- Department of Physics, Shanghai University of Electric Power, Shanghai, China
Photocatalysis, which includes water splitting for hydrogen fuel generation, degradation of organic pollutants, and CO2 reduction using renewable solar energy, is one of the most promising solutions for environmental protection and energy conversion. Halide perovskite has recently emerged as a new promising material for photocatalytic applications. The exploration of new efficient halide perovskite-based photocatalysts and understanding of photocatalytic reaction mechanisms can be revealed using theoretical calculations. The progress and applications of first-principles atomistic modeling and simulation of halide perovskite photocatalysts, including metal halide perovskites, halide perovskite heterojunctions, and other promising perovskite derivatives, are presented in this review. Critical insights into the challenges and future research directions of photocatalysis using halide perovskites are also discussed.
Introduction
As sustainable, inexhaustible, clean, and renewable energy, solar energy has been considered an attractive alternative for applications of heating systems and electricity production in the past decades. Driven by solar energy, photocatalysis is consideredone of the most important routes for using solar energy since the pioneering work on TiO2 for water splitting in 1972 (Fujishima and Honda, 1972). Photocatalytic processes are applied in four fields: water splitting, CO2 reduction, degradation of organic compounds, and organic synthesis. Semiconductors have been the most used photocatalysts because of their intrinsic optical and electronic properties, from conventional binary semiconductor photocatalysts (i.e., g-C3N4, TiO2, ZnO, CdS) (Yu et al., 2013; Jin et al., 2015; Ye et al., 2015; Adhikari et al., 2016; Cheng et al., 2018; Jaramillo-Páez et al., 2018; Yu et al., 2018) to ternary photocatalysts (i.e., BiVO4, SrTiO3, BaTi4O9) (Inoue et al., 1992; Kato and Kudo, 2002; Zeng et al., 2018; Andrei et al., 2020). However, conventional photocatalysts have drawbacks, such as large bandgap, high carrier recombination rate, and low light-absorption ability. It is critical to develop new photocatalysts with high photo-conversion efficiency.
Metal halide perovskites with a chemical formula of ABX3 (A = CH3NH3, CH (NH2)2, Cs; B = Pb, Sn; X = I, Br, or Cl) have recently attracted extensive research attention because of their extraordinary photophysical properties and performance, such as high visible-light absorption coefficients, long charge carrier diffusion lengths, high photoluminescence quantum yield, tunable bandgap, etc (Park et al., 2016; Zhang et al., 2018a; Shi and Jayatissa, 2018). Furthermore, combined with a diversity of A and B sites and ferroelectric and piezoelectric effects (Frost et al., 2014; Kanhere and Chen, 2014; Sun et al., 2016; Ji et al., 2017; Zhu et al., 2019), ABX3 halide perovskites are indubitably one of the most promising alternatives in photocatalytic applications (Zhu and Podzorov, 2015; Lee et al., 2018; Hong et al., 2019; Zhu et al., 2019; Chen et al., 2020).
First-principle atomistic modeling and simulation, such as density functional theory (DFT) and Green’s function-based many-body perturbation theory (GW), are fundamental means to investigate material properties by inputting the initial lattice structure and known atomic information. The DFT method is a calculation method based on Hohenberg-Kohn theorem and Kohn-Sham equation (Hohenberg and Kohn, 1964; Kohn and Sham, 1965). When it comes to specific practical problems, it is necessary to consider several choices of exchange-correlation functional, such as Local Density Approximation (LDA), Generalized Gradient Approximation (GGA) and hybrid density functional method (Jones and Gunnarsson, 1989; Parr Robert, 1989).
LDA is mainly aimed at uniform electron gas, meaning that the charge density needs to change slowly in the order of local Fermi wavelength. In the calculation of Raman spectrum and phonon spectrum, LDA tends to a good performance. GGA optimizes the exchange-correlation and adds the gradient of charge density change to the exchange-correlation functional, giving more reasonable simulation results for heterogeneous electronic systems (Richard, 2004; Jiang et al., 2010). The commonly used GGA functionals are Perdew-Burke-Ernzerhof (PBE) (Kresse and Joubert, 1999) and Perdew -Wang (PW91) (Blöchl, 1994). However, GGA ignores the nonlocality of exchange, which usually underestimates the band gap. Therefore, hybrid density functional method is developed, which can improve the calculation accuracy based on the exact exchange energy obtained by Hartree-Fock approximation and the partial exact exchange energy obtained by mixed density functional theory. The commonly used methods are Heyd-Scuseria-Ernzerhof (HSE), PBE0, etc. (Perdew et al., 1996; Heyd et al., 2003) In addition, there are not only correction methods for electronic correlation (Grimme et al., 2010; Cao et al., 2012), such as dispersion interaction correction (DFT + D), self-interaction correction (DFT + U), but also correction methods for magnetic materials such as Spin orbit coupling (SOC) (Afsari et al., 2017; Sehgal, 2017).
GW method provides a more rigorous theoretical framework for the theoretical description of the electronic energy band structure of materials, which can give high-precision theoretical predictions for physically simple semiconductor materials, such as G0W0 method based on LDA/GGA self-consistent calculation (Aryasetiawan and Gunnarsson, 1998; Aulbur et al., 2000). However, there is still much room for improvement for physically more complex systems with stronger electronic correlation effects, because LDA/GGA often gives wrong description, which can lead to the wrong calculation of G0W0 (Aryasetiawan and Gunnarsson, 1995; Jiang, 2010; Jiang and Zhang, 2020). Many new methods have been developed to choose an appropriate starting point to calculate the reference Hamiltonian, such as G0W0 based on DFT + U or HSE (Miyake et al., 2006; Jiang et al., 2010), or quasi particle self-consistent GW, and fully self-consistent GW (van Schilfgaarde et al., 2006; Grumet et al., 2018). Among the above-mentioned methods, GGA-PBE in DFT calculation method is the most commonly used and inexpensive calculation method, because it does not cost so much computational power, while HSE and GW methods are more suitable for dealing with band gap calculation, and there are some problems such as complex program implementation and large amount of calculation.
First-principle methods can be used to explore the lattice constants, electronic structures, carrier mobility and diffusion lengths of photocatalyst, understand the reaction mechanism, and further predict new halide perovskite-based photocatalyst (Catlow et al., 2010; Luo and Daoud, 2015; Yu, 2019). The calculation of these properties is critical for optimizing the photocatalytic performance. Thus far, there has been limited research regarding the booming use of first-principles calculations to study halide perovskites as photocatalysts. Herein, we summarize the recent progress in the theoretical calculation study on halide perovskite-based photocatalysts (Figure 1), and focus on the link between calculated material properties and performance of photocatalysts, including 1) metal halide perovskites, 2) halide perovskite heterojunctions, and 3) other promising perovskite derivatives. Then, we discuss the challenges and future research directions of halide perovskite photocatalysis.
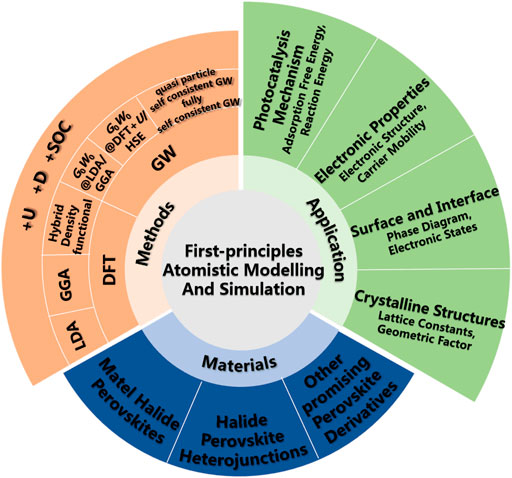
FIGURE 1. Applications of first-principles atomistic modeling and simulation in halide perovskite-based photocatalysts.
Theoretical Calculation of Properties of Halide Perovskite-Based Photocatalysts
Metal Halide Perovskites
As is well known, for the ABX3 perovskites, the BX6 octahedra can be tilted and distorted at finite temperature or pressure (Glazer, 1972), and anharmonic effects are associated with the perovskite-phase transition (Buckeridge et al., 2013). Moreover, the bandgap of ABX3 perovskites can be tuned by chemical substitution (Sutton et al., 2016; Wang et al., 2019a). Several first-principles investigations into ABX3 structures have been conducted to explore their crystal structures, material stability properties, and defect physics. Most notably, the electronic and optical properties of ABX3 perovskite as light absorbers in photocatalysis are critical, and several theoretical calculation studies have focused on the electronic energy-band structure, corresponding density of states (DOS), carrier mobility, and other electronic properties of halide perovskite-based photocatalyst.
One of the critical properties of a photocatalyst is its bandgap. Considering the utilization of visible light in the sunlight, the bandgap of ∼2 eV is desirable for photocatalytic reaction (Xiaobo et al., 2010). In addition, for CO2 reduction, the position of conduction band minimum (CBM) should be lower than −0.52 eV (vs. NHE PH = 0) (the energy barriers of reducing CO2 to CO) (Kanhere and Chen, 2014; Schneider et al., 2012), that is more advantageous to the reduction reaction in thermodynamics. Angelis et al. first computationally investigated the MAPbX3 hybrid halide perovskites in 2013 (Mosconi et al., 2013). They performed periodic DFT calculations within the GGA and found that the calculated bandgap values (MAPbI3: Eg = 1.57 eV, MAPbBr3: Eg = 1.80 eV, MAPbCl3: Eg = 2.34 eV) agreed well with the experimental values. Figures 2A–C shows the optimized geometrical structures, calculated band structure, and DOS of MAPbI3. Afterward, Umari developed an effective GW method incorporating SOC to accurately model the electronic properties of MAPbI3 and MASnI3 (Umari et al., 2014). Furthermore, the HSE06 and PBE functionals, with and without SOC calculations, were used to compute the bandgap of ABX3 halide perovskites (Giorgi et al., 2013; Brivio et al., 2014; Melissen et al., 2015). By comparing the calculated values, it is proved that the strong electron-hole interaction should consider, and the SOC plays a critical role in dominating the conduction band, although the computational requirements increase.
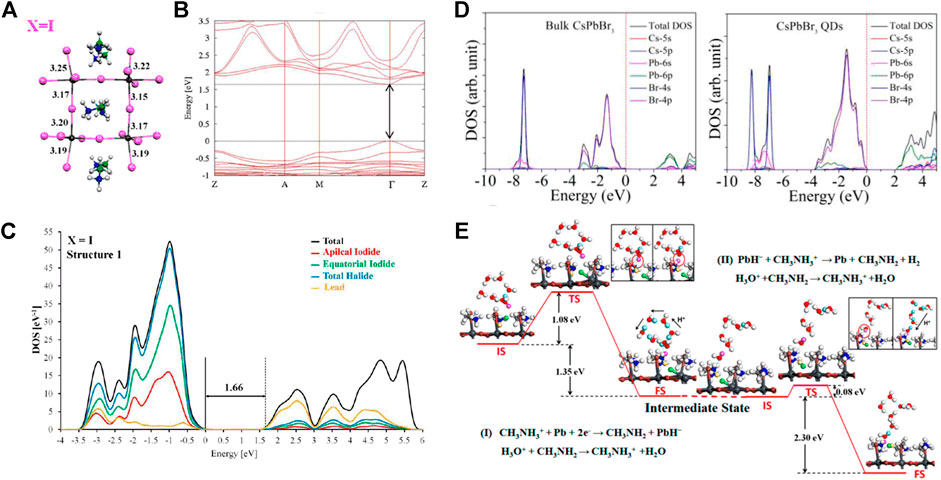
FIGURE 2. (A) Optimized geometrical structures, (B) calculated band structure, and (C) DOS of MAPbI3 (Grimme et al., 2010). Copyright (2013) American Chemical Society. (D) Calculated densities of states diagrams of bulk CsPbBr3 and CsPbBr3 QDs (Aryasetiawan and Gunnarsson, 1995). Copyright (2017) John Wiley and Sons. (E) PbAAA reaction pathway for H2 generation on MAPbI3 surface in acidic solvent (Jiang and Zhang, 2020). Copyright (2018) American Chemical Society.
Moreover, the DOS can also be used to predict the performance of photocatalysts through the analysis of total and atomic-resolved DOS. The DOS can be obtained from a non-self-consistent-field (SCF) energy-band calculation, and from the analysis of the partial DOS, the contribution of each site to the valence band and conduction band can be revealed. For ABX3 halide perovskites, the CBM is usually dominated by B p-states, whereas the valence band maximum (VBM) consists of antibonding between B s-states and X p-states (Borriello et al., 2008; Chung et al., 2012; Mosconi et al., 2013; Umari et al., 2014). Furthermore, the photogenerated carrier transfer ability can be revealed by the calculated DOS. Sun et al. synthesized colloidal CsPbBr3 quantum dots (QDs) as new photocatalytic material (Hou et al., 2017). To understand the advantages of the quantum size effect, they calculated the DOS of bulk CsPbBr3 and atomic CsPbBr3 QD slabs. Different from the bulk CsPbBr3, the conduction band of CsPbBr3 QDs was primarily contributed by the coupling of Cs p-states, Br p-states, and Pb p-states, whereas the valence band was constructed by the coupling of Cs p-states, Pb s-states, and Br p-states (as shown in Figure 2D). Due to the increased DOS at CBM, more photogenerated carriers can reach CBM, resulting in more efficient photochemical conversion.
The last, but the most important issue is the photocatalytic mechanism. Metal halide perovskites have been successfully confirmed as efficient photocatalysts. However, it is difficult to develop improved perovskite materials without a thorough analysis of reaction mechanisms. Wang et al. derived the photocatalytic hydrogen evolution reaction (HER) mechanism using PBE functional of DFT, including the D3 van der Waals correction to account for London dispersion interactions (Zhang et al., 2018b). They constructed a supercell of the (010) surface of MAPbI3 in HI aqueous solution, and by comparing the activation barriers and reaction energies of different reaction pathways, they found that the Pb atoms and surface organic molecules play critical roles in the HER reaction. Furthermore, a two-step Pb-activated amine-assisted (PbAAA) reaction mechanism was proposed (Figure 2E), which is widely accepted and approved. Tao et al. performed the DFT calculations with the PbAAA mechanism to explain the Br effect on the enhanced performance of the MAPb(I1-xBrx)3 photocatalytic HER process (Zhao et al., 2019a). Due to the smaller Br ionic radius, the Br-Pb bonds can be easily broken, and more Pb defect sites were formed for lowering the H-Pb absorption energy, and thus MAPb(I1-xBrx)3 shows a superb HER rate under visible light illumination.
Halide Perovskite Heterojunctions
To enhance the photocatalytic performance, the construction of halide perovskite heterojunctions with two-dimensional (2D) materials such as graphene, g-C3N4, and black phosphorus has caused extensive concern due to improved stability, more efficient carrier mobility, and regulated bandwidth (Xu et al., 2017; Ou et al., 2018; Wu et al., 2018; Zhao et al., 2019b; Guo et al., 2019; Paul et al., 2019; Wang, 2020). For the heterojunction calculations, the main problem is the understanding of the charge density difference and charge transfer process at the contacting interface.
Guo et al. used density-functional first-principles calculations with empirical dispersion corrections to investigate the interfacial interactions and electronic properties of graphdiyne/MAPbI3 heterostructures (Guo et al., 2019). By employing the projector-augmented wave (PAW) to describe the electron-core interaction, and GGA of PBE as the exchange-correlation functional, they found that the graphdiyne/MAPbI3 heterostructure was formed by van der Waals interaction, and the PbI2-terminated surface showed high stability when contacting graphdiyne. Combining HSE06 with SOC, the electronic properties including charge density difference, electron localization field, Bader charge, and electrostatic potential were analyzed, as shown in Figure 3A. The electron-hole separation is obviously enhanced due to the built-in electric field at the interface.
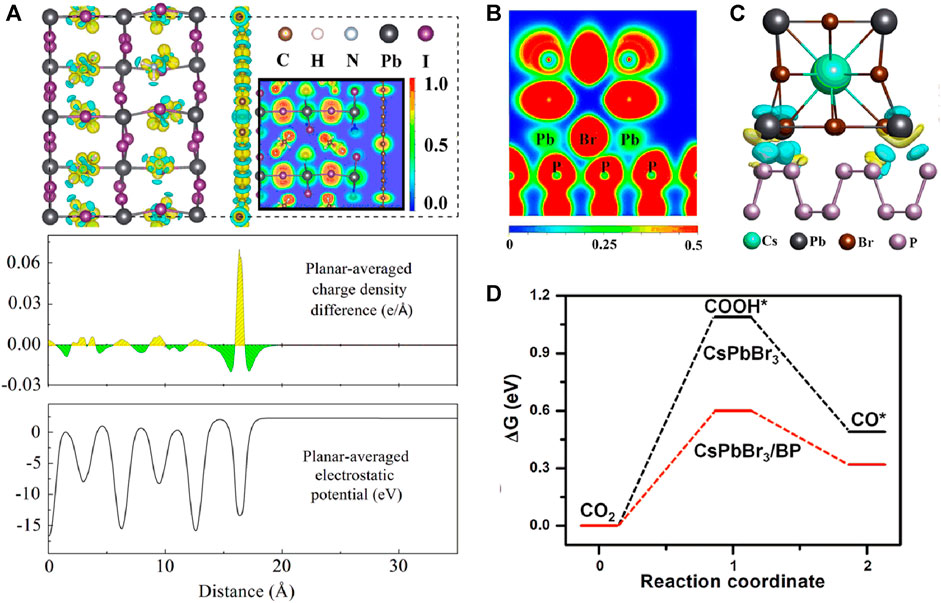
FIGURE 3. (A) Calculated electrostatic potential, charge density difference, and electron localization function plots for graphdiyne/MAPbI3 heterostructures with five-layer interfaces (Glazer, 1972). Copyright (2019) John Wiley and Sons. (B) Electron localization function, and (C) charge density difference of the interface between the CsPbBr3 and black phosphorus (Yu, 2019). (D) Gibbs free-energy diagram of CsPbBr3/black phosphorus and CsPbBr3 heterostructures in the photocatalytic CO2 reduction process (Yu, 2019). Copyright (2020) Elsevier.
In addition to the van der Waals interaction of the heterostructure interface, Zhu et al. found that a significant covalent bond between CsPbBr3 and black phosphorus nanosheets was formed in the heterostructure by DFT calculation (Wang, 2020). Moreover, charge density difference analysis revealed that a directional electron transfer channel was generated reasonably between Pb and p elements, as shown in Figure 3B,C which was consistent with the experimental results. Furthermore, using ab initio atomistic thermodynamics calculations, the possible mechanism of CO2 reduction by CsPbBr3 and black phosphorus nanosheet heterostructure was clarified. The black phosphorus nanosheets acted as the electron acceptor, which promoted the breaking of the C=O bonds and reduced the reaction barrier energy (Figure 3D).
Other Promising Perovskite Derivatives
The exploration of new photocatalysts is an important way to achieve high photocatalytic performance. And the new promising halide perovskites offer distinct advantages over the traditional perovskite materials (Volonakis et al., 2016; Liu et al., 2018; Maughan et al., 2018; Yan et al., 2018; Tang et al., 2019; Yue et al., 2020), such as substitution or mixing, double perovskites, and other perovskite derivatives. Theoretical calculation is a powerful tool to predict new functional perovskite materials suitable for photocatalysis and optimize the stoichiometric ratio of mixed halide perovskites. Especially when introducing the Goldschmidt tolerance factor
Xu et al. compared the catalytic efficiency of bulk CsPbBr3 doped with different metals (Co., Fe, Ni, Cu, Ag, Mg, Mn, and Bi) and concluded that Co. and Fe doping could be ideal for CO2 reduction (Tang et al., 2019). They specifically analyzed the adsorption-free energy using GGA of PBE functional with the PAW method. As shown in Figure 4A the ab initio molecular dynamics calculations indicated that Co− and Fe-doped systems showed stronger CO2* adsorption energy (* refers to the adsorption site), which is the most important factor to determine the catalytic efficiency. Furthermore, using HSE06 with DFT + U correction to calculate the Hirshfeld charges and DOS of the strongly correlated effect of Co. atoms, they further illustrated the proposed reaction mechanism and confirmed that Co-doped CsPbBr3 could be a great candidate for CO2 reduction.
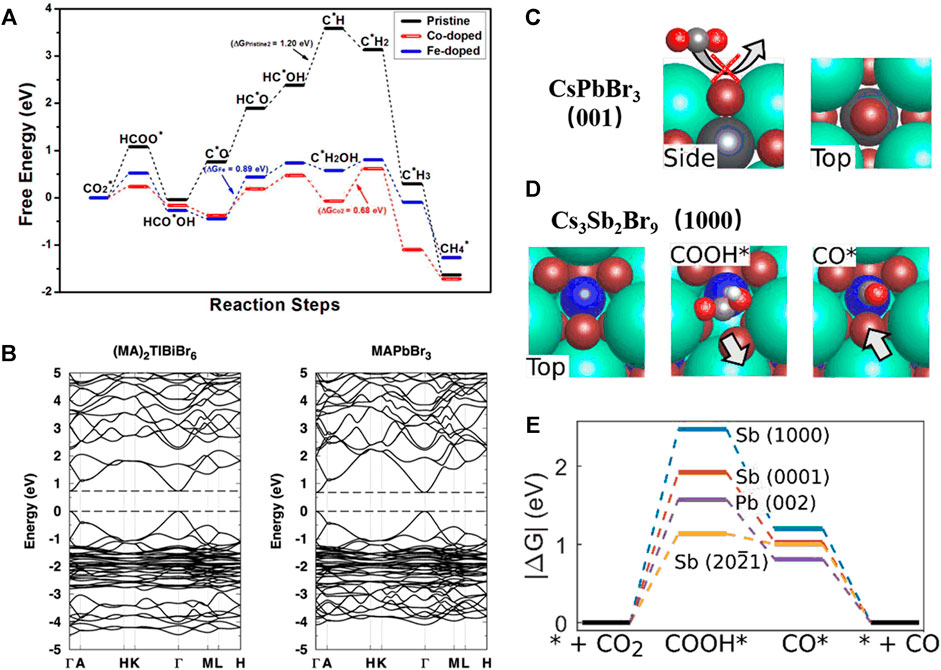
FIGURE 4. (A) The free-energy diagrams of the most favored paths of CO2 reduction on pristine, Co-doped and Fe-doped CsPbBr3 (Umari et al., 2014). Copyright (2019) Royal Society of Chemistry. (B) Calculated electronic band structures of (MA)2TlBiBr6 and MAPbBr3 (Melissen et al., 2015). Copyright (2016) Royal Society of Chemistry. Schematic of the (C) CsPbBr3 (001) surface and (D) reactivity of highly exposed Cs3Sb2Br9 (1000) surface. (E) Free-energy pathways for highly exposed Cs3Sb2Br9 (1000), (0001) and (2021) surfaces and CsPbBr3 (002) surface (Giorgi et al., 2013). Copyright (2020) Royal Society of Chemistry.
The toxicity of lead limits the wide commercialization of metal halide perovskite, and thus lead-free alternatives have recently attracted great attention, such as double perovskites (A2BX6 or A2B+1B+3X6) and other perovskite derivatives (A3B2X9, A3BX5, A4BX6, etc.), where Pb2+ cations are replaced using other metal cations, e.g., Na+, Ag+, Bi3+, and Sb3+. It is relatively difficult to experimentally synthesize thin films of these materials for photovoltaic and photocatalysis applications. However, the theoretical calculation is extremely useful because of its predicting power. For example, Cheetham et al. have used DFT calculations to compare the bandgaps and electronic structures of (MA)2B+1BiX6 (B+1 = e.g., K+1, Ag+1, Cu+1, Tl+1) and MAPbX3, and found that the new double perovskite (MA)2TL+1BiBr6, had strikingly isoelectronic properties with MAPbBr3, as shown in Figure 4 2) (Deng et al., 2016). This study provided an intriguing alternative to the lead-containing MAPbX3 for photoelectronic applications.
For the all-inorganic halide double perovskites, Giustino et al. (Volonakis et al., 2017) proposed a new promising halide double perovskites, Cs2InAgX6, with the direct band gaps between the visible and the ultraviolet as computed by the HSE06 and PBE0. Recently, Bartel et al. (Bartel et al., 2020) predicted 47 likely synthesizable compounds containing non toxic elements and with direct or nearly direct band gaps among the 311 cesium chloride double perovskites (Cs2BB’Cl6) compounds by computing with HSE06, and identified that the triple-alkali perovskites (where B refers to a group 1 alkali, and B′ refers to a transition-metal cation) showed remarkable optical properties as computed by the GW-Bethe-Salpeter equation method. These studies are helpful to realize the future studies aim and synthesize more efficient photocatalyst with unique optoelectronic properties.
Besides the analyses of the structural, electronic and optical properties, first-principles calculations can also be used to predict phase transition temperatures, which have instructive significance on the exploration of new materials. Kye et al. calculated the bandgaps and optical properties of K2SnX6 (X = I, Br, Cl) in cubic, tetragonal, and monoclinic phases, and concluded that the cubic K2SnBr6 and monoclinic K2SnI6 can be promising candidates for light absorber materials (Jong et al., 2020). Moreover, they calculated the Helmholtz-free-energy differences among the above three phases, and gave the phase transition temperatures of K2SnX6, respectively.
As mentioned above, it is difficult to experimentally synthesize uniform thin films of double perovskites or other perovskite derivatives; however, nanocrystals or other nanostructures of these materials can be synthesized more easily. Furthermore, they are proved to be promising candidates because of their highly exposed surface. Geyer et al. developed Cs3Sb2Br9 nanocrystals with significantly improved photocatalytic CO production efficiency (Lu et al., 2020). Moreover, the analogous mechanism was observed using DFT calculations, as shown in Figures 4C–E. They calculated the surface energies and adsorption free energies of the (0,001) (1,000), and (
As mentioned above, perovskite heterojunctions are usually endowed with optimized charge carrier dynamics for improved photocatalytic performance, and perovskite derivative heterojunctions are no exception (Feng et al., 2017; Guo et al., 2017; Wang et al., 2019b). By employing the DFT calculation, Kuang et al. (Wang et al., 2019b) investigated the electronic structures and interfacial properties in the Cs2SnI6/SnS2 hybrid heterojunctions. Then, they revealed that a typical type II band alignment formed in the heterojunction, which was in accordance with the experimental values. Due to the facilitated charge separation between Cs2SnI6 and SnS2 (i.e, holes from SnS2 to Cs2SnI6 and electrons from Cs2SnI6 to SnS2), the CO2 photocatalytic reduction activity was unquestionably boosted.
We believed that the theoretical calculation of perovskite derivatives and heterojunction can help predict more direct or nearly direct band-gap materials and choose some more matching materials forming heterojunction with promoted the charge carrier mobility and reduced charge carrier recombination.
Conclusion and Outlook
Herein, we summarized current computational work on the halide perovskite-based photocatalyst. To reveal the underlying photocatalytic mechanisms, first-principles calculations were used to investigate the crystal structures, electronic properties, defect physics, and surface and interface interaction of perovskites. Furthermore, the promising potential of the first-principles material design for predicting new halide perovskites such as substitution or mixing, double perovskites, or other perovskite derivatives has also been demonstrated.
From our perspective, several critical issues and challenges still need to be overcome. First, several unique properties of halide perovskites, such as piezoelectric, and ferroelectric properties due to the anharmonicity in lattice dynamics should be illustrated and explored to promote the photocatalytic reaction efficiency. Second, the influence of structure on the photocatalytic performance of halide perovskite-based heterojunctions, such as the types of ligands, defects, dopants, and crystalline phases, needs to be further researched to improve the activity of the heterostructures. Third, theoretical analytical models of perovskite derivatives can be helpful to promote the charge carrier mobility and reduce charge carrier recombination.
Author Contributions
XL proposed the research idea and manuscript; JF and CH performed check collection, collation and analysis; All authors reviewed the manuscript, have given approval to the final version of the manuscript, and confirmed the authorship to this work.
Funding
This work was supported by the National Natural Science Foundation of China (Grant No. 61875119), Shanghai Rising-Star Program (Grant No. 19QA1404000), and the “Chen Guang” project supported by Shanghai Municipal Education Commission and Shanghai Education Development Foundation (Grant No. 18CG63).
Conflict of Interest
The authors declare that the research was conducted in the absence of any commercial or financial relationships that could be construed as a potential conflict of interest.
References
Adhikari, S. P., Hood, Z. D., More, K. L., Chen, V. W., and Lachgar, A. (2016). A Visible-Light-Active Heterojunction with Enhanced Photocatalytic Hydrogen Generation. ChemSusChem 9 (14), 1869–1879. doi:10.1002/cssc.201600424
Afsari, M., Boochani, A., Hantezadeh, M., and Elahi, S. M. (2017). Topological Nature in Cubic Phase of Perovskite CsPbI 3 : By DFT. Solid State. Commun. 259, 10–15. doi:10.1016/j.ssc.2017.04.014
Andrei, V., Reuillard, B., and Reisner, E. (2020). Bias-free Solar Syngas Production by Integrating a Molecular Cobalt Catalyst with Perovskite-BiVO4 Tandems. Nat. Mater. 19 (2), 189–194. doi:10.1038/s41563-019-0501-6
Aryasetiawan, F., and Gunnarsson, O. (1995). Electronic Structure of NiO in theGWApproximation. Phys. Rev. Lett. 74 (16), 3221–3224. doi:10.1103/physrevlett.74.3221
Aryasetiawan, F., and Gunnarsson, O. (1998). TheGWmethod. Rep. Prog. Phys. 61 (3), 237–312. doi:10.1088/0034-4885/61/3/002
Aulbur, W. G., Jönsson, L., and Wilkins, J. W. (2000). “Quasiparticle Calculations in Solids,” in Solid State Physics. Editors H. Ehrenreich, and F. Spaepen (Academic Press), 1–218. doi:10.1016/s0081-1947(08)60248-9
Bartel, C. J., Clary, J. M., Sutton, C., Vigil-Fowler, D., Goldsmith, B. R., Holder, A. M., et al. (2020). Inorganic Halide Double Perovskites with Optoelectronic Properties Modulated by Sublattice Mixing. J. Am. Chem. Soc. 142 (11), 5135–5145. doi:10.1021/jacs.9b12440
Blöchl, P. E. (1994). Projector Augmented-Wave Method. Phys. Rev. B 50 (24), 17953–17979. doi:10.1103/physrevb.50.17953
Borriello, I., Cantele, G., and Ninno, D. (2008). Ab Initio investigation of Hybrid Organic-Inorganic Perovskites Based on Tin Halides. Phys. Rev. B 77 (23), 235214. doi:10.1103/physrevb.77.235214
Brivio, F., Butler, K. T., Walsh, A., and Schilfgaarde, M. V. (2014). Relativistic Quasiparticle Self-Consistent Electronic Structure of Hybrid Halide Perovskite Photovoltaic Absorbers. Phys. Rev. B 89 (15), 155204. doi:10.1103/physrevb.89.155204
Buckeridge, J., Scanlon, D. O., Walsh, A., Catlow, C. R. A., and Sokol, A. A. (2013). Dynamical Response and Instability in Ceria under Lattice Expansion. Phys. Rev. B 87 (21), 214304. doi:10.1103/physrevb.87.214304
Cao, J., Li, X., Lin, H., Chen, S., and Fu, X. (2012). In Situ preparation of Novel P-N junction Photocatalyst BiOI/(BiO)2CO3 with Enhanced Visible Light Photocatalytic Activity. J. Hazard. Mater. 239-240, 316–324. doi:10.1016/j.jhazmat.2012.08.078
Catlow, C. R. A., Guo, Z. X., Miskufova, M., Shevlin, S. A., Smith, A. G. H., Sokol, A. A., et al. (2010). Advances in Computational Studies of Energy Materials. Phil. Trans. R. Soc. A. 368, 3379–3456. doi:10.1098/rsta.2010.0111
Chen, P., Ong, W-J., Shi, Z., Zhao, X., and Li, N. (2020). Pb‐Based Halide Perovskites: Recent Advances in Photo(electro)catalytic Applications and Looking beyond. Adv. Funct. Mater. 30 (30). doi:10.1002/adfm.201909667
Cheng, L., Xiang, Q., Liao, Y., and Zhang, H. (2018). CdS-Based Photocatalysts. Energy Environ. Sci. 11 (6), 1362–1391. doi:10.1039/c7ee03640j
Chung, I., Song, J.-H., Im, J., Androulakis, J., Malliakas, C. D., Li, H., et al. (2012). CsSnI3: Semiconductor or Metal? High Electrical Conductivity and Strong Near-Infrared Photoluminescence from a Single Material. High Hole Mobility and Phase-Transitions. J. Am. Chem. Soc. 134 (20), 8579–8587. doi:10.1021/ja301539s
Deng, Z., Wei, F., Sun, S., Kieslich, G., Cheetham, A. K., and Bristowe, P. D. (2016). Exploring the Properties of lead-free Hybrid Double Perovskites Using a Combined Computational-Experimental Approach. J. Mater. Chem. A. 4 (31), 12025–12029. doi:10.1039/c6ta05817e
Fedorovskiy, A. E., Drigo, N. A., and Nazeeruddin, M. K. (2020). The Role of Goldschmidt's Tolerance Factor in the Formation of A 2 BX 6 Double Halide Perovskites and its Optimal Range. Small Methods 4 (5), 1900426. doi:10.1002/smtd.201900426
Feng, H.-J., Deng, W., Yang, K., Huang, J., and Zeng, X. C. (2017). Double Perovskite Cs2BBiX6 (B = Ag, Cu; X = Br, Cl)/TiO2 Heterojunction: An Efficient Pb-free Perovskite Interface for Charge Extraction. J. Phys. Chem. C 121 (8), 4471–4480. doi:10.1021/acs.jpcc.7b00138
Frost, J. M., Butler, K. T., Brivio, F., Hendon, C. H., van Schilfgaarde, M., and Walsh, A. (2014). Atomistic Origins of High-Performance in Hybrid Halide Perovskite Solar Cells. Nano Lett. 14 (5), 2584–2590. doi:10.1021/nl500390f
Fujishima, A., and Honda, K. (1972). Electrochemical Photolysis of Water at a Semiconductor Electrode. Nature 238 (5358), 37–38. doi:10.1038/238037a0
Giorgi, G., Fujisawa, J.-I., Segawa, H., and Yamashita, K. (2013). Small Photocarrier Effective Masses Featuring Ambipolar Transport in Methylammonium Lead Iodide Perovskite: A Density Functional Analysis. J. Phys. Chem. Lett. 4 (24), 4213–4216. doi:10.1021/jz4023865
Glazer, A. M. (1972). The Classification of Tilted Octahedra in Perovskites. Acta Crystallogr. Sect B 28 (11), 3384–3392. doi:10.1107/s0567740872007976
Goldschmidt, V. M. (1926). Die Gesetze der Krystallochemie. Naturwissenschaften 14 (21), 477–485. doi:10.1007/bf01507527
Grimme, S., Antony, J., Ehrlich, S., and Krieg, H. (2010). A Consistent and Accurate Ab Initio Parametrization of Density Functional Dispersion Correction (DFT-D) for the 94 Elements H-Pu. J. Chem. Phys. 132 (15), 154104. doi:10.1063/1.3382344
Grumet, M., Liu, P., Kaltak, M., Klimeš, J., and Kresse, G. (2018). Beyond the Quasiparticle Approximation: Fully Self-Consistent GW Calculations. Phys. Rev. B 98 (15), 155143. doi:10.1103/physrevb.98.155143
Guo, Y., Saidi, W. A., and Wang, Q. (2017). 2D halide perovskite-based van der Waals heterostructures: contact evaluation and performance modulation. 2D Mater. 4 (3), 035009. doi:10.1088/2053-1583/aa7ac3
Guo, Y., Xue, Y., Li, C., and Li, X. (2019). Electronic Properties of the Graphdiyne/CH3NH3PbI3 Interface: A First‐Principles Study. physica status solidi (Rrl) – Rapid Res. Lett. 14 (1). doi:10.1002/pssr.201900544
Heyd, J., Scuseria, G. E., and Ernzerhof, M. (2003). Hybrid Functionals Based on a Screened Coulomb Potential. J. Chem. Phys. 118 (18), 8207–8215. doi:10.1063/1.1564060
Hohenberg, P., and Kohn, W. (1964). Inhomogeneous Electron Gas. Phys. Rev. 136 (3B), B864–B871. doi:10.1103/physrev.136.b864
Hong, Z., Chong, W. K., Ng, A. Y. R., Li, M., Ganguly, R., Sum, T. C., et al. (2019). Hydrophobic Metal Halide Perovskites for Visible‐Light Photoredox C−C Bond Cleavage and Dehydrogenation Catalysis. Angew. Chem. Int. Ed. 58 (11), 3456–3460. doi:10.1002/anie.201812225
Hou, J., Cao, S., Wu, Y., Gao, Z., Liang, F., Sun, Y., et al. (2017). Inorganic Colloidal Perovskite Quantum Dots for Robust Solar CO 2 Reduction. Chem. Eur. J. 23 (40), 9481–9485. doi:10.1002/chem.201702237
Inoue, Y., Niiyama, T., Asai, Y., and Sato, K. (1992). Stable Photocatalytic Activity of BaTi4O9 Combined with Ruthenium Oxide for Decomposition of Water. J. Chem. Soc. Chem. Commun. (7), 579–580. doi:10.1039/c39920000579
Jaramillo-Páez, C. A., Navío, J. A., Hidalgo, M. C., and Macías, M. (2018). ZnO and Pt-ZnO Photocatalysts: Characterization and Photocatalytic Activity Assessing by Means of Three Substrates. Catal. Today 313, 12–19. doi:10.1016/j.cattod.2017.12.009
Ji, C., Sun, Z., Zeb, A., Liu, S., Zhang, J., Hong, M., et al. (2017). Bandgap Narrowing of Lead-Free Perovskite-type Hybrids for Visible-Light-Absorbing Ferroelectric Semiconductors. J. Phys. Chem. Lett. 8 (9), 2012–2018. doi:10.1021/acs.jpclett.7b00673
Jiang, H., Gomez-Abal, R., Rinke, P., and Scheffler, M. (2010). First-principles Modeling of Localized D States with the GW@LDA+U Approach. Phys. Rev. B 82 (4), 045108. doi:10.1103/physrevb.82.045108
Jiang, H. (2010). The GW Method: Basic Principles, Latest Developments and its Applications for D -and F -Electron Systems. Acta Phys. -Chim. Sin. 26 (04), 1017–1033.
Jiang, H., and Zhang, M. (2020). Density-functional Theory Methods for Electronic Band Structure Properties of Materials. SCIENTIA SINICA Chim. 50 (1674-7224), 1344.
Jin, J., Yu, J., Guo, D., Cui, C., and Ho, W. (2015). A Hierarchical Z-Scheme CdS-WO3Photocatalyst with Enhanced CO2Reduction Activity. Small 11 (39), 5262–5271. doi:10.1002/smll.201500926
Jones, R. O., and Gunnarsson, O. (1989). The Density Functional Formalism, its Applications and Prospects. Rev. Mod. Phys. 61 (3), 689–746. doi:10.1103/revmodphys.61.689
Jong, U.-G., Yu, C.-J., and Kye, Y.-H. (2020). Computational Prediction of Structural, Electronic, and Optical Properties and Phase Stability of Double Perovskites K2SnX6 (X = I, Br, Cl). RSC Adv. 10 (1), 201–209. doi:10.1039/c9ra09232c
Kanhere, P., and Chen, Z. (2014). A Review on Visible Light Active Perovskite-Based Photocatalysts. Molecules 19 (12), 19995–20022. doi:10.3390/molecules191219995
Kato, H., and Kudo, A. (2002). Visible-Light-Response and Photocatalytic Activities of TiO2 and SrTiO3 Photocatalysts Codoped with Antimony and Chromium. J. Phys. Chem. B 106 (19), 5029–5034. doi:10.1021/jp0255482
Kohn, W., and Sham, L. J. (1965). Quantum Density Oscillations in an Inhomogeneous Electron Gas. Phys. Rev. 137 (6A), A1697–A1705. doi:10.1103/physrev.137.a1697
Kresse, G., and Joubert, D. (1999). From Ultrasoft Pseudopotentials to the Projector Augmented-Wave Method. Phys. Rev. B 59 (3), 1758–1775. doi:10.1103/physrevb.59.1758
Lee, L. C., Huq, T. N., MacManus-Driscoll, J. L., and Hoye, R. L. Z. (2018). Research Update: Bismuth-Based Perovskite-Inspired Photovoltaic Materials. APL Mater. 6 (8), 084502. doi:10.1063/1.5029484
Liu, Y.-L., Yang, C.-L., Wang, M.-S., Ma, X.-G., and Yi, Y.-G. (2018). Theoretical Insight into the Optoelectronic Properties of lead-free Perovskite Derivatives of Cs3Sb2X9 (X = Cl, Br, I). J. Mater. Sci. 54 (6), 4732–4741. doi:10.1007/s10853-018-3162-y
Lu, C., Itanze, D. S., Aragon, A. G., Ma, X., Li, H., Ucer, K. B., et al. (2020). Synthesis of lead-free Cs3Sb2Br9 Perovskite Alternative Nanocrystals with Enhanced Photocatalytic CO2 Reduction Activity. Nanoscale 12 (5), 2987–2991. doi:10.1039/c9nr07722g
Luo, S., and Daoud, W. A. (2015). Recent Progress in Organic-Inorganic Halide Perovskite Solar Cells: Mechanisms and Material Design. J. Mater. Chem. A. 3 (17), 8992–9010. doi:10.1039/c4ta04953e
Richard, M. M. (2004). Electronic Structure: Basic Theory and Practical Methods. Cambridge: Cambridge University Press.
Maughan, A. E., Ganose, A. M., Almaker, M. A., Scanlon, D. O., and Neilson, J. R. (2018). Tolerance Factor and Cooperative Tilting Effects in Vacancy-Ordered Double Perovskite Halides. Chem. Mater. 30 (11), 3909–3919. doi:10.1021/acs.chemmater.8b01549
Melissen, S. T. A. G., Labat, F., Sautet, P., and Le Bahers, T. (2015). Electronic Properties of PbX3CH3NH3(X = Cl, Br, I) Compounds for Photovoltaic and Photocatalytic Applications. Phys. Chem. Chem. Phys. 17 (3), 2199–2209. doi:10.1039/c4cp04666h
Miyake, T., Zhang, P., Cohen, M. L., and Louie, S. G. (2006). Quasiparticle Energy of Semicore D Electrons in ZnS: Combined LDA+U and GW Approach. Phys. Rev. B 74 (24), 245213. doi:10.1103/physrevb.74.245213
Mosconi, E., Amat, A., Nazeeruddin, M. K., Grätzel, M., and De Angelis, F. (2013). First-Principles Modeling of Mixed Halide Organometal Perovskites for Photovoltaic Applications. J. Phys. Chem. C 117 (27), 13902–13913. doi:10.1021/jp4048659
Ou, M., Tu, W., Yin, S., Xing, W., Wu, S., Wang, H., et al. (2018). Amino-Assisted Anchoring of CsPbBr3 Perovskite Quantum Dots on Porous G-C3 N4 for Enhanced Photocatalytic CO2 Reduction. Angew. Chem. Int. Ed. 57 (41), 13570–13574. doi:10.1002/anie.201808930
Park, S., Chang, W. J., Lee, C. W., Park, S., Ahn, H-Y., and Nam, K. T. (2016). Photocatalytic Hydrogen Generation from Hydriodic Acid Using Methylammonium lead Iodide in Dynamic Equilibrium with Aqueous Solution. Nat. Energ. 2 (1), 16185. doi:10.1038/nenergy.2016.185
Parr Robert, G., Y. W. (1989). Density-Functional Theory of Atoms and Molecules. New York: Oxford University Press.
Paul, T., Das, D., Das, B. K., Sarkar, S., Maiti, S., and Chattopadhyay, K. K. (2019). CsPbBrCl2/g-C3N4 Type II Heterojunction as Efficient Visible Range Photocatalyst. J. Hazard. Mater. 380, 120855. doi:10.1016/j.jhazmat.2019.120855
Perdew, J. P., Ernzerhof, M., and Burke, K. (1996). Rationale for Mixing Exact Exchange with Density Functional Approximations. J. Chem. Phys. 105 (22), 9982–9985. doi:10.1063/1.472933
Schneider, J., Jia, H., Muckerman, J. T., and Fujita, E. (2012). Thermodynamics and Kinetics of CO2, CO, and H+ Binding to the Metal centre of CO2reductioncatalysts. Chem. Soc. Rev. 41 (6), 2036–2051. doi:10.1039/c1cs15278e
Sehgal, M. L. (2017). Calculation of Spin Orbit Coupling of Tungsten (III) Complexes: A DFT Application. Orient. J. Chem. 33, 1045–1046. doi:10.13005/ojc/330263
Shi, Z., and Jayatissa, A. (2018). Perovskites-Based Solar Cells: A Review of Recent Progress, Materials and Processing Methods. Materials 11 (5), 729. doi:10.3390/ma11050729
Sun, S., Tominaka, S., Lee, J-H., Xie, F., Bristowe, P. D., and Cheetham, Ak. (2016). Synthesis, crystal Structure, and Properties of a Perovskite-Related Bismuth Phase, (NH4)3Bi2I9. APL Mater. 4 (3), 031101. doi:10.1063/1.4943680
Sutton, R. J., Eperon, G. E., Miranda, L., Parrott, E. S., Kamino, B. A., Patel, J. B., et al. (2016). Bandgap-Tunable Cesium Lead Halide Perovskites with High Thermal Stability for Efficient Solar Cells. Adv. Energ. Mater. 6 (8), 1502458. doi:10.1002/aenm.201502458
Tang, C., Chen, C., Xu, W., and Xu, L. (2019). Design of Doped Cesium lead Halide Perovskite as a Photo-Catalytic CO2 Reduction Catalyst. J. Mater. Chem. A. 7 (12), 6911–6919. doi:10.1039/c9ta00550a
Umari, P., Mosconi, E., and De Angelis, F. (2014). Relativistic GW Calculations on CH3NH3PbI3 and CH3NH3SnI3 Perovskites for Solar Cell Applications. Scientific Rep. 4 (1), 4467. doi:10.1038/srep04467
van Schilfgaarde, M., Kotani, T., and Faleev, S. (2006). Quasiparticle Self-Consistent GW Theory. Phys. Rev. Lett. 96 (22), 226402. doi:10.1103/PhysRevLett.96.226402
Volonakis, G., Filip, M. R., Haghighirad, A. A., Sakai, N., Wenger, B., Snaith, H. J., et al. (2016). Lead-free Halide Double Perovskites via Heterovalent Substitution of Noble Metals. J. Phys. Chem. Lett. 7 (7), 1254–1259. doi:10.1021/acs.jpclett.6b00376
Volonakis, G., Haghighirad, A. A., Milot, R. L., Sio, W. H., Filip, M. R., Wenger, B., et al. (2017). Cs2InAgCl6: A New Lead-Free Halide Double Perovskite with Direct Band Gap. J. Phys. Chem. Lett. 8 (4), 772–778. doi:10.1021/acs.jpclett.6b02682
Wang, K., Jin, Z., Liang, L., Bian, H., Wang, H., Feng, J., et al. (2019). Chlorine Doping for Black γ-CsPbI3 Solar Cells with Stabilized Efficiency beyond 16%. Nano Energy 58, 175–182.
Wang, X.-D., Huang, Y.-H., Liao, J.-F., Jiang, Y., Zhou, L., Zhang, X.-Y., et al. (2019). In Situ Construction of a Cs2SnI6 Perovskite Nanocrystal/SnS2 Nanosheet Heterojunction with Boosted Interfacial Charge Transfer. J. Am. Chem. Soc. 141 (34), 13434–13441. doi:10.1021/jacs.9b04482
Wang, X., He, J., Li, J., Lu, G., Dong, F., Majima, T., et al. (2020). Immobilizing Perovskite CsPbBr3 Nanocrystals on Black Phosphorus Nanosheets for Boosting Charge Separation and Photocatalytic CO2 Reduction. Appl. Catal. B: Environ. 277, 119230. doi:10.1016/j.apcatb.2020.119230
Wu, Y., Wang, P., Zhu, X., Zhang, Q., Wang, Z., Liu, Y., et al. (2018). Composite of CH3 NH3 PbI3 with Reduced Graphene Oxide as a Highly Efficient and Stable Visible-Light Photocatalyst for Hydrogen Evolution in Aqueous HI Solution. Adv. Mater. 30 (7), 1704342. doi:10.1002/adma.201704342
Xiaobo, C., Shen, S., Guo, L., and Mao, S. S. (2010). Semiconductor-based Photocatalytic Hydrogen Generation. Chem. Rev. 110 (11), 6503–6570. doi:10.1021/cr1001645
Xu, Y.-F., Yang, M.-Z., Chen, B.-X., Wang, X.-D., Chen, H.-Y., Kuang, D.-B., et al. (2017). A CsPbBr3 Perovskite Quantum Dot/Graphene Oxide Composite for Photocatalytic CO2 Reduction. J. Am. Chem. Soc. 139 (16), 5660–5663. doi:10.1021/jacs.7b00489
Yan, J., Qiu, W., Wu, G., Heremans, P., and Chen, H. (2018). Recent Progress in 2D/quasi-2D Layered Metal Halide Perovskites for Solar Cells. J. Mater. Chem. A. 6 (24), 11063–11077. doi:10.1039/c8ta02288g
Ye, S., Wang, R., Wu, M.-Z., and Yuan, Y.-P. (2015). A Review on G-C3n4 for Photocatalytic Water Splitting and CO2 Reduction. Appl. Surf. Sci. 358, 15–27. doi:10.1016/j.apsusc.2015.08.173
Yu, C.-J. (2019). Advances in Modelling and Simulation of Halide Perovskites for Solar Cell Applications. J. Phys. Energ. 1 (2). doi:10.1088/2515-7655/aaf143
Yu, Z. B., Xie, Y. P., Liu, G., Lu, G. Q., Ma, X. L., and Cheng, H.-M. (2013). Self-assembled CdS/Au/ZnO Heterostructure Induced by Surface Polar Charges for Efficient Photocatalytic Hydrogen Evolution. J. Mater. Chem. A. 1 (8), 2773–2776. doi:10.1039/c3ta01476b
Yu, Z., Chen, X.-Q., Kang, X., Xie, Y., Zhu, H., Wang, S., et al. (2018). Noninvasively Modifying Band Structures of Wide-Bandgap Metal Oxides to Boost Photocatalytic Activity. Adv. Mater. 30 (14), 1706259. doi:10.1002/adma.201706259
Yue, D., Zhang, T., Wang, T., Yan, X., Guo, C., Qian, X., et al. (2020). Potassium Stabilization of Methylammonium lead Bromide Perovskite for Robust Photocatalytic H2 Generation. EcoMat 2 (1). doi:10.1002/eom2.12015
Zeng, S., Kar, P., Thakur, U. K., and Shankar, K. (2018). A Review on Photocatalytic CO2 Reduction Using Perovskite Oxide Nanomaterials. Nanotechnology 29 (5), 052001. doi:10.1088/1361-6528/aa9fb1
Zhang, J., Bai, D., Jin, Z., Bian, H., Wang, K., Sun, J., et al. (2018). 3D-2D-0D Interface Profiling for Record Efficiency All-Inorganic CsPbBrI2 Perovskite Solar Cells with Superior Stability. Adv. Energ. Mater. 8 (15), 1703246. doi:10.1002/aenm.201703246
Zhang, Q., Ting, H., Wei, S., Huang, D., Wu, C., Sun, W., et al. (2018). Recent Progress in lead-free Perovskite (-like) Solar Cells. Mater. Today Energ. 8, 157–165. doi:10.1016/j.mtener.2018.03.001
Zhao, Y., Wang, Y., Liang, X., Shi, H., Wang, C., Fan, J., et al. (2019). Enhanced Photocatalytic Activity of Ag-CsPbBr3/CN Composite for Broad Spectrum Photocatalytic Degradation of Cephalosporin Antibiotics 7-ACA. Appl. Catal. B: Environ. 247, 57–69. doi:10.1016/j.apcatb.2019.01.090
Zhao, Z., Wu, J., Zheng, Y.-Z., Li, N., Li, X., Ye, Z., et al. (2019). Stable Hybrid Perovskite MAPb(I1−Br )3 for Photocatalytic Hydrogen Evolution. Appl. Catal. B: Environ. 253, 41–48. doi:10.1016/j.apcatb.2019.04.050
Zhu, X.-Y., and Podzorov, V. (2015). Charge Carriers in Hybrid Organic-Inorganic Lead Halide Perovskites Might Be Protected as Large Polarons. J. Phys. Chem. Lett. 6 (23), 4758–4761. doi:10.1021/acs.jpclett.5b02462
Keywords: first-principles calculations, photocatalysis, halide perovskites, density functional theory, heterojunctions
Citation: Liu X, Fan J and Huang C (2021) Advances in Theoretical Calculation of Halide Perovskites for Photocatalysis. Front. Nanotechnol. 3:695490. doi: 10.3389/fnano.2021.695490
Received: 15 April 2021; Accepted: 01 June 2021;
Published: 14 June 2021.
Edited by:
Yi Yu, ShanghaiTech University, ChinaReviewed by:
Tao Cheng, Soochow University, ChinaZijing Ding, University of Science and Technology of China, China
Copyright © 2021 Liu, Fan and Huang. This is an open-access article distributed under the terms of the Creative Commons Attribution License (CC BY). The use, distribution or reproduction in other forums is permitted, provided the original author(s) and the copyright owner(s) are credited and that the original publication in this journal is cited, in accordance with accepted academic practice. No use, distribution or reproduction is permitted which does not comply with these terms.
*Correspondence: Xiaolin Liu, eGxsaXVAc2hpZXAuZWR1LmNu