- 1Department of Chemistry, Thiagarajar College, Madurai, India
- 2Department of Bioelectronics and Biosensors, Alagappa University, Karaikudi, India
In this work, we have fabricated a new electrochemical sensor based on β‐tricalcium phosphate (Ca3(PO4)2) nanoparticles (NPs) modified glassy carbon electrode (GCE) for the selective nonenzymatic determination of methyl parathion and mercury (II) ions independently. β‐tricalcium phosphate (β‐TCP) NPs were prepared by chemical precipitation method and structural and morphological properties were investigated by XRD, FTIR, and SEM. The electrochemical behavior of MP and mercury (Hg2+) ions were investigated by cyclic voltammetry (CV) and square wave voltammetry (SWV) techniques using β‐TCP/GCE. The modified electrode exhibited excellent electrocatalytic activity towards both the MP and Hg over a wide linear range from 0.15 to 141 μM and 1–381 µM with the lowest detection limits of 88 and 136.4 nM respectively. The sensor has high selectivity towards MP and Hg in the presence of major interfering compounds such as 3-nitrophenol, 4-nitrophenol, 4-aminophenol, catechol, hydroquinone and heavy metals such as lead, cadmium and arsenic. Applicability of the fabricated sensor for detection of MP and Hg (II) ions has been tested in tap water by standard addition method.
Introduction
Organophosphorus pesticides (OPs) are extensively used as insecticides in the field of agriculture to protect crops, seeds and to get high yield with high quality (Mulchandani et al., 2001; Obare et al., 2010). However, these pesticides are neurotoxic which can cause several diseases in both humans and animal (Silva et al., 2004; Chuan et al., 2015). Methyl parathion (MP) is a type of nitro aromatic organophosphorus pesticide which is widely used to prevent agricultural losses by controlling pests and insects. It is used prevalently due to its cost effectiveness and high insecticidal activity (Niazi and Yazdanipour, 2007; Sanghavi et al., 2012). MP’s ability to bio-accumulate and its low solubility means that it can easily be adsorbed on the surface of water and soil which could result in various problems to our eco-system. Moreover, high dosages of MP lead to inhibition of acetyl cholinesterase activity in the brain and leads to disturbances in normal neuron transmission (Yang et al., 2008; Thota and Ganesh, 2016). More than 100 thousand people die every year due to MP pesticide driven contamination of food and drinking water. Therefore, with the spotlight on public health, there is a need to develop reliable, highly sensitive and easily operable methods for the monitoring of MP concentration in the environment and food items. Various methods such as spectroscopic, chromatographic techniques like high performance liquid chromatography (Cappiello et al., 2002; Hyötyläinen et al., 2004; Berijani et al., 2006; Moinfar and Hosseini, 2009; Hassan et al., 2010) and electrochemical methods (Shulga and Kirchhoff, 2007; Liang et al., 2012) have been used for determination of MP. Among them, electrochemical techniques were most suitable for monitoring the MP concentration, because of its high sensitivity, selectivity and low-cost (Liu and Lin, 2006; Du et al., 2011). Though there are electrochemical sensors for MP determination, these methods depend heavily on enzymes (Mulchandani et al., 1999; Liu and Lin, 2006; Gong et al., 2009; Du et al., 2011; Hossain et al., 2011; Li et al., 2011; Du et al., 2012; Ge et al., 2013). These enzyme based electrochemical sensors are usually less favored for practical usage because they suffer from many disadvantages such as high cost, instability, de-naturation, difficulty in getting pure form of enzymes, subsequent storage problems and short life time. In order to overcome these problems, novel enzyme free sensor devices for methyl parathion detection such as MWCNTs–PAAM) based composite (Zeng et al., 2012), carboxylic acid functionalized single walled carbon nanotubes-cyclodextrin (f-SWCNTs-CD) (Yao et al., 2014), mono-6-thio-cyclodextrin–gold nanoparticles–single walled carbon nanotubes SHCD/AuNPs/SWCNTs (Fu et al., 2015), gadolinium hexacyano ferrate–graphene nanosheets (GdHCF/GNs) (Li et al., 2014) zirconia nanoparticles (ZrO2NPs) (Parham and Rahbar, 2010) have been developed.
Heavy metal ions such as cadmium, lead, arsenic, mercury are major contributors to environmental pollution, albeit in very low concentrations, because of their undesirable involvement in many natural and industrial applications. Mercury and its compounds are highly toxic and when they accumulate in human organs such as liver, heart muscle, and brain. They can easily interact with protein and enzymes which, in turn, results in the inactivation of some important cell functions in our body leading to various diseases like kidney dysfunction, central nervous system disorders, even death at high exposure (Harris et al., 2003; Triunfante et al., 2009; Bodo et al., 2010; Johri et al., 2010). As a result, attention has been directed to the determination of mercury ions in foodstuff and pharmaceutical products and the environment. Traditional analytical tools for the determination of mercury ions include atomic absorption spectroscopy (AAS) (Kenawy et al., 2000; Pohl, 2009) and inductively coupled plasma mass spectrometry (ICP-MS) (Caroli et al., 1999; Silva et al., 2009). Compared to the above-mentioned techniques, electrochemical methods offer several advantages in terms of cost, simplicity and quickness. Moreover, in electrochemical techniques, different voltammetric methods can be employed for mercury detection (Aragay and Merkoc, 2012). Several reports are available for electrochemical determination of mercury ion; PdO/NP-CPE (El Aroui et al., 2014), EDTA/CPE (Moutcine and Chtaini, 2018), EDTA-PANI/SWCNTs/SS (Deshmuk et al., 2018), Chloroplatinum (II) complex-MWCNTs paste electrode (Mdisa et al., 2017) and Av- HA/GCE (Kanchana et al., 2015).
Calcium phosphate (Ca/P) bioceramics are well known bioactive compound and are used in surgical fields for orthopedic and dental implants, because of their excellent osteoconductivity. Hydroxyapatite (Ca10(PO4)6(OH), octacalcium phosphate (Ca8H2(PO4)6·5(H2O)), tricalcium phosphate (Ca3(PO4)2), calcium hydrogen phosphate dihydrate (CaHPO4.2H2O), dicalcium phosphate anhydrous (CaHPO4), tetracalcium phosphate (Ca4(PO4)2O) and amorphous calcium phosphate (Ryu et al., 2002) are different crystalline calcium phosphates that have been applied in many biological mineralization processes. Among them, tricalcium phosphate has been given great attention and it is widely used as an ideal temporary scaffold for bone implant and dental repair material, because of its close chemical resemblance to biological apatite present in human hard tissues. Tricalcium phosphate can be divided into three forms based on its thermal stability namely α, ß- and α′-phases. Of these, a-TCP is hydrolysable while ß-TCP is a stable material with superior properties such as non-toxicity, antigenicity, non-carcinogenicity, high biodegradability and thermal stability (Tadayyon et al., 2003). Though with relatively poor performance in terms of the lowest detection limit and linear range, the a-TCP modified carbon paste electrode (TCP/CPE) was used for the determination of Pb2+ by electrochemical method (Chourak et al., 2016).
The present work reports the fabrication of an enzyme free electrochemical sensor for methyl parathion determination and mercury ion detection using ß-tricalcium phosphate modified GCE. The fabricated electrode was employed for determination of MP and mercury (II) ions in tap-water samples.
Materials and Methods
Reagents
Methyl Parathion was purchased from Sigma Aldrich, Mercuric Chloride, Calcium Nitrate tetra hydrate and di-ammonium hydrogen phosphate were purchased from Merck Private Limited, Mumbai. All other chemicals were of analytical reagent grade and used without any further purification. 0.1 M of Phosphate buffer solution was prepared from Na2HPO4 and NaH2PO4 (Merck, Mumbai) and the pH was adjusted to seven and five for MP and Hg respectively by adding 0.1 M HCl. De-ionized water was used throughout the experiments.
Apparatus
Electrochemical measurements were carried out on CHI 609D workstation. A three-electrode cell system was used with a modified glassy carbon electrode as the working electrode. An Ag/AgCl was used as the reference electrode and a platinum wire electrode as the counter electrode. All experiments were carried out at room temperature. Cyclic voltammograms (CVs) were acquired at a scan rate of 50 mVs−1 in 0.1 M phosphate buffer containing various concentrations of methyl parathion and HgCl2 individually. The structure and morphology of the ß-TCP were characterized by powder X-ray powder diffraction (XRD) using a XPERT-PRO diffractometer with Cu Kα radiation (1.5406Å) at 40 kV and Field emission scanning electron microscopy (FE-SEM) using FEG Quanta. Fourier transform infrared (FTIR) observations were recorded from 400 to 4,000 cm−1 at room temperature with a Schimadzu-IR- Affinity by KBr pellet technique.
Synthesis of ß-Tricalcium phosphate
β-Tricalcium phosphate was prepared by a simple chemical precipitation method. Initially, calcium nitrate tetrahydrate [Ca(NO3)2·4H2O] powder and di-ammonium hydrogen phosphate [(NH4)2HPO4] solution were mixed with constant stirring to get homogenous solution. The solution turned white color turbid immediately which was made transparent by adding phosphate ion solution into calcium. Subsequently, ammonium solution (NH4OH) was added drop wise in the above mixture to maintain the pH 8.0. Stirring was continued for 2°h, and the precipitate was allowed to settle overnight, then filtered, dried and finally calcinated at 700°C in ambient atmosphere.
Electrode Preparation
Glassy carbon electrodes (3 mm dia) were polished with 0.05 mm alumina powder on polishing cloth, rinsed thoroughly with deionized water between each polishing step, sonicated and dried at room temperature. These electrodes were modified by drop casting method. Here, tricalcium phosphate nanoparticles were dispersed into deionized water (5°mg/ml−1) by ultrasonicating for about 30 min to get a stable and uniform suspension. Typically, 10 µL of this ß -TCP suspension was drop casted onto the GCE and the water was allowed to evaporate at room temperature in air.
Result and Discussion
Characterization of Synthesized Powder
Powder XRD pattern of the pristine ß-Tricalcium phosphate sample is shown in (Figure 1A). The XRD pattern corresponds well with that of the standard ß-TCP crystallites (ICDD PDF Card No-09–0,169) indicating that the product obtained is mainly composed of the ß-TCP form of calcium phosphate with rhombohedral structure. No characteristics peaks of impurities such as calcium hydroxide and calcium phosphates were observed. Crystallite size was calculated by the Debye- Scherer formula (D = Kλ/β1/2cosθ) where λ is the wavelength, β1/2 is the full width at half maximum (FWHM), θ is the diffraction angle, K is the shape factor constant. The average crystallite size of ß -TCP was calculated as 28 nm after several measurements.
(Figure 1B) shows the FT-IR spectra of pure ß-TCP samples. The IR characteristic peaks of phosphate groups appeared between 900 and 1,160 cm−1. In particular, the peak around at 924 cm−1 confirms the formation of high crystalline ß-tricalcium phosphate phase. A small peak at 728 cm−1 is attributed to the presence of PO4− group, which is characteristic of calcium pyrophosphate phase. The peaks between 900 and 1,200 cm−1 represent the stretching vibration of phosphate ions. The presence of two characteristic bands at around 568 and 604 cm−1 is due to the asymmetric stretching vibration of PO3− (Supplementary Figure S1A,B) shows FE-SEM micrographs of the ß-TCP at two different magnifications confirm the formation of homogenously distributed elongated rod-shaped nanoparticles.
Electrochemical Investigation of Methyl Parathion
Cyclic voltammetry was used to investigate the electrochemical behavior of MP at the ß-TCP modified GCE in PBS (pH 7.0) at a scan rate 50 mV/s (Supplementary Figure S2A). shows the CVs of 50 μM MP at bare GCE (curve a) and a ß-TCP/GCE (curve b) respectively. Noticeably, a small defined oxidation wave and large reduction peak appeared for both bare and modified GCEs. The large cathodic peak shows the reduction of nitrogroup in MP to 2-phenylhydroxylamine. During the reverse scan, a small anodic peak indicates the conversion of hydroxylamine to nitrobenzene. When compared with bare GCE, the ß- TCP/GCE exhibited significantly enhanced redox reaction which could partly be attributed to the efficient adsorption property of tricalcium phosphate nanoparticles. No obvious peak was observed on the ß-TCP/GCE in the absence of MP. A sharp reduction peak appeared after incubation of 50 µM of MP in electrolyte solution in (Supplementary Figure S2B). These results suggest that the ß-TCP catalyzes the reaction and enhance the reduction of MP through electron transfer at the electrode-electrolyte interface.
(Supplementary Figure S2C) shows the effect of scanning rate on cyclic voltammograms of 50 μM MP at the ß-TCP/GCE in PBS at pH 7.0. The reduction peaks increase linearly with the increase of scan rates from 10 to 100 mVs−1and exhibit a linear relation to the square root of the scan rate, which implies that the redox reaction of MP at the ß-TCP/GCE is a surface adsorption-controlled process. The above results also demonstrate that the proposed sensor facilitates electron transfer towards MP sensing. The methyl parathion has different electrochemical behaviors in different electrolytes. The effects of electrolytes, such as HCl, phosphate buffer, acetate buffer, and KNO3, on reduction peak currents of MP were studied and the results show that the MP has the best electrochemical responses in 0.1 M PBS with the appearance of well defined cathodic peaks with the lowest background current.
(Supplementary Figure S2D) shows the cyclic voltammograms of 50 µM of MP at ß-TCP/GCE in 0.1 M PBS at different pH values. The pH was varied from four to nine by the addition of HCl or NaOH in PBS electrolyte. The best peak current with lowest background current appeared for pH 7.0. At lower low pH values, the peak current is reduced possibly due to the immobilization of MP on the electrode surface and/or dissolution of ß-TCP in acidic medium. When the pH is higher than 7.0, peak current decreased and the shape of the peak is changed due to the lack of protons H+. Hence, we have chosen pH value as 7.0 for all subsequent measurements.
Determination of Methyl Parathion by SWV
Electrochemical determination of MP at ß-TCP/GCE was realized by square wave voltammetric technique in 0.1 M PBS (pH 7.0) (Figure 2A). shows the voltammograms recorded during addition of aliquot from the stock of solution methyl parathion. It was noted that the cathodic peak current increases linearly with the increasing concentration of MP from 0.15 to 141 µM. A linear relationship between the cathodic peak current ipa (μA) and concentrations C (μM) of MP has been deduced as Ipa (μA) = −4.6229CMP (μM)−5.09 (R2 = 0.99518) (Figure 2B). The lowest limit of detection (LOD) was estimated as 88 nM and the results are compared with the literature values (Supplementary Table S1). This significant LOD over a wide linear range could be attributed to the intrinsic porous nature, efficient adsorptivity and improved electrocatalytic ability of the ß-TCP nanoparticles.
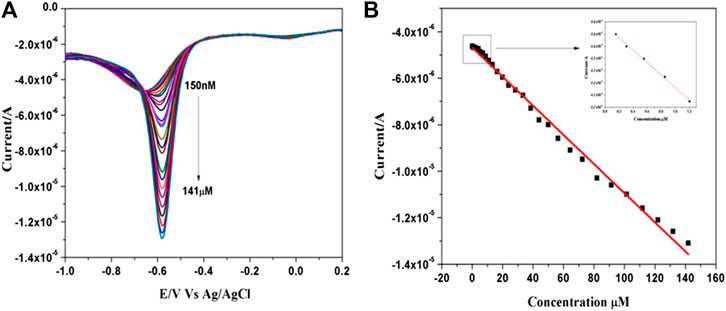
FIGURE 2. (A) SWVs recorded for various concentration of MP ranging from 0.15 to 141 µM in 0.1 M PBS (pH 7.0) at ß-TCP/GCE. (B) Corresponding linear calibration plot.
Selectivity, Reproducibility and Stability Studies for Methyl Parathion Sensor
A significant benefit of ß-TCP NPs is its good selectivity towards MP sensing by avoiding and discriminating the interference of several isostructural compounds. Here we have evaluated the spirited test for selectivity by square wave voltammetry technique (Supplementary Figure S3). shows an interference study of MP sensor in the presence of 100 fold excess of co-existing phenolic compounds such as four- aminophenol, nitrophenol isomers, hydroquinone, catechol, and some other compounds like nitrite, SO42−, Mg2+, K+, and Na+. The presence of these competing molecules and ions did not have an obvious influence on the current response of MP on the ß-TCP/GCE. The results demonstrate that the ß-TCP sensor can be used directly for detecting MP even in complex solutions. To characterize the reproducibility of the ß-TCP/GCE, 15 consecutive measurements were made for 50 μM MP at the same electrode. The results showed a relative standard deviation (RSD) of 2.97%, revealing that the ß- TCP modified GCE had good reproducibility. The stability of the ß-TCP/GCE sensing device was also examined by measuring CV peak current of 50 μM MP after storing the electrode for 10 days in a refrigerator. The ß-TCP/GCE retained 91% of the original values, demonstrating that the sensing device had better long-term stability.
Real Sample Analysis
The analytical application of the fabricated sensor was tested in tap water and vegetable samples by the standard addition method. Methyl parathion stock solution was added into tap water in 0.1 M PBS (pH 7.0) and the measurements were done at ß-TCP/GCE without any prior treatment and the results are presented in Supplementary Table S2. It can be noticed that fabricated sensor exhibits a good recovery in the range of 96–98% for MP determination in tap water. Similarly, square wave voltammograms were recorded for Momordica Charantia extract (colloquially known as bitter gourd) in the presence of various concentration of MP in 0.1 M PBS and the results are shown in Supplementary Table S2. Here also, the ß-TCP/GCE revealed highest percentage of recovery (90–100%) indicating its suitability for real sample analysis.
Electrochemical Detection of Mercury (Hg2+) at ß -TCP/GCE
(Figure 3A) shows the cyclic voltammetric behavior of mercury (Hg2+) obtained in 0.1 M PBS (pH 5.0) containing 0.1 mM HgCl2 solution at bare GCE and ß-TCP modified GCE at the scan rate of 50 mVs−1. A sharp anodic oxidation peak is observed at 0.197 V corresponding to oxidation of metallic mercury (Hg0→Hg2++ 2e–) in both bare and ß-TCP modified GCE. The peak current (ipa) values of bare GCE and ß-TCP/GCE were found to be 23.3 and 35.2 μA respectively. There is a reasonable increment in the oxidation peak current of TCP/GCE when compared to that of bare GCE which reveals that the ß-TCP has good catalytic activity. The porous nature of TCP leads to the high adsorption of mercury ion on the surface of the electrode which promotes high electron transfer at the electrode-electrolyte interface without requiring any pre-concentration time as reported in literature (Kanchana et al., 2015). Mercury (Hg2+) ions are known to have different electrochemical behaviors in different electrolytes. The effects of electrolytes, such as HCl, phosphate buffer, acetate buffer, and KNO3, on oxidation peak currents of Hg2+ were studied and the results show that the Hg2+ ions have the best electrochemical responses in 0.1 M PBS. When the measurements were performed in this electrolyte, well defined peaks with the lowest background current were obtained.
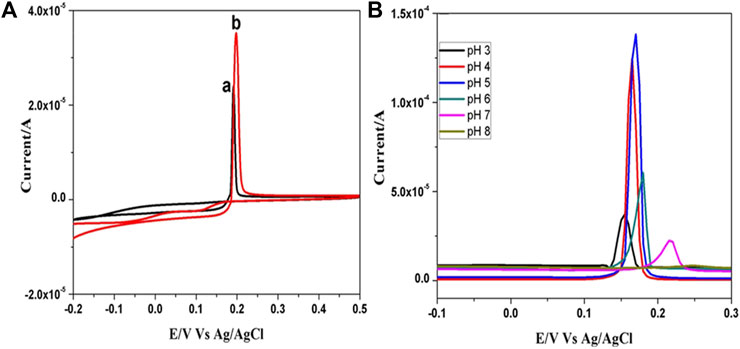
FIGURE 3. (A) CVs of 0.1 mM Hg2+ in 0.1 M PBS (pH 5.0) at a) bare GCE,b) ß-TCP modified GCE at scan rate 50 mVs−1; (B) SWV studies of 1mM Hg2+ in 0.1 M PBS at different pH from three to eight at ß-TCP/GCE at scan rate 50 mVs−1.
The effect of pH on the oxidation of mercury at the ß-TCP/GCE in 0.1 M PBS containing 1 mM HgCl2 solution was investigated by SWV studies (Figure 3B). The anodic peak current is strongly dependent on the pH of the solution with the maximum current observed at pH 5.0. At higher pH values (>5.0), the peak current decreased rapidly and the peak shape was also destroyed which could be attributed to the high participation of OH− resulting in the hydrolysis of Hg2+ to form mercury hydroxide. Similarly, SWV measurements at lower pH values (<5.0) yield lower oxidation current due to the dissolution of ß-TCP in high acidic medium. Based on these observations, pH 5.0 was chosen as the optimum value for the supporting electrolyte.
Square wave voltammetry (SWV) was used to examine the sensitivity of the fabricated electrode towards the detection of Hg2+ ions (Figure 4A). shows the SWV response of the ß- TCP/GCE to the successive addition of mercury in 0.1 M PBS at pH 5.0. The electrode exhibited a rapid and sensitive response to the change of mercury concentration and an obvious increase in anodic peak current upon successive addition of mercury. Further, the current increased linearly with increasing Hg2+ concentration over the range of 1–380 µM and a linear relationship between the anodic peak current ipa (μA) and concentrations C (μM) of Hg2+ have been deduced as follows; Ipa (μA) = 0.576 CHg2+ (μM)+7.638 (R2 = 0.99784) (Figure 4B). The lowest detection limit was found to be 136 nM and is comparable with the results obtained from many sensors (Supplementary Table S3) as reported in the literature. The present experiments demonstrated that it is possible to determine Hg2+ using ß-TCP modified GCE without surface fouling of the electrode for repeated measurements. These results confirmed that ß-TCP modified electrode provides a good electro catalytic activity towards the detection of heavy metal mercury.
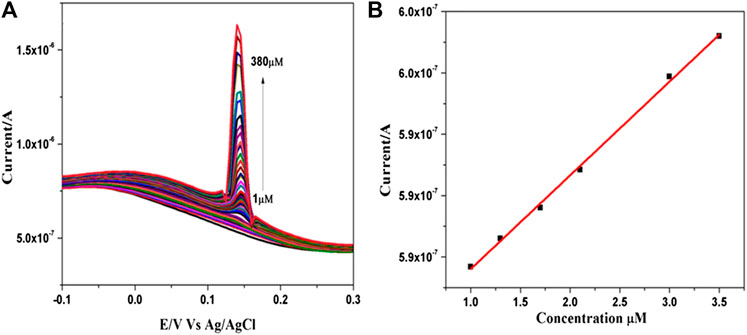
FIGURE 4. (A) SWVs recorded for various concentration of HgCl2 ranging from 1 to 380 µM in 0.1 M PBS (pH 5.0) at ß-TCP modified GCE. (B) Corresponding linear calibration plot.
Tap water samples were selected for real sample analysis using the standard addition method. The developed sensor (TCP/GCE) was applied to test mercury ion in tap water samples. Standard stocks mercuric chloride solution was added into tap water and analyzes were done without any prior treatment. The results are presented in (Supplementary Table S4). It is obvious from the table that a good recovery could be obtained. Thus, the proposed sensor can be used to determine mercury ions in environmentally polluted water samples.
Conclusion
A novel and simple strategy for the sensitive detection of methyl parathion and heavy metal mercury individually using the ß-TCP/GCE is presented in this paper for the first time. The results confirm that in the case of unmodified bare GCE, the voltammograms of both MP and mercury (II) ion exhibit just a small peak current, whereas the peak current is significantly enhanced on ß-TCP/GCE. Well-defined reduction peak for MP and enhanced oxidation peak current for mercury (II) ion have been obtained owing to the excellent catalytic activity of ß-TCP NPs. In addition, the ß -TCP/GCE electrode exhibited good reproducibility, long-term stability, and high sensitivity without using any specific electron transfer mediator or any other surfactant. The modified electrode shows the extraordinary selectivity for determination of methyl parathion and mercury without interference of high concentration of co-existing molecules. Furthermore, the practical application of the modified electrode was demonstrated in tap water samples by using SWV method with satisfactory results.
Data Availability Statement
The raw data supporting the conclusions of this article will be made available by the authors, without undue reservation.
Author Contributions
NS carried out the nanoparticles synthesis, experiments, analysed the XRD, FTIR, SEM, electrochemical behavior and wrote the paper; CS supervised the whole work and revised the paper.
Conflict of Interest
The authors declare that the research was conducted in the absence of any commercial or financial relationships that could be construed as a potential conflict of interest.
Acknowledgments
NS acknowledges with thanks the Alagappa University for providing (AURF Fellowship/2015) financial assistance. The authors CS and NS thank to Mr. S. Lokeswara Reddy and Ms.P.Archana for real sample analysis during this pandemic period.
Supplementary Material
The Supplementary Material for this article can be found online at: https://www.frontiersin.org/articles/10.3389/fnano.2021.632652/full#supplementary-material.
References
Aragay, G., and Merkoçi, A. (2012). Nanomaterials application in electrochemical detection of heavy metals. Electrochim. Acta 84, 49–61. doi:10.1016/j.electacta.2012.04.044
Berijani, S., Assadi, Y., Anbia, M., Milani Hosseini, M. R., and Aghaee, E. (2006). Dispersive liquid-liquid microextraction combined with gas chromatography-flame photometric detection. J. Chromatogr. A 1123, 1–9. doi:10.1016/j.chroma.2006.05.010
Bodo, M., Balloni, S., Lumare, E., Bacci, M., Calvitti, M., Dell’Omo, M., et al. (2010). Effects of sub-toxic Cadmium concentrations on bone gene expression program: results of an in vitro study. Toxicol. In Vitro. 24, 1670–1680. doi:10.1016/j.tiv.2010.05.020
Cappiello, A., Famiglini, G., Palma, P., and Mangani, F. (2002). Trace level determination of organophosphorus pesticides in water with the new direct-electron ionization LC/MS interface. Anal. Chem. 74, 3547–3554. doi:10.1021/ac015685f
Caroli, S., Forte, G., Iamiceli, A. L., and Galoppi, B. (1999). Determination of essential and potentially toxic trace elements in honey by inductively coupled plasma-based techniques. Talanta 50, 327–336. doi:10.1016/S0039-9140(99)00025-9
Chourak, R., Elouahli, A., Hatim, Z., Chtaini, A., and Kheribech, A. (2016). “Electro analytical determination of lead using tricalcium phosphate apatite modified carbon paste electrode,” in Research and reviews in electrochemistry 06 304155314 © 2016 (Trade Science Inc).
Chuan, Y., Hong, S., Hua-Feng, F., and Zhi-Cheng, T. (2015). Sensitive simultaneous determination of nitrophenol isomers at poly(p-aminobenzene sulfonic acid) film modified graphite electrode. Electrochim. Acta. 156, 163–170. doi:10.1016/j.elecom.2006.11.021
Deshmuk, M. A., Celiesiute, R., Ramanaviciene, A., Shirsat, M. D., and Ramanavicius, A. (2018). EDTA_PANI/SWCNTs nanocomposite modified electrode for electrochemical determination of copper (II), lead (II) and mercury (II) ions. Electrochim. Acta. 259, 930–938. doi:10.1016/j.electacta.2017.10.131
Du, D., Liu, J., Zhang, X., Cui, X., and Lin, Y. (2011). One-step electrochemical deposition of a graphene-ZrO2 nanocomposite: preparation, characterization and application for detection of organophosphorus agents. J. Mater. Chem. 21, 8032–8037. doi:10.1039/C1JM10696A
Du, D., Wang, J., Wang, L., Lu, D., and Lin, Y. (2012). Integrated lateral flow test strip with electrochemical sensor for quantification of phosphorylated cholinesterase: biomarker of exposure to organophosphorus agents. Anal. Chem. 84, 1380–1385. doi:10.1021/ac202391w
El Aroui, F., Lahrich, S., Farahi, A., Achak, M., El Gaini, L., Manoun, B., et al. (2014). Electrochemical determination of mercury(II) in ambient water at palladium oxide/graphite composite electrodes. J. Taiwan Inst. Chem. Eng. 45, 2725–2732. doi:10.1016/j.jtice.2014.05.003
Fu, X-C., Zhang, J., Tao, Y-Y., Wu, J., Xie, C-G., and Kong, L-T. (2015). Three-dimensional mono-6-thio-β-cyclodextrin covalently functionalized gold nanoparticle/single-wall carbon nanotube hybrids for highly sensitive and selective electrochemical determination of methyl parathion. Electrochimica Acta 153, 12–18. doi:10.1016/j.electacta.2014.11.144
Ge, X., Zhang, W., Lin, Y., and Du, D. (2013). Magnetic Fe3O4@TiO2 nanoparticles-based test strip immunosensing device for rapid detection of phosphorylated butyrylcholinesterase. Biosens. Bioelectron. 50, 486–491. doi:10.1016/j.bios.2013.07.017
Gong, J., Wang, L., and Zhang, L. (2009). Electrochemical biosensing of methyl parathion pesticide based on acetylcholinesterase immobilized onto Au-polypyrrole interlaced network-like nanocomposite. Biosens. Bioelectron. 24, 2285–2288. doi:10.1016/j.bios.2008.11.012
Harris, H. H., Pickering, I. J., and George, G. N. (2003). The chemical form of mercury in fish. Science 301, 1203. doi:10.1126/science.1085941
Hassan, J., Farahani, A., Shamsipur, M., and Damerchili, F. (2010). Rapid and simple low density miniaturized homogeneous liquid-liquid extraction and gas chromatography/mass spectrometric determination of pesticide residues in sediment. J. Hazard. Mater. 18, 869–871. doi:10.1016/j.jhazmat.2010.08.008
Hossain, M. M., Faisal, S. N., Kim, C. S., Cha, H. J., Nam, S. C., and Lee, H. J. (2011). Amperometric proton selective strip-sensors with a microelliptic liquid/gel interface for organophosphate neurotoxins. Electrochemistry Commun. 13, 611–614. doi:10.1016/j.elecom.2011.03.024
Hyötyläinen, T., Lüthje, K., Rautiainen-Rämä, M., and Riekkola, M-L. (2004). Determination of pesticides in red wines with on-line coupled microporous membrane liquid-liquid extraction-gas chromatography. J. Chromatogr. A. 1056, 267–271. doi:10.1016/j.chroma.2004.06.112
Johri, N., Jacquillet, G., and Unwin, R. (2010). Heavy metal poisoning: the effects of cadmium on the kidney. Biometals 23, 783–792. doi:10.1007/s10534-010-9328-y
Kanchana, P., Sudhan, N., Anandhakumar, S., Mathiyarasu, J., Manisankar, P., and Sekar, C. (2015). Electrochemical detection of mercury using biosynthesized hydroxyapatite nanoparticles modified glassy carbon electrodes without preconcentration. RSC Adv. 5, 68587–68594. doi:10.1039/C5RA11424A
Kenawy, I. M. M., Hafez, M. A. H., Akl, M. A., and Lashein, R. (2011). Determination by AAS of some trace heavy metal lons in some natural and biological samples after their preconcentration using newly chemically modified chloromethylated polystyrene-PAN ion-exchanger. Anal. Sci. 16, 493–500. doi:10.2116/analsci.16.493
Li, H., Li, J., Yang, Z., Xu, Q., and Hu, X. (2011). A novel photoelectrochemical sensor for the organophosphorus pesticide dichlofenthion based on nanometer-sized titania coupled with a screen-printed electrode. Anal. Chem. 83, 5290–5295. doi:10.1021/ac200706k
Li, Y., Xu, M., Li, P., Dong, J., and Ai, S. (2014). Nonenzymatic sensing of methyl parathion based on graphene/gadolinium Prussian Blue analogue nanocomposite modified glassy carbon electrode. Anal. Methods 6, 2157–2162. doi:10.1039/C3AY41820K
Liang, H., Miao, X., and Gong, J. (2012). One-step fabrication of layered double hydroxides/graphene hybrid as solid-phase extraction for stripping voltammetric detection of methyl parathion. Electrochemistry Commun. 20, 149–152. doi:10.1016/j.elecom.2012.04.010
Liu, G., and Lin, Y. (2006). biosensor based on self-assembling acetylcholinesterase on carbon nanotubes for flow injection/amperometric detection of organophosphate pesticides and nerve agents. Anal. Chem. 78, 835–843. doi:10.1021/ac051559q
MdIsa, I., Saidin, M. I., Ahmad, M., Hashim, N., Bakar, S. A., Ali, N. M., et al. (2017). Chloroplatinum(II) complex-modified MWCNTs paste electrode for electrochemical determination of mercury in skin lightening cosmetics Electrochim. Acta. 253, 463–471. doi:10.1016/j.electacta.2017.09.092
Moinfar, S., and Hosseini, M-R. M. (2009). Development of dispersive liquid-liquid microextraction method for the analysis of organophosphorus pesticides in tea. J. Hazard. Mater. 169, 907–911. doi:10.1016/j.jhazmat.2009.04.030
Moutcine, A., and Chtaini, A. (2018). Electrochemical determination of trace mercury in water sample using EDTA-CPE modified electrode. Sensing Bio-sensing Res. 17, 30–35. doi:10.1016/j.sbsr.2018.01.002
Mulchandani, A., Mulchandani, P., Chen, W., Wang, J., and Chen, L. (1999). Amperometric thick-film strip electrodes for monitoring organophosphate nerve agents based on immobilized organophosphorus hydrolase. Anal. Chem. 71, 2246–2249. doi:10.1021/ac9813179
Mulchandani, A., Chen, W., Mulchandani, P., Wang, J., and Rogers, K. R. (2001). Biosensors for direct determination of organophosphate pesticides. Biosens. Bioelectron. 16, 225–230. doi:10.1016/s0956-5663(01)00126-9
Niazi, A., and Yazdanipour, A. (2007). Spectrophotometric simultaneous determination of nitrophenol isomers by orthogonal signal correction and partial least squares. J. Hazard. Mater. 146, 421–427. doi:10.1016/j.jhazmat.2007.03.063
Obare, S. O., De, C., Guo, W., Haywood, T. L., Samuels, T. A., Adams, C. P., et al. (2010). Fluorescent chemosensors for toxic organophosphorus pesticides: a review. Sensors 10 (7), 7018–7043. doi:10.3390/s100707018
Parham, H., and Rahbar, N. (2010). Square wave voltammetric determination of methyl parathion using ZrO2-nanoparticles modified carbon paste electrode. J. Hazard. Mater. 177, 1077–1084. doi:10.1016/j.jhazmat.2010.01.031
Pohl, P. (2009). Determination of metal content in honey by atomic absorption and emission spectrometries. TrAC Trends Anal. Chem. 28, 117–128. doi:10.1016/j.trac.2008.09.015
Ryu, H. S., Youn, H. J., Hong, K. S., Chang, B. S., Lee, C. K., and Chung, S. S. (2002). An improvement in sintering property of β-tricalcium phosphate by addition of calcium pyrophosphate. Biomaterials 23, 909–914. doi:10.1016/S0142-9612(01)00201-0
Sanghavi, B. J., Hirsch, G., Karna, S. P., and Srivastava, A. K. (2012). Potentiometric stripping analysis of methyl and ethyl parathion employing carbon nanoparticles and halloysite nanoclay modified carbon paste electrode. Anal. Chim. Acta. 735, 37–45. doi:10.1016/j.aca.2012.05.029
Shulga, O., and Kirchhoff, J. R. (2007). An acetylcholinesterase enzyme electrode stabilized by an electrodeposited gold nanoparticle layer. Electrochem. commun. 9, 935–940. doi:10.1016/j.elecom.2006.11.021
Silva, D., Cortez, C. M., Cunha-Bastos, J., and Louro, S. R. W. (2004). Methyl parathion interaction with human and bovine serum albumin. Toxicol. Lett. 147, 53–61. doi:10.1016/j.toxlet.2003.10.014
Silva, E. L., Roldan, P. D. S., and Giné, M. F. (2009). Simultaneous preconcentration of copper, zinc, cadmium, and nickel in water samples by cloud point extraction using 4-(2-pyridylazo)-resorcinol and their determination by inductively coupled plasma optic emission spectrometry. J. Hazard. Mater. 171, 1133–1138. doi:10.1016/j.jhazmat.2009.06.127
Tadayyon, A., Arifuzzaman, S. M., and Rohani, S. (2003). Reactive crystallization of brushite under steady state and transient conditions: modeling and experiment. Ind. Eng. Chem. Res. 42, 6774–6785. doi:10.1021/ie030354+
Thota, R., and Ganesh, V. (2016). Selective and sensitive electrochemical detection of methyl parathion using chemically modified overhead projector sheets as flexible electrodes. Sensors and Actuators B. 227, 169–177. doi:10.1016/j.snb.2015.12.008
Triunfante, P., Soares, M. E., Santos, A., Tavares, S., Carmo, H., and Bastos, M. D. L. (2009). Mercury fatal intoxication: two case reports. Forensic Sci. Int. 184, e1-e6. doi:10.1016/j.forsciint.2008.10.023
Yang, J., Yang, C., Jiang, H., and Qiao, C. (2008). Overexpression of methyl parathion hydrolase and its application in detoxification of organophosphates. Biodegradation 19, 831–839. doi:10.1007/s10532-008-9186-2
Yao, Y., Zhang, L., Xu, J., Wang, X., Duan, X., and Wen, Y. (2014). Rapid and sensitive stripping voltammetric analysis of methyl parathion in vegetable samples at carboxylic acid-functionalized SWCNTs-β-cyclodextrin modified electrode. J. Electroanalytical Chem. 713, 1–8. doi:10.1016/j.jelechem.2013.11.024
Keywords: ß-Tricalcium phosphate, electrochemical sensor, methyl parathion (MP), mercury ion, environmental applications and catalysis
Citation: Sudhan N and Sekar C (2021) Nanostructured β‐tricalcium Phosphate (Ca3(PO4)2 Based Electrochemical Sensor for Detection of Methyl Parathion and Mercury (II) Ions. Front. Nanotechnol. 3:632652. doi: 10.3389/fnano.2021.632652
Received: 04 January 2021; Accepted: 16 February 2021;
Published: 16 April 2021.
Edited by:
Ajeet Kaushik, Florida Polytechnic University, United StatesReviewed by:
Arunkumar Rengaraj, UMR5628 Laboratoire des Matériaux et du Génie Physique (LMGP), FranceSandeep Dumbali, University of Texas Health Science Center at Houston, United States
Copyright © 2021 Sudhan and Sekar. This is an open-access article distributed under the terms of the Creative Commons Attribution License (CC BY). The use, distribution or reproduction in other forums is permitted, provided the original author(s) and the copyright owner(s) are credited and that the original publication in this journal is cited, in accordance with accepted academic practice. No use, distribution or reproduction is permitted which does not comply with these terms.
*Correspondence: Chinnathambi Sekar, c2VrYXIyMDI1QGdtYWlsLmNvbQ==