plastics were used. Graphite, TiO2, N, N dimethylformamide (DMF), and 2 g PVC were stirred (1 h; 60°C) followed by the ultrasonication of 10 min. Using the scraping instrument, the resulting material was uniformly spread onto a substrate. Finally, the film-generating plate was removed and soaked in DI water to obtain GrD TiO2 PVC film as depicted in Figure 5C (Peerakiatkhajohn et al., 2011; Nabi et al., 2021).
To enhance the activity of TiO2, transition metals were employed in doping. Titanium n-butoxide, ethanol, acetylacetone, nitric acid, and DI water were mixed and stirred at room temperature. Meanwhile, silver nitrate was produced by mixing AgNO3 with ethanol. The silver nitrate solution was then stirred into TiO2 and refluxed (8 h; 80°C) (Peerakiatkhajohn et al., 2011; Lee and Li, 2021).
TiO2 multi-walled carbon nanotubes (MWCNT) composites were also adopted in plastic degradation. MWCNT was treated with the acid vapor method. An MWCNT was calcined (450°C; 1 h) to eliminate the amorphous carbon. After thermal treatment, an MWCNT was put onto a silicon griddle in a Teflon (HNO3 was already present at the bottom). The resulting material was rinsed and dried. Then, titanium butoxide was mixed with ethanol and marked as A. Processed MW carbon was dissolved in ethanol through sonication, after the additament of acetic acid, with pH = 2-3, and termed B. Mixture “A” was added to “B” and mixed for about 15 min. This solution was stirred in a water bath (60°C) before being shifted to an autoclave and heated at 180°C. TiO2 particles develop over the CNT during thermal treatment, resulting in grey precipitates. Followed by the ethanol wash, the end product was dried (6 h at 90°C). This approach was employed in the degradation of PE (An et al., 2014).
4.3 Titanium dioxide assisted photocatalytic degradation of microplastics
The TiO2-embedment approach was used to degrade plastics such as PE, PP, PVC, PS, and PS polymers under diverse parameters (Fa et al., 2013; Nabi et al., 2020; Hamd et al., 2022). The PCD of PS plastics was carried out under UV irradiation using PS-TiO2 composite. Results revealed that better weight reduction (85%) was observed in PS-TiO2 composite than in pure PS film (65%). This was supported by the SEM results of PS-TiO2 samples as it has more voids compared to pure PS film. Doping also plays a crucial part in plastic degradation. Undoped and metal (Iron, silver, and Iron/silver-mix) doped TiO2 NPs were used to compare the PCD of PE films. The Fe/Ag doped TiO2 exhibited a maximum weight reduction of 14.43% (UV irradiation). As the irradiation time increases the rate of weight reduction also increased. SEM analysis revealed the formation of voids in the PE matrix. It is suggested that ROS generation on the surface of TiO2 is attributed to the degradation of the PE matrix (Figures 6A,B) (Asghar et al., 2011). Similarly, copper phthalocyanine-modified TiO2 showed better PE degradation than bare TiO2 (Zhao et al., 2008).
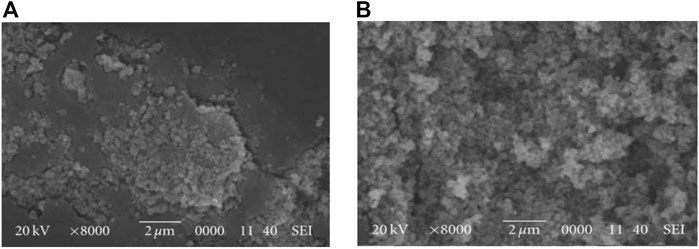
FIGURE 6. SEM images of (A) PE-TiO2 film after irradiation and (B) PE-Fe/Ag mix doped TiO2 film after irradiation (Reprinted from Asghar et al., 2011).
Reduced graphene oxide coated TiO2 (TiO2-rGO) is one of the important catalysts in the degradation of PP. This is mainly due to the Ti-O-C link, rGO extends TiO2’s absorption range to the visible area. Furthermore, 2D π-conjugation of rGO helps in the reduction of the recombination rate (Lee and Li, 2021). FT-IR indicates the presence of a carbonyl group with an increased carbonyl index, resulting in the more effective photooxidation of PP by TiO2-rGO nanocomposite than TiO2 NPs. Studies have revealed the PCD of PVC films under the air/nitrogen atmosphere. There was a 27% degradation in presence of air and no degradation was observed under a nitrogen atmosphere. This study implies the importance of O2 in photocatalysis (Cho and Choi, 2001). Applications of TiO2-based nanomaterials in PCD of MPs are depicted in Table 1.
4.3.1 Mechanism of photodegradation
When the photocatalyst absorbs UV light (with energy equal to or greater than its bandgap of TiO2), the electrons in VB get excited to CB. This forms holes in VB and subsequent electron-hole recombination (Eq. 1). Water interacts with the holes (present in VB) and generates hydroxyl radicals (•OH) (Eq. 2). The photocatalytic breakdown of plastics is significantly influenced by O2 molecules. Superoxide anions are generated by the interaction of O2 molecules (on the TiO2 surface) and free electrons (Eq. 3) (Ma et al., 2020). These superoxide anions also form •OH, which plays a key role in plastic degradation (Lee and Li, 2021). The formation of •OH can proceed in two ways, 1) The superoxide anion gets protonated to give hydrogen peroxide, which is subsequently dissociated by light energy to form •OH (Eq. 4A). 2) Superoxide anion combines with water to give HO2• and OH− . This OH− occupies holes to form •OH (Eq. 4B). This generated •OH degrades the plastics into CO2 and water. (Eqs. 5–10) (Chen et al., 2019; Lee and Li, 2021; Nabi et al., 2021). The mechanism of TiO2-based photocatalytic degradation of plastics is given in Figure 7.
4.3.2 Factors influencing the photocatalysis of plastics
The properties of photocatalysts and plastics play a prominent role in plastic degradation. Mainly, the type of photocatalyst and the physiochemical properties of the plastics are some of the critical factors in plastic degradation. As the size of the plastic decreases, the more will be the specific surface area. This leads to enhanced reactivity with O2 or photons, which enhances smaller (sized) plastic degradation than the larger ones (Sharma et al., 2021). TiO2-based nanomaterials play a vital role as a photocatalyst in the degradation of MPs. This is mostly owing to its high organic pollutant oxidation ability (Yuan et al., 2017). Furthermore, environmental factors like light, dampness, temperature, O2 concentration, and pH affect plastic deterioration.
4.3.2.1 Plastics and the photocatalyst used
The size of the plastic has a considerable influence on photodegradation efficiency. The lesser the structure complexity, the more will be the deterioration. (Song et al., 2017; Uheida et al., 2021). For instance, by using TiO2, researchers have studied the photodegradation of PE and PS. Due to its less complicated structure, the rate of degradation of PE was comparably high to that of PS (Zhao et al., 2008). Crystal type is also noted to be an influencing factor. The anatase phase in TiO2 (when doped with Mo and W) determines the PS degradation efficiency. Reports also suggest that doping Mn and Cr with TiO2 reduces the rate of degradation of PS film (Zhao et al., 2007; Ge et al., 2022). The quantity of photocatalysts used can affect the degradation efficiency of plastics. For instance, the rate of degradation of PE- TiO2 film increased as the concentration of TiO2 increased (Ge et al., 2022). The type of catalyst used is an important factor that influences plastic degradation. Ariza-Tarazona et al. (2019) used two distinct semiconductor photocatalysts relying on N-TiO2 to evaluate the breakdown of HDPE MPs obtained from a cosmetic scrub. The first catalyst was obtained from Mytilus edulis (green synthesis). The second catalyst was fabricated by utilizing urea and a tri-block copolymer. The photocatalytic technique proceeded under visible light for 20 h, and the protein-derived catalyst showed a strong capability to assist photodegradation in aqueous as well as solid media. The protein-derived catalyst degraded HDPE at a rate constant of 38.2 ± 3.7 (aqueous phase) and 12.2 ± 0.8 (solid phase). Mass loss was 6.4% in the aqueous phase and 1.1% in the solid phase (Ariza-Tarazona et al., 2019). Degradation of PE (photocatalytic) was studied between PE- TiO2 composite and pure PE (photolytic reaction). The degradation was higher in PE- TiO2 composite than pure PE. This shows the efficiency of Photocatalyst in the plastic degradation (Zhao et al., 2007).
4.3.2.2 Light
The light source can influence the result of plastic degradation to a great extent. Solar irradiation contributes inevitably to the degradation process. Mainly, IR rays (>700 nm have enhanced thermal oxidation, UV (<400 nm) direct photodegradation, and visible rays (400–700 nm) promote degradation through heat (Thomas and Sandhyarani, 2013). Under UV irradiation, most plastic particles/films degrade partially. This means that the active oxygen species produced (when exposed to visible light) are incapable of triggering chain cleavage and subsequent oxidation processes. To address this, researchers have used semiconductors as photocatalysts to improve photodegradation (Du et al., 2021). The degradation of PE in the presence of sunlight using polypyrrole (PPy)/TiO2 nanocomposite as a photocatalyst, which was synthesized using emulsion polymerization and sol-gel processes. It was noted that exposing the PE material to daylight for 240 h lowered its molecular weight up to 35.4% and 54.4% respectively (Li et al., 2010). The PCD of LDPE was achieved with the help of N- TiO2. The degradation was carried out under solar irradiation for 200 h. Results after the irradiation revealed a significant weight of 68% (Thomas and Sandhyarani, 2013).
UV radiation promotes photooxidative degradation followed by polymer breakage mediated by photons and the produced free radicals degrade the plastics (Verma et al., 2017). Studies have also revealed the significance of UV irradiation and the irradiation time in the degradation of plastics. Reports have revealed the importance of irradiation time in the PCD of PE films using undoped and metal (Fe, Ag) doped TiO2. As the irradiation time increased, the weight reduction percentage of PE films has also increased. The maximum weight reduction was observed at 300 h (Asghar et al., 2011). Figure 8 depicts the significance of irradiation time in PE film degradation.
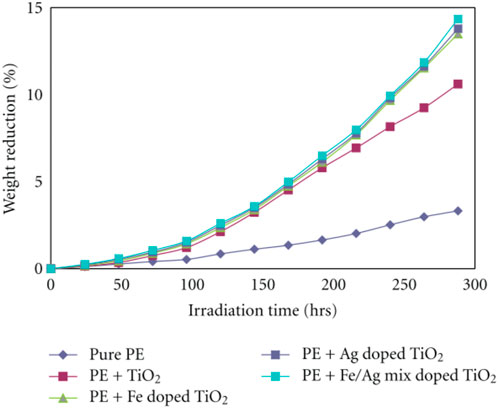
FIGURE 8. Effect of UV irradiation and irradiation time on the degradation of PE films (Reprinted from Asghar et al., 2011).
PE-goethite films were irradiated in UV light with two different intensities (2 mW/cm2 and 1 mW/cm2). The greater intensity leads to a better weight loss of 24% higher than the films treated with lesser intensity. This shift in light intensity enables changes in the electron-hole pair, such as the rate of recombination and separation on the photocatalyst surface (Li et al., 2010).
4.3.2.3 Other factors
The significance of humidity in PCD of PE was observed in a study. The degradation experiment was performed using n-TiO2 without a proper source of humidity (at room humidity). This condition leads to the termination of the reaction. Once the humidity in the ambient atmosphere was allowed to pass through the reaction chamber, the reaction proceeded (Ariza-Tarazona et al., 2019). Reports also suggest the importance of O2 in PCD. The rate of weight loss was examined between the PVC- TiO2 film in anoxic and oxic conditions. The quantity of weight loss was greater in the air, and the period of deterioration was similarly shorter. This is mainly due to the production of ROS due to the interaction of O2 with the CB electrons (Cho and Choi, 2001). The optimum pH of is one the important factor in the PCD. In the degradation of HDPE by C, N–TiO2, the interaction among MPs and colloidal NPS was promoted due to the lower pH. In addition, reduced temperature (0°C) (facilitates an increased surface area) also favors the degradation of HDPE (Ariza-Tarazona et al., 2020).
5 Challenges
Despite the numerous benefits of the photocatalytic degradation of MPs (plastics), there are still certain challenges that must be addressed. One of the important limitations is the specificity of photocatalysts i.e., photocatalysts are often suited for degrading only a particular type of plastic. Furthermore, photocatalysts cannot selectively target the plastic’s reactive sites (functional groups or defects) (Ge et al., 2022). In the environment, MPs plastics are found to be combined with other co-pollutants. Degradation of MPs along with the co-pollutants using PCD might be a challenging factor. Photocatalysis is a surface reaction, photodegradation may get impeded if light irradiation is hampered by non-transparent materials (contaminants). Additionally, certain TiO2-based photocatalysts function better in the UV band but not in visible light conditions. Owing to their versatility, TiO2-based photocatalysts are extensively employed in the photocatalytic degradation process. Reports suggest that the anatase form TiO2 is more toxic than the rutile form (Shabbir et al., 2021). This increased toxicity of the anatase form is attributed to the smaller particle size and the increased surface area (Shabbir et al., 2021). Studies have also revealed the toxicity of TiO2 toward the algae (primary producer) and its possibility to affect the (algae-crustacean) food chain (Bhuvaneshwari et al., 2018). Extensive investigations on the ecotoxicological assessment of TiO2 NPs are essential to analyze the toxicity of TiO2 NPs towards the environment. Additionally, analyzing the toxicity of the PCD intermediates would be a strenuous process.
6 Conclusion and future perspectives
Plastic pollution has become a worldwide issue due to its negative impact on human health and the environment. Furthermore, the COVID-19 pandemic has exacerbated this problem, owing to the widespread usage of PPE such as masks, gloves, and other plastic-based equipment. As these plastics get degraded into microplastics (under natural conditions), the chance of efficient mitigation is a conundrum. PCD could be a possible eco-friendly approach as it utilizes sunlight as an energy source.
Recent advancements in novel technologies and the production of innovative photocatalytic materials ameliorate plastic degradation. Future investigations on the toxicity of the intermediates that are released and the reusability of the photocatalyst are needed. In most of the PCD processes, CO2 will be generated as an end product. Even though it is less toxic than plastic waste, undoubtedly addressing these issues is necessary. Extensive investigations on the ecotoxicological assessment of TiO2 NPs are essential to analyze their toxicity towards the environment. To overcome these ecotoxicological issues, investigations on the usage of green nanomaterials as photocatalysts will be an eco-friendly approach towards plastic degradation. Green synthesis of TiO2 is a sustainable process that offers significant advantages over chemical and physical approaches such as non-toxicity, low cost, biocompatibility, and high efficiency. Future studies can focus on developing more visible-light-driven TiO2-based photocatalytic materials. As the MPs are found to be combined with other co-pollutants in the environment, suitable approaches for the mitigation of combined pollution should be taken into consideration.
Author contributions
CM: Writing- original draft, review, and editing. AM: Conceptualization, review, editing and supervision.
Conflict of interest
The authors declare that the research was conducted in the absence of any commercial or financial relationships that could be construed as a potential conflict of interest.
Publisher’s note
All claims expressed in this article are solely those of the authors and do not necessarily represent those of their affiliated organizations, or those of the publisher, the editors and the reviewers. Any product that may be evaluated in this article, or claim that may be made by its manufacturer, is not guaranteed or endorsed by the publisher.
References
Akber Abbasi, S., Khalil, A. B., and Arslan, M. (2020). Extensive use of face masks during COVID-19 pandemic: (micro-)plastic pollution and potential health concerns in the arabian peninsula. Saudi J. Biol. Sci. 27, 3181–3186. doi:10.1016/j.sjbs.2020.09.054
Alabi, O. A., Ologbonjaye, K. I., Awosolu, O., and Alalade, O. E. (2019). Public and environmental health effects of plastic wastes disposal: A review. J. Toxicol. Risk Assess. 5, 1–13. doi:10.23937/2572-4061.1510021
Alvarado, J., Acosta, G., and Perez, F. (2016). Study of the effect of the dispersion of functionalized nanoparticles TiO2 with photocatalytic activity in LDPE. Polym. Degrad. Stab. 134, 376–382. doi:10.1016/j.polymdegradstab.2016.11.009
Ammendolia, J., Saturno, J., Brooks, A. L., Jacobs, S., and Jambeck, J. R. (2021). An emerging source of plastic pollution: Environmental presence of plastic personal protective equipment (PPE) debris related to COVID-19 in a metropolitan city. Environ. Pollut. 269, 116160. doi:10.1016/j.envpol.2020.116160
An, Y., Hou, J., Liu, Z., and Peng, B. (2014). Enhanced solid-phase photocatalytic degradation of polyethylene by TiO2–MWCNTs nanocomposites. Mater. Chem. Phys. 148, 387–394. doi:10.1016/j.matchemphys.2014.08.001
Anderson, A., Chandralingam, R., and PraveenKumar, T. R. (2021). Impact of COVID-19 pandemic on plastic surge and environmental effects. Energy Sources Part A Recovery Util. Environ. Eff., 1–7. doi:10.1080/15567036.2021.1900456
Aragaw, T. A. (2020). Surgical face masks as a potential source for microplastic pollution in the COVID-19 scenario. Mar. Pollut. Bull. 159, 111517. doi:10.1016/j.marpolbul.2020.111517
Ardusso, M., Forero-López, A. D., Buzzi, N. S., Spetter, C. V., and Fernández-Severini, M. D. (2021). COVID-19 pandemic repercussions on plastic and antiviral polymeric textile causing pollution on beaches and coasts of South America. Sci. Total Environ. 763, 144365. doi:10.1016/j.scitotenv.2020.144365
Ariza-Tarazona, M. C., Villarreal-Chiu, J. F., Barbieri, V., Siligardi, C., and Cedillo-González, E. I. (2019). New strategy for microplastic degradation: Green photocatalysis using a protein-based porous N-TiO2 semiconductor. Ceram. Int. 45, 9618–9624. doi:10.1016/j.ceramint.2018.10.208
Ariza-Tarazona, M. C., Villarreal-Chiu, J. F., Hernández-López, J. M., Rivera De la Rosa, J., Barbieri, V., Siligardi, C., et al. (2020). Microplastic pollution reduction by a carbon and nitrogen-doped TiO2: Effect of pH and temperature in the photocatalytic degradation process. J. Hazard. Mater. 395, 122632. doi:10.1016/j.jhazmat.2020.122632
Asghar, W., Qazi, I. A., Ilyas, H., Khan, A. A., Awan, M. A., and Rizwan Aslam, M. (2011). Comparative solid phase photocatalytic degradation of polythene films with doped and undoped TiO2 nanoparticles. J. Nanomater. 2011, 1–8. doi:10.1155/2011/461930
Aziz, A. A., Khatun, F., Monir, M. U., Ching, S. L., and Hon, L. K. (2021). “TiO2: A semiconductor photocatalyst,” in Titatium dioxide - advances and applications. Editor H. M. Ali (Rijeka: IntechOpen). Ch. 3. doi:10.5772/intechopen.99256
Badillo-Goicoechea, E., Chang, T. H., Kim, E., LaRocca, S., Morris, K., Deng, X., et al. (2021). Global trends and predictors of face mask usage during the COVID-19 pandemic. BMC Public Health 21, 2099–2112. doi:10.1186/s12889-021-12175-9
Barnes, R. J., Molina, R., Xu, J., Dobson, P. J., and Thompson, I. P. (2013). Comparison of TiO2 and ZnO nanoparticles for photocatalytic degradation of methylene blue and the correlated inactivation of gram-positive and gram-negative bacteria. J. Nanopart. Res. 15, 1432. doi:10.1007/s11051-013-1432-9
Benson, N. U., Bassey, D. E., and Palanisami, T. (2021). COVID pollution: Impact of COVID-19 pandemic on global plastic waste footprint. Heliyon 7, e06343. doi:10.1016/j.heliyon.2021.e06343
Bhuvaneshwari, M., Thiagarajan, V., Nemade, P., Chandrasekaran, N., and Mukherjee, A. (2018). Toxicity and trophic transfer of P25 TiO2 NPs from Dunaliella salina to Artemia salina: Effect of dietary and waterborne exposure. Environ. Res. 160, 39–46. doi:10.1016/j.envres.2017.09.022
Binda, G., Bellasi, A., Spanu, D., Pozzi, A., Cavallo, D., and Bettinetti, R. (2021). Evaluating the environmental impacts of personal protective equipment use by the general population during the COVID-19 pandemic: A case study of lombardy (northern Italy). Environ. (Basel). 8, 33. doi:10.3390/ENVIRONMENTS8040033
Cai, L., Hu, L., Shi, H., Ye, J., Zhang, Y., and Kim, H. (2018). Effects of inorganic ions and natural organic matter on the aggregation of nanoplastics. Chemosphere 197, 142–151. doi:10.1016/j.chemosphere.2018.01.052
Celis, J. E., Espejo, W., Paredes-Osses, E., Contreras, S. A., Chiang, G., and Bahamonde, P. (2021). Plastic residues produced with confirmatory testing for COVID-19: Classification, quantification, fate, and impacts on human health. Sci. Total Environ. 760, 144167. doi:10.1016/j.scitotenv.2020.144167
Chang, X., Xue, Y., Li, J., Zou, L., and Tang, M. (2020). Potential health impact of environmental micro- and nanoplastics pollution. J. Appl. Toxicol. 40, 4–15. doi:10.1002/jat.3915
Chen, J., He, Z., Ji, Y., Li, G., An, T., and Choi, W. (2019). OH radicals determined photocatalytic degradation mechanisms of gaseous styrene in TiO2 system under 254 nm versus 185 nm irradiation: Combined experimental and theoretical studies. Appl. Catal. B Environ. 257, 117912. doi:10.1016/j.apcatb.2019.117912
Chen, X., Chen, X., Liu, Q., Zhao, Q., Xiong, X., and Wu, C. (2021). Used disposable face masks are significant sources of microplastics to environment. Environ. Pollut. 285, 117485. doi:10.1016/j.envpol.2021.117485
Cho, S., and Choi, W. (2001). Solid-phase photocatalytic degradation of PVC–TiO2 polymer composites. J. Photochem. Photobiol. A Chem. 143, 221–228. doi:10.1016/S1010-6030(01)00499-3
Chowdhury, H., Chowdhury, T., and Sait, S. M. (2021). Estimating marine plastic pollution from COVID-19 face masks in coastal regions. Mar. Pollut. Bull. 168, 112419. doi:10.1016/j.marpolbul.2021.112419
Comunian, S., Dongo, D., Milani, C., and Palestini, P. (2020). Air pollution and Covid-19: The role of particulate matter in the spread and increase of Covid-19’s morbidity and mortality. Int. J. Environ. Res. Public Health 17, 4487–4522. doi:10.3390/ijerph17124487
Cordova, M. R., Nurhati, I. S., Riani, E., Nurhasanah, , and Iswari, M. Y. (2021). Unprecedented plastic-made personal protective equipment (PPE) debris in river outlets into Jakarta Bay during COVID-19 pandemic. Chemosphere 268, 129360. doi:10.1016/j.chemosphere.2020.129360
de Sousa, F. D. B. (2021). Plastic and its consequences during the COVID-19 pandemic. Environ. Sci. Pollut. Res. 28, 46067–46078. doi:10.1007/s11356-021-15425-w
De-la-Torre, G. E., Rakib, M. R. J., Pizarro-Ortega, C. I., and Dioses-Salinas, D. C. (2021). Occurrence of personal protective equipment (PPE) associated with the COVID-19 pandemic along the coast of Lima, Peru. Sci. Total Environ. 774, 145774. doi:10.1016/j.scitotenv.2021.145774
Dharmaraj, S., Ashokkumar, V., Hariharan, S., Manibharathi, A., Show, P. L., Chong, C. T., et al. (2021). The COVID-19 pandemic face mask waste: A blooming threat to the marine environment. Chemosphere 272, 129601. doi:10.1016/j.chemosphere.2021.129601
Domínguez-Jaimes, L. P., Cedillo-González, E. I., Luévano-Hipólito, E., Acuña-Bedoya, J. D., and Hernández-López, J. M. (2021). Degradation of primary nanoplastics by photocatalysis using different anodized TiO2 structures. J. Hazard. Mater. 413, 125452. doi:10.1016/j.jhazmat.2021.125452
Du, H., Xie, Y., and Wang, J. (2021). Microplastic degradation methods and corresponding degradation mechanism: Research status and future perspectives. J. Hazard. Mater. 418, 126377. doi:10.1016/j.jhazmat.2021.126377
Du, H., Huang, S., and Wang, J. (2022a). Environmental risks of polymer materials from disposable face masks linked to the COVID-19 pandemic. Sci. Total Environ. 815, 152980. doi:10.1016/j.scitotenv.2022.152980
Du, H., Xie, Y., and Wang, J. (2022b). Environmental impacts of microplastics on fishery products: An overview. Gondwana Res. 108, 213–220. doi:10.1016/j.gr.2021.08.013
Enyoh, C. E., Verla, A. W., Verla, E. N., Ibe, F. C., and Amaobi, C. E. (2019). Airborne microplastics: A review study on method for analysis, occurrence, movement and risks. Environ. Monit. Assess. 191, 668. doi:10.1007/s10661-019-7842-0
Fa, W., Zan, L., Gong, C., Zhong, J., and Deng, K. (2008). Solid-phase photocatalytic degradation of polystyrene with TiO2 modified by iron (II) phthalocyanine. Appl. Catal. B Environ. 79, 216–223. doi:10.1016/j.apcatb.2007.10.018
Fa, W., Guo, L., Wang, J., Guo, R., Zheng, Z., and Yang, F. (2013). Solid-phase photocatalytic degradation of polystyrene with TiO2/Fe(St)3 as catalyst. J. Appl. Polym. Sci. 128, 2618–2622. doi:10.1002/app.37751
Fadare, O. O., and Okoffo, E. D. (2020). Covid-19 face masks: A potential source of microplastic fibers in the environment. Sci. Total Environ. 737, 140279. doi:10.1016/j.scitotenv.2020.140279
Fadli, M. H., Ibadurrohman, M., and Slamet, S. (2021). Microplastic pollutant degradation in water using modified TiO2 photocatalyst under UV-irradiation. IOP Conf. Ser. Mater. Sci. Eng. 1011, 012055. doi:10.1088/1757-899X/1011/1/012055
Fernández, C., and Anastasopoulou, A. (2019). Plastic ingestion by blue shark Prionace glauca in the south pacific ocean (south of the Peruvian sea). Mar. Pollut. Bull. 149, 110501. doi:10.1016/j.marpolbul.2019.110501
Ge, J., Zhang, Z., Ouyang, Z., Shang, M., Liu, P., Li, H., et al. (2022). Photocatalytic degradation of (micro)plastics using TiO2-based and other catalysts: Properties, influencing factor, and mechanism. Environ. Res. 209, 112729. doi:10.1016/j.envres.2022.112729
Hamd, W., Daher, E. A., Tofa, T. S., and Dutta, J. (2022). Recent advances in photocatalytic removal of microplastics: Mechanisms, kinetic degradation, and reactor design. Front. Mar. Sci. 9, 1–28. doi:10.3389/fmars.2022.885614
Hantoko, D., Li, X., Pariatamby, A., Yoshikawa, K., Horttanainen, M., and Yan, M. (2021). Challenges and practices on waste management and disposal during COVID-19 pandemic. J. Environ. Manage. 286, 112140. doi:10.1016/j.jenvman.2021.112140
Herron, J. B. T., Hay-David, A. G. C., Gilliam, A. D., and Brennan, P. A. (2020). Personal protective equipment and Covid 19- a risk to healthcare staff? Br. J. Oral Maxillofac. Surg. 58, 500–502. doi:10.1016/j.bjoms.2020.04.015
Hiemstra, A.-F., Rambonnet, L., Gravendeel, B., and Schilthuizen, M. (2021). The effects of COVID-19 litter on animal life. Anim. Biol. Leiden. Neth. 71, 215–231. doi:10.1163/15707563-bja10052
Jeong, C.-B., Won, E.-J., Kang, H.-M., Lee, M.-C., Hwang, D.-S., Hwang, U.-K., et al. (2016). Microplastic size-dependent toxicity, oxidative stress induction, and p-JNK and p-p38 activation in the monogonont rotifer (Brachionus koreanus). Environ. Sci. Technol. 50, 8849–8857. doi:10.1021/acs.est.6b01441
Kane, I. A., Clare, M. A., Miramontes, E., Wogelius, R., Rothwell, J. J., Garreau, P., et al. (2020). Seafloor microplastic hotspots controlled by deep-sea circulation. Sci. (80-. ) 368, 1140–1145. doi:10.1126/science.aba5899
Khoo, K. S., Ho, L. Y., Lim, H. R., Leong, H. Y., and Chew, K. W. (2021). Plastic waste associated with the COVID-19 pandemic: Crisis or opportunity? J. Hazard. Mater. 417, 126108. doi:10.1016/j.jhazmat.2021.126108
Kirstein, I. V., Wichels, A., Gullans, E., Krohne, G., and Gerdts, G. (2019). The Plastisphere – uncovering tightly attached plastic “specific” microorganisms. PLoS One 14, e0215859. doi:10.1371/journal.pone.0215859
Klemeš, J. J., Fan, Y. V., and Jiang, P. (2020). The energy and environmental footprints of COVID-19 fighting measures – PPE, disinfection, supply chains. Energy 211, 118701. doi:10.1016/j.energy.2020.118701
Lee, Q. Y., and Li, H. (2021). Photocatalytic degradation of plastic waste: A mini review. Micromachines 12, 907. doi:10.3390/mi12080907
Li, S., Xu, S., He, L., Xu, F., Wang, Y., and Zhang, L. (2010). Photocatalytic degradation of polyethylene plastic with polypyrrole/TiO2 nanocomposite as photocatalyst. Polym. Plast. Technol. Eng. 49, 400–406. doi:10.1080/03602550903532166
Li, W. C., Tse, H. F., and Fok, L. (2016). Plastic waste in the marine environment: A review of sources, occurrence and effects. Sci. Total Environ. 566–567, 333–349. doi:10.1016/j.scitotenv.2016.05.084
Li, L., Luo, Y., Li, R., Zhou, Q., Peijnenburg, W. J. G. M., Yin, N., et al. (2020). Effective uptake of submicrometre plastics by crop plants via a crack-entry mode. Nat. Sustain. 3, 929–937. doi:10.1038/s41893-020-0567-9
Li, L., Zhao, X., Li, Z., and Song, K. (2021). COVID-19: Performance study of microplastic inhalation risk posed by wearing masks. J. Hazard. Mater. 411, 124955. doi:10.1016/j.jhazmat.2020.124955
Li, L. (2020). The reaction mechanism of photoelectrocatalysis on the surface of TiO2 nanotube array electrode. Asia. Pac. J. Chem. Eng. 15, e2511. doi:10.1002/apj.2511
Liang, W., Luo, Y., Song, S., Dong, X., and Yu, X. (2013). High photocatalytic degradation activity of polyethylene containing polyacrylamide grafted TiO2. Polym. Degrad. Stab. 98, 1754–1761. doi:10.1016/j.polymdegradstab.2013.05.027
Liang, Y., Song, Q., Wu, N., Li, J., Zhong, Y., and Zeng, W. (2021). Repercussions of COVID-19 pandemic on solid waste generation and management strategies. Front. Environ. Sci. Eng. 15, 115. doi:10.1007/s11783-021-1407-5
Ma, Z., Jia, Q., Tao, C., and Han, B. (2020). Highlighting unique function of immobilized superoxide on TiO2 for selective photocatalytic degradation. Sep. Purif. Technol. 238, 116402. doi:10.1016/j.seppur.2019.116402
Mandade, P. (2021). “Chapter 5 - introduction, basic principles, mechanism, and challenges of photocatalysis,” in Micro and nano technologies. Editors B. Bhanvase, S. Sonawane, and V. Pawade (Elsevier), 137–154. doi:10.1016/B978-0-12-821496-1.00016-7
Martinez, U., and Hammer, B. (2011). Adsorption properties versus oxidation states of rutile TiO2(110). J. Chem. Phys. 134, 194703. doi:10.1063/1.3589861
Maulana, D. A., Ibadurrohman, M., and Slamet, (2021). Synthesis of nano-composite Ag/TiO2 for polyethylene microplastic degradation applications. IOP Conf. Ser. Mater. Sci. Eng. 1011, 012054. doi:10.1088/1757-899X/1011/1/012054
Mekprasart, W., Thongpradith, T., Pecharapa, W., and Ishihara, K. N. (2018). Photocatalytic properties and plastic degradation of TiO2 nanocomposite with synthetic-rutile from natural ore. J. Jpn. Soc. Powder Powder Metall. 65, 719–724. doi:10.2497/jjspm.65.719
Morgana, S., Casentini, B., and Amalfitano, S. (2021). Uncovering the release of micro/nanoplastics from disposable face masks at times of COVID-19. J. Hazard. Mater 419, 126507. doi:10.1016/j.jhazmat.2021.126507
Nabi, I., Bacha, A. U. R., Li, K., Cheng, H., Wang, T., Liu, Y., et al. (2020). Complete photocatalytic mineralization of microplastic on TiO2 nanoparticle film. iScience 23, 101326. doi:10.1016/j.isci.2020.101326
Nabi, I., Bacha, A. U. R., Ahmad, F., and Zhang, L. (2021). Application of titanium dioxide for the photocatalytic degradation of macro- and micro-plastics: A review. J. Environ. Chem. Eng. 9, 105964. doi:10.1016/j.jece.2021.105964
Nakata, K., and Fujishima, A. (2012). TiO2 photocatalysis: Design and applications. J. Photochem. Photobiol. C Photochem. Rev. 13, 169–189. doi:10.1016/j.jphotochemrev.2012.06.001
Okuku, E., Kiteresi, L., Owato, G., Otieno, K., Mwalugha, C., Mbuche, M., et al. (2021). The impacts of COVID-19 pandemic on marine litter pollution along the Kenyan coast: A synthesis after 100 days following the first reported case in Kenya. Mar. Pollut. Bull. 162, 111840. doi:10.1016/j.marpolbul.2020.111840
Parashar, N., and Hait, S. (2021). Plastics in the time of COVID-19 pandemic: Protector or polluter? Sci. Total Environ. 759, 144274. doi:10.1016/j.scitotenv.2020.144274
Parton, K. J., Godley, B. J., Santillo, D., Tausif, M., Omeyer, L. C. M., and Galloway, T. S. (2020). Investigating the presence of microplastics in demersal sharks of the North-East Atlantic. Sci. Rep. 10, 12204. doi:10.1038/s41598-020-68680-1
Patrício Silva, A. L., Prata, J. C., Walker, T. R., Campos, D., Duarte, A. C., Soares, A. M. V. M., et al. (2020). Rethinking and optimising plastic waste management under COVID-19 pandemic: Policy solutions based on redesign and reduction of single-use plastics and personal protective equipment. Sci. Total Environ. 742, 140565. doi:10.1016/j.scitotenv.2020.140565
Patrício Silva, A. L., Prata, J. C., Mouneyrac, C., Barcelò, D., Duarte, A. C., and Rocha-Santos, T. (2021). Risks of Covid-19 face masks to wildlife: Present and future research needs. Sci. Total Environ. 792, 148505. doi:10.1016/j.scitotenv.2021.148505
Peerakiatkhajohn, P., Onreabroy, W., Chawengkijwanich, C., and Chiarakorn, S. (2011). Preparation of visible-light-responsive TiO2 doped Ag thin film on PET plastic for BTEX treatment. J. Sustain Energy Env. 2, 121–125. Available at: https://www.scopus.com/inward/record.uri?eid=2-s2.0-84900415030&partnerID=40&md5=c55788cbeeae48ad8d32d2c2d0caa6ea.
Peris, S. J. (2003). Feeding in urban refuse dumps: Ingestion of plastic objects by the White Stork (Ciconia ciconia). Ardeola 50, 81–84. Available at: https://www.scopus.com/inward/record.uri?eid=2-s2.0-1342268055&partnerID=40&md5=72b8b0ad3d59664e6e8cc54f81bb8136.
Prata, J. C., Silva, A. L. P., Walker, T. R., Duarte, A. C., and Rocha-Santos, T. (2020). COVID-19 pandemic repercussions on the use and management of plastics. Environ. Sci. Technol. 54, 7760–7765. doi:10.1021/acs.est.0c02178
Prüst, M., Meijer, J., and Westerink, R. H. S. (2020). The plastic brain: Neurotoxicity of micro- and nanoplastics. Part. Fibre Toxicol. 17, 24. doi:10.1186/s12989-020-00358-y
Ragusa, A., Svelato, A., Santacroce, C., Catalano, P., Notarstefano, V., Carnevali, O., et al. (2021). Plasticenta: First evidence of microplastics in human placenta. Environ. Int. 146, 106274. doi:10.1016/j.envint.2020.106274
Ray, S. S., Lee, H. K., Huyen, D. T. T., Chen, S. S., and Kwon, Y. N. (2022). Microplastics waste in environment: A perspective on recycling issues from PPE kits and face masks during the COVID-19 pandemic. Environ. Technol. Innov. 26, 102290. doi:10.1016/j.eti.2022.102290
Rodríguez-Narvaez, O. M., Goonetilleke, A., Perez, L., and Bandala, E. R. (2021). Engineered technologies for the separation and degradation of microplastics in water: A review. Chem. Eng. J. 414, 128692. doi:10.1016/j.cej.2021.128692
Saliu, F., Veronelli, M., Raguso, C., Barana, D., Galli, P., and Lasagni, M. (2021). The release process of microfibers: from surgical face masks into the marine environment. Environ. Adv. 4, 100042. doi:10.1016/j.envadv.2021.100042
Schwabl, P., Köppel, S., Königshofer, P., Bucsics, T., Trauner, M., Reiberger, T., et al. (2019). Detection of various microplastics in human stool. Ann. Intern. Med. 171, 453–457. doi:10.7326/M19-0618
Seif, S., Provencher, J. F., Avery-Gomm, S., Daoust, P.-Y., Mallory, M. L., and Smith, P. A. (2018). Plastic and non-plastic debris ingestion in three gull species feeding in an urban landfill environment. Arch. Environ. Contam. Toxicol. 74, 349–360. doi:10.1007/s00244-017-0492-8
Selvaranjan, K., Navaratnam, S., Rajeev, P., and Ravintherakumaran, N. (2021). Environmental challenges induced by extensive use of face masks during COVID-19: A review and potential solutions. Environ. Challenges 3, 100039. doi:10.1016/j.envc.2021.100039
Shabbir, S., Kulyar, M. F. e. A., Bhutta, Z. A., Boruah, P., and Asif, M. (2021). Toxicological consequences of titanium dioxide nanoparticles (TiO2NPs) and their jeopardy to human population. Bionanoscience 11, 621–632. doi:10.1007/s12668-021-00836-3
Shams, M., Alam, I., and Mahbub, M. S. (2021). Plastic pollution during COVID-19: Plastic waste directives and its long-term impact on the environment. Environ. Adv. 5, 100119. doi:10.1016/j.envadv.2021.100119
Shang, J., Chai, M., and Zhu, Y. (2003a). Photocatalytic degradation of polystyrene plastic under fluorescent light. Environ. Sci. Technol. 37, 4494–4499. doi:10.1021/es0209464
Shang, J., Chai, M., and Zhu, Y. (2003b). Solid-phase photocatalytic degradation of polystyrene plastic with TiO2 as photocatalyst. J. Solid State Chem. 174, 104–110. doi:10.1016/S0022-4596(03)00183-X
Sharma, S., Basu, S., Shetti, N. P., Nadagouda, M. N., and Aminabhavi, T. M. (2021). Microplastics in the environment: Occurrence, perils, and eradication. Chem. Eng. J. 408, 127317. doi:10.1016/j.cej.2020.127317
Song, Y. K., Hong, S. H., Jang, M., Han, G. M., Jung, S. W., and Shim, W. J. (2017). Combined effects of UV exposure duration and mechanical abrasion on microplastic fragmentation by polymer type. Environ. Sci. Technol. 51, 4368–4376. doi:10.1021/acs.est.6b06155
Su, Y., Ashworth, V., Kim, C., Adeleye, A. S., Rolshausen, P., Roper, C., et al. (2019). Delivery, uptake, fate, and transport of engineered nanoparticles in plants: A critical review and data analysis. Environ. Sci. Nano 6, 2311–2331. doi:10.1039/C9EN00461K
Sullivan, G. L., Delgado-Gallardo, J., Watson, T. M., and Sarp, S. (2021). An investigation into the leaching of micro and nano particles and chemical pollutants from disposable face masks - linked to the COVID-19 pandemic. Water Res. 196, 117033. doi:10.1016/j.watres.2021.117033
Thomas, R. T., and Sandhyarani, N. (2013). Enhancement in the photocatalytic degradation of low density polyethylene–TiO2 nanocomposite films under solar irradiation. RSC Adv. 3, 14080–14087. doi:10.1039/C3RA42226G
Thomas, R. T., Nair, V., and Sandhyarani, N. (2013). TiO2 nanoparticle assisted solid phase photocatalytic degradation of polythene film: A mechanistic investigation. Colloids Surfaces A Physicochem. Eng. Aspects 422, 1–9. doi:10.1016/j.colsurfa.2013.01.017
Torres, F. G., and De-la-Torre, G. E. (2021). Face mask waste generation and management during the COVID-19 pandemic: An overview and the Peruvian case. Sci. Total Environ. 786, 147628. doi:10.1016/j.scitotenv.2021.147628
Torres-Agullo, A., Karanasiou, A., Moreno, T., and Lacorte, S. (2021). Overview on the occurrence of microplastics in air and implications from the use of face masks during the COVID-19 pandemic. Sci. Total Environ. 800, 149555. doi:10.1016/j.scitotenv.2021.149555
Turkten, N., and Bekbolet, M. (2020). Photocatalytic performance of titanium dioxide and zinc oxide binary system on degradation of humic matter. J. Photochem. Photobiol. A Chem. 401, 112748. doi:10.1016/j.jphotochem.2020.112748
Uheida, A., Mejía, H. G., Abdel-Rehim, M., Hamd, W., and Dutta, J. (2021). Visible light photocatalytic degradation of polypropylene microplastics in a continuous water flow system. J. Hazard. Mater. 406, 124299. doi:10.1016/j.jhazmat.2020.124299
Ullattil, S. G., and Periyat, P. (2017). “Sol-gel synthesis of titanium dioxide,” in Sol-gel materials for energy, environment and electronic applications. Editors S. C. Pillai, and S. Hehir (Cham: Springer International Publishing), 271–283. doi:10.1007/978-3-319-50144-4_9
Urban, R. C., and Nakada, L. Y. K. (2021). COVID-19 pandemic: Solid waste and environmental impacts in Brazil. Sci. Total Environ. 755, 142471. doi:10.1016/j.scitotenv.2020.142471
Verma, R., Singh, S., Dalai, M. K., Saravanan, M., Agrawal, V. V., and Srivastava, A. K. (2017). Photocatalytic degradation of polypropylene film using TiO2-based nanomaterials under solar irradiation. Mater. Des. 133, 10–18. doi:10.1016/j.matdes.2017.07.042
Vieten, U. M. (2020). The “new normal” and “pandemic populism”: The COVID-19 crisis and anti-hygienic mobilisation of the far-right. Soc. Sci. (Basel). 9, 165. doi:10.3390/socsci9090165
Wang, T., Hu, M., Xu, G., Shi, H., Leung, J. Y. S., and Wang, Y. (2021a). Microplastic accumulation via trophic transfer: Can a predatory crab counter the adverse effects of microplastics by body defence? Sci. Total Environ. 754, 142099. doi:10.1016/j.scitotenv.2020.142099
Wang, Z., An, C., Chen, X., Lee, K., Zhang, B., and Feng, Q. (2021b). Disposable masks release microplastics to the aqueous environment with exacerbation by natural weathering. J. Hazard. Mater. 417, 126036. doi:10.1016/j.jhazmat.2021.126036
WHO (2022). Coronavirus disease (COVID-19): Masks. Available at: https://www.who.int/emergencies/diseases/novel-coronavirus-2019/question-and-answers-hub/q-a-detail/coronavirus-disease-covid-19-masks.
WWF International (2020). Disposal of Masks and Gloves, Responsibility is Required. Available at: https://www.wwf.it/chi_siamo/organizzazione/.
Yang, C., Gong, C., Peng, T., Deng, K., and Zan, L. (2010). High photocatalytic degradation activity of the polyvinyl chloride (PVC)–vitamin C (VC)–TiO2 nano-composite film. J. Hazard. Mater. 178, 152–156. doi:10.1016/j.jhazmat.2010.01.056
Yang, S., Cheng, Y., Liu, T., Huang, S., Yin, L., Pu, Y., et al. (2022). Impact of waste of COVID-19 protective equipment on the environment, animals and human health: A review. Environ. Chem. Lett. 20, 2951–2970. doi:10.1007/s10311-022-01462-5
Yuan, K., Cao, Q., Lu, H.-L., Zhong, M., Zheng, X., Chen, H.-Y., et al. (2017). Oxygen-deficient WO3−x@TiO2−x core–shell nanosheets for efficient photoelectrochemical oxidation of neutral water solutions. J. Mater. Chem. A 5, 14697–14706. doi:10.1039/C7TA03878J
Zan, L., Tian, L., Liu, Z., and Peng, Z. (2004). A new polystyrene–TiO2 nanocomposite film and its photocatalytic degradation. Appl. Catal. A General 264, 237–242. doi:10.1016/j.apcata.2003.12.046
Zhang, F., Wang, X., Liu, H., Liu, C., Wan, Y., Long, Y., et al. (2019). Recent advances and applications of semiconductor photocatalytic technology. Appl. Sci. (Basel). 9, 2489. doi:10.3390/app9122489
Zhang, J., Wang, L., Trasande, L., and Kannan, K. (2021). Occurrence of polyethylene terephthalate and polycarbonate microplastics in infant and adult feces. Environ. Sci. Technol. Lett. 8, 989–994. doi:10.1021/acs.estlett.1c00559
Zhao, X. u., Li, Z., Chen, Y., Shi, L., and Zhu, Y. (2007). Solid-phase photocatalytic degradation of polyethylene plastic under UV and solar light irradiation. J. Mol. Catal. A Chem. 268, 101–106. doi:10.1016/j.molcata.2006.12.012
Zhao, X., Li, Z., Chen, Y., Shi, L., and Zhu, Y. (2008). Enhancement of photocatalytic degradation of polyethylene plastic with CuPc modified TiO2 photocatalyst under solar light irradiation. Appl. Surf. Sci. 254, 1825–1829. doi:10.1016/j.apsusc.2007.07.154
Keywords: COVID-19, face mask, microplasitcs, waste management, TiO2, photocatalytic remediation
Citation: Rex M C and Mukherjee A (2022) Prospects of TiO2-based photocatalytic degradation of microplastic leachates related disposable facemask, a major COVID-19 waste. Front. Nanotechnol. 4:1072227. doi: 10.3389/fnano.2022.1072227
Received: 17 October 2022; Accepted: 10 November 2022;
Published: 18 November 2022.
Edited by:
Ajeet Kaushik, Florida Polytechnic University, United StatesReviewed by:
Anshu Kumari, University of Maryland, Baltimore, United StatesSharad Ambardar, Boston College, United States
Copyright © 2022 Rex M and Mukherjee. This is an open-access article distributed under the terms of the Creative Commons Attribution License (CC BY). The use, distribution or reproduction in other forums is permitted, provided the original author(s) and the copyright owner(s) are credited and that the original publication in this journal is cited, in accordance with accepted academic practice. No use, distribution or reproduction is permitted which does not comply with these terms.
*Correspondence: Amitava Mukherjee, YW1pdC5tb29rZXJqZWFAZ21haWwuY29t