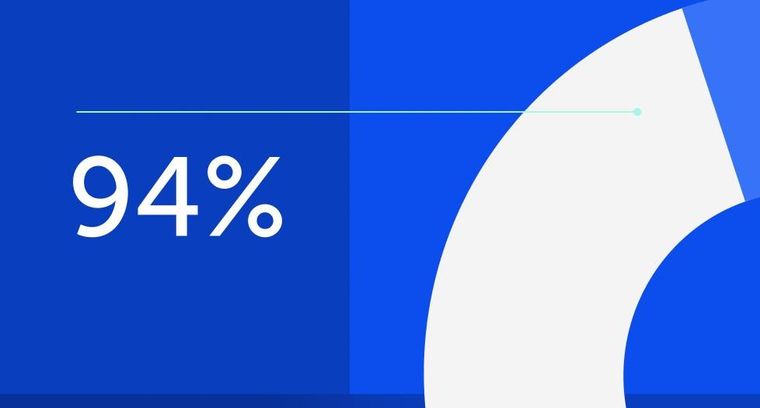
94% of researchers rate our articles as excellent or good
Learn more about the work of our research integrity team to safeguard the quality of each article we publish.
Find out more
REVIEW article
Front. Nanotechnol., 12 January 2022
Sec. Biomedical Nanotechnology
Volume 3 - 2021 | https://doi.org/10.3389/fnano.2021.813847
This article is part of the Research TopicBiosynthesis of Bio-inspired Nanoparticles/Nanomaterials and Evaluation of their Therapeutic Potential in the Medical FieldView all 8 articles
Severe acute respiratory syndrome coronavirus-2 (SARS-CoV-2) is a contagious virus that spreads exponentially across the world, resulting in serious viral pneumonia. Several companies and researchers have put their tremendous effort into developing novel vaccines or drugs for the complete eradication of COVID-19 caused by SARS-CoV-2. Bionanotechnology plays a vital role in designing functionalized biocompatible nanoparticulate systems with higher antiviral capabilities. Thus, several nanocarriers have been explored in designing and delivering drugs and vaccines. This problem can be overcome with the intervention of biomaterials or bionanoparticles. The present review describes the comparative analysis of SARS infection and its associated etiological agents. This review also highlighted some nanoparticles that have been explored in the treatment of COVID-19. However, these carriers elicit several problems once they come in contact with biological systems. Often, the body’s immune system treats these nanocarriers as foreign particles and antigens. In contrast, some bionanoparticles are highlighted here with their potential application in SARS-CoV-2. However, bionanoparticles have demonstrated some drawbacks discussed here with the possible outcomes. The scope of bioinspired nanoparticles is also discussed in detail to explore the new era of research. It is highly essential for the effective delivery of these nanoparticles to the target site. For effective management of SARS-CoV-2, different delivery patterns are also discussed here.
Severe acute respiratory syndrome (SARS) is a viral respiratory infection that happens due to the coronavirus and becomes a global threat upon their appearance. Back-to-back appearance, wider spreading, genetic mutation of etiological agents, and high mortality rate become a growing concern to human beings (Swelum et al., 2020). In the last two decades, the world has observed three occurrences of pandemics such as SARS coronavirus (SARS-CoV), Middle East Respiratory Syndrome Coronavirus (MERS-CoV), and SARS coronavirus-2 (SARS-CoV-2) (Debnath et al., 2020). However, no adequate or approved drug has been developed yet (2002–2021) against this CoV family. Thus, the current focus is based on the development of vaccines and repurposing medicines. It has been observed that the genome of COVID-19 is 80% similar to that of the first SARS in 2002 (Zheng, 2020). Therefore, there is a need for detailed analysis to understand the typical patterns of this class of infection and etiological agents. This comparative study will help target/inhibit a particular site of this virus with opportunistic drugs or particulate matter for effective infection management.
Nanotechnology has become an emerging area of interest consisting in the manipulation of material in different fields, including biology, chemistry, and engineering. The nanomedicine approach has been demonstrated as a new strategy to amplify anti-infective activities, localize delivery to the infection site, reduce off-target effects, and reduce the chances of drug resistance (Yang et al., 2019). Several novel approaches have been developed to fabricate nanomaterial or nanoparticles for different purposes. Several nanoparticulate systems have been designed to combat the SARS infection by applying nanotechnology. This review covers all different types of nanoparticle-based strategies recommended or used to treat SARS infection and highlights the selection reasons. Surprisingly, there is not enough progress in scale-up and their availability in the market.
One of the most critical barriers nanomaterials face is the protein corona (PC). It develops once nanomaterials or nanovectors interact with the circulatory proteins in vivo. This PC plays a central character in triggering the immune response and causes immunological toxicity (Park J. Y. et al., 2021). Additionally, these materials may exhibit toxicities ranging from no toxicity to high toxicity. This review highlights potential barriers and possible ways to resolve them. The possible intervention to address these problems is the introduction of bionanoparticles to treat SARS infection. Bionanomaterials are derived from biological origins with a molecular structure in nanoscale dimensions. These bionanomaterials have demonstrated several advantages: cost-effectiveness, environmentally friendly, controlled physicochemical properties, and reproducibility.
These viruses can be detected by targeting different components, like nucleocapsid protein, spike protein, small envelope protein, and ORF1ab sequence in RNA-dependent RNA polymerase genes (Udugama et al., 2020). Detection methods available for COVID-19 are antigen, antibody, and molecular tests. The molecular test is the most common detection method based on the detection of nucleic acid. This test helps detect the genetic materials of SARS-CoV-2 in the patient sample. Viral RNA is isolated first and proceeds for reverse transcription-quantitative polymerase chain reaction (RT-qPCR) that transforms viral RNA into DNA and then amplifies to produce millions of copies. Afterward, a detection probe determined the viral genetic material of patients. The RT-PCR method is designated as the gold standard due to fewer drawbacks. In contrast, clustered regularly interspaced short palindromic repeats (CRISPR) draw significant attention in nucleic acid detection due to their simplicity, high sensitivity, specificity, and rapid analysis. CRISPR proteins cut the target region complemented with CRISPR RNA (crRNA), followed by cleavage of the surrounding nucleic acids (Ganbaatar and Liu, 2021).
This review has shown a brief outlay of different types of bionanomaterials that may serve as a potential candidate to eradicate the SARS infection. SARS is especially a respiratory infection. Targeting drugs to the lungs or respiratory tract is a big challenge using any conventional or nanoparticulate form due to less available drugs at the infection site and non-specific targeting. Therefore, effective delivery patterns have been discussed here to give an overview of delivering medications to the infection site. The fabrication of nanoparticles or bionanoparticles is often time-consuming and produces lots of waste. The scientific community pits their enormous efforts into the novel fabrication process of bionanomaterials to meet industrial scalability. Bioinspired materials have been discussed here to understand their scope in treating SARS. This review will guide the researchers to explore several new areas for better management of SARS infection.
Coronaviruses are the largest RNA viruses. SARS-CoVs and SARS-CoV2 are RNA viruses that contain the largest genomes in the RNA virus family. They belong to the same subfamily, family, and order of Coronavirinae, Coronaviridae, and Nidovirales, respectively. This subfamily includes four genera such as α-, β-, γ-, and δ-coronavirus. Of these genera, two α-CoVs (HCoV-NL63 and HCoV-229E) and two β-CoVs (HCoV-OC43 and HCov-HKU1) cause mild respiratory symptoms. γ- and δ-CoVs strains are primarily observed in avian species (Davidson et al., 2020). MERS-CoVs, SARS-CoVs, and SARS-CoV2 fall in the β-coronavirus genera. These human coronaviruses (hCoVs) consist of 3′ polyadenylated tails and 5′ methylated caps. The 5′ terminal region is the non-structural part containing genes responsible for viral replication. The 3′ terminal region is responsible for encoding different types of proteins, such as spike protein, membrane protein, nucleocapsid protein, and envelope protein (Debnath et al., 2020). The spike protein consists of two subunits: S1 and S2. S1 unit acts on the virus binding to host receptors, and S2 unit induces fusion between viruses and the host membrane followed by genome penetration into host cells cytoplasm.
Among all RNA viruses, the genome size of CoVs is smaller (26–32 kilobases or kb) than the planarian of secretory cell nidovirus (41 kb). Human CoV and MERS-CoV have demonstrated a larger genomic size (30.11 kb) than SARS-CoV (29.75 kb) and SARS-CoV-2 (29.9 kb) (Zhu et al., 2020). After performing the comparative genomic analysis using zPicture, at the nucleotide level, the genomic sequences of both the stains have extremely high homology (Xu et al., 2020). In total, 89% of the nucleotide identity of the SARS-CoV2 genome has matched with that of the SARS-like-coVZXC21 (derived from the bat) and 82% with human SARS-CoV (Chan et al., 2020). Six differences have been identified between these two stains and numbered RD1 to RD6. RD1, RD2, and RD3 show partial coding sequences of the orf lab gene, whereas RD4 and RD5 are the partial coding sequences of the S gene. RD6 is part of the coding sequence of the orf7b and orf8 genes. These RDs are the new target sites for drugs and help develop new drug molecules for SARS.
All encoded proteins of both SARSs are found as highly homologous (95–100%), indicating the evolutionary similarity with only differences in the orf8 and orf10. In SARS-CoV-2, the amino acid sequence of orf8 is unlike the arrangement of orf8 or orf8b obtained from earlier human SARS-CoV. The amino acid of N-protein for SARS-CoV is identical up to 90% to SARS-CoV (Gralinski and Menachery, 2020). It signifies that the drugs and nanoparticles used to target the N-protein of SARS-CoV are equally effective in SARS-CoV-2 infection. N-antibodies do not show any immunity to SARS-CoV2. However, they had cross-reactivity against SARS-CoV. Moreover, 99% similarity has been observed in the spike stalk S2 of both SARS coronaviruses. Thus, S2-targeted nanoparticles or drug-loaded nanoparticles will serve as an ultimate opportunistic treatment to eradicate this infection (Chan et al., 2020).
As discussed earlier, the spike protein is the most crucial structural protein that engages itself in the interaction between host cells and CoVs. All CoVs enter host cells by adhering to functional receptors on the host surface. In this activity, angiotensin-converting enzyme 2 (ACE2) present in the host body helps bind and allow CoVs to invade the host body. ACE2 is homologous with an angiotensin-converting enzyme (ACE) but is not invited by ACE inhibitors. In the presence of ACE, Ang I is transformed to Ang II that is further metabolized in the presence of ACE2, and carboxypeptidase results in the formation of vasodilator and angiotensin 1–7. ACE2 plays the primary function in balancing the renin-angiotensin system (RAS), which is more critical in regulating Ang I and Ang II into Ang 1–9 and Ang 1–7, respectively (Tikellis and Thomas, 2012). This ACE2 system is associated with several critical pathways to prevent hypertension, diabetes mellitus, heart failure, and myocardial infarction. Therefore, this ACE2 is linked with several physiological activities like negative regulation of RAS, facilitating amino acid transport, and serving as a receptor for CoVs. The binding domain of SARS-CoV2 with ACE2 is substantial compared to SARS-CoV due to some chief amino acid residues. Due to this binding affinity, SARS-CoV2 has demonstrated the greatest pathogenicity in humans. Once bound with SARS-CoV2, ACE2 loses its function, which is driven by endocytosis and the process to proteolytic degradation (Gheblawi et al., 2020). Therefore, recombinant ACE2, Ang 1–7 analog, gene-delivery of Ace2, and Mas receptor agonists are some possible therapeutic options to activate RAS.
In the last couple of decades, the application of nanoparticles has increased significantly in drug delivery to overcome the limitations associated with free drugs. These nanoparticles have several promising applications, such as improving the solubility, drug residency, targeted delivery, controlling the release of drugs, and delivering combinational drugs. Not only drugs but these nanoparticles are also employed in developing vaccines by encapsulating antigens to prevent their degradation (Figure 1). Here are some nanoparticles designed to combat SARS-CoV-2 (Table 1).
Several types of nanoparticles are used as drug carriers to demonstrate the therapeutic activity. Whereas metal-based nanoparticles stand a vital role in presenting their activity without incorporating drugs. Numerous activities of metal-based nanoparticles are already well documented, like blocking the host-virus interaction in Monkeypox virus, competitive inhibition of herpes simplex virus, prevention of viral binding of hepatitis B, inactivation of tacaribe virus, and retarding viral attachment with glycoprotein to HIV-1 virus (Sadiq et al., 2021). These examples suggest that the metal-based nanoparticles work on different mechanisms like blocking viral replication and inhibiting viral attachment between host and virus. For a therapeutic intervention, restricting the viral entry of SARS-CoV-2 is an opportunistic approach. Thus, different metal-based nanoparticles are explored to combat COVID-19. Silver possesses several antimicrobial and antiviral activities. It shows effective killing efficiency to bacteria, fungi, and viruses. The positive charge of silver ions (Ag+) elicits the antimicrobial effects through distinct mechanisms. Due to these activities, silver nanoparticles (AgNPs) have been explored to inhibit SARS-CoV-2 (Jeremiah et al., 2020). Particle diameter around 10 nm of AgNPs effectively inhibited the extracellular SARS-CoV2 at the concentration range of 1–10 ppm. With an increased concentration of 20 ppm, these AgNPs showed a cytotoxic effect. AgNPs are highly potent microbicides and also found effective against SARS-CoV-2. Due to the broader activity, the formulation of Argovit® silver nanoparticles reached the clinical trial stage (Cluster de BIoeconomia de Baja California, 2021). In this study, the effects of nose rinse and mouthwash with Argovit® are expected to prevent COVID-19 infection in high-risk groups in Tijuana Hospital, Mexico.
US food and drug administration (US FDA) already approved iron oxide nanoparticles to treat anemia. These iron oxide nanoparticles (Fe3O4 and Fe2O3) were explored to interact with the spike protein of SARS-CoV-2 and found a strong interaction with this binding site (Abo-zeid et al., 2020). This interaction is interrelated with viral protein conformational changes. Thus, it is needed to initiate a clinical study on iron oxide nanoparticles in COVID-19 patients. Some phytoconstituents and nutraceuticals are found effective against SARS-CoV-2. These materials are safe, cost-effective, and readily available and have lower side effects. Zinc oxide nanoparticles (ZnO NPs) were prepared to incorporate such types of material (Attia et al., 2021). Hesperidin was isolated from orange peels and showed the highest docking score. ZnO NPs and hesperidin alone and in combination were tested on hepatitis A virus, an RNA virus. ZnO NPs showed more potent activity than hesperidin. Thus, hesperidin-mediated ZnO nanoparticles can be an optional approach to treat COVID-19, which needs to be confirmed through clinical evaluation. ZnO NPs have been used as disinfectant nanospray (El-Megharbel et al., 2021). These ZnO NPs showed higher antiviral activity against SARS-CoV-2 at the cytotoxic concentration of CC50/IC50 ≤ 1. These ZnO NPs showed antiviral activity at IC50-526 ng/ml. However, these nanoparticles showed the cytotoxic effect on host cells at CC50-292.2 ng/ml. Thus, to reduce cellular host toxicity, ZnO NPs can be complexed with other compounds in a different ratio. Therefore, a mixture of various metal nanoparticles was also tested on SARS-CoV-2. TPNT1, a metal nanoparticle composed of Au-NP, ZnO-NP, and ClO2, was prepared in an aqueous solution (Chang et al., 2021). In in vitro setting, this TPNT1 inhibited six significant clades of SARS-CoV2 with a concentration range used in food additives. This material blocked the entry of SARS-CoV-2 by inhibiting spike proteins in binding with the ACE2 receptor. All these examples suggest that metal nanoparticles play a role in eradicating the SARS-CoV-2 infection. Combining all metal nanoparticles with appropriate proportions can be the best option in managing COVID-19.
Lipid nanoparticles (LNPs) draw significant interest due to their biocompatibility, ease of industrial scale-up, safe, and non-toxicity. A wide range of therapeutic agents, including small drug molecules to biotechnological products, can be delivered using these types of formulations. LNPs were explored to encapsulate messenger RNAs (mRNA) encoding the receptor-binding domain of SARS-CoV2 in developing a vaccine (ARCoV) (Zhang N. N. et al., 2020). mRNAs have emerged as an opportunistic platform for vaccine development. Intramuscular administration (mice and non-human primates) of this mRNA LNP provoked antibody neutralization against SARS-CoV2 and Th1-based cellular response. Two doses of ARCoV eradicate the viruses in immunized mice. Currently, this vaccine is under phase II clinical trials. Due to its superior action, this vaccine can be granted for phase III clinical trials.
A different mRNA vaccine was designed in a separate study by encapsulating the SARS-CoV-2 human Fc-conjugated receptor-binding domain in lipid nanoparticles (Elia et al., 2021). Mostly ionizable amine lipids promoted nucleic acid for endosomal escape, followed by cellular uptake. Additionally, these lipids also increased the encapsulation efficiency of nucleic acid. A phase 1 trial was conducted with two lipid nanoparticle formulations containing nucleoside-modified RNA vaccine: BNT162b1 and BNT162b2 (Walsh et al., 2020). These vaccines provoked SARS-CoV-2 neutralizing geometric mean titer in a dose-dependent manner. The BNT162b1 encoded the SARS-CoV-2 spike RBD and demanded it as a potential vaccine candidate. This vaccine-induced robust γ-interferon T-cell response in younger to older Chinese adults (Li et al., 2021). However, some local and systemic events have been reported with this dose-dependent vaccine, sometimes mild to moderate. Despite these unwanted events, this vaccine has an acceptable safety profile. Another vaccine, BNT162b2, also has a favorable safety profile and is highly effective against COVID-19. Apart from the regular two doses, an additional third booster dose was given to improve immunity over time and protect from the delta variant. Another promising and equally efficient vaccine is mRNA1273, formulated with lipid nanoparticles. A phase 3 randomized clinical trial was recently conducted with an mRNA-1273 vaccine (Baden et al., 2021). Two mRNA-1273 intramuscular injections were given to patients, and the primary endpoint was fixed to prevent COVID-19 illness. These LPNs-based vaccines demonstrated 94.1% efficacy in preventing COVID-19 without adverse issues. US FDA has authorized this vaccine under emergency use to prevent COVID-19.
The soluble form of human angiotensin-converting enzyme 2 (hACE2) binds with SARS-CoV-2 spike protein and restricts the viral entry into target cells. This soluble recombinant ACE2 improves the lung’s injury. However, the main barrier in the therapeutic application is its short half-life. Thus, synthetic mRNA was encoded with a soluble hACE2 that was further packed with lipid nanoparticles and transferred to mammalian cells to enhance the production of secreted proteins (Kim et al., 2020). This lipoidal formulation was administered via the intravenous route that led to hepatic delivery of mRNA. Within 2 h of administration, elicited secretion of soluble hACE2 was observed, and the level was maintained for 6 h. Soluble hACE2 was detected in the bronchoalveolar lavage fluid after 24 h and persisted up to 48 h.
Lipid-derived TT3 nanoparticles were used to deliver NASAR (optimum combination of 5′ and 3′ untranslated regions of mRNA) mRNAs to trigger the expression of SARS-CoV-2 antigens (Zeng et al., 2020). The antigen-specific antibodies induction was observed twofold more than the previously approved LNPs material MC3 in vaccinated mice. Thus, it was believed that these NASAR mRNAs could be an alternative to SARS-CoV-2 vaccines. In vaccine development, self-amplifying RNA encoding SARS-CoV-2 spike protein was also used by encapsulating it within LPNs (McKay et al., 2020). Dose-dependent SARS-CoV-2-specific antibody titers were observed in mouse sera and successfully neutralized both pseudo-virus and wild-type virus. This neutralization was proportional to the amount of specific IgG. This LNP immunization induced Th1-based responses in mice. Additionally, no antibody-dependent enhancement was observed.
Despite the development of several emerging vaccines, no efficient vaccine has been identified in targeting this novel coronavirus. In an attempt, a highly effective small interfering RNA (siRNA) therapy was developed using a novel LNP delivery system (Idris et al., 2021). Three siRNA candidates were effective and inhibited the virus up to 90% alone or in combination. These siRNAs were successfully delivered to the lungs, mainly damaged due to the SARS-CoV-2 infection. These LNP-siRNA approaches are scalable from industrial prospects and recommended once the first sign of COVID-19 infection is observed in humans. Therefore, this formulation can be an adjunctive therapy to current vaccine strategies.
Polymeric nanoparticles are the primary type of nanocarriers. These nanocarriers can change the pharmacokinetic profile of drugs by modifying the release kinetics. Likewise, they elucidate the required drug concentration for optimum biological activity. These nanoparticles can be fabricated by several techniques like spray-drying, ionic gelation, solvent evaporation, living or free-radical polymerization, polymer dispersion, and nanoprecipitation. They possess several additional advantages over other types of drug delivery like site-specific delivery, low toxicity, improved cellular uptake, controlled release drug molecules, and modified degradation. Nanoparticles with a size range of 100–500 nm can target the cytoplasm (polyisobutyl cyanoacrylate and polyethyleneimine shell). In contrast, particles sizes between 10 and 200 nm can adsorb drugs in the matrix and prevent the unwanted degradation of drugs (Rana, 2021).
Poly(lactic-co-glycolic acid) (PLGA) is one of the most effective biodegradable polymeric nanoparticles. The US FDA approves it due to its low toxic profile, biocompatibility, and sustained and controlled release properties. This polymer is employed to deliver several antiviral drugs in the COVID-19 treatment. Ivermectin-loaded PLGA-b-PEG-MAL nanoparticles were prepared to deliver a more potent antiviral dose and avoid protein spike binding and its receptor ACE2 (Surnar et al., 2020). The targeted nanoparticles containing ivermectin inhibited the nuclear transport activities using proteins, such as importin α/β1 heterodimer. These nanoparticles were not immunogenic, resulting in no initiation of immune cell activation after accumulating at alveolar lung cells. Several drugs were explored to complete the eradication of this COVID-19. Remdesivir is a potent antiviral drug found effective against SARS-CoV-2. A combination of molecular docking with dissipative particle dynamic simulations was used to design ACEI-containing remdesivir-loaded PLGA nanoparticles to improve clinical outcomes (Wu et al., 2020). In this formulation, a lisinopril molecule covalent graft was employed onto PLGA to encapsulate remdesivir. These nanoparticles were spherical with increasing the concentration of remdesivir and underwent precipitation due to high remdesivir loading. PLGA nanoparticles are also prepared for sustained release of the drug at the target site. Oseltamivir phosphate (OP) is an oral antiviral prodrug. It works in therapy after activating into carboxylate form. This drug was encapsulated into PLGA nanoparticles to develop an efficient and prolonged release antiviral drug delivery system (Ucar et al., 2021). This formulation development aimed to target the spike-binding peptide-1. This formulation showed sustained release of OP over 2 months. Thus, it can be an adequate formulation in the treatment of COVID-19.
The advanced understanding suggests a new approach to developing a drug by focusing on the host cells instead of targeting causative agents. The infectivity of SARS-CoV-2 relies on the binding with protein receptors. Nanosponges were prepared using human cell-derived membranes for medical countermeasures (Zhang Q. et al., 2020). For this fabrication, human lung epithelial cells were coated onto PLGA nanoparticles. This coating allowed nanosponges to inherit the viral receptors that retard the entry of coronavirus into the host cells. These PLGA nanocarriers are the most studied polymeric platform for DNA vaccine development as they show improved antigen-specific responses. Composite of PLGA nanoparticles (PLGA+ chitosan; PLGA+ polyethylenimine) was explored to improve the efficiency of DNA loading and systemic protection (Chauhan et al., 2020). Previously, stimulator of interferon genes (STING) agonist-loaded hollow PLGA nanoparticles were prepared for safe and effective vaccination against MERS (Lin et al., 2019). The release of STING agonists from this hollow PLGA was attributed to reduced systemic reactogenicity, prominent local immune activation, and a pH-responsive release profile. After antigen conjugation, these nanoparticles facilitated the co-delivery of antigens and STING agonists to the immune cells and lymph nodes for immune potentiation. These previous experiences suggested that this biodegradable polymer is the ultimate weapon in designing vaccines.
Carbon-based nanomaterials (CBNs) are a growing field of drug delivery. The main reason behind this is their diverse structural and physicochemical configurations. Functionalized CBNs are extensively used to deliver different nanomedicines in numerous diseases. The classification of carbon-based nanomaterials depends on their size. These materials show excellent optical properties. Additionally, these materials have a higher drug-loading capacity, lower immunogenicity, and improved biocompatibility (Debnath and Srivastava, 2021). CBNs are found to be effective against 13 single-stranded RNA viruses, including SARS-CoV-2. Due to the negligible or low toxicity, these materials are the opportunistic therapeutic approach to treat COVID-19 pneumonia and co-associated with other viruses, bacteria, fungi, and multi-drug resistant organisms (Serrano-Aroca et al., 2021b). These materials consist of carbon, a vital element of the human body. Apart from this, they are biodegradable and biocompatible and promote tissue regeneration. They possess a high surface area that helps functionalize or interact with other biocompatible polymers to improve biocompatibility and efficacy. SARS-CoV-2 infection is associated with several physiopathological changes and interferes with several pathways after reaching organs. CBNs induce the production of angiogenic factors followed by the initiation of signaling pathways by promoting the proliferation and differentiation of endothelial cells or mesenchymal stem cells (MSCs). These MSCs are not infected with SARS-CoV-2. Thus, these MSCs become a promising approach to treat COVID-19 patients with moderate and/or severe infection. These stem cells downregulate Th1 and Th17 inflammation immunity and upregulate the anti-inflammation immunity of Th2 and Treg cells. Studies showed that graphene materials interact with viruses via binding and wrapping mechanisms. These materials act as virustatic inhibitors, resulting in the inhibition of virus interaction with host cells (Mallakpour et al., 2021). A series of graphene derivatives altering the length of aliphatic chains were synthesized to investigate the inhibitory activity against SARS-CoV-2 (Donskyi et al., 2021). The coronavirus’s replication was inhibited by disrupting the viral envelope with long alkyl chains (>C9). This study demonstrated strong antiviral activity in a large concentration window without eliciting the toxicity to human cells.
Many biomaterials are designed as drug carriers like nanoparticles, microparticles, or capsules. These biomaterials play a vital role in mitigating the issues associated with the therapeutic administration (Ertas et al., 2021). These nanoparticles can be fabricated from several materials, such as synthetic polymers, polysaccharides, and proteins. These nanoparticles have a wide range of applications in overcoming the current therapeutic challenges of SARS-CoV-2 (Table 2).
Protein nanomaterials are a promising candidate for drug delivery due to their inherent characteristics like biodegradability, less immunogenicity, nontoxicity, less immunogenicity, and higher storage stability. They are easy to fabricate, and manufacturing scale-up is also possible.
Albumin is the most crucial protein in blood plasma and possesses several physiological activities. Albumin-sensitive cases are rare in individuals. Albumin nanoparticles are non-toxic, non-immunogenic, biocompatible, and biodegradable. The residue of albumin degradation is amino acids used to make body proteins. This protein has several functional groups and can bind with a significant amount of drugs. It is well documented that the COVID-19 infection is associated with increased cytokine levels, blood coagulation, NETosis, and a decrease in albumin levels. Drug-loaded albumin nanoparticles can be an opportunistic approach to restore the clinical outcomes associated with this infection. PEGylated nanoparticle albumin-bound (PNAB) derivatives were developed to improve the sustained bioactivity of steroidal ginsenoside saponins, such as PNAB-Rgx365 and PNAB-Rg6 (Park H. H. et al., 2021). Prepared PNAB ginsenoside effectively reduces NETosis-related factors and histone H4 in plasma and improves systemic inflammation in COVID-19 patients admitted to ICU. This study confirmed that these drugs effectively reduced the cytokine storm and tissue damage and enhanced the survival rate.
Additionally, these conjugated materials effectively reduce vascular inflammation and the formation of blood clotting. In animal model-based validation, this formulation demonstrated special applications like prevention of tissue damage, improved survival rate, and suppression of cytokine storm. In this ongoing pandemic, repurposing existing drugs is ideal for developing effective medication to fight COVID-19. A self-assembling technique was developed to synthesize niclosamide (NIC) loaded zinc nanoparticles that were further coated with bovine serum albumin (BSA) (Rejinold et al., 2021). The introduction of BSA improved drug stability, selectivity towards virus-infected cells, and injectability. The optimized particle size of the BSA-stabilized zinc-NIC nanohybrid was less than 200 nm. This hybrid drug delivery system is beneficial for treating COVID-19 patients suffering from high endothelial glycocalyx damage, followed by cytokine storm related to severe inflammation.
Gelatin (denatured protein) is obtained from the collagen base of animals. This protein is a polyampholyte type containing anionic (glutamic and aspartic amino acids), cationic (amino acids lysine and arginine), and hydrophobic groups (leucine, isoleucine, methionine, and valine) in a ratio of 1:1:1. This protein is successfully employed in various drug formulations in systemic administration. It has several applications like stabilizing gelatin sponges, vaccines, and protein formulations. It is also used to enhance plasma volume (Kianfar, 2021). Gelatin nanoparticles have been explored for several drug deliveries. Nanodeocoys (NDs) were developed with cell membranes of the Zika virus and gelatin nanoparticles (Rao et al., 2018). This composite captured the Zika virus and inhibited its entry and associated disease propagation. NDs restricted the passage of this virus through physiological barriers in the fetal brain. Likewise, it prevents virus-induced fetal microcephaly in pregnant mice. Therefore, nanodeocoys prepared from gelatin have a promising application in the fabrication of novel nanoparticulate forms to avoid the SARS-CoV-2 infection. siRNA silencing iNOS-loaded gelatin nanoparticles cross-linked with glutaraldehyde were explored for intranasal delivery (Kim et al., 2016; Rinoldi et al., 2021). After intranasal delivery, this formulation was detected in a different brain region like the olfactory bulb, striatum, and cerebral cortex for one day. The protective effect was shown for two weeks. These examples demand that gelatin nanoparticles are another potential drug carrier to combat COVID-19. Moreover, gelatin is biocompatible and non-toxic. Gelatin nanoparticles release drugs in a controlled manner by altering gelatin degradation and controlling the degree of cross-linking.
Polysaccharide-derived nanoparticles improve the biocompatibility of toxic cell material. Nanoparticles derived from naturally occurring polysaccharides have been used in delivering protein, peptides, and nucleic acids.
Alginate is a naturally occurring, water-soluble, linear unbranched polysaccharide and is composed of two types of uronic acids: α-L-guluronic acid and β-D-mannuronic acid. These are non-immunogenic, biocompatible, and mucoadhesive and dissociate in the typical physiological environment. The solubility of alginate in water depends on the type of associated cations. For example, sodium alginate is water-soluble, whereas the calcium derivatives form a gel. Alginate also forms complexes with several polycations like chitosan, polyenimine, or essential peptides like polyarginine and polyarginine. Recently, alginate and its related biomaterials were tested on 17 types of viruses (Serrano-Aroca et al., 2021a). These materials showed inhibitory activity against human immunodeficiency virus, herpes simplex virus type 1 and type 2, hepatitis A, B, and C viruses, rubella virus, and rabies virus. Many of them have enveloped, single-stranded, positive-sense RNA viruses like SARS-CoV-2.
Chitosan is a natural and biocompatible polysaccharide obtained from chitin that contains randomly distributed linked N-acetyl glucosamine and glucosamine units. Chitosan exhibits positive charges in the physiological environment due to quaternary ammonium salt groups in its backbone. Several active functional groups are present in the chitosan chain and make it easy to fabricate to elicit immune stimulation, antibacterial, antifungal, and wound healing properties. Predominantly, amino groups and hydroxyl groups mediate the biological activity of chitosan. Additionally, deacetylation and molecular weight play a crucial role in eliciting biological activity. Chitosan serves as a noninvasive mucosal vaccine vector due to its adhesiveness characteristics (Boroumand et al., 2021; Meng et al., 2021). Chitosan can be an opportunistic approach to fighting against COVID-19 infection. It can stimulate the immune system and penetrate through tight junctions of mucous epithelial cells. Chitosan nanoparticles reversibly open the tight epithelial junction to improve the paracellular and intracellular antigen transportation through the mucosal surfaces. These nanoparticles also enhanced the antigen uptake by the antigen-presenting cells (APCs). Therefore, chitosan can be employed as an attractive mucosal vaccine delivery vehicle (Dhakal and Renukaradhya, 2019).
Biotinylated chitosan nanoparticles were developed using a fusion protein vector for the dendritic cells. They successfully delivered plasmid DNA encoding SARS-CoV N-protein that increased IgA concentration in mucosa after intranasal administration. Bioavanta-Bosti developed aerosol formulation of anti-COVID-19 drugs. Aerosol formulations of NovochizolTM nanoparticles (chitosan-based) delivered anti-COVID-19 drugs directly to the lungs in severely ill patients (Safarzadeh et al., 2021). These formulations can be administered at all disease stages in ventilators. After administration, these nanoparticles can adhere to the mucosal membrane, and the encapsulated drugs can release by diffusion and slow degradation. Studies showed that polysaccharides could destroy the viruses in situ by invading the infected cell. The electrostatic interaction (positive charge of chitosan vs. negatively charged surface of the virus) interferes virus’s ability to cause infection and kills them by disrupting its protective membrane. N-(2-hydroxypropyl)-3-trimethylammonium chitosan chloride (HTCC) demonstrated effective inhibitory activity against SARS-CoV-2 and MERS propagation (Jaber et al., 2021). HTCC showed a 50–190 kDa molecular weight and contained 57–77% quaternary ammonium groups. HTCC was bound electrostatically with the S-protein of coronavirus, followed by blocking the interaction with the entry receptor and subsequent virus replication.
Polydopamine (PDA) is a bioinspired, nature-derived biopolymer material. This material possesses many exciting properties like universal adhesion and self-assembly. It can conjugate with several metal ions to form thermal annealing in a protective environment (Li et al., 2020). Magnetic nanoparticles were prepared by incorporating magnetic molecularly imprinted polymer based on self-polymerization of dopamine (Fresco-Cala et al., 2021). A peptide of viral spike protein was used here instead of using the whole SARS-CoV-2 virion template. After confirming the computational screening approach, dopamine was used here as a functional monomer. This study revealed the high binding energy of the dopamine-peptide complex and multi-point interactions in the entire template surface. Erythromycin decorated with virus-mimetic nanoparticles can carry a viral antigen and a toll-like receptor agonist. Polydopamine nanoparticles were synthesized using in situ polymerization to achieve this, where nanoparticles were conjugated with the SARS-CoV-2 spike protein S1 subunit (Wang et al., 2021). The prepared conjugates were further attached with erythromycin via catechol groups. In the mice model, the virus-mimetic nanoparticles showed several activities like the production of IgG antibodies, humoral and cellular immune responses, high maturation, and activation of antigen-presenting cells. This erythromycin-mediated antiviral immunization is a versatile concept of vaccine development.
Elevated amounts of cell-free DNA (cfDNA) are observed in SARS-CoV-2 sepsis patients, resulting in the progression of multiple organ failure. In such a situation, DNase-1 levels in plasma are reduced significantly. DNase-1 was encapsulated into polydopamine-poly(ethylene glycol) nanoparticles to suppress this cytokine storm (Lee et al., 2021). The exogenous administration of this long-acting DNase-1 effectively reduced the cfDNA level and neutrophil activities. Polydopamine derived from marine mussels shows an excellent potency in the early diagnosis of diseases and targeted delivery. Thus, this material can be used to deliver a wide range of drugs in the management of COVID-19.
The human body has an inbuilt, highly efficient defense system against foreign species/substances like viruses, fungi, bacteria, and chemicals, including nanomaterials. Different physiological barriers like skin, air-lung-blood barriers, blood-brain barriers, and circulation barriers are available in the body to protect respective organs and allow selective access to a specific substance such as messenger molecules and nutrients. The growing use of nanomaterials in medicine and diagnosis causes safety concerns about complex behavior. These barriers often regulate the access of nanomaterials and develop a selective mechanism to block nanomaterials. In contrast to bulk materials, these nanomaterials have demonstrated complicated physiological properties like size, shape, surface area, colloidal stability, mechanical property chemical composition, and crystallinity (Meng et al., 2018).
Most nanoparticle-based formulations have been designed for intravenous administration to spread deeper to organ tissue without surgery. These nanovectors interact with the biological system, mainly with the circulatory proteins, upon being available into the systemic circulation. One set of proteins binds with nanoparticles’ surface and forms a protein corona (PC) conjugate. After adhering, this PC makes the particles recognized by the innate immune system followed by quicker elimination by phagocytic cells present in the liver, lungs, and spleen. Additionally, these PCs increase the physical disability and agglomeration of particles. To overcome this problem, the utmost requirement is to develop new strategies to minimize PC formation with improved stability and circulatory time of nanovectors (Rampado et al., 2020). The composition and kinetics of PC vary (decrease or increase) over time. It was believed that the PCs are responsible for the instability of NPs. However, a separate study demonstrated the aggregation of nanoparticles inversely proportion with protein [human serum albumin (HSA), fibrinogen, immunoglobulin, and apolipoprotein A1 (APOA1)] concentration (Ho et al., 2018). It was observed that NPs aggregation was increased at lower protein concentrations, whereas these NPs were stable at higher protein concentrations. The interaction of the protein with the nanoparticles is varied based on the type of protein. Immunoglobulin and fibrinogens are sticky proteins either form clotting or bind with foreign bodies, whereas HAS and APOA1 interact with more NPs simultaneously to form bridges.
The concentration and tagged surfactants charged on NPs determine the PC characters (Zhang et al., 2019). Charges on NPs were changed after the formation of PC. Particles with negative charge persisted, whereas positively charged particles acquired a negative charge. Ovisprin-1 and lysozyme protein coronas were tested on plain gold nanoparticles to understand the protein corona formation in an aqueous environment (Jahan Sajib et al., 2020). It was observed that the appearance of PC is caused due to protein-protein interaction and protein’s surface and hydrophobic surface effect. The size of nanoparticles and protein types modulate the structure of protein size.
Several factors influence the toxicity of nanomaterials, like particle size, surface charge, surface chemistry, and dose. After entering the body, these nanoparticles elicit toxicity in mostly all types of systems (e.g., circulatory system, digestive system, endocrine system, immune system, respiratory system, nervous system, and reproductive system). The main reason behind this toxicity is the formation of reactive oxygen species (ROS) either directly or indirectly. The formed ROS interfere in the multiple mechanisms of cells and elicit toxicities (Akcan et al., 2020). Mainly, all bionanomaterials/bionanoparticles have demonstrated less to negligible toxicity. These biomaterials might be metabolized in the body compartment, and their by-product may elicit toxic effects in the body. Thus, it is highly essential to study the degradation patterns of these bionanomaterials. Sometimes, the synthesis method of bionanomaterials also contributes to demonstrating toxicity. However, albumin-based nanoparticles have shown numerous advantages over other types of nanoparticles. However, their way of preparation may elicit toxicity. Albumin-based nanocarriers are prepared using desolvation, thermal-induced aggregation, self-assembly, and albumin-bound technology. The desolvation technique is used most frequently, where a desolvating agent like ethanol or acetone is used in the dehydration process of albumin. This process causes conformation changes of albumin from stretched to coiled. The addition of these organic solvents induces potential toxicity in the human body (Hassanin and Elzoghby, 2020).
Bioinspired and biomimetic drug delivery becomes an emerging trade to bypass natural barriers. These delivery patterns depend on unique mechanisms, morphologies, and biomaterials with remarkable properties. It is a multidisciplinary research area having the equal involvement in biology, engineering, material science, and physics. Nature has developed numerous effective schemes to adapt different biomaterials and mechanisms. These schemes help the researcher design biocompatible and biodegradable materials to improve drug delivery (Rahamim and Azagury, 2021).
Next-generation nanoscale vectors have been developed using the inorganic components to attain inert structures using a mimic biological form that serves additional functionalities (Table 3). It is highly essential to reveal the capability of biomimetics in penetrating multiple layers of defense of organs and tissues without inferring the normal biological function of the body. Whatever the route of administration, every nanoparticulate system needs to interact with biological fluids and organic compounds present in the body. As per the appearance of the biological system, material interactions follow a unique way based on composition, physical properties, and body region or tissue of exposure. In contrast, inorganic nanoparticles can be demonstrated as a mimic of globular proteins (Weiss et al., 2020). These similarities can intervene in the cell-receptor interaction and the virus pathways. Neutrophil-specific programmed cell death through activation of NETosis is a good reason for COVID-19 pathogenesis. Bioinspired DNase-I-coated melanin-like nanospheres were developed to dysregulate this infection-induced NETosis (Park et al., 2020). In this study, poly(ethylene glycol) and DNase-I were used as coating materials that improve the circulation and dissolution of neutrophil extracellular traps (NET) structure. These bioinspired DNase-I coated melanin-like nanospheres reduced neutrophil counts and NETosis-related factors in SARS-CoV-2 infection. Additionally, these nanoparticles reduced systemic inflammation and associated mortality in a septic mouse model.
In the advance cases of SARS-CoV-2 infection, cytokine storm syndrome has been observed with prominent inflammation and multi-organ failure. Lopinavir-loaded PLGA nanoparticles (PLGA-LPV NPs) were prepared to downregulate these multiple pro-inflammatory cytokines and suppress the activation of neutrophils and macrophages (Tan et al., 2021). Afterward, these nanoparticles were coated with macrophages (a membrane donator) to form biomimetic nanocarriers. Blank PLGA nanoparticles were coated with macrophage (PLGA@M). Due to this surface coating, PLGA@M mimic a macrophage that absorbs pro-inflammatory substance. This biomimetic-based drug delivery showed a targeted antiviral effect with improved anti-inflammation.
Conventional viral vaccine development faces challenges in a sudden pandemic due to higher mutation rates and antigen diversity. The introduction of nanotechnology tunes this development process significantly with improved efficacy, specificity, and prolonged antiviral activity. Bioinspired nanoparticles have demonstrated diverse functions, prolonged circulation time, improved biocompatibility, and accumulated at target sites. Significantly, the unique antigenic characteristics and immunostimulatory properties of biomimetic nanoparticles help develop effective vaccine formulations (Huang et al., 2021). Currently, several biomimetic nanoparticles, including virus-like particles (VLPs), outer membrane vesicles, self-assembling protein nanoparticles, and virosomes, are available for vaccine development (Figure 2).
VLPs-based human papillomavirus vaccines are already available in the market with the advancement of technology. Due to design flexibility, VLPs are the ultimate opportunistic approach to fabricating modern vaccine and disease eradication through rational bioengineering due to design flexibility. These novel nanoparticles can incorporate different virus subtypes to prepare potent antigen proteins. Using structural elucidation, epitope mapping, and prediction can be an excellent option to develop protective vaccine antigens (Liljeroos et al., 2015; Hume et al., 2019). Thus, manipulating nanoparticles parameters can obtain more efficient vaccines than traditional ones. Previously, MERS-CoV-specific immunoglobulin A was helpful for infected patients but not recommended long term. MERS-CoV-specific memory CD8+ T-cells persist in the body for 6 years to sustain promising antiviral effects on numerous subtypes of viruses. The recurrence of virulence can hamper the potential application of live-attenuated vaccines’ development. However, these vaccines are efficient in memory T-cells formation. The next-generation biomimetic nanoparticles can be retained on the pathogen surface and interfere with their physicochemical properties. These types of biomimetic-based nanoparticles vaccines mainly consist of viral antigen molecules (for adaptive immune response) in addition to adjuvants (innate immune response) and protein scaffolds. These biomimetic nanoparticles protect adjuvants from degradation and prevent systemic toxicity caused by these free adjuvants. VLP vaccines trigger an immune response that results in neutralizing antibodies. Conjugated B-cells on the surface of VLPs bind with antigens through B-cell receptors. After\activation, B-cells are converted into plasmablast that leads the production of antibodies. The maturation of dendritic cells is crucial to demonstrate the cellular immune response prompted by the vaccine (Huang et al., 2021). These VLPs are explored for vaccine development against COVID-19.
A randomized, blinded, dose-dependent controlled study was conducted on a VLP-based vaccine produced in plants that have demonstrated SARS-CoV-2 spike glycoprotein (CoVLP) (Ward et al., 2021). These formulations were found to be well tolerated with mild to moderate adverse events. There was no dose-dependent effect of CoVLP observed on the serum-neutralized antibodies. However, titers were significantly increased once used with the adjuvants of AS03 and CpG1018. Baculovirus has a gene encoded full-length SARS-CoV-2 spike glycoprotein, a prototype sequence of Wuhan-Hu-1. A recombinant SARS-CoV-2 nanoparticle vaccine (NVX-CoV2373) was developed using this vaccine (Shinde et al., 2021). In a randomized, placebo-controlled phase 1-2 trial, the NVX-CoV2373 vaccine prompted neutralizing antibodies and demonstrated a strong antigen-specific CD4+ T-cell response.
Bioinspired nanoparticles have been successfully developed by combining nanotechnology with biomimetic strategies to optimize surface bio-physicochemical properties for vaccine development. Due to the intrinsic activity, these nanoparticles can be an effective therapeutic option in many infectious diseases. Outer membrane vesicles (OMV) are generally referred to as bacterial-derived nanoparticles, also known as bacterial extracellular vesicles. These nano-sized spherical vesicles contain immunostimulatory molecules like lipoprotein, peptidoglycans, lipopolysaccharide, and bacterial surface-exposed antigens. Likewise, these vesicles are non-living but mimic the outside of bacteria. These OMVs can be derived from many organisms, like S. typhimurium, H. pylori, P. aeruginosa, and B. melitensis. In a study, gold nanoparticles were employed to coat E. coli OMVs to obtain hybrid and biomimetic nanovaccines (Gao et al., 2015). Prepared bacterial membrane-coated gold nanoparticles (BM-AuNPs) showed improved stability in vitro. This conjugated material activates and maturates dendritic cells in mice upon subcutaneous administration. These BM-AuNPs also initiate the production of interferon-gamma and interleukin-17.
Not only bacterial vaccines but also these OMVs have also been explored to develop the COVID-19 vaccine. A novel platform has been designed based on the Neisseria meningitides OMVs and recombinant receptor-binding domain (rRBD) (Gaspar et al., 2021). Previously, the OMVs-based vaccine was effective against Neisseria meningitidis serogroup B and successfully combated epidemic strains in different countries. Against COVID-19 infection, a high IgG production has been observed when rRBD mixed with OMV and adjuvant like aluminum hydroxide. This combinational formulation generated 13 times more IFN-γ producing cells in splenocytes culture than the rRBD-treated group. Sessional influenza epidemics and MERS have presented a substantial health burden. To combat these dual infections, an OMVs-based vaccine was proposed that simultaneously binded with RBD of MERS-CoV and served as a stable antigenic stable fusion protein of the H1-type hemagglutinin of influenza A virus (Shehata et al., 2019). This chimeric vaccine demonstrated a higher neutralizing antibodies titer against the H1N1 virus. In addition, this OMVs-based vaccine also induced cellular immunity without significant loss in body weight. The synergistic effect of humoral and cellular immunities was observed in mice when this formulation was administered. This OMVs-based vaccine overcomes several limitations faced with an mRNA-based vaccine. An increased amount of detoxified OMVs was prepared from genetically modified Vibrio cholerae and enterotoxigenic E. coli (ETEC) (Thapa et al., 2021). Noninvasive intranasal immunization has confirmed a higher titer, induced protective, long-lasting antibody response in the murine model. The most elevated titers against the SARS-CoV-2 were observed in ETEC-derived OMVs compared to V. cholera.
Protein self-assembly serves as a new platform in the fabrication of nanoparticles with tailored functions. It shows many nanoscale structures like wires, tubes, cages, and rings. Due to these different structural configurations, symmetry, easy fabrication, and functionalization, these particles are successfully used as carriers of the drug, protein, peptides, and synthetic molecules. The popularity of protein assembly-based is increasing gradually due to easy fusion between protein assemblies with inactivated pathogens to prepare safe medicine (Nguyen et al., 2021). Nanovaccines have numerous advantages like antigen stabilization, improved uptake by antigen-presenting cells, and improved immunogenicity. Synthetic and inorganic nanoparticles have demonstrated numerous drawbacks like low stability, poor biocompatibility, and toxicity. In contrast, nanovaccines composed of self-assemble protein are superior in overcoming the above-mentioned challenges. In addition, it has demonstrated molecular specificity and multivalence that lead to efficient delivery to antigenic determinants (Zottig et al., 2020). In mice, self-assembling protein nanoparticles were developed to improve antibody responses against SARS-CoV-2 (Walls et al., 2020). This nanoparticle-based vaccine demonstrated a high immunogenic array in 60 SARS-CoV-2 spike RBDs and induced neutralizing antibody titers 10-fold more than the perfusion-stabilized spike deposit.
Additionally, multiple epitopes can be targeted by the antibodies elicited by RBD nanoparticles that exhibited a lower binding-neutralizing ratio than the convalescent human sera. Likewise, these protein nanoparticle-based vaccines decrease the threat of respiratory diseases. Ferritin is a protein containing iron and stored inside cells. Self-assembling ferritin nanoparticles were designed to develop subunit vaccine candidates (Powell et al., 2021). This ferritin has demonstrated an intelligent nanocarrier platform for the development of vaccines and also passed through clinical trials. The mean neutralizing antibody titers was 2-fold higher with two spike ferritin particles than the convalescent plasma from COVID-19. Vaccination with certain subunit vaccines has demonstrated successful protection in many viruses like Gardasil, Cervarix, and human papillomavirus. The main scenario behind the success is the capsid protein to self-assemble into oligomeric spherical. In the case of the influenza subunit vaccine, self-assembly occurred due to the attachment of antigen to oligoproteins. Protein nanoparticles are helpful in the development of a vaccine in different immune-evasive pathogens like influenza, malaria, and human immunodeficiency virus. After injection, protein nanoparticles travel freely through blood and lymph and rapidly accumulate in other organs like the spleen and thyroid to elicit humoral immunity (Nguyen and Tolia, 2021). A prototypic SARS vaccine was developed with self-assembling polypeptide nanoparticles for SARS-CoV (Pimentel et al., 2009). These nanoparticles showed a SARS B-cell epitope from the C-terminal heptad repeat of the virus spike protein.
It is crucial to establish a similar conformation between the B-cell epitope of the vaccine with targeted protein. High specific conformation was observed in the binding affinity in these types of polypeptide nanoparticles. This peptide vaccine showed the heptad repeat region as anα-helical trimeric coiled coil. Nanoparticles conjugated with antigen are used to develop a vaccine against SARS-CoV-2. Using the SpyTag/SpayCatcher system, the RBD can be visible on the self-assembling protein that contains an inner layer of locking domains (RBD) composed of two 60 mer and 24 mer ferritins (He et al., 2021). An immunoaffinity column was developed that contained a CR3022 antibody to purify the tag-free vaccine. In this study, a scaffold receptor-binding domain trimer was constructed to mimic the RBD-spike conformation. This technique provides a robust process in developing RBD-based nanovaccines.
Virosome is an FDA-approved lipoidal nanocarrier that possesses viral protection against infections. Surface modification of virosome with critical viral fusion proteins is a novel prospect for vaccine developments. Surface antigens on the virosome interact with targeted receptors that induce cellular immune response using antibody-producing B-cells. A virosome structure like a virus is enveloped without nucleocapsid. Due to their attractive features, virosome-based vaccines have been approved in more than 45 countries (Asadi and Gholami, 2021). The virosome-based vaccine, Transvac-2 (by European MI Matric company), was developed for COVID-19. Virosome particles absorb epitopes of antigens using lipid-linker or hydrophobic bonding. These particles can incorporate both hydrophilic and hydrophobic drugs. Surface modification of these nanoparticles with hydrophilic polymer improves circulation time. Conjugation of antibodies, ligands, and peptides is also feasible (Wang and Xu, 2020).
Eradication of SARS-CoV-2 can be done in several ways, like viral death (extracellular or intracellular) and inhibition of ACE2 receptors to prevent viral entry to the host cells (Figure 3).
Drug targeting the SARS-CoV-2 virus requires identifying a suitable delivery system that ensures better absorption and intracellular delivery. In addition, it should maintain the adequate concentration between the organ of interest and systemic circulation. The targeted delivery serves optimum activity at the administration site with minimal toxicity and a high drug payload. Aerosol-based drug delivery systems have drawn sufficient interest in managing different pulmonary disorders. Therefore, this type of delivery becomes an ultimate weapon to treat the COVID-19 infection, where lungs are mainly infected. Novochizol, a nanoparticle-based aerosol formulation, has demonstrated adequate transportation of COVID-19 drugs and maintained the drug concentration at optimum level in the lungs (Mehta et al., 2020). Drug-loaded theranostic nanoparticles can effectively deliver drugs in intranasal delivery to combat SARS-CoV-2 transmission. This intranasal delivery system represents an alternative route of drug administration for viral pulmonary diseases.
The respiratory tract and lungs are the primary target sites for SARS-CoV-2 infection. Higher viral load in this respiratory tract is observed in advanced cases of SARS-CoV-2 infection. Several neutralizing monoclonal antibodies (mAbs) were administered through intravenous infusion. However, there is a lack of efficient access to IgG antibodies in the mucosal compartments. It has been observed that the antibody levels in the lungs are 200–500 times lower than those in the serum. To obtain the potential antiviral effect, these neutralizing IgG1 mAbs need to be administered at high doses. Even resistance to potent neutralizing IgG1 was observed in clinical trials authorized for emergency use. In a single intranasal dose, IgM was administered to address this issue. This study conferred therapeutic protection against several variants and demonstrated desirable pharmacokinetics and safety profiles (Ku et al., 2021).
A vesicular drug delivery system serves as an advanced theranostic approach in treating COVID-19 infections. The surface charges on the vesicular system play a crucial role in modulating the pharmacokinetic parameters of drug molecules. Vesicular carriers containing positive charges increase the adherence property to the negative charges of the mucosal membrane through the electrostatic interactions and concurrently prevent mucociliary clearance and enzymatic degradation. Using this vesicular drug delivery, multifunctional- and multimodality-based theranostic techniques were employed to fetch information on COVID-19 etiology (Satija et al., 2020). The application of vesicular-delivery systems in the medical field will be a revolutionary approach in developing new therapeutic and diagnoses of COVID-19. Additionally, this delivery system serves as an innovative solution to combat future coronavirus outbreaks.
Nanoparticles (polymer lipid or metal) are successfully used in several diseases to deliver drugs or biological molecules. Metal nanoparticles are mainly employed alone to treat SARS-CoV-2, whereas other nanoparticles encapsulated drugs, mRNA, and other biological molecules. Much literature highlighted their potential drawbacks, like partial biocompatibility, no biodegradability, eliciting an immune response, and toxicity. In this scenario, biomaterials/bionanomaterials serve many scopes to overcome the current therapeutic challenges. The applications and properties of the protein or polysaccharide-based nanoparticles are emerging for therapeutic purposes. However, there is very little evidence of the potential application of these biomaterials in COVID-19 infection. There are many scopes to improve the treatment efficiency using these biomaterials. The biodegradability, biocompatibility, cytotoxicity, and immune response of these biopolymeric nanoparticles need to assess their potential application in drug delivery for COVID-19. Often, nanocarriers face several problems once they enter into the biological systems. The body’s immune system treats these nanocarriers as foreign particles and antigens. These problems can be manageable with bioinspired and biomimetic drug delivery. It is an emerging trade to bypass natural barriers. These delivery patterns have unique mechanisms and morphologies and contain biomaterials with remarkable properties. The fabrication of a next-generation biomimetic system is possible with an inorganic entity that can mimic biological structure with additional functionalities. Once they interact with bodily fluids, these bioinspired materials follow an intelligent way to overcome the problems associated with other types of nanoparticles. Therefore, the implication of this bioinspired and biomimetic system needs to be explored more for better management of SARS-CoV-2 infection.
SD wrote this article. RS supervised this work and revised the manuscript. Both authors have contributed equally to the article and approved the submitted version.
The authors declare that the research was conducted in the absence of any commercial or financial relationships that could be construed as a potential conflict of interest.
All claims expressed in this article are solely those of the authors and do not necessarily represent those of their affiliated organizations, or those of the publisher, the editors and the reviewers. Any product that may be evaluated in this article, or claim that may be made by its manufacturer, is not guaranteed or endorsed by the publisher.
Abo-zeid, Y., Ismail, N. S. M., McLean, G. R., and Hamdy, N. M. (2020). A Molecular Docking Study Repurposes FDA Approved Iron Oxide Nanoparticles to Treat and Control COVID-19 Infection. Eur. J. Pharm. Sci. 153, 105465. doi:10.1016/J.EJPS.2020.105465
Akçan, R., Aydogan, H. C., Yildirim, M. Ş., Taşteki̇n, B., and Sağlam, N. (2020). Nanotoxicity: A challenge for Future Medicine. Turk J. Med. Sci. 50, 1180–1196. doi:10.3906/SAG-1912-209
Asadi, K., and Gholami, A. (2021). Virosome-based Nanovaccines; a Promising Bioinspiration and Biomimetic Approach for Preventing Viral Diseases: A Review. Int. J. Biol. Macromol. 182, 648–658. doi:10.1016/J.IJBIOMAC.2021.04.005
Attia, G. H., Moemen, Y. S., Youns, M., Ibrahim, A. M., Abdou, R., and El Raey, M. A. (2021). Antiviral Zinc Oxide Nanoparticles Mediated by Hesperidin and In Silico Comparison Study between Antiviral Phenolics as Anti-SARS-CoV-2. Colloids Surf. B: Biointerfaces 203, 111724. doi:10.1016/J.COLSURFB.2021.111724
Baden, L. R., El Sahly, H. M., Essink, B., Kotloff, K., Frey, S., Novak, R., et al. (2021). Efficacy and Safety of the mRNA-1273 SARS-CoV-2 Vaccine. N. Engl. J. Med. 384, 403–416. doi:10.1056/NEJMOA2035389
Boroumand, H., Badie, F., Mazaheri, S., Seyedi, Z. S., Nahand, J. S., Nejati, M., et al. (2021). Chitosan-based Nanoparticles against Viral Infections. Front. Cel. Infect. Microbiol. 11, 175. doi:10.3389/FCIMB.2021.643953
Chan, J. F.-W., Kok, K.-H., Zhu, Z., Chu, H., To, K. K.-W., Yuan, S., et al. (2020). Genomic Characterization of the 2019 Novel Human-Pathogenic Coronavirus Isolated from a Patient with Atypical Pneumonia after Visiting Wuhan. Emerging Microbes Infect. 9, 221–236. doi:10.1080/22221751.2020.1719902
Chang, S.-Y., Huang, K.-Y., Chao, T.-L., Kao, H.-C., Pang, Y.-H., Lu, L., et al. (2021). Nanoparticle Composite TPNT1 Is Effective against SARS-CoV-2 and Influenza Viruses. Sci. Rep. 11, 8692. doi:10.1038/s41598-021-87254-3
Chauhan, G., Madou, M. J., Kalra, S., Chopra, V., Ghosh, D., and Martinez-Chapa, S. O. (2020). Nanotechnology for COVID-19: Therapeutics and Vaccine Research. ACS Nano 14, 7760–7782. doi:10.1021/ACSNANO.0C04006
Cluster de BIoeconomia de Baja California (2021). Evaluation of Silver Nanoparticles for the Prevention of COVID-19. ClinicalTrials.Gov. 1. Available at: https://clinicaltrials.gov/ct2/show/NCT04894409 (Accessed October 30, 2021).
Davidson, A. M., Wysocki, J., and Batlle, D. (2020). Interaction of SARS-CoV-2 and Other Coronavirus with ACE (Angiotensin-Converting Enzyme)-2 as Their Main Receptor. Hypertension 76, 1339–1349. doi:10.1161/HYPERTENSIONAHA.120.15256
Debnath, S. K., and Srivastava, R. (2021). Drug Delivery with Carbon-Based Nanomaterials as Versatile Nanocarriers: Progress and Prospects. Front. Nanotechnol. 3, 1–22. doi:10.3389/fnano.2021.644564
Debnath, S. K., Srivastava, R., and Omri, A. (2020). Emerging Therapeutics for the Management of COVID 19. Expert Opin. Emerging Drugs 25, 337–351. doi:10.1080/14728214.2020.1810663
Dhakal, S., and Renukaradhya, G. J. (2019). Nanoparticle-based Vaccine Development and Evaluation against Viral Infections in Pigs. Vet. Res. 50, 1–14. doi:10.1186/S13567-019-0712-5
Donskyi, I. S., Nie, C., Ludwig, K., Trimpert, J., Ahmed, R., Quaas, E., et al. (2021). Graphene Sheets with Defined Dual Functionalities for the Strong SARS‐CoV‐2 Interactions. Small 17, 2007091. doi:10.1002/SMLL.202007091
Elia, U., Ramishetti, S., Rosenfeld, R., Dammes, N., Bar-Haim, E., Naidu, G. S., et al. (2021). Design of SARS-CoV-2 hFc-Conjugated Receptor-Binding Domain mRNA Vaccine Delivered via Lipid Nanoparticles. ACS Nano 15, 9627–9637. doi:10.1021/acsnano.0c10180
El-Megharbel, S. M., Alsawat, M., Al-Salmi, F. A., and Hamza, R. Z. (2021). Utilizing of (Zinc Oxide nano-spray) for Disinfection against “SARS-CoV-2” and Testing its Biological Effectiveness on Some Biochemical Parameters during (COVID-19 pandemic)—”ZnO Nanoparticles Have Antiviral Activity against (SARS-CoV-2)”. Coatings 11, 388. doi:10.3390/COATINGS11040388
Ertas, Y. N., Mahmoodi, M., Shahabipour, F., Jahed, V., Diltemiz, S. E., Tutar, R., et al. (2021). Role of Biomaterials in the Diagnosis, Prevention, Treatment, and Study of corona Virus Disease 2019 (COVID-19). Emergent Mater. 4, 35–55. doi:10.1007/S42247-021-00165-X
Fresco-Cala, B., Rajpal, S., Rudolf, T., Keitel, B., Groß, R., Münch, J., et al. (2021). Development and Characterization of Magnetic SARS-CoV-2 Peptide-Imprinted Polymers. Nanomaterials 11, 2985. doi:10.3390/NANO11112985
Ganbaatar, U., and Liu, C. (2021). CRISPR-based COVID-19 Testing: Toward Next-Generation point-of-care Diagnostics. Front. Cel. Infect. Microbiol. 11, 373. doi:10.3389/FCIMB.2021.663949/BIBTEX
Gao, W., Fang, R. H., Thamphiwatana, S., Luk, B. T., Li, J., Angsantikul, P., et al. (2015). Modulating Antibacterial Immunity via Bacterial Membrane-Coated Nanoparticles. Nano Lett. 15, 1403–1409. doi:10.1021/NL504798G
Gaspar, E. B., Prudencio, C. R., and De Gaspari, E. (2021). Experimental Studies Using OMV in a New Platform of SARS-CoV-2 Vaccines. Hum. Vaccin. Immunother. 17, 2968. doi:10.1080/21645515.2021.1920272
Gheblawi, M., Wang, K., Viveiros, A., Nguyen, Q., Zhong, J. C., Turner, A. J., et al. (2020). Angiotensin-converting Enzyme 2: SARS-CoV-2 Receptor and Regulator of the Renin-Angiotensin System: Celebrating the 20th Anniversary of the Discovery of ACE2. Circ. Res. 126, 1456–1474. doi:10.1161/CIRCRESAHA.120.317015
Gralinski, L. E., and Menachery, V. D. (2020). Return of the Coronavirus: 2019-nCoV. Viruses 12, 135. doi:10.3390/v12020135
Hassanin, I., and Elzoghby, A. (2020). Albumin-based Nanoparticles: a Promising Strategy to Overcome Cancer Drug Resistance. Cancer Drug Resist. 3, 930–946. doi:10.20517/CDR.2020.68
He, L., Lin, X., Wang, Y., Abraham, C., Sou, C., Ngo, T., et al. (2021). Single-component, Self-Assembling, Protein Nanoparticles Presenting the Receptor Binding Domain and Stabilized Spike as SARS-CoV-2 Vaccine Candidates. Sci. Adv. 7, eabf1591. doi:10.1126/SCIADV.ABF1591
Ho, Y. T., Azman, N. A., Loh, F. W. Y., Ong, G. K. T., Engudar, G., Kriz, S. A., et al. (2018). Protein corona Formed from Different Blood Plasma Proteins Affects the Colloidal Stability of Nanoparticles Differently. Bioconjug. Chem. 29, 3923–3934. doi:10.1021/acs.bioconjchem.8b00743
Huang, L., Rong, Y., Pan, Q., Yi, K., Tang, X., Zhang, Q., et al. (2021). SARS-CoV-2 Vaccine Research and Development: Conventional Vaccines and Biomimetic Nanotechnology Strategies. Asian J. Pharm. Sci. 16, 136–146. doi:10.1016/J.AJPS.2020.08.001
Hume, H. K. C., Vidigal, J., Carrondo, M. J. T., Middelberg, A. P. J., Roldao, A., and Lua, L. H. L. (2019). Synthetic Biology for Bioengineering Virus-like Particle Vaccines. Biotechnol. Bioeng. 116, 919–935. doi:10.1002/BIT.26890
Idris, A., Davis, A., Supramaniam, A., Acharya, D., Kelly, G., Tayyar, Y., et al. (2021). A SARS-CoV-2 Targeted siRNA-Nanoparticle Therapy for COVID-19. Mol. Ther. 29, 2219–2226. doi:10.1016/J.YMTHE.2021.05.004
Jaber, N., Al-Remawi, M., Al-Akayleh, F., Al-Muhtaseb, N., Al-Adham, I. S. I., and Collier, P. J. (2021). A Review of the Antiviral Activity of Chitosan, Including Patented Applications and its Potential Use against COVID-19. J. Appl. Microbiol. 132, 41–58. doi:10.1111/JAM.15202
Jahan Sajib, M. S., Sarker, P., Wei, Y., Tao, X., and Wei, T. (2020). Protein corona on Gold Nanoparticles Studied with Coarse-Grained Simulations. Langmuir 36, 13356–13363. doi:10.1021/acs.langmuir.0c02767
Jeremiah, S. S., Miyakawa, K., Morita, T., Yamaoka, Y., and Ryo, A. (2020). Potent Antiviral Effect of Silver Nanoparticles on SARS-CoV-2. Biochem. Biophys. Res. Commun. 533, 195–200. doi:10.1016/j.bbrc.2020.09.018
Kianfar, E. (2021). Protein Nanoparticles in Drug Delivery: Animal Protein, Plant Proteins and Protein Cages, Albumin Nanoparticles. J. Nanobiotechnol. 19, 1–32. doi:10.1186/S12951-021-00896-3
Kim, I. D., Sawicki, E., Lee, H. K., Lee, E. H., Park, H. J., Han, P. L., et al. (2016). Robust Neuroprotective Effects of Intranasally Delivered iNOS siRNA Encapsulated in Gelatin Nanoparticles in the Postischemic Brain. Nanomedicine 12, 1219–1229. doi:10.1016/J.NANO.2016.01.002
Kim, J., Mukherjee, A., Nelson, D., Jozic, A., and Sahay, G. (2020). Rapid Generation of Circulating and Mucosal Decoy ACE2 Using mRNA Nanotherapeutics for the Potential Treatment of SARS-CoV-2, PubMed. bioRxiv, 205583. Available at: https://pubmed.ncbi.nlm.nih.gov/32743574/(Accessed July 5, 2021).
Ku, Z., Xie, X., Hinton, P. R., Liu, X., Ye, X., Muruato, A. E., et al. (2021). Nasal Delivery of an IgM Offers Broad protection from SARS-CoV-2 Variants. Nature 595, 718–723. doi:10.1038/s41586-021-03673-2
Lee, Y. Y., Park, H. H., Park, W., Kim, H., Jang, J. G., Hong, K. S., et al. (2021). Long-acting Nanoparticulate DNase-1 for Effective Suppression of SARS-CoV-2-Mediated Neutrophil Activities and Cytokine Storm. Biomaterials 267, 120389. doi:10.1016/j.biomaterials.2020.120389
Li, H., Xi, J., Donaghue, A. G., Keum, J., Zhao, Y., An, K., et al. (2020). Synthesis and Catalytic Performance of Polydopamine Supported Metal Nanoparticles. Sci. Rep. 10, 1–7. doi:10.1038/s41598-020-67458-9
Li, J., Hui, A., Zhang, X., Yang, Y., Tang, R., Ye, H., et al. (2021). Safety and Immunogenicity of the SARS-CoV-2 BNT162b1 mRNA Vaccine in Younger and Older Chinese Adults: a Randomized, Placebo-Controlled, Double-Blind Phase 1 Study. Nat. Med. 27, 1062–1070. doi:10.1038/s41591-021-01330-9
Liljeroos, L., Malito, E., Ferlenghi, I., and Bottomley, M. J. (2015). Structural and Computational Biology in the Design of Immunogenic Vaccine Antigens. J. Immunol. Res. 2015, 156241. doi:10.1155/2015/156241
Lin, L. C. W., Huang, C. Y., Yao, B. Y., Lin, J. C., Agrawal, A., Algaissi, A., et al. (2019). Viromimetic STING Agonist-Loaded Hollow Polymeric Nanoparticles for Safe and Effective Vaccination against Middle East Respiratory Syndrome Coronavirus. Adv. Funct. Mater. 29, 1807616. doi:10.1002/ADFM.201807616
Mallakpour, S., Azadi, E., and Hussain, C. M. (2021). Fight against COVID-19 Pandemic with the Help of Carbon-Based Nanomaterials. New J. Chem. 45, 8832–8846. doi:10.1039/D1NJ01333E
McKay, P. F., Hu, K., Blakney, A. K., Samnuan, K., Brown, J. C., Penn, R., et al. (2020). Self-amplifying RNA SARS-CoV-2 Lipid Nanoparticle Vaccine Candidate Induces High Neutralizing Antibody Titers in Mice. Nat. Commun. 11, 3523. doi:10.1038/s41467-020-17409-9
Mehta, M., Prasher, P., Sharma, M., Shastri, M. D., Khurana, N., Vyas, M., et al. (2020). Advanced Drug Delivery Systems Can Assist in Targeting Coronavirus Disease (COVID-19): A Hypothesis. Med. Hypotheses 144, 110254. doi:10.1016/J.MEHY.2020.110254
Meng, H., Leong, W., Leong, K. W., Chen, C., and Zhao, Y. (2018). Walking the Line: the Fate of Nanomaterials at Biological Barriers. Biomaterials 174, 41. doi:10.1016/J.BIOMATERIALS.2018.04.056
Meng, Q., Sun, Y., Cong, H., Hu, H., and Xu, F.-J. (2021). An Overview of Chitosan and its Application in Infectious Diseases. Drug Deliv. Transl. Res. 11, 1340–1351. doi:10.1007/S13346-021-00913-W
Nguyen, B., and Tolia, N. H. (2021). Protein-based Antigen Presentation Platforms for Nanoparticle Vaccines. Npj Vaccin. 6, 70. doi:10.1038/s41541-021-00330-7
Nguyen, Q. D., Kikuchi, K., Maity, B., and Ueno, T. (2021). The Versatile Manipulations of Self-Assembled Proteins in Vaccine Design. Int. J. Mol. Sci. 22, 1–21. doi:10.3390/ijms22041934
Park, H. H., Park, W., Lee, Y. Y., Kim, H., Seo, H. S., Choi, D. W., et al. (2020). Bioinspired DNase-I-Coated Melanin-like Nanospheres for Modulation of Infection-Associated NETosis Dysregulation. Adv. Sci. 7, 2001940. doi:10.1002/ADVS.202001940
Park, H. H., Kim, H., Lee, H. S., Seo, E. U., Kim, J. E., Lee, J. H., et al. (2021a). PEGylated Nanoparticle Albumin-Bound Steroidal Ginsenoside Derivatives Ameliorate SARS-CoV-2-Mediated Hyper-Inflammatory Responses. Biomaterials 273, 120827. doi:10.1016/J.BIOMATERIALS.2021.120827
Park, J. Y., Park, S. J., Park, J. Y., Kim, S. H., Kwon, S., Jung, Y. J., et al. (2021b). Unfolded Protein corona Surrounding Nanotubes Influence the Innate and Adaptive Immune System. Adv. Sci. 8, 2004979. doi:10.1002/ADVS.202004979
Pimentel, T. A. P. F., Yan, Z., Jeffers, S. A., Holmes, K. V., Hodges, R. S., and Burkhard, P. (2009). Peptide Nanoparticles as Novel Immunogens: Design and Analysis of a Prototypic Severe Acute Respiratory Syndrome Vaccine. Chem. Biol. Drug Des. 73, 53–61. doi:10.1111/J.1747-0285.2008.00746.X
Powell, A. E., and Zhang, K., Sanyal, M., Tang, S., Weidenbacher, P. A., Li, S., et al. (2021). A Single Immunization with Spike-Functionalized Ferritin Vaccines Elicits Neutralizing Antibody Responses against SARS-CoV-2 in Mice. ACS Cent. Sci. 7, 183–199. doi:10.1021/ACSCENTSCI.0C01405
Pyrc, K., Milewska, A., Duran, E. B., Botwina, P., Dabrowska, A., Jedrysik, M., et al. (2021). SARS-CoV-2 Inhibition Using a Mucoadhesive, Amphiphilic Chitosan that May Serve as an Anti-viral Nasal spray. Sci. Rep. 11, 1–11. doi:10.1038/s41598-021-99404-8
Rahamim, V., and Azagury, A. (2021). Bioengineered Biomimetic and Bioinspired Noninvasive Drug Delivery Systems. Adv. Funct. Mater. 31, 2102033. doi:10.1002/ADFM.202102033
Rampado, R., Crotti, S., Caliceti, P., Pucciarelli, S., and Agostini, M. (2020). Recent Advances in Understanding the Protein corona of Nanoparticles and in the Formulation of “Stealthy” Nanomaterials. Front. Bioeng. Biotechnol. 8, 166. doi:10.3389/fbioe.2020.00166
Rana, M. M. (2021). Polymer-based Nano-Therapies to Combat COVID-19 Related Respiratory Injury: Progress, Prospects, and Challenges. J. Biomater. Sci. Polym. Ed. 32, 31. doi:10.1080/09205063.2021.1909412
Rao, L., Wang, W., Meng, Q.-F., Tian, M., Cai, B., Wang, Y., et al. (2018). A Biomimetic Nanodecoy Traps Zika Virus to Prevent Viral Infection and Fetal Microcephaly Development. Nano Lett. 19, 2215–2222. doi:10.1021/ACS.NANOLETT.8B03913
Rejinold, S. N., Choi, G., Piao, H., and Choy, J.-H. (2021). Bovine Serum Albumin-Coated Niclosamide-Zein Nanoparticles as Potential Injectable Medicine against COVID-19. Materials (Basel) 14, 3792. doi:10.3390/MA14143792
Rinoldi, C., Zargarian, S. S., Nakielski, P., Li, X., Liguori, A., Petronella, F., et al. (2021). Nanotechnology-assisted RNA Delivery: From Nucleic Acid Therapeutics to COVID-19 Vaccines. Small Methods 5, 2100402. doi:10.1002/SMTD.202100402
Sadiq, I. Z., Abubakar, F. S., and Dan-Iya, B. I. (2021). Role of Nanoparticles in Tackling COVID-19 Pandemic: a Bio-Nanomedical Approach. J. Taibah Univ. Sci. 15, 198–207. doi:10.1080/16583655.2021.1944488
Safarzadeh, M., Sadeghi, S., Azizi, M., Rastegari-Pouyani, M., Pouriran, R., and Hoseini, M. H. M. (2021). Chitin and Chitosan as Tools to Combat COVID-19: A Triple Approach. Int. J. Biol. Macromol. 183, 235. doi:10.1016/J.IJBIOMAC.2021.04.157
Satija, S., Mehta, M., Sharma, M., Prasher, P., Gupta, G., Chellappan, D. K., et al. (2020). Vesicular Drug Delivery Systems as Theranostics in COVID-19. Futur. Sci. 12, 1607–1609. doi:10.4155/FMC-2020-0149
Serrano-Aroca, Á., Ferrandis-Montesinos, M., and Wang, R. (2021a). Antiviral Properties of Alginate-Based Biomaterials: Promising Antiviral Agents against SARS-CoV-2. ACS Appl. Bio Mater. 4, 5897–5907. doi:10.1021/ACSABM.1C00523
Serrano-Aroca, Á., Takayama, K., Tuñón-Molina, A., Seyran, M., Hassan, S. S., Pal Choudhury, P., et al. (2021b). Carbon-based Nanomaterials: Promising Antiviral Agents to Combat COVID-19 in the Microbial-Resistant Era. ACS Nano 15, 8069–8086. doi:10.1021/ACSNANO.1C00629
Shehata, M. M., Mostafa, A., Teubner, L., Mahmoud, S. H., Kandeil, A., Elshesheny, R., et al. (2019). Bacterial Outer Membrane Vesicles (OMVs)-Based Dual Vaccine for Influenza A H1N1 Virus and MERS-CoV. Vaccines 7, 46. doi:10.3390/VACCINES7020046
Shinde, V., Bhikha, S., Hoosain, Z., Archary, M., Bhorat, Q., Fairlie, L., et al. (2021). Efficacy of NVX-CoV2373 Covid-19 Vaccine against the B.1.351 Variant. N. Engl. J. Med. 384, 1899–1909. doi:10.1056/NEJMOA2103055
Surnar, B., Kamran, M. Z., Shah, A. S., and Dhar, S. (2020). Clinically Approved Antiviral Drug in an Orally Administrable Nanoparticle for COVID-19. ACS Pharmacol. Transl. Sci. 3, 1371–1380. doi:10.1021/acsptsci.0c00179
Swelum, A. A., Shafi, M. E., Albaqami, N. M., El-Saadony, M. T., Elsify, A., Abdo, M., et al. (2020). COVID-19 in Human, Animal, and Environment: A Review. Front. Vet. Sci. 7, 578. doi:10.3389/FVETS.2020.00578/BIBTEX
Tan, Q., He, L., Meng, X., Wang, W., Pan, H., Yin, W., et al. (2021). Macrophage Biomimetic Nanocarriers for Anti-inflammation and Targeted Antiviral Treatment in COVID-19. J. Nanobiotechnol. 19, 1–16. doi:10.1186/S12951-021-00926-0
Thapa, H. B., Müller, A. M., Camilli, A., and Schild, S. (2021). An Intranasal Vaccine Based on Outer Membrane Vesicles against SARS-CoV-2. Front. Microbiol. 12, 752739. doi:10.3389/FMICB.2021.752739
Tikellis, C., and Thomas, M. C. (2012). Angiotensin-converting Enzyme 2 (ACE2) Is a Key Modulator of the Renin Angiotensin System in Health and Disease. Int. J. Pept. 2012, 256294. doi:10.1155/2012/256294
Ucar, B., Acar, T., Arayici, P. P., and Derman, S. (2021). A Nanotechnological Approach in the Current Therapy of COVID-19: Model Drug Oseltamivir-Phosphate Loaded PLGA Nanoparticles Targeted with Spike Protein Binder Peptide of SARS-CoV-2. Nanotechnology 32, 485601. doi:10.1088/1361-6528/AC1C22
Udugama, B., Kadhiresan, P., Kozlowski, H. N., Malekjahani, A., Osborne, M., Li, V. Y. C., et al. (2020). Diagnosing COVID-19: The Disease and Tools for Detection. ACS Nano 14, 3822–3835. doi:10.1021/ACSNANO.0C02624
Walls, A. C., Fiala, B., Schäfer, A., Wrenn, S., Pham, M. N., Murphy, M., et al. (2020). Elicitation of Potent Neutralizing Antibody Responses by Designed Protein Nanoparticle Vaccines for SARS-CoV-2. Cell 183, 1367–1382.e17. doi:10.1016/j.cell.2020.10.043
Walsh, E. E., Frenck, R. W., Falsey, A. R., Kitchin, N., Absalon, J., Gurtman, G., et al. (2020). Safety and Immunogenicity of Two RNA-Based Covid-19 Vaccine Candidates. N. Engl. J. Med. 383, 2439–2450. doi:10.1056/NEJMOA2027906
Wang, Z.-B., and Xu, J. (2020). Better Adjuvants for Better Vaccines: Progress in Adjuvant Delivery Systems, Modifications, and Adjuvant–Antigen Codelivery. Vaccines 8, 128. doi:10.3390/VACCINES8010128
Wang, L., Wang, X., Yang, F., Liu, Y., Meng, L., Pang, Y., et al. (2021). Systemic Antiviral Immunization by Virus-Mimicking Nanoparticles-Decorated Erythrocytes. Nano Today 40, 101280. doi:10.1016/J.NANTOD.2021.101280
Ward, B. J., Gobeil, P., Séguin, A., Atkins, J., Boulay, I., Charbonneau, P. Y., et al. (2021). Phase 1 Randomized Trial of a Plant-Derived Virus-like Particle Vaccine for COVID-19. Nat. Med. 27, 1071–1078. doi:10.1038/s41591-021-01370-1
Weiss, C., Carriere, M., Fusco, L., Capua, I., Regla-Nava, J. A., Pasquali, M., et al. (2020). Toward Nanotechnology-Enabled Approaches against the COVID-19 Pandemic. ACS Nano 14, 6383–6406. doi:10.1021/ACSNANO.0C03697
Wu, J., Wang, H., and Li, B. (2020). Structure-aided ACEI-Capped Remdesivir-Loaded Novel PLGA Nanoparticles: toward a Computational Simulation Design for Anti-SARS-CoV-2 Therapy. Phys. Chem. Chem. Phys. 22, 28434–28439. doi:10.1039/D0CP04389C
Xu, J., Zhao, S., Teng, T., Abdalla, A. E., Zhu, W., Xie, L., et al. (2020). Systematic Comparison of Two Animal-To-Human Transmitted Human Coronaviruses: SARS-CoV-2 and SARS-CoV. Viruses 12, 32098422. doi:10.3390/v12020244
Yang, G., Chen, S., and Zhang, J. (2019). Bioinspired and Biomimetic Nanotherapies for the Treatment of Infectious Diseases. Front. Pharmacol. 10, 751. doi:10.3389/FPHAR.2019.00751/BIBTEX
Zeng, C., Hou, X., Yan, J., Zhang, C., Li, W., Zhao, W., et al. (2020). Leveraging mRNA Sequences and Nanoparticles to Deliver SARS-CoV-2 Antigens In Vivo. Adv. Mater. 32, e2004452. doi:10.1002/adma.202004452
Zhang, Z., Guan, J., Jiang, Z., Yang, Y., Liu, J., Hua, W., et al. (2019). Brain-targeted Drug Delivery by Manipulating Protein corona Functions. Nat. Commun. 10, 1–11. doi:10.1038/s41467-019-11593-z
Zhang, N. N., Li, X. F., Deng, Y. Q., Zhao, H., Huang, Y. J., Yang, G., et al. (2020a). A Thermostable mRNA Vaccine against COVID-19. Cell 182, 1271–1283.e16. doi:10.1016/j.cell.2020.07.024
Zhang, Q., Honko, A., Zhou, J., Gong, H., Downs, S. N., Vasquez, J. H., et al. (2020b). Cellular Nanosponges Inhibit SARS-CoV-2 Infectivity. Nano Lett. 20, 5570–5574. doi:10.1021/ACS.NANOLETT.0C02278
Zheng, J. (2020). SARS-CoV-2: An Emerging Coronavirus that Causes a Global Threat. Int. J. Biol. Sci. 16, 1685. doi:10.7150/IJBS.45053
Zhu, Z., Lian, X., Su, X., Wu, W., Marraro, G. A., and Zeng, Y. (2020). From SARS and MERS to COVID-19: A Brief Summary and Comparison of Severe Acute Respiratory Infections Caused by Three Highly Pathogenic Human Coronaviruses. Respir. Res. 21, 1–14. doi:10.1186/s12931-020-01479-w
Keywords: SARS, SARS-CoV-2, COVID-19, bionanoparticles, bioinspired, nanoparticles
Citation: Debnath SK and Srivastava R (2022) Potential Application of Bionanoparticles to Treat Severe Acute Respiratory Syndrome Coronavirus-2 Infection. Front. Nanotechnol. 3:813847. doi: 10.3389/fnano.2021.813847
Received: 12 November 2021; Accepted: 22 December 2021;
Published: 12 January 2022.
Edited by:
Srinivasan Ramanathan, Fujian Agriculture and Forestry University, ChinaReviewed by:
Arunachalam Kannappan, Shanghai Jiao Tong University, ChinaCopyright © 2022 Debnath and Srivastava. This is an open-access article distributed under the terms of the Creative Commons Attribution License (CC BY). The use, distribution or reproduction in other forums is permitted, provided the original author(s) and the copyright owner(s) are credited and that the original publication in this journal is cited, in accordance with accepted academic practice. No use, distribution or reproduction is permitted which does not comply with these terms.
*Correspondence: Sujit K. Debnath, ZHJza2RlYkBnbWFpbC5jb20=; Rohit Srivastava, cnNyaXZhc3RhQGlpdGIuYWMuaW4=
Disclaimer: All claims expressed in this article are solely those of the authors and do not necessarily represent those of their affiliated organizations, or those of the publisher, the editors and the reviewers. Any product that may be evaluated in this article or claim that may be made by its manufacturer is not guaranteed or endorsed by the publisher.
Research integrity at Frontiers
Learn more about the work of our research integrity team to safeguard the quality of each article we publish.