- Department of Integrative Biology, School of Bio Sciences and Technology, Vellore Institute of Technology, Vellore, TN, India
Diseases have always been a disconcerting issue and have changed into being an inevitable member of the world’s population. Medical advancements have brought in improved treatments for particular ailments, but unfortunately those betterments have resulted in either side effects or turned out futile to a certain extent. The emergence of nanotechnology has considerably benefitted medical experts in disease diagnosis and therapeutics. Currently, an expansive range of nanoparticles is being explored for their effectiveness in therapies, and one among them is selenium nanoparticles (SeNPs). Nano-selenium exhibits significant properties which make it best suited for this purpose. The article highlights the key role of SeNPs in treating major diseases like cancer, diabetes, and microbial infections.
1 Introduction
“Nanotechnology is the sixth truly revolutionary technology introduced to the modern world.” This was a notable statement made by D. Allan Bromley, Former Assistant for Science and Technology, to the President of the United States and as days go past, advancement in nanotechnology is full steam ahead. This evolving technology intently stands to produce significant scientific and technological advances in diverse fields including food packaging and safety, cosmetics, healthcare, medicine, environment management, optoelectronics, chemical industries, drug delivery, space industries, and energy science. The basis of these applications lies in the unique magnetic, optical, structural, and chemical properties of nanometer-sized particles that are not exhibited by bulk substances (Yang et al., 2012). Currently, nanotechnology has updated its version to a new sphere of biology focusing on treatment and diagnosis of various ailments or other lethal diseases (Man et al., 2018; Beltrán-Gracia et al., 2019). It has acquired the potentiality to analyze biology from an in vitro and in vivo outlook. Several spectacular advancements have transpired in this field over the past years that elicits standard methodologies to synthesize nanosystems of particular size and form for essential needs. NPs incorporate exceptional functionalities to facilitate several biomedical applications like cancer therapy, treatment of microbial infections, diagnosis and treatment of diabetes, gene delivery, biosensing, and bioimaging. The large surface-to-volume ratio due to their small size makes them distinctive from bulk materials (Wong et al., 2013). When the particle size decreases to a certain extent, a large number of constituting atoms can be found around the surface of NPs, thereby making them highly reactive with exemplary physical properties. Numerous studies have brought out the unique properties of NPs like iron oxide (McNamara and Tofail, 2017), zinc oxide (Mirzaei and Darroudi, 2017), silver NPs (Rai et al., 2012), gold NPs (Elahi et al., 2018), quantum dots (Wagner et al., 2019), and nanorobots (Vega Baudrit and Villalobos, 2017) that play an inevitable role in curing diseases. The prime factors responsible for some of the life-threatening illnesses can either be specific pathogenic infections, unhealthy food habits, or the environment to which we are exposed. Amidst various ailments, cancer and its associated types are strikingly popular due to the massive death tolls it has generated for over a decade. A lack of appropriate diagnostic tools for early detection or the consequential side effects produced from radiation and chemical exposure as a part of cancer cell destruction are major issues perturbing populations globally. Correspondingly, diabetes is another disease that has startled people of all age groups. With a soar in the blood sugar level, patients are impelled to hold on to anti-diabetic regimens for a lifetime owing to the unavailability of appropriate therapeutic facilities. Similarly, an upsurge in cases entangling microbial infections is frequent with the victims eventually ending up consuming ineffective antidotes. The capability of pathogens to resist otherwise known as multidrug resistance has ultimately doomed the profitability of pharmaceutical firms. Selenium (Se) has been proved to be a crucial element performing undeniable tasks in mammalian life notably in disease treatment. The onset of nanotechnology and the introduction to nanomedicine have brought in even more supremacy to Se in a therapeutic perspective. SeNPs are widely utilized in medical sectors due to their smaller size, biocompatibility, lower toxicity, improved efficacy, and other remarkable features (Chhabria and Desai, 2016). This attractive carrier permits the delivery of therapeutics to specific sites of infection or disease, thereby dwindling the off-target drug toxicity eventually hindering the side effects. Depending on the synthesis method performed at specific pH, temperature, and concentration, the unique characteristics vary for each NP yielding particles of different shapes, sizes, and properties. SeNPs have emerged to be a powerful remedy, and in a situation where the world relies on alternatives for disease diagnosis and further expedition of treatment on finding the medical facets of these NPs are indispensable.
There are appreciably even more NPs that have marked their excellence in various biomedical applications. Due to the distinguishing properties, SeNPs have occupied a prime space in the research world. This narrow band gap (∼1.7 eV) semiconductor (Se) is massively used in solar cells, X-ray sensing, xerography, and rectifiers (Ingole et al., 2010). The article predominantly aims to discuss all about SeNPs and how their discovery has enlightened a new path to the treatment of cancer, microbial infections, and diabetes.
2 Selenium nanoparticles
Selenium discovered by Jons Jacob Berzelius is a vital micronutrient that has come to the fore in the field of medicine on account of their exclusive properties and is indispensable to human health. It is derived from the Greek word “selene” meaning moon and is discovered to have chemical properties similar to sulfur as well as analogy to tellurium. Being a semi-solid metal, it was found to be the by-product of sulfuric acid and is often recognized in the form of a red colored powder (Khurana et al., 2019). Se is also obtained as a by-product of copper refining and recovered from the anode slimes generated in the electrolytic production of copper. Scientists have proved Se deficiency to be one of the causative factors for Kashin–Beck disease (KBD). The European Food Safety Authority recommends a daily intake of Se by women (60 μg/day), men (70 μg/day), pregnant women (65 μg/day), and lactating women (75 μg/day) (Shi et al., 2021). This negatively charged trace element associates with at least 25 selenoproteins in the human body that take up different roles as an anti-oxidant, anti-inflammatory, anti-viral, anti-tumor agent, and so on (Boroumand et al., 2019). Moreover, it is a cofactor of anti-oxidant enzymes (glutathione peroxidase and thioredoxin reductase) that safeguards our human body from reactive oxygen species (ROS). It survives in the environment in different oxidation states (−2, 0, +4, and +6) and forms some of which include selenium methyl selenocysteine, mineral selenite, selenate, and selenomethionine (Wadhwani et al., 2016). Zero oxidation state Se is least toxic and less soluble among all other selenium compounds (Tran et al., 2019). They play a significant role in impeding adhesion of carcinogenic factors on to DNA along with inhibition of tumor growth and angiogenesis. Selenite possesses the highest toxicity amidst the various forms of Se. Surface decoration of nano-Se with carriers or ligands is reported to improve their selectivity and efficacy. Encapsulation of Se in carriers like chitosan can act as a proteinase inhibitor and also elevate immunity for the treatment of certain cancers, like fibrosarcoma and colorectal cancer (Shoeibi et al., 2017). A lack of Se can cause serious metabolic disorders like white muscle disease and can even have adverse effects on immune functionality. Although Se has marked its high excellence, it is highly noxious above a certain level and biologically inert for a long period of time which has ultimately dropped its high profile. For this reason, scientists have come up with the nano-form of Se as a solution.
SeNPs have displayed stupendous properties which have resulted in their maximum utilization for various crucial applications. They are biocompatible and exhibit excellent bioavailability, high affinity, biological activity, good permeability, and intestinal absorption, as well as anti-oxidant activities (Deng et al., 2017). It has been proved that these NPs have lower toxicity than inorganic Se and other organoselenium compounds (Chen et al., 2017). SeNPs are known for their capability to ameliorate selenoenzyme activity when administered orally. Compared to selenite, SeNPs have potent therapeutic effects mostly due to better ROS production in a dose-dependent manner. This is because only a single-step reduction from the elemental selenium atom to selenide anion is required to activate redox cycling with oxygen to produce ROS while multiple-step reduction is necessary for selenite (Zhao et al., 2018). SeNPs are normally unstable in the liquid phase and extremely easy to aggregate which results in the formation of gray or black selenium with a large particle size. This eventually leads to a major loss in their bioavailability and activity. Addition of stabilizing or capping agents (polysaccharides, proteins, and surfactants, etc.) can resolve this problem, thereby improving their stability. These agents even affect the surface properties of chemically produced NPs which predominantly includes surface charge. For example, Rohan Jain et al. elucidated the role of EPS (extracellular polymeric substances) in improving the overall colloidal stability of biogenic SeNPs and its effect on surface charge (Jain et al., 2015). Nowadays, SeNPs with a size less than 100 nm are effectively used as food additives (Liu et al., 2018). In addition, an increase in dimension can be noted in SeNPs with application of different heat treatments (Wang et al., 2015). The applications of these NPs are highly influenced by some of their features such as size, shape, structure, surface charge, and atomic arrangement. Depending on the chemicals and solvents used for their preparation, SeNPs are endowed with the physical property of forming different shapes. Hence, the crucial part lies in selecting a proper synthesis method to obtain SeNPs of desired qualities and effects.
2.1 Synthesis of SeNPs
There are numerous different routes through which SeNPs can be synthesized (Figure 1). Among them, the most common and studied are physical, chemical, and biological (green synthesis) methods.
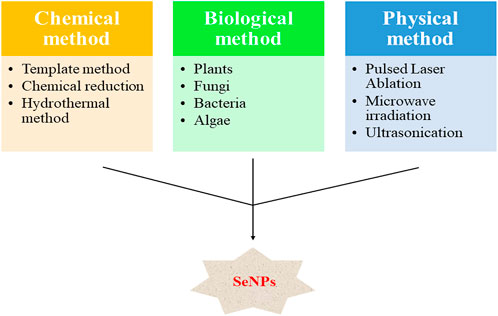
FIGURE 1. Various routes for the synthesis of SeNPs [created based on Chhabria and Desai (2016)].
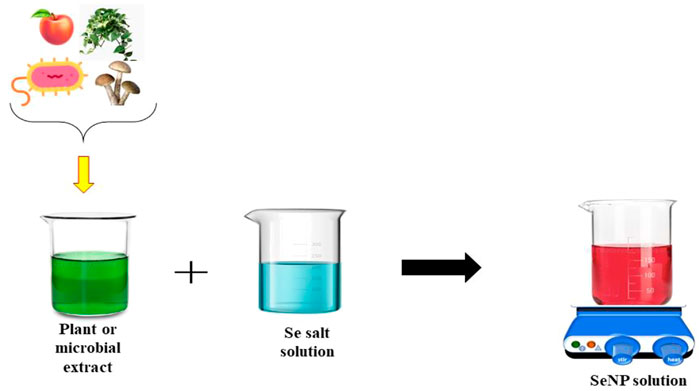
FIGURE 2. Schematic representation of the synthesis of SeNPs through biological routes [created based on Chhabria and Desai (2016)].
2.1.1 Chemical synthesis
In chemical synthesis of SeNPs, metallic precursors (sodium selenite, sodium selenosulfate, selenous acid, and selenium dioxide) and reducing agents (ascorbic acid, hydrazine, and sodium sulfite, etc.) are utilized for initiating the reaction.
The template method is one of the chemical methods used for the preparation of SeNPs where chemical templates that act as stabilizers are used. Lin et al. synthesized SeNPs with the aid of PEG200 that acted as a template as well as a surface decorator (Lin et al., 2021). Here, gray Se was dissolved in PEG200 solution for 15–20 min (210–220°C), and this was followed by the addition of water at a 1:1 ratio. Subsequently, the mixture was centrifuged for 10 min at 10,000 rpm and washed with Milli-Q water for about five times in order to remove excess PEG. Thus, a monodisperse, homogeneous spherical structure of PEG-SeNPs with an average diameter of 5 nm was obtained. Studies have also successfully explored various other chemicals that can be used as templates. These include folate, chitosan, ferulic acid, polyethylenimine (PEI), and hyaluronic acid. Another method to synthesize SeNP is a simple wet chemical method. For example, Langi et al. synthesized SeNPs by the reaction of sodium selenosulfate with 3-methylimidazolinium as an ionic-liquid (Langi et al., 2010). The reaction was carried out under ambient conditions in the presence of polyvinyl alcohol as a stabilizer in the aqueous medium, and spherical SeNPs of size ranging between 76 and 150 nm were produced. In most of the cases, sodium selenite (Na2SeO3) and ascorbic acid are the commonly used reagents in chemical methods. Shi et al. synthesized SeNP–polysaccharide nanocomposite via the chemical reduction method (Shi et al., 2021). Generally, for this preparation, a fixed volume of Na2SeO3 solution is added into the polysaccharide suspension, and then ascorbic acid is added into the mixture. Na2SeO3 and ascorbic acid are to be added dropwise under magnetic stirring in dark so as to prevent the formation of aggregates. The hydrothermal method has also been explored widely for the purpose of SeNP preparation. Researchers have reported the formation of SeNPs by the reduction of sodium selenite using the cellulose nanocrystal that directs the formation of NP and acts as a reducing agent under hydrothermal conditions. NPs with diameters ranging from 10 to 1000 nm can be produced by this method (Lin et al., 2021).
Although SeNPs of different sizes, shapes, and surface properties can be obtained, there are certain challenges that make chemical synthesis inappropriate. Environmental toxicity and instability of NPs are major drawbacks of this method that results in their low-bioactivity along with their precipitation. To improve the stability of NPs at times, polysaccharides like chitosan, carboxymethylcellulose, glucomannan, and acacia gum are added (Badgar, 2019; Shi et al., 2021). These stabilizing agents provide steric hindrance that averts agglomeration of prepared NPs.
2.1.2 Physical synthesis
Physical synthesis of SeNPs has less hazardous effects than the chemical method. A physical approach enables reduction in the size of particles through microwave irradiation, ultrasonication, hydrothermal method, and pulsed laser ablation (PLA).
The method of removing a substance from a surface by a laser pulse, the cause of which is the reaction of breaking polymer chains inside the irradiated volume due to a reaction activated by laser heating, is known as laser ablation. Scientists have reported the preparation of SeNPs via PLA in order to inhibit bacterial and fungal growth (Lin et al., 2021). The laser beam with fast pulse duration and repetition rate was directed to the surface of Se pellets in the bottom of a microcentrifuge tube. To obtain a more stable colloidal solution, the irradiation time is fixed at 15 min together with a wavelength of 355 nm. SeNPs have also been produced using 248 and 532 nm lasers where the NPs got deposited on to the substrates like glass, metallic gold films, and silicon wafers. In PLA, the size of the nanoparticle formed is controlled by laser parameters like pulse duration, power density, wavelength, and ambient gas conditions such as pressure, flow rate, and nature of gas. In addition to PLA, NPs are also widely synthesized by the microwave irradiation technique. Kalamuei et al. produced SeNPs through the microwave irradiation method under various conditions using selenium tetrachloride as a starting reagent and investigated its solar cell applications (Panahi-Kalamuei et al., 2014). The reaction mixture containing Se precursor (SeCl4), surfactant (SDS), and hydrazine was irradiated at 750 W. At a reaction time of 4 min, a color change to dark red was observed indicating the presence of SeNPs.
Thus, the physical method consumes less energy with the rapid reaction rate and time. It has become a much more economical method than chemical synthesis. Even though the physical technique generates ultra-small particles of uniform size, high cost and sophisticated instruments, especially for industrial production curtail their broad range of applications (Badgar, 2019; Alipour et al., 2021).
2.1.3 Biological synthesis
A biological route for the synthesis of Se has gained popularity in resolving major issues of both chemical and physical methods. Either plants or microorganisms like bacteria, fungi, and algae are the key players of biosynthesis, otherwise known as green synthesis. Green synthesis can produce environment-benign, biodegradable, cost-effective, and non-toxic SeNPs that are encapsulated by green material, which deters instant reactions of generated NPs from the surroundings (Figure 2). Srivastava and Mukhopadhyay synthesized SeNPs through non-pathogenic, Gram-negative bacterium Zooglea ramigera and observed a greater stability of produced biogenic SeNPs lasting for more than 6 months (Srivastava and Mukhopadhyay, 2013). The study proposed bacterial proteins to be responsible for this unique property. Also, Zhang et al. reported the biosynthesis of stable monoclinic SeNPs from Pseudomonas alcaliphila (Zhang et al., 2011). Among the biological alternatives, plants are considered to be a better option for the synthesis of NPs as it is relatively faster and there is no requirement of maintaining specific conditions in media and culture, which is a requisite for microorganisms. In addition, plant extracts contain enzymes, proteins, and other crucial phytochemicals (flavonoids, terpenoids, and alkaloids, etc.) which serve as reducing and capping agents. NPs produced via this route exhibit high catalytic ability that eventually activates greater toxicity in other disease-causing microbes and carcinomas. Several scientists and researchers have performed plant-mediated synthesis of SeNPs and worked on exploring their various applications. For example, P. Sowndarya et al. produced SeNPs through Clausena dentata plant leaf extracts and evaluated their insecticidal potential on different mosquito vectors (Sowndarya et al., 2016). It was found that the NPs displayed strong larvicidal activity on mosquitoes in a dose-dependent manner at low concentrations. A change in color from black to yellow from UV-vis spectra studies confirmed the synthesis of SeNPs.
Similarly, Anu et al. synthesized SeNPs via garlic cloves (Allium sativum) and investigated their cytotoxicity on Vero cell lines (Anu et al., 2016). Transformation of the solution mixture from colorless to brick red color confirmed the formation of SeNPs. Garlic cloves acted as a capping and reducing agent, and the results conveyed limited cytotoxicity of prepared green-synthesized SeNPs when compared to the chemically synthesized ones. In another study, SeNPs were synthesized from Aloe vera leaf extract, and their in vitro anti-microbial activity was estimated against spoilage fungi and pathogen bacterial strains (Fardsadegh and Jafarizadeh-Malmiri, 2019). The color of the SeNP solution changed from dark yellow to dark red, and synthesized NPs showed bactericidal effects on S. aureus and E. coli and fungicidal effects on P. digitatum and C. coccodes. Ramamurthy et al. performed green synthesis of SeNPs through fenugreek seed extract and elucidated the cytotoxicity of doxorubicin in combination with SeNPs on MCF-7 breast cancer cell lines (Wadhwani et al., 2017). It was concluded that this combination enhanced the anti-cancer effect on MCF-7 cell lines better than individual treatment. Recently, Cittrarasu et al. synthesized SeNPs via Ceropegia bulbosa tuber aqueous extract and analyzed their anti-microbial, cytotoxicity, mosquitocidal, and photocatalytic activities (Cittrarasu et al., 2021). The IC50 value of 34 μg/ml SeNPs was observed against MDA-MB-231 cells (breast cancer cell line) after 48 h, and further SeNPs showed growth inhibitory effects on B. subtilis and E. coli at different concentrations (25, 50, 75, and 100 μL/ml). Also, the histopathology results confirmed that after 24 h exposure, SeNPs displayed larvicidal activity at a concentration of 250 g/ml on Aedes albopictus mosquito larvae, and a 96% degradation result was observed approving the photocatalytic activity of SeNPs against methylene blue dye.
3 Therapeutic applications
3.1 Cancer therapy
Cancer has become a major threat in the modern world risking the lives of millions. Several treatments and advancements have been discovered to cure this disastrous disease, but then as a boon cancer cells have developed the ability of resistance eventually making the therapies futile (Manjunatha et al., 2021). Normally, chemotherapy is the most favored regimen for destruction of tumor cells, but the harmful chemicals used throughout the process have generated severe side effects creating vexation among the patients (Menon et al., 2018). A lack of effective cancer-targeting systems and occurrence of collateral damage are the major challenges faced by cancer researchers presently. The birth of nanotechnology has resolved these tribulations to an extent grabbing attention worldwide. It has been discovered that NPs of sizes ranging between 10 and 100 nm can penetrate deep into the tumor tissues and destroy cancer cells without affecting healthier ones, an effect termed as “enhanced permeation and retention” (Tran and Webster, 2011). Numerous nanosystems have been utilized productively for ameliorating targeting efficacy and suppressing cytotoxicity (Khurana et al., 2019). Amidst the efficient nanoparticle family, SeNPs have excelled in proving to be one of the best NPs available for cancer therapy. Menon et al. delineated the role of SeNPs as a powerful chemotherapeutic agent along with its mechanism (Menon et al., 2018). Their reports suggested that the activation of apoptotic pathways and cell cycle arrest were the actual mechanism behind the anti-cancer property of SeNPs. The compound Se was found to be cancer-causing and genotoxic at high concentrations, and further reports proved that the conjugation or surface modification of these NPs enhanced the anti-cancer effectiveness. Figure 3 illustrates the mechanism of action of SeNPs on cancer cells.
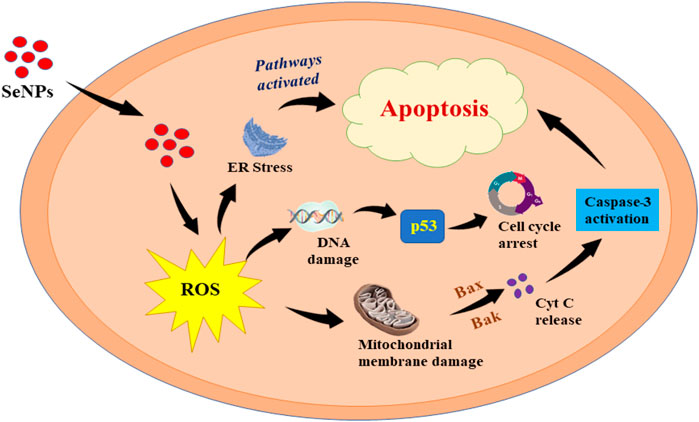
FIGURE 3. Action of SeNPs on cancer cells [modified based on Khurana et al. (2019)].
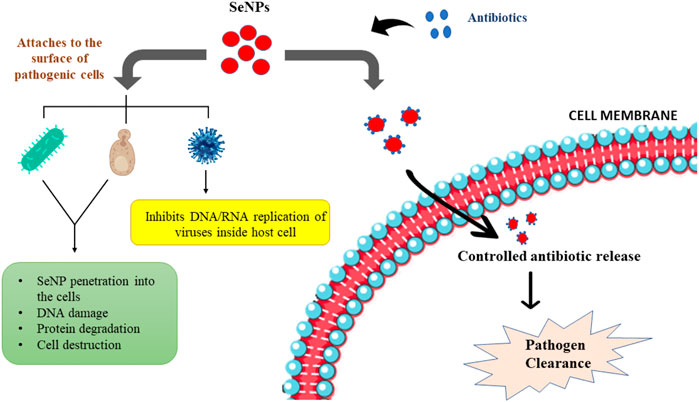
FIGURE 4. Anti-microbial action of SeNPs and the effect of SeNP-antibiotic combination in the infected body [modified based on Lin et al. (2021)].
Similarly, the effects of SeNPs on prostate LNCaP cancer cells were investigated by Kong et al. (2011). The prime mediator that facilitates prostate cancer progression is the androgen receptor (AR). Their results revealed that SeNPs induced significant growth inhibition of LNCaP cells through caspase-mediated apoptosis by destruction of ARs. Nano-Se downregulated the AR transcriptional activity in a dose-dependent manner, thereby causing rapid deterioration of AR through proteasome-dependent degradation by phosphorylation of Akt (also known as protein kinase B) at Ser 473 and Thr 308. A severe drop in the survival rate of LNCaP cancer cells was observed on the treatment with SeNPs with concentrations ranging from 1 μM to 100 μM. Liu et al. fabricated folate (FA)-conjugated SeNPs to target and destroy cancer cells with an ultimate objective to resolve multidrug resistance in cancer therapy (Liu et al., 2015). Cancer cells are multidrug resistant as a result of overexpression of P-glycoproteins (P-gp or ABCB1) and ATP-dependent active efflux pump in their plasma membrane. In their study, FA-conjugated SeNPs were designed as a nano-based drug delivery system for ruthenium polypyridyl (RuPOP) that exhibits the fluorescence property, which eventually permits direct imaging of the nanosystem inside the cancer cells. The work focused on R-HepG2 cells (liver cancer cell line), and it was reported that SeNPs averted multidrug resistance in R-HepG2 cells through ROS overproduction and activation of p53 and MAPK pathways, which induced apoptosis in cancer cells that in turn mitigated ABC family protein expression. Chen et al. studied the combined effect of SeNPs and radiation therapy on breast cancer cell lines (MCF-7 cells) in order to improve the therapy without harming normal cells (Chen et al., 2017). They concluded that SeNPs (act as a radiosensitizer) were not affected by radiation instead a greater concentration of intracellular Se ions was induced, leading to an increase in its toxicity by rapid generation of free radicals. Furthermore, combined treatment was found to be highly effective in causing cell death rather than irradiation alone.
Correspondingly, better production of ROS by SeNPs than selenite with the aid of thioredoxin or glutaredoxin-coupled glutathione systems was elucidated by Zhao et al. (2018). In addition, they analyzed a strong therapeutic effect being produced on a mouse model due to the accumulation of SeNPs in the cancer cells after intraperitoneal administration without triggering any toxic reactions. Moreover, the effects of size of SeNPs on cancer cells were determined by Wang et al. (2015). Small-sized SeNPs exhibited greater inhibition of cancer cell progression through the ROS-mediated system, and no side effects were observed on increasing selenoenzyme activities. Eventually, these activities truly relied on the glutathione (GSH) concentration, which could cause transformation of SeNPs. There are also certain reports that have interpreted the induction of cancer-targeted apoptosis by SeNPs as well as its selective cellular uptake (Huang et al., 2013). Additionally, biosynthesis of SeNPs using the cell protein of Acinetobacter sp. SW30 was proved to be selective against breast cancer cells and non-toxic to normal cells by Wadhwani et al. (2017). In addition, studies have identified the importance of functionalization of SeNPs as a critical step in cancer drug and gene delivery systems (Maiyo and Singh, 2017). Yang et al. well explained the functionalization of SeNPs with spirulina polysaccharides which further improved the cellular uptake and anti-cancer efficiency of NPs (Yang et al., 2012). There are various literature works that have pointed out the greater cytotoxicity of biogenic SeNPs on cancer cells than normal cells (Vahidi et al., 2019). Although several reports have come up with the anti-cancer mechanism of SeNPs, the exact process behind its action is still elusive, and more advanced research is indispensable for a successful outcome.
3.2 Anti-diabetic treatment
Diabetes mellitus (DM) has turned into a serious disease haunting people belonging to different age groups for over decades. Even though several medicines like sulfonylureas and biguanides are commercially available for diabetes treatment, severe side effects pop out as a result of its usage (Ferro et al., 2021). Some of these side effects include gastrointestinal distress, hypoglycemia, and pancreatic and liver damage. Researchers have discovered the brilliance of SeNPs in curing this dreadful disease to a certain extent. Liu et al. produced SeNPs by the addition of Catathelasma ventricosum polysaccharides (CVPs) to the redox system of selenite and ascorbic acid with an aim to evaluate the anti-diabetic potential of CVP-SeNP on STZ (streptozotocin)-induced diabetic mice (Liu et al., 2018). Streptozotocin causes type-2 diabetes through production of ROS that leads to oxidative damage of pancreatic β cells. The addition of polysaccharides to NPs helps in the modification of interface of NPs, controlling their growth, and eventually providing greater stability to the NP solution. Their study revealed that CVP-SeNPs synthesized under optimal conditions (ultrasonic time: 60 min s, reaction time: 2h, pH 7.0) were smaller and possessed high anti-oxidant activity. Furthermore, anti-oxidant enzyme activities, lipid levels, body weight, and blood sugar levels of STZ-induced diabetic mice were improved on exposure to CVP-SeNPs indicating its enhanced anti-diabetic activity. Ahmed et al. estimated the anti-diabetic activity of liposome-delivered SeNPs (L-Se) against type-2 DM in STZ-induced adult female albino rats of Wistar strain (Ahmed et al., 2016). In type-2 DM, oxidative stress is involved during the progression of β-cell dysfunction. A decrease in blood glucose and increase in serum insulin levels were observed on the treatment of diabetic rats with L-Se. The mechanism involved insulin-like action, and Se had the ability to restore β-cell activity along with increasing insulin release with a rapid decline in blood glucose levels. Low bioavailability, GI instability, and poor permeability have always been major setbacks of oral insulin delivery. Thus, Deng et al. worked on insulin-loaded SeNPs (INS-SeNPs) to overcome this issue (Zhao et al., 2018). INS-SeNPs were constructed by ionic cross-linking/in situ reduction and interestingly exhibited numerous benefits such as controlled insulin release and exceptional stability in digestive fluids. Since SeNPs showed good intestinal absorption, high affinity, and permeability, this advanced version curtailed oxidative stress, improved pancreatic islet function, and promoted glucose utilization. A hypoglycemic effect was even noticed when INS-SeNPs were treated on diabetic rats signifying its high anti-diabetic potentiality.
Likewise, Al-Quraishy et al. (2015) investigated the anti-hyperglycemic activity and atherogenic effects of SeNPs on STZ (55 mg/kg)-induced diabetic rats. A remarkable rise in insulin levels was noted in the diabetic rats treated with SeNPs alone or in combination with insulin, resulting in better glycemic control and prevention of their weight loss. Selenium has the ability to mimic insulin due to the activation of Akt and related kinases such as p70 S6 kinase of the insulin-signaling cascade. Additionally, GPx-1 overexpression protected STZ-induced diabetic rats from β-cell damage attributing to their hypoglycemic action. Thus, their study concluded that SeNPs reduced the risk of diabetic complications. Nowadays, diabetes during pregnancy otherwise known as gestational diabetes (G.D) and its effects on the offsprings have evolved to be a severe concern among women. Hassan et al. examined the effect of SeNPs in curing G.D by experimenting on diabetic rats and their offsprings, thereby understanding the role of these NPs in curtailing diabetic nephropathy and pancreatopathy (Hassan et al., 2021). The results of their findings showed that SeNPs prevented the transmission of G.D. to the offsprings offering protection from diabetic-related complications. Also, these NPs balanced oxidative stress and inflammation-mediated diabetic condition of the mother which strongly signifies their greater anti-diabetic and anti-oxidant properties. Kumar et al. examined the role of SeNPs in treating type 1 diabetic nephropathy (DN) using STZ-injected Sprague–Dawley (SD) rats as a model (Kumar et al., 2014). Increased plasma creatinine and blood urea nitrogen (BUN) levels indicate the progression of DN. Treatment of rats with SeNPs leads to a massive decrease in plasma creatinine and BUN levels along with an increase in albumin. The overexpression of HSP-70 (cytoprotective protein) and SIRT-1 (longevity protein) was noticed on treatment with SeNPs which eventually led to a reduction in oxidative damage, thereby impeding type 1 DN progression. In addition, SeNPs lowered apoptosis by altering Bcl-2 and Bax expression. In another research, Zeng et al. produced and analyzed the anti-diabetic properties of chitosan-stabilized SeNPs (CTS-SeNPs) (Zeng et al., 2018). Results suggested that the synthesized CTS-SeNPs (54 nm) were uniformly spherical with a stability lasting for 60 days at 4°C and also exhibited high anti-diabetic activity at a dose of 2 mg in comparison to other selenium compounds when experimented on STZ-injected male ICR (Institute of Cancer Research, United States) mice. Various studies on the anti-diabetic property of SeNPs are elicited in different literature works, but the exact mechanism of how the NP actually showcases this potency is still unknown and limited data are available for which more research is a requisite.
3.3 Anti-microbial treatment
Microbes have always posed peril to public health causing severe infectious diseases that are fatal (Chick et al., 2001). These disease-causing microorganisms also known as pathogens even contribute to different non-infectious long-term diseases including some forms of cancer and coronary heart disease. Most commonly known microbes are bacteria, fungi, virus, protozoan, and algae inducing different infections on the respiratory tract, gastrointestinal tract, urogenital tract, and skin, etc. These microbes once entered the body, attach themselves to the target site within the body, rapidly multiply utilizing host nutrients, and survive inside weakening the host immune system (Zachary, 2017). Table 1 lists out some of the diseases and their respective causative agents.
Albeit numerous antibiotics and specific drugs are commercially available for the treatment of microbial infections, these have become least effective as the microbes have developed multidrug resistance. Nanotechnology has displayed its distinctive anti-microbial properties, and SeNPs are one among the top list of nanomaterials. Reports have demonstrated the exemplary features of SeNPs that enable them to be a powerful anti-microbial agent (Figure 4). Lin et al. worked on fabricating bacteria-responsive biomimetic Se nanosystem that can detect and inhibit methicillin-resistant Staphylococcus aureus (MRSA) infections (Lin et al., 2019). This was accomplished with an aid of a combined scheme using Ru-SeNP complex, natural red blood cell membrane (RBCM), and bacteria-responsive gelatin NPs (GNPs) eventually forming an effective Ru-Se@GNP-RBCM nanosystem that can be an alternative to antibiotics. Ruthenium with its greater fluorescence on combining with SeNPs provided real-time imaging ultimately sustaining anti-bacterial activity. GNPs with good biocompatibility and degradability were excellent drug delivery vehicles, while RBCM strictly combated bacteria. It was suggested that Ru-Se@GNP-RBCM produced ROS in situ after rupture of the bacterial membrane system, leading to leakage of contents, thereby killing the bacteria. Tran et al. performed a work using SeNPs as an anti-infective coating for titanium orthopedic implants against drug-resistant bacteria like MRSA and MRSE (methicillin-resistant Staphylococcus epidermidis) (Tran et al., 2019). This efficacy was evaluated on a rat femur model, and the NPs were immobilized onto the implants using the surface-induced nucleation-deposition method. SeNP coating had the potential to generate ROS damaging cellular constituents, disrupt microbial membranes, interrupt transmembrane transport processes, inhibit enzyme activity, and damage microbial DNA. A significant decline in bacteria was observed on treatment with SeNPs at a Se concentration as low as 0.5 ppm, and further this coating acted as an impediment without losing its activity preventing biofilm formation leading to bacterial death. Similarly, Huang and co-workers explored the anti-bacterial activity of SeNPs synergistically conjugated with quercetin (Qu) and acetylcholine (Ach) [Qu-Ach@SeNPs] against multidrug-resistant superbugs (MDRs) (Huang et al., (2016). The bactericidal compound Qu exhibited potent anti-bacterial action, while the neurotransmitter Ach interacted with Ach receptors on bacterial cell membranes increasing permeability, thereby damaging and killing the bacteria. A soar in anti-bacterial efficiency was observed in Qu-Ach@SeNPs at a low dose when compared to Qu@SeNPs and Ach@SeNPs treatment alone, and it was suggested that this anti-bacterial activity was connected to the generation of ROS in bacteria. Moreover, no emergence of resistance was found on exposure to Qu-Ach@SeNPs. The cytotoxicity study of produced Qu-Ach@SeNPs on HEK293T (human embryonic kidney cells) showed no significant effects proving their low cytotoxicity.
Tran et al. analyzed the potentiality of SeNPs against the growth of Gram-positive Staphylococcus aureus (Tran and Webster, 2011). It was observed that in comparison to control (without SeNPs), the bacterial growth was suppressed approximately 20 times after 3 h, 50 times after 4 h, and 60 times after 5 h of exposure to SeNPs at different concentrations (7.8, 15.5, and 31 μg/ml, respectively). Furthermore, the percentage of live bacteria reduced on SeNP treatment at each point of time indicating that SeNP had more potential in killing rather than inhibiting S. aureus growth. Thus, their study brought out SeNP as a perfect medication for S. aureus infections. Fillipovic et al. compared the anti-microbial activity of three different formulations of SeNPs with different surface chemistry and structure on eight standard bacterial strains and one yeast strain (S. aureus, E. faecalis, B. subtilis, K. rhizophila, E. coli, S. abony, K. pneumoniae, and P.aeruginosa and C.albicans) (Filipović et al., 2021). Using a simple chemical reduction method, SeNPs of size ranging between 75 and 200 nm were produced, and the results revealed C.albicans to be the most sensitive toward SeNPs among all the chosen organisms with MIC values of 25 and 72 μg/ml. Moreover, positive surface charge of SeNPs exhibited superior anti-microbial activity specifically against C. albicans, and Gram-positive ones showed higher activity out of all bacterial strains used. Menon et al. generated SeNPs from cow urine extract (a green chemistry approach), statistically optimized the produced NPs and investigated its anti-microbial activities (Menon et al., 2020). Cow-urine was observed to have diverse therapeutic applications which include the treatment of cardiac diseases, diarrhea, edema, jaundice, and hemorrhoids due to the presence of 2.5% urea and 24 different salts along with other essential minerals within it. Optimization using the Box–Behnken method boosted the production and yield of SeNPs, thereby enhancing its application in various biomedical fields. It was concluded that SeNPs exhibited elevated anti-bacterial activity against Klebsiella species on account of ROS production, leading to destruction of the phospholipid bilayer that enabled NPs to inactivate surface functional groups eventually causing cell death. The anti-viral effects of SeNPs have also been investigated widely. For example, studies have explored the effectiveness of SeNPs in fighting hepatitis, H1N1 influenza, enterovirus, and SARS-COV-2 virus infections (Mal’tseva et al., 2022; Lin et al., 2021). In addition, SeNPs have unique anti-fungal and anti-parasitic properties racking up its treatment potential to a greater extent. At a very low dosage, SeNPs were capable of inducing 50% suppression of Candida albicans biofilm after adhering on to the biofilm, penetrating and disrupting the fungi cell structure. Scientists have also reported the powerful activity of SeNPs on inhibiting Colletotrichum capsici and Alternaria solani. When focusing on to their anti-parasitic properties, SeNPs are proven to be strong cytotoxic agents against promastigote and amastigote forms of Leishmania (Lin et al., 2021). However, their in vivo efficacy is to be explored yet. Though several works have reported stating ROS generation to be the mechanism behind the anti-microbial property of SeNPs, the exact cause is obscure. Further advanced research is the crucial need of the hour, so that novel therapeutics involving SeNPs to fight antibiotic resistance within microorganisms could actually be an asset worldwide.
4 Conclusion and future prospects
Selenium has attracted attention globally due to their peerless facets. This review focused to elicit the importance of nano-Se in the biomedical domain particularly in the treatment of cancer, diabetes, and microbial infections. Studies have fortunately proved the vital role taken up by Se and its nano-forms in therapeutics due to their extra special physical and chemical properties. The ability of SeNPs to generate ROS and destroy the tumor cells, microbes, and other noxious agents is reckoned to be the prime source of origin behind its aforementioned properties. Despite the literature works, an even more exploration is further needed to acquire more information on the exact process behind the anti-cancer, anti-diabetic, and anti-microbial properties possessed by SeNPs. As per the research reports and other data collected, these amazing properties make SeNPs unparalleled and best suited for a wide variety of several other therapeutic functions.
The mechanism of SeNPs varies based on the preparation methods, targeting moieties used, and loadings applied. A prior focus on physicochemical parameters like salt concentration, temperature, pH, aeration, and reaction time plays an integral role in producing the top versions of this unique NP. In addition, factors including NP size, shape, composition, surface property, and dispersion are to be analyzed to have an insight into NP. Targeted drug delivery of SeNPs can be considered a better option in treating various dreadful diseases. However, toxicity remains a challenge. Thus, studies prioritizing the long-term toxicity of SeNPs must be extensively carried out to ensure safety before application to humans. Implementation of biogenic SeNPs would be a more promising, cost-effective, and safer approach due to their lower toxicity than the synthetic ones. Biogenic synthesis and controlled release of SeNPs should be the primary concerns of treatment regimens. Once SeNPs are approved free from extended toxicity, then the next trials of pre-clinical and clinical phases can be accomplished that is utmost required in the near future to have an in-depth knowledge of its therapeutic effect on humans and research focusing more on this aspect can be a breakthrough. The development of biogenic and biocompatible Se nanorobots capable of eliminating major diseases could be a novel Se form that can be worked upon in the future and can be a milestone for medical research. A better understanding of NP characteristics, their interactions with the biological environment-like receptors, or the mechanism of action in specific diseases would immensely help in overcoming major limitations and establish novel strategies to deal with both treatable and untreatable ailments. The metabolism of SeNPs in the biological system is another criterion that needs to be looked upon closely as reports are obscure. Functionalization, encapsulation, and developing SeNPs with different combinations of drugs (combination therapy) can be even more explored to sort out the best ones out of these techniques. Lipid-based formulations of SeNPs could also be implemented since they are capable to cross the hard-to-reach sites even without any surface functionalization. This can be considered a benchmark for next generation therapeutics. Biogenic SeNPs that aim at early detection of cancer and permanently eliminates diabetes and microbial infections in less time is the ultimate goal henceforth.
Author contributions
DK: writing—original draft and writing—review and editing. SV: conceptualization, editing, and supervision.
Acknowledgments
The authors would like to sincerely acknowledge the support offered by the Vellore Institute of Technology toward the study.
Conflict of interest
The authors declare that the research was conducted in the absence of any commercial or financial relationships that could be construed as a potential conflict of interest.
Publisher’s note
All claims expressed in this article are solely those of the authors and do not necessarily represent those of their affiliated organizations, or those of the publisher, the editors, and the reviewers. Any product that may be evaluated in this article, or claim that may be made by its manufacturer, is not guaranteed or endorsed by the publisher.
References
Abo-Zeid, Y., Williams, G., Touabi, L., and McLean, G. (2020). An investigation of rhinovirus infection on cellular uptake of poly (glycerol-adipate) nanoparticles. Int. J. Pharm. X. 589, 119826. doi:10.1016/j.ijpharm.2020.119826
Ahmed, H., Abd El-Maksoud, M., Abdel Moneim, A., and Aglan, H. (2016). Pre-clinical study for the antidiabetic potential of selenium nanoparticles. Biol. Trace Elem. Res. 177 (2), 267–280. doi:10.1007/s12011-016-0876-z
Al-Quraishy, S., Dkhil, M., and Abdel Moneim, A. (2015). Anti-hyperglycemic activity of selenium nanoparticles in streptozotocin-induced diabetic rats. Int. J. Nanomedicine 10, 6741–6756. doi:10.2147/IJN.S91377
Alipour, S., Kalari, S., Morowvat, M., Sabahi, Z., and Dehshahri, A. (2021). Green synthesis of selenium nanoparticles by cyanobacterium spirulina platensis (abdf2224): Cultivation condition quality controls. Biomed. Res. Int. 2021, 1–11. doi:10.1155/2021/6635297
Anu, K., Singaravelu, G., Murugan, K., and Benelli, G. (2016). Green-synthesis of selenium nanoparticles using garlic cloves (allium sativum): Biophysical characterization and cytotoxicity on Vero cells. J. Clust. Sci. 28 (1), 551–563. doi:10.1007/s10876-016-1123-7
Badgar, K. (2019). The synthesis of selenium nаnоpаrtiсle (SeNPs) – Review. Acta Agrar. debr. (1), 5–8. doi:10.34101/actaagrar/1/2359
Beltrán-Gracia, E., López-Camacho, A., Higuera-Ciapara, I., Velázquez-Fernández, J., and Vallejo-Cardona, A. (2019). Nanomedicine review: Clinical developments in liposomal applications. Cancer Nanotechnol. 10 (1), 11. doi:10.1186/s12645-019-0055-y
Boroumand, S., Safari, M., Shaabani, E., Shirzad, M., and Faridi-Majidi, R. (2019). Selenium nanoparticles: Synthesis, characterization and study of their cytotoxicity, antioxidant and antibacterial activity. Mat. Res. Express 6 (8), 0850d8. doi:10.1088/2053-1591/ab2558
Chen, F., Zhang, X., Hu, X., Liu, P., and Zhang, H. (2017). The effects of combined selenium nanoparticles and radiation therapy on breast cancer cells in vitro. Artif. Cells Nanomed. Biotechnol. 46 (5), 937–948. doi:10.1080/21691401.2017.1347941
Chhabria, S., and Desai, K. (2016). Selenium nanoparticles and their applications. Encycl. Nanosci. Nanotechnol. 20, 1–32. https://www.researchgate.net/publication/307606511.
Chick, S., Koopman, J., Soorapanth, S., and Brown, M. (2001). Infection transmission system models for microbial risk assessment. Sci. Total Environ. 274 (1-3), 197–207. doi:10.1016/s0048-9697(01)00749-5
Cittrarasu, V., Kaliannan, D., Dharman, K., Maluventhen, V., Easwaran, M., Chao Liu, W., et al. (2021). Green synthesis of selenium nanoparticles mediated from Ceropegia bulbosa Roxb extract and its cytotoxicity, antimicrobial, mosquitocidal and photocatalytic activities. Sci. Rep. 11 (1), 1032. doi:10.1038/s41598-020-80327-9
Deng, W., Xie, Q., Wang, H., Ma, Z., Wu, B., and Zhang, X. (2017). Selenium nanoparticles as versatile carriers for oral delivery of insulin: Insight into the synergic antidiabetic effect and mechanism. Nanomedicine Nanotechnol. Biol. Med. 13 (6), 1965–1974. doi:10.1016/j.nano.2017.05.002
Elahi, N., Kamali, M., and Baghersad, M. (2018). Recent biomedical applications of gold nanoparticles: A review. Talanta 184, 537–556. doi:10.1016/j.talanta.2018.02.088
Elmi, T., Ardestani, M., Hajialiani, F., Motevalian, M., Mohamadi, M., Sadeghi, S., et al. (2020). Novel chloroquine loaded curcumin based anionic linear globular dendrimer G2: A metabolomics study on Plasmodium falciparum in vitro using 1H NMR spectroscopy. Parasitology 147 (7), 747–759. doi:10.1017/S0031182020000372
Fardsadegh, B., and Jafarizadeh-Malmiri, H. (2019). Aloe vera leaf extract mediated green synthesis of selenium nanoparticles and assessment of their in vitro antimicrobial activity against spoilage fungi and pathogenic bacteria strains. Green process. Synth. 8 (1), 399–407. doi:10.1515/gps-2019-0007
Ferro, C., Florindo, H., and Santos, H. (2021). Selenium nanoparticles for biomedical applications: From development and characterization to therapeutics. Adv. Healthc. Mat. 10 (16), 2100598. doi:10.1002/adhm.202100598
Filipović, N., Ušjak, D., Milenković, M., Zheng, K., Liverani, L., Boccaccini, A., et al. (2021). Comparative study of the antimicrobial activity of selenium nanoparticles with different surface chemistry and structure. Front. Bioeng. Biotechnol. 8, 624621. doi:10.3389/fbioe.2020.624621
Forbes, J. (2020). Clinically important toxins in bacterial infection: Utility of laboratory detection. Clin. Microbiol. Newsl. 42 (20), 163–170. doi:10.1016/j.clinmicnews.2020.09.003
Hassan, I., Ebaid, H., Al-Tamimi, J., Habila, M., Alhazza, I., and Rady, A. (2021). Selenium nanoparticles mitigate diabetic nephropathy and pancreatopathy in rat offspring via inhibition of oxidative stress. J. King Saud Univ. - Sci. 33 (1), 101265. doi:10.1016/j.jksus.2020.101265
Huang, X., Chen, X., Chen, Q., Yu, Q., Sun, D., and Liu, J. (2016). Investigation of functional selenium nanoparticles as potent antimicrobial agents against superbugs. Acta Biomater. 30, 397–407. doi:10.1016/j.actbio.2015.10.041
Huang, Y., He, L., Liu, W., Fan, C., Zheng, W., Wong, Y., et al. (2013). Selective cellular uptake and induction of apoptosis of cancer-targeted selenium nanoparticles. Biomaterials 34 (29), 7106–7116. doi:10.1016/j.biomaterials.2013.04.067
Ingole, A., Thakare, S., Khati, N., Wankhade, A., and Burghate, D. (2010). Green synthesis of selenium nanoparticles under ambient condition. Chalcogenide Lett. 7 (7), 485–489. https://www.researchgate.net/publication/268198258.
Jain, R., Jordan, N., Weiss, S., Foerstendorf, H., Heim, K., Kacker, R., et al. (2015). Extracellular polymeric substances govern the surface charge of biogenic elemental selenium nanoparticles. Environ. Sci. Technol. 49 (3), 1713–1720. doi:10.1021/es5043063
Kaur, R., Kaur, R., Singh, C., Kaur, S., Goyal, A., Singh, K., et al. (2019). Inhalational drug delivery in pulmonary aspergillosis. Crit. Rev. Ther. Drug Carr. Syst. 36 (3), 183–217. doi:10.1615/CritRevTherDrugCarrierSyst.2018025781
Khurana, A., Tekula, S., Saifi, M., Venkatesh, P., and Godugu, C. (2019). Therapeutic applications of selenium nanoparticles. Biomed. Pharmacother. 111, 802–812. doi:10.1016/j.biopha.2018.12.146
Kong, L., Yuan, Q., Zhu, H., Li, Y., Guo, Q., Wang, Q., et al. (2011). The suppression of prostate LNCaP cancer cells growth by Selenium nanoparticles through Akt/Mdm2/AR controlled apoptosis. Biomaterials 32 (27), 6515–6522. doi:10.1016/j.biomaterials.2011.05.032
Kumar, G., Kulkarni, A., Khurana, A., Kaur, J., and Tikoo, K. (2014). Selenium nanoparticles involve HSP-70 and SIRT1 in preventing the progression of type 1 diabetic nephropathy. Chem. Biol. Interact. 223, 125–133. doi:10.1016/j.cbi.2014.09.017
Langi, B., Shah, C., Singh, K., Chaskar, A., Kumar, M., and Bajaj, P. (2010). Ionic liquid-induced synthesis of selenium nanoparticles. Mater.Res. Bull. 45 (6), 668–671. doi:10.1016/j.materresbull.2010.03.005
Lin, A., Liu, Y., Zhu, X., Chen, X., Liu, J., Zhou, Y., et al. (2019). Bacteria- responsive biomimetic selenium nanosystem for multidrug-resistant bacterial infection detection and inhibition. ACS Nano 13 (12), 13965–13984. doi:10.1021/acsnano.9b05766
Lin, W., Zhang, J., Xu, J., and Pi, J. (2021). The advancing of selenium nanoparticles against infectious diseases. Front. Pharmacol. 12, 682284. doi:10.3389/fphar.2021.682284
Liu, T., Zeng, L., Jiang, W., Fu, Y., Zheng, W., and Chen, T. (2015). Rational design of cancer-targeted selenium nanoparticles to antagonize multidrug resistance in cancer cells. Nanomedicine Nanotechnol. Biol. Med. 11 (4), 947–958. doi:10.1016/j.nano.2015.01.009
Liu, Y., Zeng, S., Liu, Y., Wu, W., Shen, Y., Zhang, L., et al. (2018). Synthesis and antidiabetic activity of selenium nanoparticles in the presence of polysaccharides from Catathelasma ventricosum. Int. J. Biol. Macromol. 114, 632–639. doi:10.1016/j.ijbiomac.2018.03.161
Maiyo, F., and Singh, M. (2017). Selenium nanoparticles: Potential in cancer gene and drug delivery. Nanomedicine 12 (9), 1075–1089. doi:10.2217/nnm-2017-0024
Mal’tseva, V., Goltyaev, M., Turovsky, E., and Varlamova, E. (2022). Immunomodulatory and anti-inflammatory properties of selenium-containing agents: Their role in the regulation of defense mechanisms against COVID-19. Int. J. Mol. Sci. 23 (4), 2360. doi:10.3390/ijms23042360
Man, F., Lammers, T., and de Rosales, R. T. M. (2018). Imaging nanomedicine-based drug delivery: A review of clinical studies. Mol. Imaging Biol. 20 (5), 683–695. doi:10.1007/s11307-018-1255-2
Manjunatha, C., Rao, P., Bhardwaj, P., Raju, H., and Ranganath, D. (2021). New insight into the synthesis, morphological architectures and biomedical applications of elemental selenium nanostructures. Biomed. Mat. 16, 02201, doi:10.1088/1748-605X/abc026
McNamara, K., and Tofail, S. (2017). Nanoparticles in biomedical applications. Adv. Phys. X 2 (1), 54–88. doi:10.1080/23746149.2016.1254570
Menon, S., Agarwal, H., Rajeshkumar, S., Rosy, P., and Shanmugam, V. (2020). Investigating the antimicrobial activities of the biosynthesized selenium nanoparticles and its statistical analysis. Bionanoscience 10 (1), 122–135. doi:10.1007/s12668-019-00710-3
Menon, S., Devi Ks, S., R, S., S, R., and Kumar S, V. (2018). Selenium nanoparticles: A potent chemotherapeutic agent and an elucidation of its mechanism. Colloids Surf. B Biointerfaces 170, 280–292. doi:10.1016/j.colsurfb.2018.06.006
Mirzaei, H., and Darroudi, M. (2017). Zinc oxide nanoparticles: Biological synthesis and Biomedical applications. Ceram. Int. 43 (1), 907–914. doi:10.1016/j.ceramint.2016.10.051
Müller, C., Glamuzina, B., Pozniak, I., Weber, K., Cialla, D., Popp, J., et al. (2014). Amnesic shellfish poisoning biotoxin detection in seawater using pure or amino- functionalized Ag nanoparticles and SERS. Talanta 130, 108–115. doi:10.1016/j.talanta.2014.06.059
Panahi-Kalamuei, M., Salavati-Niasari, M., and Hosseinpour-Mashkani, S. (2014). Facile microwave synthesis, characterization, and solar cell application of selenium nanoparticles. J. Alloys Compd. 617, 627–632. doi:10.1016/j.jallcom.2014.07.174
Rai, M., Deshmukh, S., Ingle, A., and Gade, A. (2012). Silver nanoparticles: The powerful nano weapon against multidrug-resistant bacteria. J. Appl. Microbiol. 112 (5), 841–852. doi:10.1111/j.1365-2672.2012.05253.x
Shi, X., Tian, Y., Wu, J., and Wang, S. (2021). Synthesis, characterization, and biological activity of selenium nanoparticles conjugated with polysaccharides. Crit. Rev. Food Sci. Nutr. 61 (13), 2225–2236. doi:10.1080/10408398.2020.1774497
Shoeibi, S., Mozdziak, P., and Golkar-Narenji, A. (2017). Biogenesis of selenium nanoparticles using green chemistry. Top. Curr. Chem. 375 (6), 88–21. doi:10.1007/s41061-017-0176-x
Singh, L., Kruger, H., Maguire, G., Govender, T., and Parboosing, R. (2017). The role of nanotechnology in the treatment of viral infections. Ther. Adv. Infect. Dis. 4 (4), 105–131. doi:10.1177/2049936117713593
Sousa, F., Ferreira, D., Reis, S., and Costa, P. (2020). Current insights on antifungal therapy: Novel nanotechnology approaches for drug delivery systems and new drugs from natural sources. Pharmaceuticals 13 (9), 248. doi:10.3390/ph13090248
Sowndarya, P., Ramkumar, G., and Shivakumar, M. (2016). Green synthesis of selenium nanoparticles conjugated Clausena dentata plant leaf extract and their insecticidal potential against mosquito vectors. Artif. Cells Nanomed. Biotechnol. 45 (8), 1490–1495. doi:10.1080/21691401.2016.1252383
Srivastava, N., and Mukhopadhyay, M. (2013). Biosynthesis and structural characterization of selenium nanoparticles mediated by Zooglea ramigera. Powder Technol. 244, 26–29. doi:10.1016/j.powtec.2013.03.050
Tran, P., Simpson, N., Palmer, J., Bock, N., Reynolds, E., Webster, T., et al. (2019). Selenium nanoparticles as anti-infective implant coatings for trauma orthopedics against methicillin-resistant Staphylococcus aureus and epidermidis: In vitro and in vivo assessment. Int. J. Nanomedicine 14, 4613–4624. doi:10.2147/IJN.S197737
Tran, P., and Webster, T. (2011). Selenium nanoparticles inhibit Staphylococcus aureus growth. Int. J. Nanomedicine 6, 1553–1558. doi:10.2147/IJN.S21729
Vahidi, H., Barabadi, H., and Saravanan, M. (2019). Emerging selenium nanoparticles to combat cancer: A systematic review. J. Clust. Sci. 31 (2), 301–309. doi:10.1007/s10876-019-01671-z
Vega Baudrit, J., and Villalobos, C. (2017). Nanobots: Development and future. Int. J. Biosens. Bioelectron. 2 (5). doi:10.15406/ijbsbe.2017.02.00037
Vivancos, V., González-Alvarez, I., Bermejo, M., and Gonzalez-Alvarez, M. (2018). Giardiasis: Characteristics, pathogenesis and new insights about treatment. Curr. Top. Med. Chem. 18 (15), 1287–1303. doi:10.2174/1568026618666181002095314
Wadhwani, S., Gorain, M., Banerjee, P., Shedbalkar, U., Singh, R., Kundu, G., et al. (2017). Green synthesis of selenium nanoparticles using Acinetobacter sp. SW30: Optimization, characterization and its anticancer activity in breast cancer cells. Int. J. Nanomedicine 12, 6841–6855. doi:10.2147/IJN.S139212
Wadhwani, S., Shedbalkar, U., Singh, R., and Chopade, B. (2016). Biogenic selenium nanoparticles: Current status and future prospects. Appl. Microbiol. Biotechnol. 100 (6), 2555–2566. doi:10.1007/s00253-016-7300-7
Wagner, A., Knipe, J., Orive, G., and Peppas, N. (2019). Quantum dots in biomedical applications. Acta Biomater. 94, 44–63. doi:10.1016/j.actbio.2019.05.022
Wang, Y., Chen, P., Zhao, G., Sun, K., Li, D., Wan, X., et al. (2015). Inverse relationship between elemental selenium nanoparticle size and inhibition of cancer cell growth in vitro and in vivo. Food Chem. Toxicol. 85, 71–77. doi:10.1016/j.fct.2015.08.006
Wang, Y., Qi, J., Cao, H., and Liu, C. (2021). Immune responses to varicella-zoster virus glycoprotein E formulated with poly (Lactic-co-Glycolic acid) nanoparticles and nucleic acid adjuvants in mice. Virol. Sin. 36 (1), 122–132. doi:10.1007/s12250-020-00261-y
Wong, I., Bhatia, S., and Toner, M. (2013). Nanotechnology: Emerging tools for biology and medicine. Genes Dev. 27 (22), 2397–2408. http://www.genesdev.org/cgi/doi/10.1101/gad.226837.113.
Yang, F., Tang, Q., Zhong, X., Bai, Y., Chen, T., Zhang, Y., et al. (2012). Surface decoration by Spirulina polysaccharide enhances the cellular uptake and anticancer efficacy of selenium nanoparticles. Int. J. Nanomedicine 7, 835–844. doi:10.2147/IJN.S28278
Zachary, J., Mechanisms of microbial infections, Pathologic basis of veterinary disease, (2017) p.132.
Zeng, S., Ke, Y., Liu, Y., Shen, Y., Zhang, L., Li, C., et al. (2018). Synthesis and antidiabetic properties of chitosan-stabilized selenium nanoparticles. Colloids Surfaces B Biointerfaces 170, 115–121. doi:10.1016/j.colsurfb.2018.06.003
Zhang, W., Chen, Z., Liu, H., Zhang, L., Gao, P., and Li, D. (2011). Biosynthesis and structural characteristics of selenium nanoparticles by Pseudomonas alcaliphila. Colloids Surfaces B Biointerfaces 88 (1), 196–201. doi:10.1016/j.colsurfb.2011.06.031
Zhao, G., Wu, X., Chen, P., Zhang, L., Yang, C., and Zhang, J. (2018). Selenium nanoparticles are more efficient than sodium selenite in producing reactive oxygen species and hyper-accumulation of selenium nanoparticles in cancer cells generates potent therapeutic effects. Free Radic. Biol. Med. 126, 55–66. doi:10.1016/j.freeradbiomed.2018.07.017
Keywords: cancer, diabetes, microbial infection, nanoparticle, selenium nanoparticle
Citation: K D and Venugopal S (2022) Therapeutic potential of selenium nanoparticles. Front. Nanotechnol. 4:1042338. doi: 10.3389/fnano.2022.1042338
Received: 12 September 2022; Accepted: 06 October 2022;
Published: 24 October 2022.
Edited by:
Kumar Ponnuchamy, Alagappa University, IndiaReviewed by:
J. Wilson, Alagappa University, IndiaCopyright © 2022 K and Venugopal. This is an open-access article distributed under the terms of the Creative Commons Attribution License (CC BY). The use, distribution or reproduction in other forums is permitted, provided the original author(s) and the copyright owner(s) are credited and that the original publication in this journal is cited, in accordance with accepted academic practice. No use, distribution or reproduction is permitted which does not comply with these terms.
*Correspondence: Subhashree Venugopal, dnN1Ymhhc2hyZWU2M0BnbWFpbC5jb20=