- Institute of Biochemistry, Biocenter, Goethe University Frankfurt, Frankfurt, Germany
The transport of nutrients, xenobiotics, and signaling molecules across biological membranes is essential for life. As gatekeepers of cells, membrane proteins and nanopores are key targets in pharmaceutical research and industry. Multiple techniques help in elucidating, utilizing, or mimicking the function of biological membrane-embedded nanodevices. In particular, the use of DNA origami to construct simple nanopores based on the predictable folding of nucleotides provides a promising direction for innovative sensing and sequencing approaches. Knowledge of translocation characteristics is crucial to link structural design with function. Here, we summarize recent developments and compare features of membrane-embedded nanopores with solid-state analogues. We also describe how their translocation properties are characterized by microchip systems. The recently developed silicon chips, comprising solid-state nanopores of 80 nm connecting femtoliter cavities in combination with vesicle spreading and formation of nanopore-suspended membranes, will pave the way to characterize translocation properties of nanopores and membrane proteins in high-throughput and at single-transporter resolution.
Introduction
Nanopores are holes with diameters of a few nanometers that already found multiple applications in basic research and industry facilitating our daily life. They are used for water desalination (O’Hern et al., 2015; Amadei et al., 2017), filtering of ions (Walker et al., 2017; Caglar et al., 2020), gas separation (Dong et al., 2016; Park et al., 2017), biological analysis (Miles et al., 2013), DNA/RNA sensing (Wanunu et al., 2010; Riedl et al., 2015; Xi et al., 2016; Shang et al., 2018), and detection of diverse analytes of biomedical and environmental relevance (Litvinchuk et al., 2007; Howorka and Siwy, 2009; Movileanu, 2009; Reiner et al., 2012; Miles et al., 2013; Stoloff and Wanunu, 2013; Wang et al., 2013). The commercialization of DNA sequencing by Oxford Nanopore Technologies extended the field significantly (Cherf et al., 2012; Manrao et al., 2012; Deamer et al., 2016; Quick et al., 2016).
Nanopores can be classified into two groups based on their material and fabrication procedure: 1) membrane-embedded pores and 2) solid-state pores. The latter can be fabricated with tunable sizes in materials such as silicon derivatives (Li et al., 2003; Chang et al., 2004; Bafna and Soni, 2016; Deng et al., 2016), graphene (Merchant et al., 2010), molybdenum disulfide (MoS2) (Feng et al., 2015), as well as various polymers (Choi et al., 2019). In contrast, membrane-embedded nanopores are assemblies spanning biological or semifluid membranes, typically with diameters below 10 nm. They are compatible with cell, organelle, and liposome applications and possess a great potential for biological functions and interactions (Banghart et al., 2004; Volgraf et al., 2006; Howorka, 2017). Nevertheless, the number of channels per square area cannot be controlled precisely, and they lack the chemical and environmental stability of their solid-state counterparts (Wanunu et al., 2010; Wang et al., 2011; Yusko et al., 2011; Wei et al., 2012a; Traversi et al., 2013; Heerema and Dekker, 2016; Ding et al., 2020). By reducing the bilayer patches (Urban et al., 2014) and using polymerized lipids (Daly et al., 2006; Heitz et al., 2010) or amphiphilic polymers (Meier et al., 2000; Nardin et al., 2000), the stability of membrane nanopores can be increased.
Membrane-embedded nanopores can either be engineered from biological channels and peptides or can be composed of building blocks of synthetic organic materials (Bayley and Jayasinghe, 2004; Gokel and Negin, 2013; Montenegro et al., 2013; Sakai and Matile, 2013; Vargas Jentzsch et al., 2013; Zhao et al., 2013). Protein-based nanopores are the current flagships in single-molecule studies and sequencing (Howorka and Siwy, 2009; Quick et al., 2016). They were fine-tuned by genetic engineering to enhance stability (Soskine et al., 2012), remove unnecessary moieties (Chen et al., 2008), and add new functional modules and structures (Moreau et al., 2008), or were modified by specific reactions to reduce current flickering (Spicer and Davis, 2014). Pioneering work in sequencing and sensing was performed with the heptameric pore α-hemolysin. However, the Mycobacterium smegmatis porin A (MspA) (Faller et al., 2004) and the Escherichia coli outer membrane curli secretion protein G (CsgG) (Goyal et al., 2014) replaced the original toxin pore in many applications in the field of DNA sequencing. Other channels, such as the outer membrane proteins OmpG and OmpF (Cowan et al., 1992; Liang and Tamm, 2007; Chen et al., 2008; Fahie et al., 2016; Perez-Rathke et al., 2018), aerolysin (Cao et al., 2016), or cytotoxic cytolysin A (ClyA) (Mueller et al., 2009; Bo et al., 2018), can also be used.
Protein pores cannot be easily engineered from scratch as the folding of a polypeptide chain in the quaternary structure is highly complex, and defined pores of >5 nm are very rare or experimentally challenging. For example, the release of proteins from therapeutic vesicles is yet not possible with biological channels (Mahendran et al., 2017). Here, the recently engineered DNA origami-based nanopores constitute a prosperous alternative, featuring the characteristics of simple self-assembly in a bottom-up fashion (Langecker et al., 2012; Burns et al., 2013; Seifert et al., 2015; Burns et al., 2016; Chidchob et al., 2016; Göpfrich et al., 2016a; Göpfrich et al., 2016b; Howorka, 2016; Krishnan et al., 2016; Diederichs et al., 2019; Thomsen et al., 2019; Chen et al., 2020). DNA nanopores can be precisely designed due to the predictable and programmable folding scheme based on simple base-pairing rules of DNA (Douglas et al., 2009a; Douglas et al., 2009b). De novo design generates structures of >20 nm (Rothemund, 2006; Seeman, 2010; Pinheiro et al., 2011; Chen et al., 2015; Jones et al., 2015), which are assembled by simple heating and cooling protocols in the classical scaffold and staple approach, according to which a long scaffold strand is folded with shorter staple nucleotides into a DNA structure (Rothemund, 2006). DNA nanostructures with controlled curvature and twists were generated (Dietz et al., 2009). The first membrane-embedded DNA nanopore was developed by the Simmel group (Langecker et al., 2012) (Figure 1A), directly followed by a six-helix bundle pore of the Howorka lab (Burns et al., 2013) (Figure 1B), likely indicating an independent parallel development. Both structures share a core of six hexagonally arranged DNA duplexes, differing in wall thickness and height (Langecker et al., 2012; Burns et al., 2013). However, also combinations of DNA origami structures or pores with solid-state nanopores or glass capillaries were developed (Bell et al., 2012; Wei et al., 2012b; Hernández-Ainsa et al., 2013; Hernández-Ainsa et al., 2014). For a detailed description see references (Bell and Keyser, 2014; Göpfrich and Keyser, 2019). Different pore widths were constructed, ranging from 0.5 to 9.6 nm (Krishnan et al., 2016; Göpfrich et al., 2016b; Thomsen et al., 2019), even allowing the translocation of folded proteins (Diederichs et al., 2019) or enabling the interaction with tumor cells (Guo et al., 2017). Nanopores with pore diameters of ∼4.0 and ∼7.5 nm are illustrated in Figures 1C,D (Krishnan et al., 2016; Diederichs et al., 2019). Lipid insertions were achieved by introducing anchors of cholesterol (Langecker et al., 2012; Burns et al., 2013; Burns et al., 2016), porphyrin (Burns et al., 2014; Seifert et al., 2015), or tocopherol (Krishnan et al., 2016). Despite successful incorporation in bilayers, aggregations of multiple pores were observed after attachment of lipophilic groups (Burns and Howorka, 2019; Ohmann et al., 2019). Other modifications such as the removal of negative charges at the backbone (Burns et al., 2013; Burns et al., 2014) or the replacement with neutral peptide nucleic acids were established (Ackermann and Famulok, 2013). By using complementary single strands acting as lock and key at the nanopore entrance, the efflux of small molecules through a DNA nanopore can be controlled, peaking in the discrimination of cargos based on their charges (Burns et al., 2016). The disadvantages of DNA as building blocks are the flexibility and loose packing of the structures so that ions can diffuse through their walls (Maingi et al., 2015; Yoo and Aksimentiev, 2015), which can be partially improved by thickening of the nanopore scaffold (Langecker et al., 2012). It is also reported that small water-filled channels can be formed at the interface between nanopore and membrane, allowing the passage of small molecules (Göpfrich et al., 2016a). The strengths and weaknesses of solid-state and membrane-embedded nanopores are summarized in Figure 2.
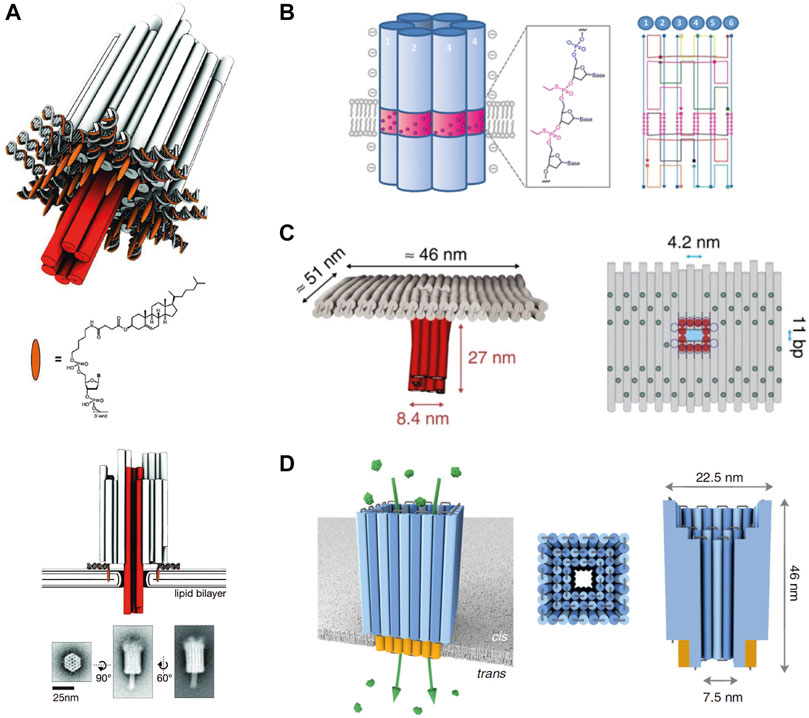
FIGURE 1. Architectures of membrane-embedded DNA nanopores. (A), Design and TEM images of the first described membrane-embedded DNA nanopore. Reprinted (adapted) with permission from (Langecker et al., 2012). (B), Schematic illustration and design of the second described cylindrical DNA nanopore. Reprinted (adapted) with permission from (Burns et al., 2013). Copyright (2021) American Chemical Society. (C), Architecture of the T-shaped DNA nanopore with a diameter of ∼4 nm. Reprinted (adapted) with permission from (Krishnan et al., 2016). (D), Protein-conductive DNA nanopore with a diameter of ∼7.5 nm. Reprinted (adapted) with permission from (Diederichs et al., 2019).
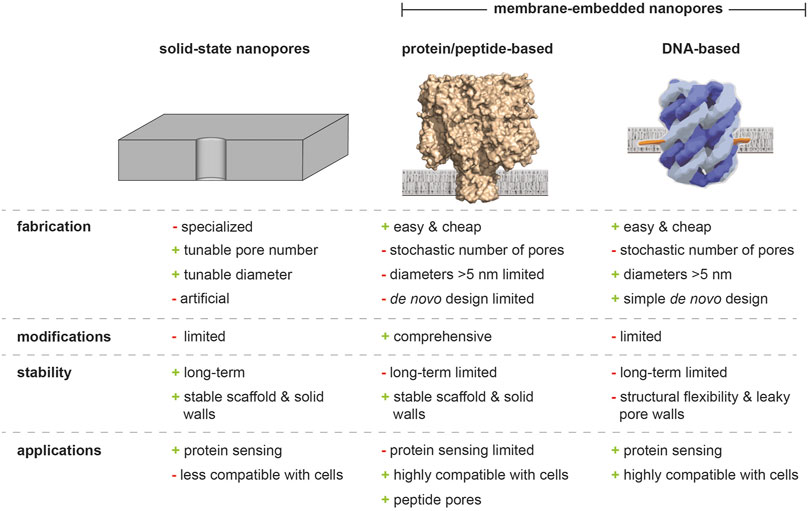
FIGURE 2. Solid-state, protein/peptide-based, and DNA nanopores. Advantages and weaknesses of solid-state nanopores, biological nanochannels, and DNA origami-based analogues are highlighted.
Microchip Arrays Suspended By Nanopore-Embedded Biological Membranes
Nanopores are often combined with microchips to ensure parallelization, high-throughput, and single-molecule resolution. Here, micro- or nanocavities are connected via solid-state nanopores with a second aqueous compartment. These nanostructures are fabricated in top-down or bottom-up approaches by photolithography or electron-beam lithography in combination with etching techniques, thermal oxidation, spin coating, and sputtering (Sharma et al., 2006; Buchholz et al., 2008; Herzer et al., 2010; Liebes-Peer et al., 2016; Xu et al., 2019). They provide benefits of miniaturized sizes, high reliability, parallelization, and biocompatibility (Jeon et al., 2010; Ho et al., 2013; Meng et al., 2014; Santos et al., 2015). By spanning lipid bilayers across these solid connections, translocation processes of membrane-embedded nanopores or membrane proteins became observable. These chip architectures are ideal for fluorescent-based high-throughput screenings as exemplified by α-hemolysin and the mechanosensitive channel of large conductance (MscL) (Kleefen et al., 2010; Urban et al., 2014; Diederichs et al., 2018). Membrane coverage across the nanoapertures is achieved by techniques such as lipid painting from organic solvents (Han et al., 2007), vesicle spreading in physiological buffers (Schmidt et al., 2000; Buchholz et al., 2008; Kleefen et al., 2010; Kumar et al., 2011; Wu et al., 2013), or shear-driven membrane formation (Jönsson et al., 2009). The self-organized vesicle spreading approach enabled the use of proteoliposomes resulting in solvent-free membranes harboring complex proteins. Here, the vesicle diameter is critical to successfully ensure coverage by simultaneously avoiding lipid intrusions (Urban et al., 2014; Janshoff and Steinem, 2015). The suspended membrane combines the advantages of increased stability provided by the solid support and high membrane fluidity created by the free-standing patches (Sun et al., 2020). Nevertheless, a tight seal for small ions could not be demonstrated so far. In contrast, painting requires the use of organic solvents, which can harm the function of membrane proteins, however, supports a tight membrane seal impermeable for small ions (Watanabe et al., 2014a).
Femtoliter Cavity-Based Microchips for Optical Recordings
The first cavity-based chips for optical single-channel recordings were developed in the 1990s mainly by the Peters group (Peters et al., 1990; Tschödrich-Rotter et al., 1996; Tschödrich-Rotter and Peters, 1998; Siebrasse and Peters, 2002; Kiskin et al., 2003; Peters, 2003). However, fabrication techniques regrading reliability, accuracy, and parallelism were limited. With the development of advanced fabrication methods, micro- and nanopore arrays with thousands of micro- or nanocavities were constructed and covered by lipid membranes (Buchholz et al., 2008; Sumitomo et al., 2012; Watanabe et al., 2014b). These microchip systems allow high-throughput screening applications for individual translocation processes as exemplified by the bacterial pore α-hemolysin (Kleefen et al., 2010; Watanabe et al., 2014a) and MscL (Urban et al., 2014). Applications are not limited to the analysis of channels and transporters, as cell-penetrating peptides (Kolesinska et al., 2013) or viral capsid membrane fusion and cargo release were investigated with cavity-based chips (Kusters et al., 2014). The Noji lab developed carbon fluorine polymer (CYTOP) coated chips by combining microfluidics and sequential liquid injections (Soga et al., 2015; Watanabe et al., 2016). First, an aqueous solution is infused to fill the cavities with substrates, followed by phospholipids dissolved in chloroform, creating a lipid monolayer at the chamber opening due to the hydrophobicity of the coated material. Finally, a second aqueous solution is injected forming a bilayer at the cavity entrance, whereas a monolayer is formed elsewhere (Watanabe, 2020). Asymmetric membranes were generated by introducing a second chloroform-based solution supplemented with different lipid species (Watanabe et al., 2014b). In addition to the bacterial pore α-hemolysin, the FoF1-ATP synthase (Watanabe et al., 2014a) and the phospholipid scramblase TMEM16F were examined by this approach (Watanabe et al., 2018b). Based on the advection-diffusion model, the sequential injection of aqueous solutions with various concentrations of analytes allows to produce concentration gradients of encapsulated molecules across the chip (Watanabe et al., 2018a). Recently, the platform was used to measure single-protein activities in high-throughput by encapsulating soluble enzymes in the microchambers (Sakamoto et al., 2020) or creating hybrid cell reactor systems (Moriizumi et al., 2018).
Despite these multiple applications, the use of organic solvent does not allow the investigation of complex and fragile membrane proteins as required for biomedical screenings. In general, the vesicle-spreading method is superior, as delicate transport complexes can be reconstituted beforehand in proteoliposomes followed by SLB formation as shown in Urban et al. (2014). For vesicle spreading, different chip architectures exist, varying in cavity shape, volume, nanopore diameter, and surface roughness. A comparison of two chip designs published by Urban et al. (2014) and Diederichs et al. (2018) is displayed in Figure 3. The latest silicon chip features truncated inverted pyramidal cavities of ∼50 fL volumes, which are connected by a solid-state nanopore of 80 nm with a buffer reservoir. The transparent Si3N4 top and SiO2 bottom layers allow readout of both compartments, whereas the second chip architecture displays cylindrical cavities of ∼6–15 fL with nanopores ranging in diameter between 70 and 120 nm. A large difference was observed in the surface roughness which is ∼3.6 nm for the opaque chip (Figure 3A), whereas the most recent chip design exhibits a surface roughness of ∼0.2 nm (Figure 3B). These extremely smooth, low strain surfaces in combination with well-defined and tapered pore edges provide optimal conditions for a high coverage upon membrane spreading.
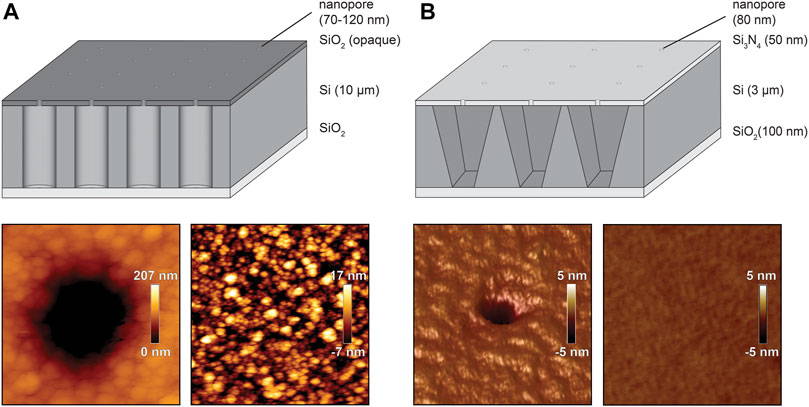
FIGURE 3. Comparison of different silicon chip architectures. Schematic illustration of the silicon chips (dimensions are not drawn in scale) published by Urban et al. (2014) and Diederichs et al. (2018) including AFM roughness analysis of the vicinity and in distance of the nanopore (Urban et al., 2014; Reprinted (adpated) with permission form Diederichs et al., 2018. Copyright (2021) American Chemical Society). (A), Silicon-on-insulator chip featuring cylindrical cavities of 6–15 fL and the including AFM images. (B), Transparent silicon chip featuring an inverted pyramidal shape of 50 fL.
Membrane-Embedded DNA Nanopores Analyzed by Microchips
The recently designed silicon microcavity array was used to analyze translocation processes of two different DNA nanopores at single-channel resolution in high-throughput. The first investigated DNA pore is composed of six interconnected oligonucleotides forming a channel with a height of ∼9.0 nm, a width of ∼5.0 nm, and an inner diameter of ∼1.8 nm (Diederichs et al., 2021). Cholesterol-functionalized DNA strands were attached to drive membrane insertion. This six-helix bundle was used to elucidate how translocation is affected by the analytes’ charge and size. Three different organic fluorophores possessing different net charges and sizes were studied. The translocation rate through the negatively charged DNA nanopore decreased with increasing negative net charges of the analytes. The (-1)-charged fluorophore ATTO655 fluxes faster than the (-3)-charged dye FAM, whereas the (-4)-charged complexometric calcium indicator Calcein cannot translocate through the DNA nanopore (Figures 4A,B) (Diederichs et al., 2021). This charge-dependent effect is most likely based on repulsion with the negatively charged pore walls of the DNA backbone. However, only a minor effect of the translocation is assumed for analytes with small numbers of net charges, as the flux of the (-1)-charged ATTO655 is only ∼2.5 times faster through the protein pore α-hemolysin, after correction for the cross-sectional area of the pore lumen (Diederichs et al., 2021). Strikingly, the lipid environment affects the translocation properties of the DNA nanopore, as 30 mol% of the negatively charged 1-palmitoyl-2-oleoyl-sn-glycero-3-phospho-(1′-rac-glycerol) (POPG) limits the translocation of the (-3)-charged dye FAM (Diederichs et al., 2021). POPG is expected to cause a constriction of the pore lumen based on electrostatic compensation between lipid and DNA backbone. Thereby, a direct effect of the lipid environment on translocation properties of DNA nanopores was demonstrated (Diederichs et al., 2021).
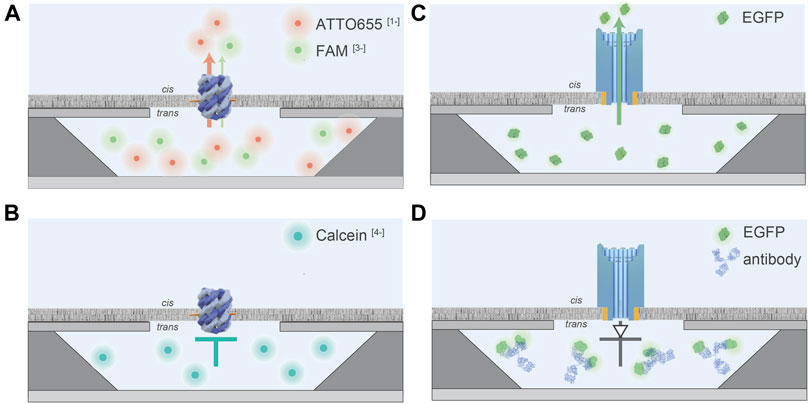
FIGURE 4. Schematic illustration of flux characteristics of DNA nanopores measured by the silicon chip array. Dimensions of the silicon chip and of the nanopores are not drawn in scale. (A), The co-flux experiment of the (-1)-charged ATTO655 (red sphere) and the (-3)-charged FAM (green sphere) through the small six helix DNA nanopore. A faster flux of ATTO655-1 was observed in comparison to FAM-3. (B), The (-4)-charged Calcein (blue sphere) cannot flux through the small DNA nanopore. (C), EGFP can flux through the DNA nanofunnel. (D), Antibodies against the His-tag of His6EGFP can be used to trap the protein inside the nanoconfinement as the complex cannot penetrate the pore. This system acts as a molecular diode allowing unidirectional translocation.
Small pores are well suitable for DNA sequencing due to the tight fitting within the translocation pore (Clarke et al., 2009; Quick et al., 2016). However, bigger pores provide the opportunity for stochastic sensing of proteins and complexes. Currently, biological pores are rather unsuitable for the passage of folded proteins based on their heterogeneities in diameter and due to their complex structures (Knockenhauer and Schwartz, 2016; Kosinski et al., 2016; Lukoyanova et al., 2016; Mahendran et al., 2017). Recently, the new nanopore silicon chip was used to demonstrate translocation of folded EGFP molecules through an artificial DNA nanopore (Diederichs et al., 2019). The latter resembles a funnel with a narrow and wide opening of 7.5 and 22.5 nm and a total height of 46 nm (Diederichs et al., 2019). Smooth membrane insertion was ensured by 24 cholesterol anchors, and the stability of the pore was guaranteed by multiple layers of duplexes to increase the wall thickness. A remarkable number of ∼66 EGFP molecules can flux through the DNA assembly per second (Diederichs et al., 2019) (Figure 4C). To mediate transport surpassing the equilibrium of passive diffusion, DNA nanopore protein translocation was coupled to biomolecular antibody recognition, allowing the affinity-based accumulation or trapping of EGFP molecules in the nanoconfinements (Diederichs et al., 2019) (Figure 4D). Current limitations of the recently developed silicon-based cavity array are the comparatively large cavity volumes of 50 fL, permitting the visualization of substrate translocation processes greater than one per second. Additionally, the characterization of transport processes of secondary active transporters is difficult as the membrane does not seal the cavity for small ions, including protons.
Future Prospective
In general, the field of solid-state nanopores and biological counterparts has grown tremendously. Some years ago, it was not imaginable that synthetic pores would ever reach transport behaviors of biological channels (Tagliazucchi and Szleifer, 2015). The multitude of applications and the chemical and physical stimuli to gate conductance equals or exceeds multiple properties of their biological counterparts (Perez Sirkin et al., 2020). Nevertheless, synthetic devices are behind biological pores in ion selectivity, signal amplification, transport of larger analytes, specificity, and many more (Perez Sirkin et al., 2020). Biological analogs inspire research and drive the design of new nanopores also in the field of protein sensing, as it was shown for solid-state nanopores and DNA funnels (Diederichs et al., 2019; Zeng et al., 2019). More and more sensing and sequencing applications with a multitude of analytes are being published. Nevertheless, the possibilities of transferring the knowledge of structural aspects to functional properties are still limited. Especially the research on DNA nanopores has to be intensified regarding controllability, stability, and functionalization to pave the way for diagnostic applications. The detection of cancer biomarkers from blood samples derived from DNA-assisted nanopores, which can also be used for the development of drug-delivery systems, is a good example for this (Liu et al., 2018; Ding et al., 2020).
By combining solid-state nanopores with microchip systems, single-molecule resolution can be linked with high-throughput and microfluidics. In future, smaller microchips with drastically reduced volumes will be fabricated allowing to characterize medical relevant transporters or to screen lipid membrane conditions affecting the translocation properties of membrane proteins. A promising direction for optical single-molecule detection with nanopores are zero-mode waveguides (ZMWs). ZMWs are small holes in metal films with sub-wavelength apertures for nanophotonic and sensing applications (Assad et al., 2017; Larkin et al., 2017; Han et al., 2019; Spitzberg et al., 2019) as commercialized by Pacific Biosciences for DNA sequencing (Levene et al., 2003; Foquet et al., 2008; Korlach et al., 2008; Lundquist et al., 2008; Eid et al., 2009; Metzker, 2010; Uemura et al., 2010). As the wavelength of light is smaller than the nanoaperture itself, light cannot penetrate in the confined chamber, which results in an exponential decay of the optical field creating a zeptoliter volume (Levene et al., 2003; Foquet et al., 2008). By immobilization of the DNA polymerase at the bottom of these cavities, only photolabeled molecules in the first tens of nanometer can be observed (Korlach et al., 2008; Eid et al., 2009). Sensing applications with ZMWs bear the risk of unspecific binding and biocompatibility, however, protein sensing has recently been demonstrated with palladium ZMWs indicating a promising direction (Klughammer and Dekker, 2021).
Author Contributions
TD and RT wrote the manuscript. RT conceived and supervised the work.
Funding
This research was supported by the German Research Foundation (SFB 807, TA 157/12-1, and GRK 1986 to RT), the LOEWE program (DynaMem A3 to RT) and ERC Advanced Grant (789121 to RT).
Conflict of Interest
The authors declare that the research was conducted in the absence of any commercial or financial relationships that could be construed as a potential conflict of interest.
Acknowledgments
We thank Michael Urban for providing the AFM images of the opaque silicon-on-insulator chip and Elina Nürenberg-Goloub, Ma. Florencia Sanchez, Ralph Wieneke, Andrea Pott, Inga Nold, and all members of the Institute for Biochemistry, Goethe University Frankfurt for discussion and comments.
References
Ackermann, D., and Famulok, M. (2013). Pseudo-Complementary PNA Actuators as Reversible Switches in Dynamic DNA Nanotechnology. Nucleic Acids Res. 41, 4729–4739. doi:10.1093/nar/gkt121
Amadei, C. A., Montessori, A., Kadow, J. P., Succi, S., and Vecitis, C. D. (2017). Role of Oxygen Functionalities in Graphene Oxide Architectural Laminate Subnanometer Spacing and Water Transport. Environ. Sci. Technol. 51, 4280–4288. doi:10.1021/acs.est.6b05711
Assad, O. N., Gilboa, T., Spitzberg, J., Juhasz, M., Weinhold, E., and Meller, A. (2017). Light-Enhancing Plasmonic-Nanopore Biosensor for Superior Single-Molecule Detection. Adv. Mater. 29, 1605442. doi:10.1002/adma.201605442
Bafna, J. A., and Soni, G. V. (2016). Fabrication of Low Noise Borosilicate Glass Nanopores for Single Molecule Sensing. PLoS One 11, e0157399. doi:10.1371/journal.pone.0157399
Banghart, M., Borges, K., Isacoff, E., Trauner, D., and Kramer, R. H. (2004). Light-Activated Ion Channels for Remote Control of Neuronal Firing. Nat. Neurosci. 7, 1381–1386. doi:10.1038/nn1356
Bayley, H., and Jayasinghe, L. (2004). Functional Engineered Channels and Pores (Review). Mol. Membr. Biol. 21, 209–220. doi:10.1080/09687680410001716853
Bell, N. A. W., Engst, C. R., Ablay, M., Divitini, G., Ducati, C., Liedl, T., et al. (2012). DNA Origami Nanopores. Nano Lett. 12, 512–517. doi:10.1021/nl204098n
Bell, N. A. W., and Keyser, U. F. (2014). Nanopores Formed by DNA Origami: A Review. FEBS Lett. 588, 3564–3570. doi:10.1016/j.febslet.2014.06.013
Buchholz, K., Tinazli, A., Kleefen, A., Dorfner, D., Pedone, D., Rant, U., et al. (2008). Silicon-On-Insulator Based Nanopore Cavity Arrays for Lipid Membrane Investigation. Nanotechnology 19, 445305. doi:10.1088/0957-4484/19/44/445305
Burns, J., and Howorka, S. (2019). Structural and Functional Stability of DNA Nanopores in Biological Media. Nanomaterials 9, 490. doi:10.3390/nano9040490
Burns, J. R., Al-Juffali, N., Janes, S. M., and Howorka, S. (2014). Membrane-Spanning DNA Nanopores with Cytotoxic Effect. Ang. Chem. Int. Ed. 53, 12466–12470. doi:10.1002/anie.201405719
Burns, J. R., Seifert, A., Fertig, N., and Howorka, S. (2016). A Biomimetic DNA-Based Channel for the Ligand-Controlled Transport of Charged Molecular Cargo across a Biological Membrane. Nat. Nanotech 11, 152–156. doi:10.1038/nnano.2015.279
Burns, J. R., Stulz, E., and Howorka, S. (2013). Self-Assembly DNA Nanopores that Span Lipid Bilayers. Nano Lett. 13, 2351–2356. doi:10.1021/nl304147f
Caglar, M., Silkina, I., Brown, B. T., Thorneywork, A. L., Burton, O. J., Babenko, V., et al. (2020). Tunable Anion-Selective Transport through Monolayer Graphene and Hexagonal Boron Nitride. ACS Nano 14, 2729–2738. doi:10.1021/acsnano.9b08168
Cao, C., Ying, Y.-L., Hu, Z.-L., Liao, D.-F., Tian, H., and Long, Y.-T. (2016). Discrimination of Oligonucleotides of Different Lengths with a Wild-Type Aerolysin Nanopore. Nat. Nanotech 11, 713–718. doi:10.1038/nnano.2016.66
Chang, H., Kosari, F., Andreadakis, G., Alam, M. A., Vasmatzis, G., and Bashir, R. (2004). DNA-Mediated Fluctuations in Ionic Current through Silicon Oxide Nanopore Channels. Nano Lett. 4, 1551–1556. doi:10.1021/nl049267c
Chen, L., Liang, S., Chen, Y., Wu, M., and Zhang, Y. (2020). Destructing the Plasma Membrane with Activatable Vesicular DNA Nanopores. ACS Appl. Mater. Inter. 12, 96–105. doi:10.1021/acsami.9b14746
Chen, M., Khalid, S., Sansom, M. S. P., and Bayley, H. (2008). Outer Membrane Protein G: Engineering a Quiet Pore for Biosensing. Proc. Natl. Acad. Sci. USA 105, 6272–6277. doi:10.1073/pnas.0711561105
Chen, Y.-J., Groves, B., Muscat, R. A., and Seelig, G. (2015). DNA Nanotechnology from the Test Tube to the Cell. Nat. Nanotech 10, 748–760. doi:10.1038/nnano.2015.195
Cherf, G. M., Lieberman, K. R., Rashid, H., Lam, C. E., Karplus, K., and Akeson, M. (2012). Automated Forward and Reverse Ratcheting of DNA in a Nanopore at 5-Å Precision. Nat. Biotechnol. 30, 344–348. doi:10.1038/nbt.2147
Chidchob, P., Edwardson, T. G. W., Serpell, C. J., and Sleiman, H. F. (2016). Synergy of Two Assembly Languages in DNA Nanostructures: Self-Assembly of Sequence-Defined Polymers on DNA Cages. J. Am. Chem. Soc. 138, 4416–4425. doi:10.1021/jacs.5b12953
Choi, J., Lee, C. C., and Park, S. (2019). Scalable Fabrication of Sub-10 nm Polymer Nanopores for DNA Analysis. Microsyst. Nanoeng. 5, 12. doi:10.1038/s41378-019-0050-9
Clarke, J., Wu, H.-C., Jayasinghe, L., Patel, A., Reid, S., and Bayley, H. (2009). Continuous Base Identification for Single-Molecule Nanopore DNA Sequencing. Nat. Nanotech 4, 265–270. doi:10.1038/nnano.2009.12
Cowan, S. W., Schirmer, T., Rummel, G., Steiert, M., Ghosh, R., Pauptit, R. A., et al. (1992). Crystal Structures Explain Functional Properties of Two E. coli Porins. Nature 358, 727–733. doi:10.1038/358727a0
Daly, S. M., Heffernan, L. A., Barger, W. R., and Shenoy, D. K. (2006). Photopolymerization of Mixed Monolayers and Black Lipid Membranes Containing Gramicidin A and Diacetylenic Phospholipids. Langmuir 22, 1215–1222. doi:10.1021/la052327p
Deamer, D., Akeson, M., and Branton, D. (2016). Three Decades of Nanopore Sequencing. Nat. Biotechnol. 34, 518–524. doi:10.1038/nbt.3423
Deng, T., Wang, Y., Chen, Q., Chen, H., and Liu, Z. (2016). Massive Fabrication of Silicon Nanopore Arrays with Tunable Shapes. Appl. Surf. Sci. 390, 681–688. doi:10.1016/j.apsusc.2016.07.171
Diederichs, T., Ahmad, K., Burns, J. R., Nguyen, Q. H., Siwy, Z., Tornow, M., et al. (2021). Principles of Small-Molecule Translocation through Synthetic Membrane Pores. submitted
Diederichs, T., Nguyen, Q. H., Urban, M., Tampé, R., and Tornow, M. (2018). Transparent Nanopore Cavity Arrays Enable Highly Parallelized Optical Studies of Single Membrane Proteins on Chip. Nano Lett. 18, 3901–3910. doi:10.1021/acs.nanolett.8b01252
Diederichs, T., Pugh, G., Dorey, A., Xing, Y., Burns, J. R., Hung Nguyen, Q., et al. (2019). Synthetic Protein-Conductive Membrane Nanopores Built with DNA. Nat. Commun. 10, 5018. doi:10.1038/s41467-019-12639-y
Dietz, H., Douglas, S. M., and Shih, W. M. (2009). Folding DNA into Twisted and Curved Nanoscale Shapes. Science 325, 725–730. doi:10.1126/science.1174251
Ding, T., Yang, J., Pan, V., Zhao, N., Lu, Z., Ke, Y., et al. (2020). DNA Nanotechnology Assisted Nanopore-Based Analysis. Nucleic Acids Res. 48, 2791–2806. doi:10.1093/nar/gkaa095
Dong, G., Hou, J., Wang, J., Zhang, Y., Chen, V., and Liu, J. (2016). Enhanced CO2/N2 Separation by Porous Reduced Graphene Oxide/Pebax Mixed Matrix Membranes. J. Membr. Sci. 520, 860–868. doi:10.1016/j.memsci.2016.08.059
Douglas, S. M., Dietz, H., Liedl, T., Högberg, B., Graf, F., and Shih, W. M. (2009a). Self-Assembly of DNA into Nanoscale Three-Dimensional Shapes. Nature 459, 414–418. doi:10.1038/nature08016
Douglas, S. M., Marblestone, A. H., Teerapittayanon, S., Vazquez, A., Church, G. M., and Shih, W. M. (2009b). Rapid Prototyping of 3D DNA-Origami Shapes with caDNAno. Nucleic Acids Res. 37, 5001–5006. doi:10.1093/nar/gkp436
Eid, J., Fehr, A., Gray, J., Luong, K., Lyle, J., Otto, G., et al. (2009). Real-Time DNA Sequencing from Single Polymerase Molecules. Science 323, 133–138. doi:10.1126/science.1162986
Fahie, M. A., Yang, B., Pham, B., and Chen, M. (2016). Tuning the Selectivity and Sensitivity of an OmpG Nanopore Sensor by Adjusting Ligand Tether Length. ACS Sens. 1, 614–622. doi:10.1021/acssensors.6b00014
Faller, M., Niederweis, M., and Schulz, G. E. (2004). The Structure of a Mycobacterial Outer-Membrane Channel. Science 303, 1189–1192. doi:10.1126/science.1094114
Feng, J., Liu, K., Bulushev, R. D., Khlybov, S., Dumcenco, D., Kis, A., et al. (2015). Identification of Single Nucleotides in MoS2 Nanopores. Nat. Nanotech 10, 1070–1076. doi:10.1038/nnano.2015.219
Foquet, M., Samiee, K. T., Kong, X., Chauduri, B. P., Lundquist, P. M., Turner, S. W., et al. (2008). Improved Fabrication of Zero-Mode Waveguides for Single-Molecule Detection. J. Appl. Phys. 103, 034301. doi:10.1063/1.2831366
Gokel, G. W., and Negin, S. (2013). Synthetic Ion Channels: From Pores to Biological Applications. Acc. Chem. Res. 46, 2824–2833. doi:10.1021/ar400026x
Göpfrich, K., and Keyser, U. F. (2019). DNA Nanotechnology for Building Sensors, Nanopores and Ion-Channels. In Advances In Experimental Medicine And Biology, 1174:331–370. doi:10.1007/978-981-13-9791-2_11
Göpfrich, K., Li, C.-Y., Mames, I., Bhamidimarri, S. P., Ricci, M., Yoo, J., et al. (2016a). Ion Channels Made from a Single Membrane-Spanning DNA Duplex. Nano Lett. 16, 4665–4669. doi:10.1021/acs.nanolett.6b02039
Göpfrich, K., Li, C.-Y., Ricci, M., Bhamidimarri, S. P., Yoo, J., Gyenes, B., et al. (2016b). Large-Conductance Transmembrane Porin Made from DNA Origami. ACS Nano 10, 8207–8214. doi:10.1021/acsnano.6b03759
Goyal, P., Krasteva, P. V., Van Gerven, N., Gubellini, F., Van Den Broeck, I., Troupiotis-Tsaïlaki, A., et al. (2014). Structural and Mechanistic Insights into the Bacterial Amyloid Secretion Channel CsgG. Nature 516, 250–253. doi:10.1038/nature13768
Guo, X.-L., Yuan, D.-D., Song, T., and Li, X.-M. (2017). DNA Nanopore Functionalized with Aptamer and Cell-Penetrating Peptide for Tumor Cell Recognition. Anal. Bioanal. Chem. 409, 3789–3797. doi:10.1007/s00216-017-0321-y
Han, D., Crouch, G. M., Chao, Z., Fullerton-Shirey, S. K., Go, D. B., and Bohn, P. W. (2019). Nanopore-templated Silver Nanoparticle Arrays Photopolymerized in Zero-Mode Waveguides. Front. Chem. 7, 216. doi:10.3389/fchem.2019.00216
Han, X., Studer, A., Sehr, H., Geissbühler, I., Di Berardino, M., Winkler, F. K., et al. (2007). Nanopore Arrays for Stable and Functional Free-Standing Lipid Bilayers. Adv. Mater. 19, 4466–4470. doi:10.1002/adma.200700468
Heerema, S. J., and Dekker, C. (2016). Graphene Nanodevices for DNA Sequencing. Nat. Nanotech 11, 127–136. doi:10.1038/nnano.2015.307
Heitz, B. A., Jones, I. W., Hall, H. K., Aspinwall, C. A., and Saavedra, S. S. (2010). Fractional Polymerization of a Suspended Planar Bilayer Creates a Fluid, Highly Stable Membrane for Ion Channel Recordings. J. Am. Chem. Soc. 132, 7086–7093. doi:10.1021/ja100245d
Hernández-Ainsa, S., Bell, N. A. W., Thacker, V. V., Göpfrich, K., Misiunas, K., Fuentes-Perez, M. E., et al. (2013). DNA Origami Nanopores for Controlling DNA Translocation. ACS Nano 7, 6024–6030. doi:10.1021/nn401759r
Hernández-Ainsa, S., Misiunas, K., Thacker, V. V., Hemmig, E. A., and Keyser, U. F. (2014). Voltage-Dependent Properties of DNA Origami Nanopores. Nano Lett. 14, 1270–1274. doi:10.1021/nl404183t
Herzer, N., Hoeppener, S., and Schubert, U. S. (2010). Fabrication of Patterned Silane Based Self-Assembled Monolayers by Photolithography and Surface Reactions on Silicon-Oxide Substrates. Chem. Commun. 46, 5634–5652. doi:10.1039/c0cc00674b
Ho, J.-W., Wee, Q., Dumond, J., Tay, A., and Chua, S.-J. (2013). Versatile Pattern Generation of Periodic, High Aspect Ratio Si Nanostructure Arrays with Sub-50-nm Resolution on a Wafer Scale. Nanoscale Res. Lett. 8, 506. doi:10.1186/1556-276X-8-506
Howorka, S. (2017). Building Membrane Nanopores. Nat. Nanotech. 12, 619–630. doi:10.1038/nnano.2017.99
Howorka, S., and Siwy, Z. (2009). Nanopore Analytics: Sensing of Single Molecules. Chem. Soc. Rev. 38, 2360–2384. doi:10.1039/b813796j
Janshoff, A., and Steinem, C. (2015). Mechanics of Lipid Bilayers: What Do We Learn from Pore-Spanning Membranes?. Biochim. Biophys. Acta (Bba) - Mol. Cel Res. 1853, 2977–2983. doi:10.1016/j.bbamcr.2015.05.029
Jeon, H.-J., Kim, K. H., Baek, Y.-K., Kim, D. W., and Jung, H.-T. (2010). New Top-Down Approach for Fabricating High-Aspect-Ratio Complex Nanostructures with 10 nm Scale Features. Nano Lett. 10, 3604–3610. doi:10.1021/nl1025776
Jones, M. R., Seeman, N. C., and Mirkin, C. A. (2015). Programmable Materials and the Nature of the DNA Bond. Science 347, 1260901. doi:10.1126/science.1260901
Jönsson, P., Beech, J. P., Tegenfeldt, J. O., and Höök, F. (2009). Shear-Driven Motion of Supported Lipid Bilayers in Microfluidic Channels. J. Am. Chem. Soc. 131, 5294–5297. doi:10.1021/ja809987b
Kiskin, N. I., Siebrasse, J. P., and Peters, R. (2003). Optical Microwell Assay of Membrane Transport Kinetics. Biophysical J. 85, 2311–2322. doi:10.1016/S0006-3495(03)74655-4
Kleefen, A., Pedone, D., Grunwald, C., Wei, R., Firnkes, M., Abstreiter, G., et al. (2010). Multiplexed Parallel Single Transport Recordings on Nanopore Arrays. Nano Lett. 10, 5080–5087. doi:10.1021/nl1033528
Klughammer, N., and Dekker, C. (2021). Palladium Zero-Mode Waveguides for Optical Single-Molecule Detection with Nanopores. Nanotechnology 32, 18LT01. doi:10.1088/1361-6528/abd976
Knockenhauer, K. E., and Schwartz, T. U. (2016). The Nuclear Pore Complex as a Flexible and Dynamic Gate. Cell 164, 1162–1171. doi:10.1016/j.cell.2016.01.034
Kolesinska, B., Podwysocka, D. J., Rueping, M. A., Seebach, D., Kamena, F., Walde, P., et al. (2013). Permeation through Phospholipid Bilayers, Skin-Cell Penetration, Plasma Stability, and CD Spectra of alpha and beta Oligoproline Derivatives. Chem. Biodiversity 10, 1–38. doi:10.1002/cbdv.201200393
Korlach, J., Marks, P. J., Cicero, R. L., Gray, J. J., Murphy, D. L., Roitman, D. B., et al. (2008). Selective Aluminum Passivation for Targeted Immobilization of Single DNA Polymerase Molecules in Zero-Mode Waveguide Nanostructures. Proc. Natl. Acad. Sci. USA 105, 1176–1181. doi:10.1073/pnas.0710982105
Kosinski, J., Mosalaganti, S., Von Appen, A., Teimer, R., Diguilio, A. L., Wan, W., et al. (2016). Molecular Architecture of the Inner Ring Scaffold of the Human Nuclear Pore Complex. Science 352, 363–365. doi:10.1126/science.aaf0643
Krishnan, S., Ziegler, D., Arnaut, V., Martin, T. G., Kapsner, K., Henneberg, K., et al. (2016). Molecular Transport through Large-Diameter DNA Nanopores. Nat. Commun. 7, 12787. doi:10.1038/ncomms12787
Kumar, K., Isa, L., Egner, A., Schmidt, R., Textor, M., and Reimhult, E. (2011). Formation of Nanopore-Spanning Lipid Bilayers through Liposome Fusion. Langmuir 27, 10920–10928. doi:10.1021/la2019132
Kusters, I., van Oijen, A. M., and Driessen, A. J. M. (2014). Membrane-On-A-Chip: Microstructured Silicon/silicon-Dioxide Chips for High-Throughput Screening of Membrane Transport and Viral Membrane Fusion. ACS Nano 8, 3380–3392. doi:10.1021/nn405884a
Langecker, M., Arnaut, V., Martin, T. G., List, J., Renner, S., Mayer, M., et al. (2012). Synthetic Lipid Membrane Channels Formed by Designed DNA Nanostructures. Science 338, 932–936. doi:10.1126/science.1225624
Larkin, J., Henley, R. Y., Jadhav, V., Korlach, J., and Wanunu, M. (2017). Length-independent DNA Packing into Nanopore Zero-Mode Waveguides for Low-Input DNA Sequencing. Nat. Nanotech. 12, 1169–1175. doi:10.1038/NNANO.2017.176
Levene, M. J., Korlach, J., Turner, S. W., Foquet, M., Craighead, H. G., and Webb, W. W. (2003). Zero-Mode Waveguides for Single-Molecule Analysis at High Concentrations. Science 299, 682–686. doi:10.1126/science.1079700
Li, J., Gershow, M., Stein, D., Brandin, E., and Golovchenko, J. A. (2003). DNA Molecules and Configurations in a Solid-State Nanopore Microscope. Nat. Mater. 2, 611–615. doi:10.1038/nmat965
Liang, B., and Tamm, L. K. (2007). Structure of Outer Membrane Protein G by Solution NMR Spectroscopy. Proc. Natl. Acad. Sci. USA 104, 16140–16145. doi:10.1073/pnas.0705466104
Liebes-Peer, Y., Bandalo, V., Sökmen, Ü., Tornow, M., and Ashkenasy, N. (2016). Fabrication of Nanopores in Multi-Layered Silicon-Based Membranes Using Focused Electron Beam Induced Etching with XeF2 Gas. Microchim. Acta 183, 987–994. doi:10.1007/s00604-015-1557-x
Litvinchuk, S., Tanaka, H., Miyatake, T., Pasini, D., Tanaka, T., Bollot, G., et al. (2007). Synthetic Pores with Reactive Signal Amplifiers as Artificial Tongues. Nat. Mater 6, 576–580. doi:10.1038/nmat1933
Liu, L., Li, T., Zhang, S., Song, P., Guo, B., Zhao, Y., et al. (2018). Simultaneous Quantification of Multiple Cancer Biomarkers in Blood Samples through DNA-Assisted Nanopore Sensing. Angew. Chem. Int. Ed. 57, 11882–11887. doi:10.1002/anie.201803324
Lu, B., Stokes, C., Fahie, M., Chen, M., Golovchenko, J. A., and Hau, L. V. (2018). Protein Motion and Configurations in a Form-Fitting Nanopore: Avidin in ClyA. Biophys. J. 115, 801–808. doi:10.1016/j.bpj.2018.07.024
Lukoyanova, N., Hoogenboom, B. W., and Saibil, H. R. (2016). The Membrane Attack Complex, Perforin and Cholesterol-Dependent Cytolysin Superfamily of Pore-Forming Proteins. J. Cel Sci. 129, 2125–2133. doi:10.1242/jcs.182741
Lundquist, P. M., Zhong, C. F., Zhao, P., Tomaney, A. B., Peluso, P. S., Dixon, J., et al. (2008). Parallel Confocal Detection of Single Molecules in Real Time. Opt. Lett. 33, 1026–1028. doi:10.1364/ol.33.001026
Mahendran, K. R., Niitsu, A., Kong, L., Thomson, A. R., Sessions, R. B., Woolfson, D. N., et al. (2017). A Monodisperse Transmembrane α-helical Peptide Barrel. Nat. Chem. 9, 411–419. doi:10.1038/nchem.2647
Maingi, V., Lelimousin, M., Howorka, S., and Sansom, M. S. P. (2015). Gating-Like Motions and wall Porosity in a DNA Nanopore Scaffold Revealed by Molecular Simulations. ACS Nano 9, 11209–11217. doi:10.1021/acsnano.5b06357
Manrao, E. A., Derrington, I. M., Laszlo, A. H., Langford, K. W., Hopper, M. K., Gillgren, N., et al. (2012). Reading DNA at Single-Nucleotide Resolution with a Mutant MspA Nanopore and Phi29 DNA Polymerase. Nat. Biotechnol. 30, 349–353. doi:10.1038/nbt.2171
Meier, W., Nardin, C., and Winterhalter, M. (2000). Reconstitution of Channel Proteins in (Polymerized) ABA Triblock Copolymer Membranes. Angew. Chem. Int. Ed.2-Y. 39, 4599–4602. doi:10.1002/1521-3773(20001215)39:24<4599::AID-ANIE4599>3.0.CO;2-Y
Meng, L., He, X., Li, C., Li, J., Hong, P., Li, J., et al. (2014). Transistor Gate Line Roughness Formation and Reduction in Sub-30-nm Gate Patterning Using Multilayer Hard Mask Structure. J. Micro/Nanolith. MEMS MOEMS 13, 033010. doi:10.1117/1.jmm.13.3.033010
Merchant, C. A., Healy, K., Wanunu, M., Ray, V., Peterman, N., Bartel, J., et al. (2010). DNA Translocation through Graphene Nanopores. Nano Lett. 10, 2915–2921. doi:10.1021/nl101046t
Metzker, M. L. (2010). Sequencing Technologies - The Next Generation. Nat. Rev. Genet. 11, 31–46. doi:10.1038/nrg2626
Miles, B. N., Ivanov, A. P., Wilson, K. A., Doğan, F., Japrung, D., and Edel, J. B. (2013). Single Molecule Sensing with Solid-State Nanopores: Novel Materials, Methods, and Applications. Chem. Soc. Rev. 42, 15–28. doi:10.1039/c2cs35286a
Montenegro, J., Ghadiri, M. R., and Granja, J. R. (2013). Ion Channel Models Based on Self-Assembling Cyclic Peptide Nanotubes. Acc. Chem. Res. 46, 2955–2965. doi:10.1021/ar400061d
Moreau, C. J., Dupuis, J. P., Revilloud, J., Arumugam, K., and Vivaudou, M. (2008). Coupling Ion Channels to Receptors for Biomolecule Sensing. Nat. Nanotech 3, 620–625. doi:10.1038/nnano.2008.242
Moriizumi, Y., Tabata, K. V., Watanabe, R., Doura, T., Kamiya, M., Urano, Y., et al. (2018). Hybrid Cell Reactor System from Escherichia coli Protoplast Cells and Arrayed Lipid Bilayer Chamber Device. Sci. Rep. 8, 11757. doi:10.1038/s41598-018-30231-0
Movileanu, L. (2009). Interrogating Single Proteins through Nanopores: Challenges and Opportunities. Trends Biotechnol. 27, 333–341. doi:10.1016/j.tibtech.2009.02.008
Mueller, M., Grauschopf, U., Maier, T., Glockshuber, R., and Ban, N. (2009). The Structure of a Cytolytic α-Helical Toxin Pore Reveals its Assembly Mechanism. Nature 459, 726–730. doi:10.1038/nature08026
Nardin, C., Winterhalter, M., and Meier, W. (2000). Giant Free-Standing ABA Triblock Copolymer Membranes. Langmuir 16, 7708–7712. doi:10.1021/la000204t
O’Hern, S. C., Jang, D., Bose, S., Idrobo, J. C., Song, Y., Laoui, T., et al. (2015). Nanofiltration across Defect-Sealed Nanoporous Monolayer Graphene. Nano Lett. 15, 3254–3260. doi:10.1021/acs.nanolett.5b00456
Ohmann, A., Göpfrich, K., Joshi, H., Thompson, R. F., Sobota, D., Ranson, N. A., et al. (2019). Controlling Aggregation of Cholesterol-Modified DNA Nanostructures. Nucleic Acids Res. 47, 11441–11451. doi:10.1093/nar/gkz914
Park, H. B., Kamcev, J., Robeson, L. M., Elimelech, M., and Freeman, B. D. (2017). Maximizing the Right Stuff: The Trade-Off between Membrane Permeability and Selectivity. Science 356, eaab0530. doi:10.1126/science.aab0530
Perez Sirkin, Y. A., Tagliazucchi, M., and Szleifer, I. (2020). Transport in Nanopores and Nanochannels: Some Fundamental Challenges and Nature-Inspired Solutions. Mater. Today Adv. 5, 100047. doi:10.1016/j.mtadv.2019.100047
Perez-Rathke, A., Fahie, M. A., Chisholm, C., Liang, J., and Chen, M. (2018). Mechanism of OmpG pH-Dependent Gating from Loop Ensemble and Single Channel Studies. J. Am. Chem. Soc. 140, 1105–1115. doi:10.1021/jacs.7b11979
Peters, R. (2003). Optical Single Transporter Recording: Transport Kinetics in Microarrays of Membrane Patches. Annu. Rev. Biophys. Biomol. Struct. 32, 47–67. doi:10.1146/annurev.biophys.32.110601.142429
Peters, R., Sauer, H., Tschopp, J., and Fritzsch, G. (1990). Transients of Perforin Pore Formation Observed by Fluorescence Microscopic Single Channel Recording. EMBO J. 9, 2447–2451. doi:10.1002/j.1460-2075.1990.tb07421.x
Pinheiro, A. V., Han, D., Shih, W. M., and Yan, H. (2011). Challenges and Opportunities for Structural DNA Nanotechnology. Nat. Nanotech 6, 763–772. doi:10.1038/nnano.2011.187
Quick, J., Loman, N. J., Duraffour, S., Simpson, J. T., Severi, E., Cowley, L., et al. (2016). Real-Time, Portable Genome Sequencing for Ebola Surveillance. Nature 530, 228–232. doi:10.1038/nature16996
Reiner, J. E., Balijepalli, A., Robertson, J. W. F., Campbell, J., Suehle, J., and Kasianowicz, J. J. (2012). Disease Detection and Management via Single Nanopore-Based Sensors. Chem. Rev. 112, 6431–6451. doi:10.1021/cr300381m
Riedl, J., Ding, Y., Fleming, A. M., and Burrows, C. J. (2015). Identification of DNA Lesions Using a Third Base Pair for Amplification and Nanopore Sequencing. Nat. Commun. 6, 8807. doi:10.1038/ncomms9807
Rothemund, P. W. K. (2006). Folding DNA to Create Nanoscale Shapes and Patterns. Nature 440, 297–302. doi:10.1038/nature04586
Sakai, N., and Matile, S. (2013). Synthetic Ion Channels. Langmuir 29, 9031–9040. doi:10.1021/la400716c
Sakamoto, S., Komatsu, T., Watanabe, R., Zhang, Y., Inoue, T., Kawaguchi, M., et al. (2020). Multiplexed Single-Molecule Enzyme Activity Analysis for Counting Disease-Related Proteins in Biological Samples. Sci. Adv. 6, eaay0888. doi:10.1126/sciadv.aay0888
Santos, A., Deen, M. J., and Marsal, L. F. (2015). Low-Cost Fabrication Technologies for Nanostructures: State-Of-The-Art and Potential. Nanotechnology 26, 042001. doi:10.1088/0957-4484/26/4/042001
Schmidt, C., Mayer, M., and Vogel, H. (2000). A Chip-Based Biosensor for the Functional Analysis of Single Ion Channels. Angew. Chem. Int. Ed.D. 39 (17), 3137–3140. doi:10.1002/1521-3773(20000901)39:17<3137::AID-ANIE3137>3.0.co;2-d
Seeman, N. C. (2010). Nanomaterials Based on DNA. Annu. Rev. Biochem. 79, 65–87. doi:10.1146/annurev-biochem-060308-102244
Seifert, A., Göpfrich, K., Burns, J. R., Fertig, N., Keyser, U. F., and Howorka, S. (2015). Bilayer-spanning DNA Nanopores with Voltage-Switching between Open and Closed State. ACS Nano 9, 1117–1126. doi:10.1021/nn5039433
Shang, J., Li, Z., Liu, L., Xi, D., and Wang, H. (2018). Label-free Sensing of Human 8-Oxoguanine DNA Glycosylase Activity with a Nanopore. ACS Sens. 3, 512–518. doi:10.1021/acssensors.7b00954
Sharma, S., Buchholz, K., Luber, S. M., Rant, U., Tornow, M., and Abstreiter, G. (2006). Silicon-On-Insulator Microfluidic Device with Monolithic Sensor Integration for µTAS Applications. J. Microelectromech. Syst. 15, 308–313. doi:10.1109/JMEMS.2006.872222
Siebrasse, J. P., and Peters, R. (2002). Rapid Translocation of NTF2 through the Nuclear Pore of Isolated Nuclei and Nuclear Envelopes. EMBO Rep. 3, 887–892. doi:10.1093/embo-reports/kvf171
Soga, N., Watanabe, R., and Noji, H. (2015). Attolitre-Sized Lipid Bilayer Chamber Array for Rapid Detection of Single Transporters. Sci. Rep. 5, 11025. doi:10.1038/srep11025
Soskine, M., Biesemans, A., Moeyaert, B., Cheley, S., Bayley, H., and Maglia, G. (2012). An Engineered ClyA Nanopore Detects Folded Target Proteins by Selective External Association and Pore Entry. Nano Lett. 12, 4895, 4900. doi:10.1021/nl3024438
Spicer, C. D., and Davis, B. G. (2014). Selective Chemical Protein Modification. Nat. Commun. 5, 4740. doi:10.1038/ncomms5740
Spitzberg, J. D., Zrehen, A., van Kooten, X. F., and Meller, A. (2019). Plasmonic‐Nanopore Biosensors for Superior Single‐Molecule Detection. Adv. Mater. 31, 1900422. doi:10.1002/adma.201900422
Stoloff, D. H., and Wanunu, M. (2013). Recent Trends in Nanopores for Biotechnology. Curr. Opin. Biotechnol. 24, 699–704. doi:10.1016/j.copbio.2012.11.008
Sumitomo, K., McAllister, A., Tamba, Y., Kashimura, Y., Tanaka, A., Shinozaki, Y., et al. (2012). Ca2+ Ion Transport through Channels Formed by α-Hemolysin Analyzed Using a Microwell Array on a Si Substrate. Biosens. Bioelectron. 31, 445–450. doi:10.1016/j.bios.2011.11.010
Sun, Y., Zang, X., Sun, Y., Wang, L., and Gao, Z. (2020). Lipid Membranes Supported by Planar Porous Substrates. Chem. Phys. Lipids 228, 104893. doi:10.1016/j.chemphyslip.2020.104893
Tagliazucchi, M., and Szleifer, I. (2015). Transport Mechanisms in Nanopores and Nanochannels: Can We Mimic Nature? Mater. Today 18, 131–142. doi:10.1016/j.mattod.2014.10.020
Thomsen, R. P., Malle, M. G., Okholm, A. H., Krishnan, S., Bohr, S. S.-R., Sørensen, R. S., et al. (2019). A Large Size-Selective DNA Nanopore with Sensing Applications. Nat. Commun. 10, 5655. doi:10.1038/s41467-019-13284-1
Traversi, F., Raillon, C., Benameur, S. M., Liu, K., Khlybov, S., Tosun, M., et al. (2013). Detecting the Translocation of DNA through a Nanopore Using Graphene Nanoribbons. Nat. Nanotech 8, 939–945. doi:10.1038/nnano.2013.240
Tschödrich-Rotter, M., Kubitscheck, U., Ugochukwu, G., Buckley, J. T., and Peters, R. (1996). Optical Single-Channel Analysis of the Aerolysin Pore in Erythrocyte Membranes. Biophysical J. 70, 723–732. doi:10.1016/S0006-3495(96)79612-1
Tschödrich-Rotter, M., and Peters, R. (1998). An Optical Method for Recording the Activity of Single Transporters in Membrane Patches. J. Microsc. 192, 114–125. doi:10.1046/j.1365-2818.1998.00411.x
Uemura, S., Aitken, C. E., Korlach, J., Flusberg, B. A., Turner, S. W., and Puglisi, J. D. (2010). Real-Time tRNA Transit on Single Translating Ribosomes at Codon Resolution. Nature 464, 1012–1017. doi:10.1038/nature08925
Urban, M., Kleefen, A., Mukherjee, N., Seelheim, P., Windschiegl, B., Vor der Brüggen, M., et al. (2014). Highly Parallel Transport Recordings on a Membrane-On-Nanopore Chip at Single Molecule Resolution. Nano Lett. 14, 1674–1680. doi:10.1021/nl5002873
Vargas Jentzsch, A., Hennig, A., Mareda, J., and Matile, S. (2013). Synthetic Ion Transporters that Work with Anion−π Interactions, Halogen Bonds, and Anion-Macrodipole Interactions. Acc. Chem. Res. 46, 2791, 2800. doi:10.1021/ar400014r
Volgraf, M., Gorostiza, P., Numano, R., Kramer, R. H., Isacoff, E. Y., and Trauner, D. (2006). Allosteric Control of an Ionotropic Glutamate Receptor with an Optical Switch. Nat. Chem. Biol. 2, 47–52. doi:10.1038/nchembio756
Walker, M. I., Ubych, K., Saraswat, V., Chalklen, E. A., Braeuninger-Weimer, P., Caneva, S., et al. (2017). Extrinsic Cation Selectivity of 2D Membranes. ACS Nano 11, 1340–1346. doi:10.1021/acsnano.6b06034
Wang, G., Wang, L., Han, Y., Zhou, S., and Guan, X. (2013). Nanopore Stochastic Detection: Diversity, Sensitivity, and Beyond. Acc. Chem. Res. 46, 2867–2877. doi:10.1021/ar400031x
Wang, Y., Zheng, D., Tan, Q., Wang, M. X., and Gu, L.-Q. (2011). Nanopore-Based Detection of Circulating microRNAs in Lung Cancer Patients. Nat. Nanotech 6, 668–674. doi:10.1038/nnano.2011.147
Wanunu, M., Dadosh, T., Ray, V., Jin, J., McReynolds, L., and Drndić, M. (2010). Rapid Electronic Detection of Probe-Specific MicroRNAs Using Thin Nanopore Sensors. Nat. Nanotech 5, 807–814. doi:10.1038/nnano.2010.202
Watanabe, R., Komatsu, T., Sakamoto, S., Urano, Y., and Noji, H. (2018a). High-Throughput Single-Molecule Bioassay Using Micro-Reactor Arrays with a Concentration Gradient of Target Molecules. Lab. Chip 18, 2849–2853. doi:10.1039/c8lc00535d
Watanabe, R. (2020). Microsystem for the Single Molecule Analysis of Membrane Transport Proteins. Biochim. Biophys. Acta (BBA) - Gen. Subjects 1864, 129330. doi:10.1016/j.bbagen.2019.03.016
Watanabe, R., Sakuragi, T., Noji, H., and Nagata, S. (2018b). Single-Molecule Analysis of Phospholipid Scrambling by TMEM16F. Proc. Natl. Acad. Sci. USA 115, 3066–3071. doi:10.1073/pnas.1717956115
Watanabe, R., Soga, N., Fujita, D., Tabata, K. V., Yamauchi, L., Hyeon Kim, S., et al. (2014a). Arrayed Lipid Bilayer Chambers Allow Single-Molecule Analysis of Membrane Transporter Activity. Nat. Commun. 5, 4519. doi:10.1038/ncomms5519
Watanabe, R., Soga, N., Hara, M., and Noji, H. (2016). Arrayed Water-In-Oil Droplet Bilayers for Membrane Transport Analysis. Lab. Chip 16, 3043–3048. doi:10.1039/c6lc00155f
Watanabe, R., Soga, N., Yamanaka, T., and Noji, H. (2014b). High-Throughput Formation of Lipid Bilayer Membrane Arrays with an Asymmetric Lipid Composition. Sci. Rep. 4, 7076. doi:10.1038/srep07076
Wei, R., Gatterdam, V., Wieneke, R., Tampé, R., and Rant, U. (2012a). Stochastic Sensing of Proteins with Receptor-Modified Solid-State Nanopores. Nat. Nanotech 7, 257–263. doi:10.1038/nnano.2012.24
Wei, R., Martin, T. G., Rant, U., and Dietz, H. (2012b). DNA Origami Gatekeepers for Solid-State Nanopores. Angew. Chem. Int. Ed. 51, 4864–4867. doi:10.1002/anie.201200688
Wu, H.-L., Chen, P.-Y., Chi, C.-L., Tsao, H.-K., and Sheng, Y.-J. (2013). Vesicle Deposition on Hydrophilic Solid Surfaces. Soft Matter 9, 1908–1919. doi:10.1039/c2sm27450g
Xi, D., Shang, J., Fan, E., You, J., Zhang, S., and Wang, H. (2016). Nanopore-Based Selective Discrimination of microRNAs with Single-Nucleotide Difference Using Locked Nucleic Acid-Modified Probes. Anal. Chem. 88, 10540–10546. doi:10.1021/acs.analchem.6b02620
Xu, Y., Hu, X., Kundu, S., Nag, A., Afsarimanesh, N., Sapra, S., et al. (2019). Silicon-Based Sensors for Biomedical Applications: A Review. Sensors 19, 2908. doi:10.3390/s19132908
Yoo, J., and Aksimentiev, A. (2015). Molecular Dynamics of Membrane-Spanning DNA Channels: Conductance Mechanism, Electro-Osmotic Transport, and Mechanical Gating. J. Phys. Chem. Lett. 6, 4680–4687. doi:10.1021/acs.jpclett.5b01964
Yusko, E. C., Johnson, J. M., Majd, S., Prangkio, P., Rollings, R. C., Li, J., et al. (2011). Controlling Protein Translocation through Nanopores with Bio-Inspired Fluid Walls. Nat. Nanotech 6, 253–260. doi:10.1038/nnano.2011.12
Zeng, S., Wen, C., Solomon, P., Zhang, S.-L., and Zhang, Z. (2019). Rectification of Protein Translocation in Truncated Pyramidal Nanopores. Nat. Nanotechnol. 14, 1056–1062. doi:10.1038/s41565-019-0549-0
Keywords: DNA nanopores, solid-state nanopores, membrane proteins, membrane transport, silicon chips, biosenors, single-molecule analyses
Citation: Diederichs T and Tampé R (2021) Membrane-Suspended Nanopores in Microchip Arrays for Stochastic Transport Recording and Sensing. Front. Nanotechnol. 3:703673. doi: 10.3389/fnano.2021.703673
Received: 30 April 2021; Accepted: 14 June 2021;
Published: 25 June 2021.
Edited by:
Jiahai Wang, Guangzhou University, ChinaReviewed by:
Hai-Chen Wu, University of Chinese Academy of Sciences, ChinaDaqi Chen, Guangzhou University, China
Copyright © 2021 Diederichs and Tampé. This is an open-access article distributed under the terms of the Creative Commons Attribution License (CC BY). The use, distribution or reproduction in other forums is permitted, provided the original author(s) and the copyright owner(s) are credited and that the original publication in this journal is cited, in accordance with accepted academic practice. No use, distribution or reproduction is permitted which does not comply with these terms.
*Correspondence: Robert Tampé, dGFtcGVAZW0udW5pLWZyYW5rZnVydC5kZQ==