- 1Department of Energy Science (DOES) and Center for Artificial Atoms, Sungkyunkwan University (SKKU), Suwon, Republic of Korea
- 2Sungkyunkwan Institute of Energy Science and Technology (SIEST), Sungkyunkwan University (SKKU), Suwon, Republic of Korea
Semiconductor clusters have been implicated as reaction intermediates between molecular precursors and colloidal quantum dots (CQDs). The success of isolation of semiconductor clusters have enabled detailed investigation of the atomic information of semiconductor clusters. The identification of atomic information has emerged as an important topic because knowledge of the structure-function relationship of intermediate clusters has been helpful to reveal the synthetic mechanism of CQDs. Recently, they have been utilized as the synthetic precursors for CQDs, which was not readily achieved using conventional molecular precursors. This mini review briefly introduces the current understanding of their atomic information such as the composition, structure, and surface. We then discuss advantages, limitations, and the perspective of semiconductor clusters as a precursor for synthesis of CQDs.
Introduction
Semiconductor clusters have been identified as reaction intermediates in the formation of CQDs. They contain tens to hundreds of inorganic atoms, where the surface is coordinated with molecular ligands (Park et al., 2021). Their unique photophysical properties originate from smaller entities than conventional CQDs, which have recently intrigued this field of study. Moreover, as they exist in a thermodynamically local minimum state, such that these clusters can be isolated or synthesized in order to investigate atomic information using various chemical techniques assisted by quantum simulation.
Knowledge of the atomic information of semiconductor clusters such as the composition, coordination geometry of inorganic atoms, and the coordination geometry of organic functional groups in molecular ligands is required to understand the structure-function relationship. For example, Cossairt and co-workers synthesized truly monodispersed indium phosphide (InP) magic-sized clusters (MSC) and fully resolved their atomic information with single crystal X-ray crystallography. Furthermore, the electronic structure of InP MSCs based on atomic information was elucidated via optical transition analysis with density functional theory calculation (Gary et al., 2016).
In lieu of one of the functions, semiconductor clusters play key roles as precursors for the synthesis of CQDs. Jensen and co-workers showed that InP clusters could serve as a monomer-supplier for the formation of larger InP nanocrystals (Xie et al., 2016). Jeong and co-worker employed the indium arsenide (InAs) cluster as a source of monomer to induce a size-focused growth mechanism (Tamang et al., 2016; Kim et al., 2021).
In this paper, we introduce the current research activities on the structure-function relationship based on atomic information for semiconductor clusters. Moreover, we examine the advantages and usage limitations as a synthetic precursor for synthesis of CQDs.
Atomic information of semiconductor clusters
The physical properties of semiconductor clusters are determined by their composition, structure, and surface chemistry. The presence of cluster isomers, however, makes it hard to isolate and characterize semiconductor clusters. Moreover, it is difficult to configure the atomic information of semiconductor clusters due to a smaller size, lower crystallinity, and lower stability than CQDs (Palencia et al., 2020). Nevertheless, using various chemical analysis techniques, partial information about the cluster composition and structure can be obtained (Kwon and Kim, 2021). For example, with composition analysis such as inductively coupled plasma (ICP) and energy-dispersive spectroscopy (EDS), both InP and cadmium sulfide (CdS) clusters show cation rich composition (Nevers et al., 2017; Kwon et al., 2020). In powder X-ray diffraction (pXRD) analysis, spectra of semiconductor clusters typically exhibit a broad peak, which was different from the well-defined peak of CQDs because of its small size, less than 2 nm (Figure 1A; Tamang et al., 2016; Ning and Banin, 2017; Kwon et al., 2020). Structural information such as interatomic distance and coordination number of elements could be resolved using X-ray absorption fine structure spectroscopy (EXAFS) analysis, as confirmed from the change of interatomic distance depending on ligands in semiconductor cluster (Rockenberger et al., 1997; Hsieh et al., 2018; Palencia et al., 2020). Further, with pair distribution function (PDF) analysis, it was identified that core structures differed depending on ligands (Gary et al., 2015).
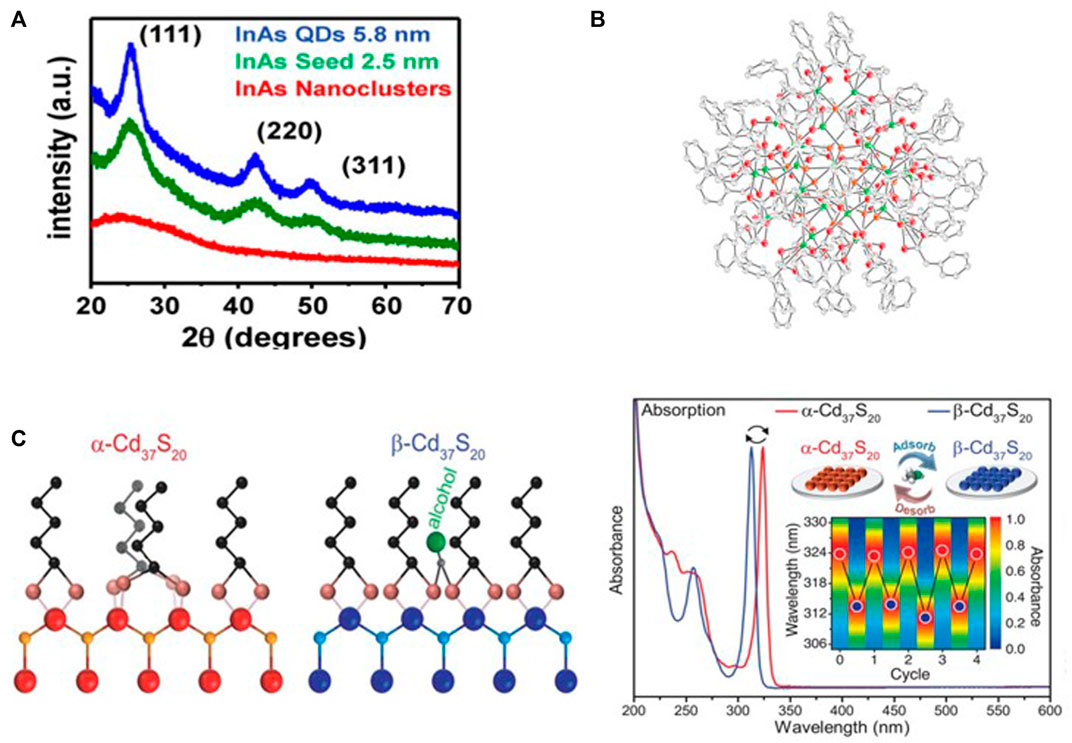
FIGURE 1. (A) XRD spectra of InAs CQDs, InAs seed, and amorphous InAs semiconductor cluster (reprinted with permission from Tamang et al., 2016). (B) Molecular structure and absorption spectra of In37P20(O2CCH2Ph)51 semiconductor cluster (InP MSC). The cluster has the [In21P20]3+ core and is passivated by carboxylate ligands. (reprinted with permission from Gary et al., 2016). (C) Ligand configuration and absorption spectra of the Cd37S20 isomer. The adsorption of methanol induces reconfiguration of ligands and isomerization from α-Cd37S20 to β-Cd37S20. The structural change was reversible and shifts the excitonic peaks of the absorption spectra (reprinted with permission from Williamson et al., 2019).
Through the combination of several analysis techniques, estimated sizes and further structural information about the clusters have been identified. Also, changes in physical properties according to cluster structure have been observed. For example, Kim and co-worker isolated early and later stage InP MSC during the CQDs synthesis process and identified the differences in the structural and optical properties of the MSCs. They reveal that the later stage InP MSC has a zinc-blende like structure, and has a composition which is similar to InP CQDs. They also reported that the later stage InP MSC has InP CQD-like optical properties such as sharp and intense band feature in photoluminescence (PL) spectra and band-edge bleach transient absorption (TA) signal in the lower-energy region. On the other hand, the early stage InP MSC showed different structural and optical features which are more similar with molecule than InP CQDs (Kwon et al., 2020).
Additionally, single crystal X-ray crystallography (SCXRD) analysis was performed to obtain a detailed description regarding the structure of the cluster. It has been performed only with a few clusters because of the practical limitations that include difficulty in obtaining the single crystal of the clusters by existence of cluster isomers. SCXRD analysis was used to define the physical structure of CdSe magic-sized clusters (CdSe MSCs). Hyeon and co-workers reported the structure of Cd14Se13 MSC which has a unique non-tetrahedral arrangement where the Se atom is encapsulated by adamantane-like Cd14Se12 cage (Bootharaju et al., 2022). Also, the Cossairt group identified the whole structure and composition of InP MSC by SCXRD which has an absorption transition at 386 nm (Figure 1B). The structure of InP MSC was unveiled as In37P20(O2CCH2Ph)51, which was divided into two parts: core and surface. The core of InP MSC is consisted of [In21P20]3+ and has C2 rotation axis. Additionally, 16 surface indium atoms are bound to the core P atoms and 51 carboxylate ligands are coordinated on the surface of InP MSC. They also defined the electronic structure of InP MSC through absorption spectrum and time-dependent density functional theory (TDDFT) calculations. They showed that the peak shape of the absorption spectrum of InP MSC has asymmetry and line broadening, and suggests that the peak shape is determined by various separated electronic transitions coupling, which originates with lattice vibrations (Gary et al., 2016). In short, structure information of semiconductor clusters has been obtained by complementary analytical tools and the connection between optoelectronic properties and atomic structure of semiconductor clusters was identified.
The proportion of surface per unit mass in the structure on semiconductor cluster is higher than that on larger nanocrystals because of their small size. The surface of the clusters was covered with ligands and thereby surface-ligand chemistry of the clusters was directly connected to the properties of the clusters. Robinson and co-workers observed that adsorption of hydroxyl species on surface of cadmium sulfide magic-sized clusters (CdS MSC) induce reversible isomerization from wurtzite-like structure to zinc blende-like structure by changing ligand configurations (Figure 1C). Methanol adsorbed on surface of the cluster forms hydrogen bonds with the oleate ligands. This hydrogen bonding distorts the bond angle of the oleate ligands that induces the change of the ligand binding modes, and it was followed by isomerization of the cluster. The structural change shifts the excitonic energy gaps of two isomers about 140 meV (Williamson et al., 2019). In addition to ligand reconfiguration of the cluster by hydroxyl species treatment, it has been reported in CdS MSC that other chemical treatments on the cluster also induce the surface change and absorption spectra shift. For example, thiol treatment adds a monolayer of sulfur on the surface of the cluster and a red shift was observed on absorption spectra. Also, amine treatment causes surface etching by L-promoted Z-type ligand displacement, and a red shift in the excitonic peak was observed after the chemical treatment (Nevers et al., 2017). In the case of the InP cluster, Cossairt and co-workers characterized the surface properties and quantified the surface-exchange equilibria according to ligand exchange of carboxylate-capped InP clusters in the carboxylate, phosphonate, and thiolate systems. By using the InP cluster, which is molecularly precise, they simplified the equilibrium models and reduced variables that can occur by the particle sizes, composition and morphology of samples (Ritchhart and Cossairt., 2019).
To sum up, chemical treatments on the surface of clusters modify not only surface properties but also structure and optoelectronic properties of the clusters. This change of the properties is difficult to observe in larger CQDs that have a lower surface ratio than clusters; therefore, the clusters provide a good platform to study ligand-surface interactions (Nevers et al., 2017).
Cluster as a synthetic precursor
Semiconductor clusters have been reported as intermediates or byproducts on CQD synthesis. These clusters, which are isolated, can be used as a reservoir of monomers to synthesize CQDs (Tamang et al., 2016; Park et al., 2022). Mainly because of the structural and compositional inhomogeneity in semiconductor clusters, understanding the exact role of the clusters in the CQDs synthesis process have been limited. By analyzing the cluster as a synthetic precursor and the product produced in the synthesis process, the researchers have limitedly inferred indirect information about the reaction pathway and functionality of the semiconductor cluster as a precursor (Gary et al., 2015; Friedfeld et al., 2017; Kwon and Kim, 2021). Studies on the reaction pathway of the clusters in the synthesis of CQDs, revealed that thermally stable clusters could not effectively supply monomers without the additional of other materials to destabilize the clusters. However, thermally unstable clusters can be easily decomposed into monomers, increasing the monodispersity of CQDs when the clusters are used as the precursor (Gary et al., 2015). For example, the highly stable InP MSC, which has different optical properties from the carboxylate-ligated InP MSC, were formed with a phosphonate ligand set; and it has a resistance on conversion step to monomers, which is important for acting as precursor (Figure 2A). Additionally, before carboxylate-ligated InP MSC can react as the precursor, the structure of the clusters show neither zinc-blende nor wurtzite structure, but InP CQDs formed by using InP MSC as a precursor has a zinc-blende structure. So, carboxylate-ligated InP MSC seems to be completely dissolved and undergoes the procedure of crystal structure rearrangement (Gary et al., 2016; Friedfeld et al., 2017).
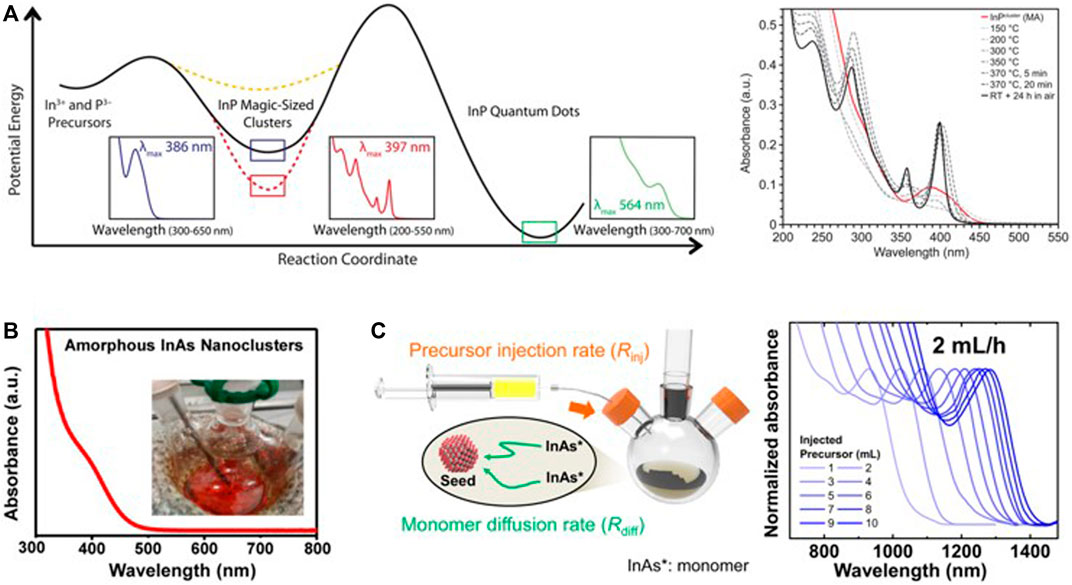
FIGURE 2. (A) Energy landscape on reaction coordinates from In and P precursors to InP CQDs. Synthesis of octadecylphosphonate (ODPA) coordinated InP MSC via heat up with myristic acid (MA) coordinated InP MSC and In(ODPA)1.5 (reprinted with permission from Gary et al., 2015). (B) Absorption spectrum of amorphous InAs semiconductor cluster (reprinted with permission from Tamang et al., 2016). (C) The strategy of InAs amorphous cluster-based single source precursor for synthesis monodisperse InAs CQDs. Absorption spectra on InAs CQD synthesis with precursor injection rate, 2 ml/h (reprinted with permission from Kim et al., 2021).
In the case of the InAs cluster, it has been reported that the cluster acts as an effective single-source precursor to form high-quality InAs CQDs, which have a narrow size distribution through the seed growth mechanism where monomers generated by the cluster deposit onto seed CQDs. Jeong and co-workers employed InAs amorphous clusters as a precursor which are synthesized at room temperature using indium oleate and tris(trimethylsilyl)arsine. In Figure 2B, InAs amorphous clusters are not uniform in size, have amorphous nature, and a broad absorption feature (∼400 nm), which is different than InAs MSC. InAs MSC showed thermally stable at high temperature and with a distinct absorption feature at 420 and 460 nm (Xie and Peng, 2008; Xie et al., 2009), on the other hand, the InAs amorphous cluster showed easy decomposition and continuous growth behavior when InAs amorphous clusters are heated (Tamang et al., 2016).
Slow and continuous injection of InAs amorphous cluster could maintain the monomer reservoir in the growth step and excluded the adverse effect of precursor depletion such as Ostwald ripening (Tamang et al., 2016). However, there are limitations to growing CQDs larger than 5 nm, obtained only by slow and continuous injection of InAs amorphous cluster. They investigated that monomer concentration is controlled by precursor injection rate (Rinj) and monomer diffusion rate (Rriff). Through the monitoring of CQD growth at different Rinj, they observed that slow Rinj (2 ml/h) can prevent burst precursor conversion that can induce secondary nucleation (Figure 2C). Moreover, the diffusion-dynamics-controlled synthesis process which controls the monomer flux shows that the clusters can overcome the size limit originated from monomer reactivity (Tamang et al., 2016; Kim et al., 2021). To sum up, semiconductor clusters are used as synthetic precursors to overcome the limit of monomer precursor.
Conclusion
Concerted efforts have been made with the semiconductor clusters to identify their atomic information and elucidate the photophysical properties in relation to the structure. As a result, unique ligand-surface interactions that could not be identified in large CQDs through surface chemistry analysis have been revealed. In addition, high-quality CQDs that could not be obtained using conventional molecular precursors were synthesized by using a semiconductor cluster with suitable characteristics as a precursor. Therefore, semiconductor clusters are worth research efforts, not only for their own analysis but also for application as precursors.
Author contributions
JS, MC, MK, and SJ co-wrote the manuscript. All authors have approved the final version of the manuscript.
Funding
This research was supported by the Creative Materials Discovery Program through the National Research Foundation (NRF) of Korea (NRF-2019M3D1A1078299) and an NRF grant funded by the MSIT (NRF-2020M3H4A3081813, NRF-2021K1A3A1A21039697 and 2022M3H4A1A03076626).
Conflict of interest
The authors declare that the research was conducted in the absence of any commercial or financial relationships that could be construed as a potential conflict of interest.
Publisher’s note
All claims expressed in this article are solely those of the authors and do not necessarily represent those of their affiliated organizations, or those of the publisher, the editors and the reviewers. Any product that may be evaluated in this article, or claim that may be made by its manufacturer, is not guaranteed or endorsed by the publisher.
References
Bootharaju, M. S., Baek, W., Deng, G., Singh, K., Voznyy, O., Zheng, N., et al. (2022). Structure of a subnanometer-sized semiconductor Cd14Se13 cluster. Chem 8, 2978–2989. doi:10.1016/j.chempr.2022.06.025
Friedfeld, M. R., Stein, J. L., and Cossairt, B. M. (2017). Main-group-semiconductor cluster molecules as synthetic intermediates to nanostructures. Inorg. Chem. 56, 8689–8697. doi:10.1021/acs.inorgchem.7b00291
Gary, D. C., Flowers, S. E., Kaminsky, W., Petrone, A., Li, X., and Cossairt, B. M. (2016). Single-crystal and electronic structure of a 1.3 nm indium phosphide nanocluster. J. Am. Chem. Soc. 138, 1510–1513. doi:10.1021/jacs.5b13214
Gary, D. C., Terban, M. W., Billinge, S. J. L., and Cossairt, B. M. (2015). Two-step nucleation and growth of InP quantum dots via magic-sized cluster intermediates. Chem. Mat. 27, 1432–1441. doi:10.1021/acs.chemmater.5b00286
Hsieh, T. E., Yang, T. W., Hsieh, C. Y., Huang, S. J., Yeh, Y. Q., Chen, C. H., et al. (2018). Unraveling the structure of magic-size (CdSe)13 cluster pairs. Chem. Mat. 30, 5468–5477. doi:10.1021/acs.chemmater.8b02468
Kim, T., Park, S., and Jeong, S. (2021). Diffusion dynamics controlled colloidal synthesis of highly monodisperse InAs nanocrystals. Nat. Commun. 12, 3013. doi:10.1038/s41467-021-23259-w
Kwon, Y., Bang, G., Kim, J., Agnes, A., and Kim, S. (2020). Synthesis of InP branched nanostructures by controlling the intermediate nanoclusters. J. Mat. Chem. C Mat. 8, 1118–1124. doi:10.1039/c9tc05845a
Kwon, Y., and Kim, S. (2021). Indium phosphide magic-sized clusters: Chemistry and applications. NPG Asia Mat. 13, 37. doi:10.1038/s41427-021-00300-4
Kwon, Y., Oh, J., Lee, E., Lee, S. H., Agnes, A., Bang, G., et al. (2020). Evolution from unimolecular to colloidal-quantum-dot-like character in chlorine or zinc incorporated InP magic size clusters. Nat. Commun. 11, 3127–3215. doi:10.1038/s41467-020-16855-9
Nevers, D. R., Williamson, C. B., Hanrath, T., and Robinson, R. D. (2017). Surface chemistry of cadmium sulfide magic-sized clusters: A window into ligand-nanoparticle interactions. Chem. Commun. 53, 2866–2869. doi:10.1039/c6cc09549f
Ning, J., and Banin, U. (2017). Magic size InP and InAs clusters: Synthesis, characterization and shell growth. Chem. Commun. 53, 2626–2629. doi:10.1039/c6cc09778b
Palencia, C., Yu, K., and Boldt, K. (2020). The future of colloidal semiconductor magic-size clusters. ACS Nano 14, 1227–1235. doi:10.1021/acsnano.0c00040
Park, H. J., Shin, D. J., and Yu, J. (2021). Categorization of quantum dots, clusters, nanoclusters, and nanodots. J. Chem. Educ. 98, 703–709. doi:10.1021/acs.jchemed.0c01403
Park, N., Friedfeld, M. R., and Cossairt, B. M. (2022). Semiconductor clusters and their use as precursors to nanomaterials. Nanomater. via Single-Source Precursors Synthesis, Process. Appl. 2022, 165–200. doi:10.1016/B978-0-12-820340-8.00002-2
Ritchhart, A., and Cossairt, B. M. (2019). Quantifying ligand exchange on InP using an atomically precise cluster platform. Inorg. Chem. 58, 2840–2847. doi:10.1021/acs.inorgchem.8b03524
Rockenberger, J., Tröger, L., Kornowski, A., Vossmeyer, T., Eychmüller, A., Feldhaus, J., et al. (1997). EXAFS studies on the size dependence of structural and dynamic properties of CdS nanoparticles. J. Phys. Chem. B 101, 2691–2701. doi:10.1021/jp963266u
Tamang, S., Lee, S., Choi, H., and Jeong, S. (2016). Tuning size and size distribution of colloidal InAs nanocrystals via continuous supply of prenucleation clusters on nanocrystal seeds. Chem. Mat. 28, 8119–8122. doi:10.1021/acs.chemmater.6b03585
Tamang, S., Lincheneau, C., Hermans, Y., Jeong, S., and Reiss, P. (2016). Chemistry of InP nanocrystal syntheses. Chem. Mat. 28, 2491–2506. doi:10.1021/acs.chemmater.5b05044
Williamson, C. B., Nevers, D. R., Nelson, A., Hadar, I., Banin, U., Hanrath, T., et al. (2019). Chemically reversible isomerization of inorganic clusters. Science 363, 731–735. doi:10.1126/science.aau9464
Xie, L., Shen, Y., Franke, D., Sebastián, V., Bawendi, M. G., and Jensen, K. F. (2016). Characterization of indium phosphide quantum dot growth intermediates using MALDI-TOF mass spectrometry. J. Am. Chem. Soc. 138, 13469–13472. doi:10.1021/jacs.6b06468
Xie, R., Li, Z., and Peng, X. (2009). Nucleation kinetics vs chemical kinetics in the initial formation of semiconductor nanocrystals. J. Am. Chem. Soc. 131, 15457–15466. doi:10.1021/ja9063102
Keywords: semiconductor clusters, colloidal quantum dots, atomic information, optoelectronic properties, synthetic precursors
Citation: Shin J, Choi M, Kim M and Jeong S (2022) Semiconductor clusters: Synthetic precursors for colloidal quantum dots. Front. Nanotechnol. 4:1069178. doi: 10.3389/fnano.2022.1069178
Received: 13 October 2022; Accepted: 23 November 2022;
Published: 09 December 2022.
Edited by:
Brandi Cossairt, University of Washington, United StatesReviewed by:
Sungjee Kim, Pohang University of Science and Technology, South KoreaJunhua Yu, Seoul National University, South Korea
Copyright © 2022 Shin, Choi, Kim and Jeong. This is an open-access article distributed under the terms of the Creative Commons Attribution License (CC BY). The use, distribution or reproduction in other forums is permitted, provided the original author(s) and the copyright owner(s) are credited and that the original publication in this journal is cited, in accordance with accepted academic practice. No use, distribution or reproduction is permitted which does not comply with these terms.
*Correspondence: Sohee Jeong, cy5qZW9uZ0Bza2t1LmVkdQ==