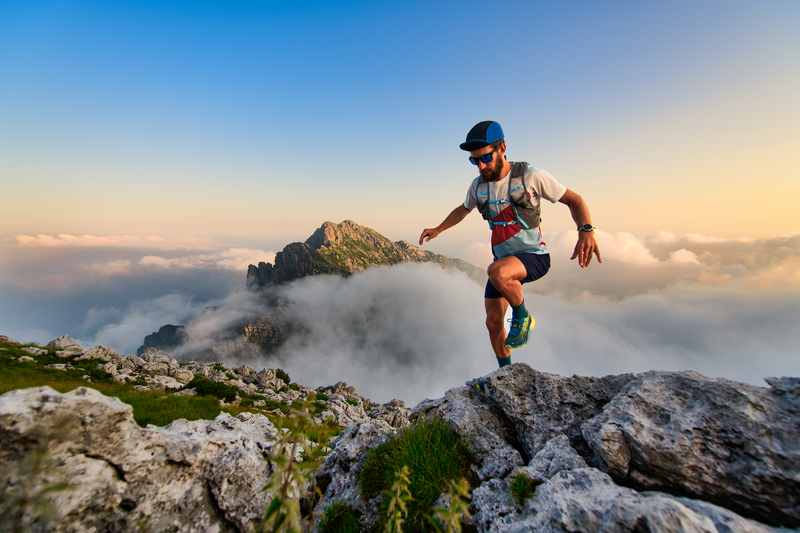
95% of researchers rate our articles as excellent or good
Learn more about the work of our research integrity team to safeguard the quality of each article we publish.
Find out more
REVIEW article
Front. Nanotechnol. , 09 December 2020
Sec. Biomedical Nanotechnology
Volume 2 - 2020 | https://doi.org/10.3389/fnano.2020.610465
This article is part of the Research Topic Nanotechnology Solutions to Mitigate COVID-19: Detection, Protection, Medication View all 13 articles
Since its first emergence in December 2019, the coronavirus-2 infection has quickly spread around the world and the severity of the pandemic has already re-shaped our lives. This review highlights the role of nanotechnology in the fight against this pandemic with a focus on the design of effective nano-based prevention and treatment options that overcome the limitations associated with conventional vaccines and other therapies. How nanotechnology could be utilized to understand the pathology of the ongoing pandemic is also discussed as well as how our knowledge about SARS-CoV-2 cellular uptake and toxicity could influence future nanotoxicological considerations and nanomedicine design of safe yet effective nanomaterials.
In December 2019, a novel severe acute respiratory syndrome-coronavirus-2 (nCoV or SARS-CoV-2) emerged and rapidly progressed into a global pandemic, as declared on 11 March 2020 (Baloch et al., 2020), shutting economies and leaving societies in a state of uncertainty. At the time of writing this article (End of October 2020), more than 42 million cases were confirmed, and more than 1.1 million deaths reported globally (WHO, 2020d). While treatment options are still lacking, there have been joint efforts from both medical and scientific professionals to come up with effective treatments that would minimize the severe impact of the pandemic. Tremendous work has been put so far into this either to understand the mechanism of SARS-CoV-2 infection, Accelerate drug repurposing, develop novel treatments and mass vaccination. This review focuses on the contribution of the biomedical nanotechnology community to the pandemic and how SARS-CoV-2 could reshape the future of nanomedicine and nanotoxicology. For other potential applications of nanotechnology to the pandemic please refer to some excellent reviews that have been recently published that gave a comprehensive overview on: (1) the application of nanotechnology in the design of effective therapeutics and viral vaccines (Chauhan et al., 2020; Shin et al., 2020; Weiss et al., 2020), (2) the potential of hard nanomaterials in the fight again the pandemic(Reina et al., 2020), and (3) the potential of nanomaterial for efficient viral detection and the design of disinfectants and personal protective equipment (Sportelli et al., 2020; Weiss et al., 2020).
The therapeutic options considered so far are mainly based on repurposing other treatments developed for related viral infections such as SARC-COV, MERS-COV by taking advantages of some of the similarities found between them (WHO, 2020a; Zhou et al., 2020). Examples of those include; different antiviral drugs [e.g., lopinavir/ritonavir and remdesivir (Beigel et al., 2020; NCT04381936, 2020)] to inhibit viral replication, plasma from COVID-19 recovered patients who have developed antibodies for the virus (NCT04381936, 2020), immune modulators such as anti-interleukin-6 (Campochiaro and Dagna, 2020), anti-inflammatory treatments, e.g., dexamethasone (NCT04381936, 2020) and interleukin 1 receptor agonist IL-1Ra (NCT04443881, 2020) as well as vaccines [such as attenuated viruses (NCT04456595, 2020), messenger RNA (mRNA) (NCT04283461, 2020), DNA (NCT04447781, 2020), and recombinant proteins (NCT04466085, 2020)] delivered through either viral-vectors (NCT04516746, 2020) or via nanoparticles (NCT04368728, 2020). The results of the ongoing trials with these treatments are not yet conclusive with only a few options showing promising live-saving effects such as dexamethasone (NCT04381936, 2020).
Viruses are naturally occurring nanoparticles that operate at the same metric scale as other nanomaterials. For years the nanomedicine community invested lots of efforts to copy viruses behavior by designing viral-like nanoparticles for efficient targeted therapy and gene delivery (Jeevanandam et al., 2019; Hashemzadeh et al., 2020). It is therefore not surprising that nanotechnology tools offered great utility in the ongoing pandemic through different routes spinning from viral neutralization and detection to vaccine developments and treatment (Mujawar et al., 2020). This section will cover the most outstanding nanomedicine platforms that have been tested in those areas and highlight the promises that these hold so far.
The availability of viral information in record time at the start of the outbreak (Rao et al., 2015; Grifoni et al., 2020; GISAID Next hCoV-9 App, 2020), helped catalyze the design and development of many vaccine candidates. In total, the world health organization (WHO) reported 44 vaccine candidates in clinical trials and 154 in preclinical stages (WHO, 2020b). For full overview of those please refer to the WHO DRAFT landscape of COVID-19 candidate vaccines (WHO, 2020b) and other excellent review articles that have detailed the different vaccine technologies developed so far (Chauhan et al., 2020; Florindo et al., 2020; Shin and Shukla, 2020). Several of those are novel vaccines designed using nanotechnology tools. From those different technologies mRNA vaccine formulated as lipid nanoparticles was the first to progress into a clinical trial (NCT04283461, 2020). The urgency created by the pandemic prompted the leverage of many traditional approaches in vaccine development including; live attenuated viruses (LAV), inactivated viruses and viral vectors which are after all considered natural nanoparticles. LAV are based on live reproducing but avirulent viruses that result in a single-dose immunity without illness (Shin et al., 2020). The maturity of this technology allowed their rapid progression into clinical trial and Codagenix Incorporation's is currently leading this area (Shin et al., 2020). Although this technology has been in use for a long time, it still raises safety concerns as it carries the risk of reverting into the infectious state or becoming reactivated in immunocompromised individuals (Luo et al., 2018). This is quite worrying for a novel disease like COVID19 especially that the pathological consequences are not fully understood (Shin et al., 2020).
Other vaccine approaches are based on repurposing mammalian virus vectors that have been developed for other applications. From those, the leading candidates in COVID-19 is the chimpanzee adenovirus vaccine vector (ChAdOx1) developed by the University of Oxford named as AZD1222 which is currently in Phase III testing (NCT04516746, 2020). The advantages of this class of vaccines are in their inherent adjuvant capability and scalability. However, the downside of this therapeutic modality is the risk of developing immunity in individuals who have been exposed to similar viruses before, which could compromise the vaccine efficacy (Mullard, 2020).
Due to the limitations observed in the vaccine candidates listed above, novel candidates were developed. Nucleic acid-based vaccines have shown a great promise especially those acting by delivering genetic material (either as DNA or mRNA) via nanoparticles (Figure 1). This stimulates the production of specific viral proteins such as the spike protein that the virus uses to bind and enter the host cells. For this class, the use of nanoparticles is decisive to protect the structure of the genetic material besides the added advantage of improved cellular uptake, ability to deliver multiple antigens to antigen-presenting cells and training the immune system to produce both antibody and T cell responses (Pardi et al., 2018; Smith et al., 2020). Nucleic acid-based vaccines are also superior to traditional ones in terms of safety, ease of design, stability and scalability (Shin et al., 2020). At the forefront in DNA vaccines is INO-4800 combined with electroporation which progressed into Phase I clinical trial on the 7th of April 2020 (NCT04336410, 2020) and Covigenix vaccine developed by Entos pharmaceuticals Inc. which uses Fusogenix drug delivery platforms (Pharmaceuticals, 2020) and few other candidates (WHO, 2020c) which are still in phase I/II clinical trials. The ability to produce mRNA vaccine by in vitro transcription offers added benefits and can therefore overcome many regulatory hurdles (Iavarone et al., 2017). mRNA is also non-integrating compared to DNA vaccines and thus lacks the issues of insertional mutagenesis. Within this area, Moderna's mRNA-vaccine (mRNA-1273) was the first to enter phase I clinical testing on the 16th March 2020 (NCT04283461, 2020) and is currently in phase III (NCT04470427, 2020). This was then followed by BioNTech-Pfizer vaccine candidate (BNT-162) which also reached phase III (NCT04368728, 2020). To take this a step further, scientists at Imperial College London (ISRCTN17072692, 2020) and Arcturus Therapeutics (NCT04480957, 2020) developed self-replicating RNA vaccines delivered via lipid nanoparticles (LNPs). The encapsulation of self-replicating RNA in lipid nanoparticles (LNPs) has a critical role in the success of this type of vaccines. LNPs protects the RNA from enzymatic degradation; which translates into a more efficient mRNA expression. LNPs are not limited by anti-vector immunity since unlike viral vectors they do not carry any surface proteins. Moreover, RNA amplification and protein expression processes take place in the cytoplasm, which avoid any risk of genetic integration (Geall et al., 2012). In addition, the half-life of RNA can be extended and a higher level of the viral spike protein can be achieved with a lower initial dose. Although nucleic acid-based vaccines have not been clinically approved yet, this technology is becoming very popular following the very recent approval of RNA interference products [patisiran (Adams et al., 2018) and givosiran (Balwani et al., 2020)]. Besides, many efforts have been directed in recent years toward the development of nano-cancer vaccines which provided a solid platform that was efficiently repurposed for COVID-19 vaccines. For mRNA vaccines, the synthetic lipid nanoparticles are integral to their success in order to overcome the delivery challenge and facilitate nucleic acid trafficking at the cellular and subcellular levels (Pardi et al., 2018). In addition to lipid nanoparticles, other nanocarriers are also emerging in this field including; nanoemulsions, cationic polymers, peptides, dendrimers, natural and synthetic polysaccharides, etc. (Shin et al., 2020). Another promising nanotechnology platform for vaccine development is the mannosylated polymeric vaccine. This platform has been developed before for cancer prevention and has demonstrated strong cellular and humoral immune responses (Conniot et al., 2019). This technology is currently being tested preclinically for SARS-CoV2 in combination with immunomodulators (Florindo et al., 2020).
Figure 1. Schematic presentation of nucleic acid-based vaccine technologies which includes either; (1) DNA vaccines that delivers plasmid to the nucleus of antigen presenting cell and (2&3) RNA vaccines which deliver either replicating or non-replicating mRNA into the cytoplasm of antigen presenting cells. Nucleic acid-based vaccines stimulate the production of specific viral proteins such as the spike protein that the virus uses to bind and enter host cells. For this class, the use of nanoparticles is decisive to protect the structure of the genetic material besides the added advantage of improved cellular uptake, ability to deliver multiple antigens and training the immune system to produce both antibody and T cell responses.
Apart from enabling the development of modern vaccines, nanotechnology platforms demonstrated excellent ability in viral neutralization. In this approach, cellular nanosponges were designed by covering the surface of the nanoparticles with human-cell derived membrane. These nanoparticles are made to display angiotensin-converting enzyme 2 (ACE2) and CD147 receptors that mediate viral entry into host cells (Zhang et al., 2020). Once the virus binds to the receptors at the surface of nanoparticles, it becomes no longer available to infect the usual cellular targets (Zhang et al., 2020). This technology is still in its preclinical stage and further validation in animal models are required before its translation into the clinical testing.
Another advantage that nanomedicine offers in this pandemic is through targeting the SARS-CoV-2 viral RNA using RNA interference (RNAi). Interesting examples developed previously for other infections include; gene silencing of SARS M protein gene expression using double-stranded RNAs (Patent application CN101173275) or siRNAs to silence SARS genes encoding RNA polymerase, helicase, nucleoprotein N, and proteolytic enzymes (Patent application CN1569233). In these examples, excellent viral inhibition was achieved reaching between 50 and 90% antiviral activity (Chauhan et al., 2020).
From repurposed medications that have been tested for COVID-19 in the RECOVERY Trial (NCT04381936, 2020), dexamethasone was the first to demonstrate live-saving activity, particularly in severe cases. Since in those cases, fatality is triggered by an overreaction of the immune response leading to hyperinflammation and macrophage activation syndrome. This causes the overproduction of several proinflammatory cytokines or what is called as the “cytokine storm” and also trigger coagulation abnormalities causing multiple organ failure and sadly death (Merad and Martin, 2020). Dexamethasone reduced the risk of death by 35% for patients in intensive care units and shortened the hospitalization time for COVID-19 patients. Until now it is considered the only drug to demonstrate an impact and improved patient survival (Ledford, 2020). The other attractive side about dexamethasone is being of low cost, widely available and has been used for many years in the treatment of other pathologies. Nanomedicine formulations of dexamethasone have been previously developed and already demonstrated positive results in Phase I/II clinical trials in multiple myeloma patients (NCT03033316, 2017) and many other preclinical models of rheumatoid arthritis (Quan et al., 2014), inflammatory bowel disease (Bartneck et al., 2015), wound healing (Gauthier et al., 2018) and cancer (Banciu et al., 2008). Therefore, treatment of COVID-19 patients with liposomal dexamethasone is expected to outperform the free drug by taking advantages of nanomaterials tendency to accumulate in hyperactivated alveolar macrophages that play a critical role in the trigger of the disease. Ultimately this is expected to improve oedema resolution and fibrinolytic effects (Lammers et al., 2020). Similar effects have already been demonstrated from liposomal amikacin which is approved in 2019 for complex lung infection with Mycobacterium avium (Zhang et al., 2018). Apart from targeting local inflammation, liposomal dexamethasone can also accumulate in the spleen and bone marrow and therefore has a clear potential to attenuate peripheral inflammation (Metselaar et al., 2003). Although understandably, liposomal dexamethasone is more expensive than the free drug, if its efficacy proves to outperform free dexamethasone formulations then this might save more lives and could significantly reduce healthcare costs in the long term.
Moreover, the unique properties of nanomaterials (e.g., thermal, electrical and optical, etc.) make them ideal for developing highly sensitive sensors for biomarkers detection. Although this area is outside the scope of this review, we would like to mention here that new diagnostics tools based on nanomaterials have already been developed and approved for other infectious diseases such as Ebola (Tsang et al., 2016) and Zika (Song et al., 2016; Afsahi et al., 2018) viruses. Recently, Moitra et al. reported the development of a rapid and accurate colorimetric assay that is suitable for detecting positive COVID-19 cases from RNA samples in only 10 minutes. In this assay, gold nanoparticles (AuNPs) are surface-functionalized with thiol-modified antisense oligonucleotides specific for multiple genetic regions of nucleocapsid phosphoprotein of SARS-CoV-2 to ensure detection sensitivity. Once thiol capped AuNPs are in contact with the target RNA sequence of SARS-CoV-2, agglomeration occurs leading to a change in AuNPs surface plasmon resonance within a few minutes. Amplification with ribonuclease H leads to visual detection of AuNPs precipitation by the naked eye without the need for highly specialized instruments (Moitra et al., 2020). All of the above makes such nanotechnology platform ideal for mass screening as it is simple, affordable, rapid, user friendly and can operate without the need for sophisticated instruments. As such, this could make a generic sensor platform that can be easily adapted to detect different genetic regions of the virus such as the spike gene (S-gene) or envelope gene (E-gene) or could be easily adapted for future infectious disease and pathologies. For other related examples of nanotechnology-based platforms for COVID-19 detection please refer to a recent excellent review on that (Talebian et al., 2020).
Despite the wide range of nanomaterials, their different applications and varied interactions with cells and organs, SARS-CoV-2 virus belongs to the exciting family of nature-inspired nanoparticles. SARS-CoV2 virus interaction with cells has proven to lead to a dramatic nanotoxicity and damage to different organs leading to death if the body defense system is unable to defend itself or to shut it down. Nature has always surprised us with the development of such terrific systems that not only do they feed on our bodies and cause illnesses (such as the flu and now the COVID-19 pandemic), but also show unique ability to transfect cells which have led to their development as powerful drug delivery systems (Xu et al., 2020). Viruses are therefore double-edged-swords with harmful effects yet bringing positive therapeutic opportunities. While COVID-19 has and might change our lives forever, one should learn from the SARS-CoV-2 virus interaction with cells and organs and apply this knowledge in the future development of safe nanomaterials. Therefore, what have we learnt so far from SARS-CoV- 2 and what can we reap and use in the development of safe and effective nanomedicines?
It has been reiterated that the power of SARS-CoV-2 is in its cellular entry mechanism which is highly dependent on the presence of angiotensin-converting enzyme 2 (ACE2) receptors in the lungs (Xu et al., 2020) as represented in Figure 2. More specifically these receptors are present in alveolar epithelial cells, macrophages in the lung as well as vascular endothelial cells (Varga et al., 2020; Zhou et al., 2020). It has also been reported that the virus induces down-regulation of ACE2 which can be an important factor in the pathology of the disease especially that vascular permeability in the airways, as well as inflammation, are enhanced as a consequence (Tay et al., 2020).
Figure 2. Schematic representation of SARS-CoV-2 entry in cells through the ACE2 receptors Reprinted from “SARS-CoV-2 Targeting of ACE2 Receptor and Entry in Infected Cell” template, by BioRender.com (2020). Available online at: https://app.biorender.com/biorender-templates.
While specificity and targeting have always been the dream in any design of nanomedicines, we can clearly see that these are the principles by which SARS-CoV-2 interacted with cells. The so-called enhanced permeation and retention, or EPR effect for short, has always been employed in the design of nanoparticles to achieve passive targeting in cancer therapy. The focus has therefore been in the careful tailoring of the physicochemical properties of nanoparticles especially size and charge. In addition, active targeting (through the attachment of targeting ligands such as specific antibodies, aptamers and vitamins) has been the highlight and focus in the design of targeted drug delivery systems. In the case of COVID-19, the structure of SARS-CoV-2 virus allowed for the specific targeting of lung cells through ACE2 receptors and cellular uptake via clathrin-mediated endocytosis (Brahim Belhaouari et al., 2020). The Spike (S) protein on the SARS-CoV-2 virus, in particular, derives the entry of the virus into cells with the S1 surface unit targeting ACE2 receptors (Figure 2). Moreover, it is suggested that the cellular entry of SARS-CoV-2 is primed by transmembrane protease, serine 2 (TMPRSS2) which leads to the S protein cleavage and fusion of viral and cellular membranes (Hoffmann et al., 2020). While this is a “live” nanoparticle, it is still important to consider the effects of synthetic nanoparticles on cellular membranes and clearly understand if any proteins or structures within the membrane facilitate the entry of NPs especially those that do not rely on targeting ligands. For instance, with carbon nanotubes (CNTs), molecular dynamics simulation highlighted that salt bridges can facilitate the flip-flop and translocation of CNTs to the cytoplasm (Lopez et al., 2004) but is there more to this story than what we are aware of? Moreover, one still wonders about what facilitates the cellular uptake of 2D like nanomaterials such as graphene oxide. Even with spherical NPs such as gold NPs (AuNPs), we continue to learn about what drives their interaction with lipid membranes. Contini et al. have recently described, using a model liposomal membrane, the existence of size thresholds at 10 and 50 nm that can determine different fates of citrate coated AuNPs interaction with membranes (Contini et al., 2020). While technology was initially limited in exploring the cellular membrane, there are now versatile approaches to track membrane proteins and control their movement such that described by Li et al. which uses fluorescent magnetic nanoparticles (FMNPs) (Li et al., 2020). The latter allows single-particle tracking at 10 nm and 5 milliseconds spatiotemporal resolution and in this way, the direct effects of NPs on membrane proteins can perhaps be studied.
Besides, with a new paradigm evolving in regard to the extravasation of nanomedicine in tumors (de Lázaro and Mooney, 2020; Sindhwani et al., 2020) and brain (Al-Ahmady et al., 2019) through transcytosis rather than the EPR effect, it is time to revisit our understanding of nanomedicines cellular uptake and interaction with cells and organs. SARS-CoV-2 might bring us closer to the perfect experimental design to fundamentally understand any potential direct or indirect effects of nanoparticles on cells. Moreover, drug delivery has always focused on cancer therapy through nanomedicines intravenous administration and it is now the perfect time to apply this knowledge in the development of novel therapeutics for the treatment of other diseases. The fact that SARS-CoV-2 nanoparticles have led to a significant systemic effect through inhalation, means the use of different routes of administrations rather than the traditional intravenous route should be further explored for nanomedicines. Although this strong systemic effect seen with SARS-CoV-2 might sound alarming to some, it will definitely allow the nanotoxicology community to better refine current nano-risk assessments as well as to think wider about the potential effects of NPs in vivo following human exposure (for example through inhalation).
Understanding where nanoparticles end up being within the cells is as important as our understanding of their pharmacokinetics in vivo. Cellular kinetics will help in the design of site-specific nanoparticles as well as punctual nanomedicines that can deliver the therapeutic cargo in a timely fashion within cells. Belhaouari et al. observed the infectious cycle of SARS-CoV2 using scanning electron microscopy and found that its virions were located at the cell surface early post-infection (Brahim Belhaouari et al., 2020) and that the virus corona spikes were sandwiched between the particles and the cellular membrane. As most spherical nanoparticles that are taken up inside the cell via endocytosis, the virus then escapes the endosome and the single-stranded RNA is released within the cytoplasm where replication and transcription take place through the replication/transcription complex (RTC) (Boopathi et al., 2020).
However, one of the main consequences of the cellular translocation of CoV-SARS-2 is the disruption and downregulation of ACE2 which leads to unregulated inflammatory response and a severe lung pathology (Offringa et al., 2020). What is crucial here is to understand the molecular basis that allows for such disruption to ACE2 to occur and investigate whether synthetic NPs could cause similar effects on certain proteins? Moreover, there is a growing evidence that the SARS-CoV-2 can cause analgesia through its effects on the VEGF-A/Neuropilin-1 receptor signaling pathway (Moutal et al., 2020). It was found that SARS-CoV-2 not only binds to ACE2 protein but also to neuropilin-1, which is a transmembrane glycoprotein highly expressed in the respiratory and olfactory epithelium, vasculature and has several roles within the nervous system (Cantuti-Castelvetri et al., 2020). Within most of the nanotoxicology studies, the effect of nanoparticles on cells and organs is usually purely concentrated on the overall death or live effects and the complete understanding of the effects of nanoparticles on signaling pathways for example and proteins levels is often neglected. Recent advances in remotely controlling cellular processes using biofunctional magnetic nanoparticles have created the field of “magnetogenetics” (Monzel et al., 2017) but in the absence of magnetism what can NPs further do to cells in addition to them just getting inside. It is therefore crucial to completely understand how nanoparticles behave at the molecular level to allow for a better and safe design of nanomedicines with a higher success rate of translation to the clinic.
In addition to the lungs, ACE2 is also expressed in other organs such as the heart, kidneys and intestines (Crackower et al., 2002; Hamming et al., 2004; Soler et al., 2013; Fan et al., 2020). This meant that SARS-CoV-2 can cause damage to these organs and has therefore proven to be a fierceful virus. In this infection, one can think of the body as one entity and if one organ is attacked, then the rest of the body and organs are affected.
While a fever, a dry cough, and shortness of breath are the common early signs of COVID-19, pneumonia can develop as a consequence of SARS-CoV-2 infection (George et al., 2020). While the virus directly infects the air sacs cell linings, it also triggers an immune response causing an inflammation and fluid collection in the lung tissues. This is not surprising news as some nanoparticles have been reported to cause cell and lung epithelium damage especially following repeated exposures. For example, Shvedova et al. showed that the pharyngeal aspiration of single-walled carbon nanotubes (SWNTs) dispersed in PBS lead to an early onset of an acute inflammation which progressed into fibrosis and granuloma formation (Shvedova et al., 2005). However, the physicochemical properties of SWNTs and their aggregation could have led to these pulmonary effects. Direct inhalation toxicological studies with nanoparticles are non-existing and most models used (pharyngeal aspiration, intraperitoneal injections) only mimic to a certain extent what could really happen if NPs are inhaled. Determining the actual inhaled dose(s) of NPs is challenging and this has limited the specific design of direct NPs inhalation studies. The question that poses itself here is about whether the current doses used in these pseudo-inhalation studies are too high leading to such detrimental effects or they are too low and therefore the real consequences are perhaps not picked-up.
With SARS-CoV-2 being a “live” nanoparticle this has meant that (1) its nanosized particle size helped in its travel within the lungs, (2) that the targeted nature of the virus enhanced its specificity to particular cells, and (3) its replication within tissues potentiated its ferocity. Moreover, the recent discovery of lioneic acid (LA) presence within a pocket in the Spike protein which is affecting LA metabolic pathways and leading to the dramatic inflammation and pneumonia observed with COVID-19 (due to the importance of LA in maintaining cellular membranes in lungs) has unveiled a common character seen with NPs (Toelzer et al., 2020). While this is a significant discovery in understanding SARS-CoV-2, it isn't really a surprising one in the nano-field as it is known that NPs can adsorb other materials and a good example about this is the tremendous work done on the protein corona and its effects on the toxicity of NPs as reviewed in Neagu et al. (2017).
While SARS-CoV-2 has proven to be a savage virus through its associated complications causing coagulation disorders for COVID-19 patients (Al-Samkari et al., 2020; Kander, 2020) and other extrapulmonary ramifications (Gupta et al., 2020) such those on the heart (Samidurai and Das, 2020), brain (Lu et al., 2020), kidney (Fanelli et al., 2020) and gastrointestinal tract (Ng and Tilg, 2020; Villapol, 2020), there are still limited studies on the effects of synthetic NPs on these organs following different routes of administration. Perhaps the slight exception is with CNTs as there are more studies about their pseudo-inhalation consequences due to some resemblance to asbestos fibers. For instance, Legramante et al. described that SWNTs can cause dysautonomia following intratracheal administration in rats which was manifested as an increase in baroreflex sequences and a decrease in heart rate (Legramante et al., 2009). The other pseudo-inhalation studies that discuss the effects of NPs on some of those other organs are summarized in these excellent reviews (Mann et al., 2012; Kan et al., 2018).
However, we are still far from including such studies regularly in the nanotoxicology assessments of NPs. This could be due to absence of tools such as relevant disease models or expensive animal experimentation or lack of expertise in the area. Nevertheless, the establishment of centers like the European Nanomedicine Characterization Laboratory (euNCL) and Nanotechnology Characterization Lab in the US (NCL) may facilitate these studies through the usage of their many well-developed assay cascades. These assays might however not be relevant to all NPs such as carbon-based nanomaterials due to the problems of interference (Ali-Boucetta et al., 2011) but the nanomedicine/nanotoxicology communities can help in applying their already developed assays for use in these centers. The COVID-19 pandemic has highlighted more than ever the need to study the effects of nanoparticles on multiple organs following acute and long term dosing but more importantly the effects of comorbidities (such as obesity, diabetes and cardiovascular diseases) (Johns et al., 1996; King, 2012) on nanoparticles toxicological consequences and severity. While older animals can be used to replicate the age effect, the most challenging of all will be to replicate the ethnicity within animals to better understand the predisposition of people to illnesses like COVID-19 but more so to an increased toxicity risk after exposure to nanoparticles either unintentionally or intentionally for therapeutic purposes. Will some healthy individuals be more susceptible to NPs than others? This will be the question that needs answering in the near future especially as more nanomedicines are making their way to the clinic.
In an attempt to reduce animal testing, new in vitro models are evolving and will hopefully allow us to better understand the potential and toxicological effects of NPs (Stueckle and Roberts, 2019). With the severity of the COVID-19 disease, scientists around the globe quickly started developing COVID-19 animal models (Imai et al., 2020; Singh et al., 2020) but more interestingly relevant 3D models known as organoids to be able to understand the drastic effects of SARS-CoV-2 in vitro (Allison, 2020; Clevers, 2020; Dickson, 2020; Elbadawi and Efferth, 2020; Yang et al., 2020). The joint research efforts in understanding SARS-CoV-2 gives hope that similar efforts will be put together to combat other illnesses such as cancer and neurodegenerative disorders. It has also allowed us to realize that what is seen with SARS-CoV-2 is a form of nanotoxicity and that several new tools such as artificial intelligence have been deployed to understand the disease and develop therapies to combat it (Ahuja et al., 2020; Shaker et al., 2020). Therefore, similar tools should be used in the design of future nanotoxicology studies but also in the development of bioinspired drug delivery systems like viruses (Aulicino et al., 2020).
Advances in nanotechnology design and fabrication set the foundation for several innovative solutions many of those have been repurposed in response to SARS-CoV-2 which highlights the potential that nanotechnology hold for several applications (Kaushik, 2019; Shin et al., 2020). Accelerated translation of those technologies into the clinic and breaking barriers of regulatory authorities indicates a build of trust in this growing field. The use of lipid nanoparticle, viral based vaccines, viral-like nanoparticles and other hard nanomaterials for vital detection and fabrication of personal protection equipment has been at the forefront in the COVID-19 pandemic. While these technologies are all very promising, it is understandable that some may take many months before proving their potential and may not realize their true impact for COVID- 19 pandemic. However, this will lay the basis for these platform technologies to adapt to other currently existing health challenges and future health crises. We believe that this will be a big push for nanomedical applications and become an incentive for the scientific and industrial communities, stakeholders, funding and regulatory bodies to invest more effort in this ever-growing field.
An interesting feature of nanomedicine is the ability to provide a generic platform that can be easily adapted to suit the application in need. This has been extensively demonstrated during this short period since the start of the pandemic. For example, just by changing the therapeutic molecule encapsulated inside natural or synthetic nanoparticles different examples of COVID-19 vaccine have been developed. As of 29th of October 2020, there are already 13 vaccine candidates in clinical trials that are based on nanotechnology, many are in Phase III and already showing promising results. This is indeed not the case for small drug molecules that must be fully modified and undergo retesting for each new application. Despite such clear differences, specific regulations for nanomedicine approval is still lacking and any new nanomedicine product still has to go through the full clinical approval process (Germain et al., 2020). A recent comparison of the approval rates of nanomedicine products compared to small drug molecules has shown that nanomedicine outperformed traditional drugs 4 times in oncology. Not many differences have been observed in other medical conditions which have been an area of debate recently, questioning whether nanomedicines are still delivering to their potential and whether they have any applicability outside oncology (Park, 2019). We believe that during the coronavirus pandemic the nanomedicine field has shined building up on excising platforms and knowledge and provided innovative excellent therapeutic modalities for COVID-19. Therefore, there is a need to build on existing power and enable the clinical development of nanoproducts to solve unmet clinical needs outside oncology. It is also crucial to address the gaps facing nanomedicine development such as; clinical and business engagement, nanotoxicological aspect of new classes of nanomaterials, mass production to GMP standards and global clarification on regulatory approval both on the physicochemical characterization and biological activity particularly when it comes to multifunctional products. In addition the design of nanotoxicological studies should be expanded to include relevant models (including co-morbidities) and exploit modern tools to understand nanomaterials toxicity. History had shown that crises can create new potential for innovative technology, and this pandemic could be the time to re-shape the future of the nanomedicine and nanotoxicology fields.
ZA-A and HA-B contributed equally to the design and the writing of this review. All authors contributed to the article and approved the submitted version.
The authors declare that the research was conducted in the absence of any commercial or financial relationships that could be construed as a potential conflict of interest.
Adams, D., Gonzalez-Duarte, A., O'Riordan, W. D., Yang, C. C., Ueda, M., Kristen, A. V., et al. (2018). Patisiran, an RNAi therapeutic, for hereditary transthyretin amyloidosis. N. Engl. J. Med. 379, 11–21. doi: 10.1056/NEJMoa1716153
Afsahi, S., Lerner, M. B., Goldstein, J. M., Lee, J., Tang, X., Bagarozzi, D. A., et al. (2018). Novel graphene-based biosensor for early detection of Zika virus infection. Biosens. Bioelectron. 100, 85–88. doi: 10.1016/j.bios.2017.08.051
Ahuja, A. S., Reddy, V. P., and Marques, O. (2020). Artificial intelligence and COVID-19: a multidisciplinary approach. Integr. Med. Res. 9:100434. doi: 10.1016/j.imr.2020.100434
Al-Ahmady, Z. S., Jasim, D., Ahmad, S. S., Wong, R., Haley, M., Coutts, G., et al. (2019). Selective liposomal transport through blood brain barrier disruption in ischemic stroke reveals two distinct therapeutic opportunities. ACS Nano 13, 12470–12486. doi: 10.1021/acsnano.9b01808
Ali-Boucetta, H., Al-Jamal, K. T., Müller, K. H., Li, S., Porter, A. E., Eddaoudi, A., et al. (2011). Cellular uptake and cytotoxic impact of chemically functionalized and polymer-coated carbon nanotubes. Small 7, 3230–3238. doi: 10.1002/smll.201101004
Allison, S. J. (2020). SARS-CoV-2 infection of kidney organoids prevented with soluble human ACE2. Nat. Rev. Nephrol. 16:316. doi: 10.1038/s41581-020-0291-8
Al-Samkari, H., Karp Leaf, R. S., Dzik, W. H., Carlson, J. C. T., Fogerty, A. E., Waheed, A., et al. (2020). COVID-19 and coagulation: bleeding and thrombotic manifestations of SARS-CoV-2 infection. Blood 136, 489–500. doi: 10.1182/blood.2020006520
Aulicino, F., Capin, J., and Berger, I. (2020). Synthetic virus-derived nanosystems (SVNs) for delivery and precision docking of large multifunctional DNA circuitry in mammalian cells. Pharmaceutics 12:759. doi: 10.3390/pharmaceutics12080759
Baloch, S., Baloch, M. A., Zheng, T., and Pei, X. (2020). The coronavirus disease 2019 (COVID-19) pandemic. Tohoku J. Exp. Med. 250, 271–278. doi: 10.1620/tjem.250.271
Balwani, M., Sardh, E., Ventura, P., Peir,ó, P. A., Rees, D. C., Stölzel, U., et al. (2020). Phase 3 trial of RNAi therapeutic givosiran for acute intermittent porphyria. N. Engl. J. Med. 382, 2289–2301. doi: 10.1056/NEJMoa1913147
Banciu, M., Metselaar, J. M., Schiffelers, R. M., and Storm, G. (2008). Liposomal glucocorticoids as tumor-targeted anti-angiogenic nanomedicine in B16 melanoma-bearing mice. J. Steroid Biochem. Mol. Biol. 111, 101–110. doi: 10.1016/j.jsbmb.2008.05.004
Bartneck, M., Scheyda, K. M., Warzecha, K. T., Rizzo, L. Y., Hittatiya, K., Luedde, T., et al. (2015). Fluorescent cell-traceable dexamethasone-loaded liposomes for the treatment of inflammatory liver diseases. Biomaterials 37, 367–382. doi: 10.1016/j.biomaterials.2014.10.030
Beigel, J. H., Tomashek, K. M., Dodd, L. E., Mehta, A. K., Zingman, B. S., Kalil, A. C., et al. (2020). Remdesivir for the treatment of covid-19 — preliminary report. N. Engl. J. Med. 383, 993–994. doi: 10.1056/NEJMoa2007764
Boopathi, S., Poma, A. B., and Kolandaivel, P. (2020). Novel 2019 coronavirus structure, mechanism of action, antiviral drug promises and rule out against its treatment. J. Biomol. Struct. Dyn. 1–14. doi: 10.1080/07391102.2020.1758788
Brahim Belhaouari, D., Fontanini, A., Baudoin, J.-P., Haddad, G., Le Bideau, M., Bou Khalil, J. Y., et al. (2020). The strengths of scanning electron microscopy in deciphering SARS-CoV-2 infectious cycle. Front. Microbiol. 11:2014. doi: 10.3389/fmicb.2020.02014
Campochiaro, C., and Dagna, L. (2020). The conundrum of interleukin-6 blockade in COVID-19. Lancet Rheumatol. 2, e579–e580. doi: 10.1016/S2665-9913(20)30287-3
Cantuti-Castelvetri, L., Ojha, R., Pedro, L., Djannatian, M., Franz, J., Kuivanen, S., et al. (2020). Neuropilin-1 facilitates SARS-CoV-2 cell entry and provides a possible pathway into the central nervous system. bioRxiv 370, 856–860. doi: 10.1101/2020.06.07.137802
Chauhan, G., Madou, M. J., Kalra, S., Chopra, V., Ghosh, D., and Martinez-Chapa, S. O. (2020). Nanotechnology for COVID-19: therapeutics and vaccine research. ACS Nano 14, 7760–7782. doi: 10.1021/acsnano.0c04006
Clevers, H. (2020). COVID-19: organoids go viral. Nat. Rev. Mol. Cell Biol. 21, 355–356. doi: 10.1038/s41580-020-0258-4
Conniot, J., Scomparin, A., Peres, C., Yeini, E., Pozzi, S., Matos, A. I., et al. (2019). Immunization with mannosylated nanovaccines and inhibition of the immune-suppressing microenvironment sensitizes melanoma to immune checkpoint modulators. Nat. Nanotechnol. 14, 891–901. doi: 10.1038/s41565-019-0512-0
Contini, C., Hindley, J. W., Macdonald, T. J., Barritt, J. D., Ces, O., and Quirke, N. (2020). Size dependency of gold nanoparticles interacting with model membranes. Commun. Chem. 3130. doi: 10.1038/s42004-020-00377-y
Crackower, M. A., Sarao, R., Oliveira-dos-Santos, A. J., Da Costa, J., and Zhang, L. (2002). Angiotensin-converting enzyme 2 is an essential regulator of heart function. Nature 417, 822–828. doi: 10.1038/nature00786
de Lázaro, I., and Mooney, D. J. (2020). A nanoparticle's pathway into tumours. Nat. Mater. 19, 486–487. doi: 10.1038/s41563-020-0669-9
Dickson, I. (2020). Organoids demonstrate gut infection by SARS-CoV-2. Nat. Rev. Gastroenterol. Hepatol. 17:383. doi: 10.1038/s41575-020-0317-5
Elbadawi, M., and Efferth, T. (2020). Organoids of human airways to study infectivity and cytopathy of SARS-CoV-2. Lancet Respir. Med. 8, e55–e56. doi: 10.1016/S2213-2600(20)30238-1
Fan, C., Li, K., Ding, Y., Lu, W. L., and Wang, J. (2020). ACE2 expression in kidney and testis may cause kidney and testis damage after 2019-nCoV infection. medRxiv 30, 118–120. doi: 10.1101/2020.02.12.20022418
Fanelli, V., Fiorentino, M., Cantaluppi, V., Gesualdo, L., Stallone, G., Ronco, C., et al. (2020). Acute kidney injury in SARS-CoV-2 infected patients. Crit. Care 24:155. doi: 10.1186/s13054-020-02872-z
Florindo, H. F., Kleiner, R., Vaskovich-Koubi, D., Acúrcio, R. C., Carreira, B., Yeini, E., et al. (2020). Immune-mediated approaches against COVID-19. Nat. Nanotechnol. 15, 630–645. doi: 10.1038/s41565-020-0732-3
Gauthier, A., Fisch, A., Seuwen, K., Baumgarten, B., Ruffner, H., Aebi, A., et al. (2018). Glucocorticoid-loaded liposomes induce a pro-resolution phenotype in human primary macrophages to support chronic wound healing. Biomaterials 178, 481–495. doi: 10.1016/j.biomaterials.2018.04.006
Geall, A. J., Verma, A., Otten, G. R., Shaw, C. A., Hekele, A., Banerjee, K., et al. (2012). Nonviral delivery of self-amplifying RNA vaccines. Proc. Natl. Acad. Sci. U. S. A. 109, 14604–14609. doi: 10.1073/pnas.1209367109
George, P. M., Barratt, S. L., Condliffe, R., Desai, S. R., Devaraj, A., Forrest, I., et al. (2020). Respiratory follow-up of patients with COVID-19 pneumonia. Thorax, 1–8. doi: 10.1136/thoraxjnl-2020-215314
Germain, M., Caputo, F., Metcalfe, S., Tosi, G., Spring, K., Åslund, A. K. O., et al. (2020). Delivering the power of nanomedicine to patients today. J. Control. Release 326, 164–171. doi: 10.1016/j.jconrel.2020.07.007
GISAID Next hCoV-9 App (2020). Available online at: https://www.gisaid.org/epiflu-applications/next-hcov-19-app/ (accessed August 25, 2020).
Grifoni, A., Weiskopf, D., Ramirez, S. I., Mateus, J., Dan, J. M., Moderbacher, C. R., et al. (2020). Targets of T cell responses to SARS-CoV-2 coronavirus in humans with COVID-19 disease and unexposed individuals. Cell 181, 1489–1501.e15. doi: 10.1016/j.cell.2020.05.015
Gupta, A., Madhavan, M. V., Sehgal, K., Nair, N., Mahajan, S., Sehrawat, T. S., et al. (2020). Extrapulmonary manifestations of COVID-19. Nat. Med. 26, 1017–1032. doi: 10.1038/s41591-020-0968-3
Hamming, I., Timens, W., Bulthuis, M. L. C., Lely, A. T., Navis, G. J., and van Goor, H. (2004). Tissue distribution of ACE2 protein, the functional receptor for SARS coronavirus. A first step in understanding SARS pathogenesis. J. Pathol. 203, 631–637. doi: 10.1002/path.1570
Hashemzadeh, A., Avan, A., Ferns, G. A., and Khazaei, M. (2020). Vaccines based on virus-like nano-particles for use against middle east respiratory syndrome (MERS) coronavirus. Vaccine 38, 5742–5746. doi: 10.1016/j.vaccine.2020.07.003
Hoffmann, M., Kleine-Weber, H., Schroeder, S., Mü, M. A., Drosten, C., and Pöhlmann, S. (2020). SARS-CoV-2 cell entry depends on ACE2 and TMPRSS2 and is blocked by a clinically proven protease inhibitor. Cell 181, 271–280. doi: 10.1016/j.cell.2020.02.052
Iavarone, C., O'hagan, D. T., Yu, D., Delahaye, N. F., and Ulmer, J. B. (2017). Mechanism of action of mRNA-based vaccines. Expert Rev. Vaccines 16, 871–881. doi: 10.1080/14760584.2017.1355245
Imai, M., Iwatsuki-Horimoto, K., Hatta, M., Loeber, S., Halfmann, P. J., Nakajima, N., et al. (2020). Syrian hamsters as a small animal model for SARS-CoV-2 infection and countermeasure development. Proc. Natl. Acad. Sci. U. S. A. 117, 16587–16595. doi: 10.1073/pnas.2009799117
ISRCTN17072692 (2020). ISRCTN - ISRCTN17072692: Clinical Trial to Assess the Safety of a Coronavirus Vaccine in Healthy Men and Women. Available online at: http://www.isrctn.com/ISRCTN17072692 (accessed August 28, 2020).
Jeevanandam, J., Pal, K., and Danquah, M. K. (2019). Virus-like nanoparticles as a novel delivery tool in gene therapy. Biochimie 157, 38–47. doi: 10.1016/j.biochi.2018.11.001
Johns, C., Gavras, I., Handy, D. E., Salomao, A., and Gavras, H. (1996). Models of experimental hypertension in mice. Hypertension 28, 1064–1069. doi: 10.1161/01.HYP.28.6.1064
Kan, H., Pan, D., and Castranova, V. (2018). Engineered nanoparticle exposure and cardiovascular effects: the role of a neuronal-regulated pathway. Inhal. Toxicol. 30, 335–342. doi: 10.1080/08958378.2018.1535634
Kander, T. (2020). Coagulation disorder in COVID-19. Lancet Haematol. 7, e630–e632. doi: 10.1016/S2352-3026(20)30218-0
Kaushik, A. (2019). Biomedical nanotechnology related grand challenges and perspectives. Front. Nanotechnol. 1:1. doi: 10.3389/fnano.2019.00001
King, A. J. F. (2012). The use of animal models in diabetes research. Br. J. Pharmacol. 166, 877–894. doi: 10.1111/j.1476-5381.2012.01911.x
Lammers, T., Sofias, A. M., van der Meel, R., Schiffelers, R., Storm, G., Tacke, F., et al. (2020). Dexamethasone nanomedicines for COVID-19. Nat. Nanotechnol. 15, 622–624. doi: 10.1038/s41565-020-0752-z
Ledford, H. (2020). Coronavirus breakthrough: dexamethasone is first drug shown to save lives. Nature 582, 469–469. doi: 10.1038/d41586-020-01824-5
Legramante, J. M., Valentini, F., Magrini, A., Palleschi, G., Sacco, S., Iavicoli, I., et al. (2009). Cardiac autonomic regulation after lung exposure to carbon nanotubes. Hum. Exp. Toxicol. 28, 369–375. doi: 10.1177/0960327109105150
Li, J. H., Santos-Otte, P., Au, B., Rentsch, J., Block, S., and Ewers, H. (2020). Directed manipulation of membrane proteins by fluorescent magnetic nanoparticles. Nat. Commun. 11:4259. doi: 10.1016/j.bpj.2019.11.1764
Lopez, C. F., Nielsen, S. O., Moore, P. B., and Klein, M. L. (2004). Understanding nature's design for a nanosyringe. Proc. Natl. Acad. Sci. U. S. A. 101, 4431–4434. doi: 10.1073/pnas.0400352101
Lu, Y., Li, X., Geng, D., Mei, N., Wu, P. Y., Huang, C. C., et al. (2020). Cerebral micro-structural changes in COVID-19 patients – an MRI-based 3-month follow-up study: a brief title: cerebral changes in COVID-19. EClinicalMedicine 25:100484. doi: 10.1016/j.eclinm.2020.100484
Luo, F., Liao, F. L., Wang, H., Tang Bin, H., Yang, Z. Q., and Hou, W. (2018). Evaluation of antibody-dependent enhancement of SARS-CoV infection in rhesus macaques immunized with an inactivated SARS-CoV vaccine. Virol. Sin. 33, 201–204. doi: 10.1007/s12250-018-0009-2
Mann, E. E., Thompson, L. C., Shannahan, J. H., and Wingard, C. J. (2012). Changes in cardiopulmonary function induced by nanoparticles. Wiley Interdiscip. Rev. Nanomed. Nanobiotechnol. 4, 691–702. doi: 10.1002/wnan.1194
Merad, M., and Martin, J. C. (2020). Pathological inflammation in patients with COVID-19: a key role for monocytes and macrophages. Nat. Rev. Immunol. 20, 355–362. doi: 10.1038/s41577-020-0331-4
Metselaar, J. M., Wauben, M. H. M., Wagenaar-Hilbers, J. P. A., Boerman, O. C., and Storm, G. (2003). Complete remission of experimental arthritis by joint targeting of glucocorticoids with long-circulating liposomes. Arthritis Rheum. 48, 2059–2066. doi: 10.1002/art.11140
Moitra, P., Alafeef, M., Alafeef, M., Alafeef, M., Dighe, K., Frieman, M. B., et al. (2020). Selective naked-eye detection of SARS-CoV-2 mediated by N gene targeted antisense oligonucleotide capped plasmonic nanoparticles. ACS Nano 14, 7617–7627. doi: 10.1021/acsnano.0c03822
Monzel, C., Vicario, C., Piehler, J., Coppey, M., and Dahan, M. (2017). Magnetic control of cellular processes using biofunctional nanoparticles. Chem. Sci. 8, 7330–7338. doi: 10.1039/C7SC01462G
Moutal, A., Martin, L., Boinon, L., Gomez, K., Ran, D., Zhou, Y., et al. (2020). SARS-CoV-2 Spike protein hijacks VEGF-A/Neuropilin-1 receptor signaling to induce analgesia. bioRxiv doi: 10.1097/j.pain.0000000000002097
Mujawar, M. A., Gohel, H., Bhardwaj, S. K., Srinivasan, S., Hickman, N., and Kaushik, A. (2020). Nano-enabled biosensing systems for intelligent healthcare: towards COVID-19 management. Mater. Today Chem. 17:100306. doi: 10.1016/j.mtchem.2020.100306
Mullard, A. (2020). COVID-19 vaccine development pipeline gears up. Lancet 395, 1751–1752. doi: 10.1016/S0140-6736(20)31252-6
NCT03033316 (2017). A Evaluation of the Safety of Oncocort IV Pegylated Liposomal Dexamethasone Phosphate in Patients With Progressive Multiple Myeloma - Full Text View - ClinicalTrials.gov. Available online at: https://clinicaltrials.gov/ct2/show/NCT03033316 (accessed August 26, 2020).
NCT04283461 (2020). Safety and Immunogenicity Study of 2019-nCoV Vaccine (mRNA-1273) for Prophylaxis of SARS-CoV-2 Infection (COVID-19) - Full Text View - ClinicalTrials.gov. Available online at: https://clinicaltrials.gov/ct2/show/NCT04283461?term=vaccine&cond=covid-19&draw=2 (accessed August 27, 2020).
NCT04336410 (2020). Safety, Tolerability and Immunogenicity of INO-4800 for COVID-19 in Healthy Volunteers - Full Text View - ClinicalTrials.gov. Available online at: https://clinicaltrials.gov/ct2/show/NCT04336410?term=inovio&cond=covid-19&draw=2 (accessed August 28, 2020).
NCT04368728 (2020). Study to Describe the Safety, Tolerability, Immunogenicity, and Efficacy of RNA Vaccine Candidates Against COVID-19 in Healthy Adults - Full Text View - ClinicalTrials.gov. Available online at: https://clinicaltrials.gov/ct2/show/NCT04368728?term=vaccine&cond=covid-19&draw=3 (accessed August 27, 2020).
NCT04381936 (2020). Randomised Evaluation of COVID-19 Therapy (RECOVERY). Available online at: https://clinicaltrials.gov/ct2/show/NCT04381936 (accessed September 25, 2020).
NCT04443881 (2020). Clinical Trial of the Use of Anakinra in Cytokine Storm Syndrome Secondary to Covid-19 (ANA-COVID-GEAS) - Full Text View - ClinicalTrials.gov. Available at: https://clinicaltrials.gov/ct2/show/NCT04443881?term=IL-1&cond=COVID&draw=2&rank=2 (accessed August 27, 2020).
NCT04447781 (2020). Safety, Tolerability and Immunogenicity of INO-4800 Followed by Electroporation in Healthy Volunteers for COVID19 - Full Text View - ClinicalTrials.gov. Available online at: https://clinicaltrials.gov/ct2/show/NCT04447781 (accessed August 27, 2020).
NCT04456595 (2020). NCT04. Available online at: https://clinicaltrials.gov/ct2/show/NCT04456595?term=vaccine&cond=covid-19&draw=2 (accessed August 27, 2020).
NCT04466085 (2020). Clinical Study of Recombinant Novel Coronavirus Vaccine - Full Text View - ClinicalTrials.gov. Available online at: https://clinicaltrials.gov/ct2/show/NCT04466085?term=NCT04466085&draw=2&rank=1 (accessed August 27, 2020).
NCT04470427 (2020). A Study to Evaluate Efficacy, Safety, and Immunogenicity of mRNA-1273 Vaccine in Adults Aged 18 Years and Older to Prevent COVID-19 - No Study Results Posted - ClinicalTrials.gov. Available online at: https://clinicaltrials.gov/ct2/show/results/NCT04470427?term=vaccine&cond=covid-19&draw=5 (accessed August 27, 2020).
NCT04480957 (2020). Ascending Dose Study of Investigational SARS-CoV-2 Vaccine ARCT-021 in Healthy Adult Subjects - Full Text View - ClinicalTrials.gov. Available online at: https://clinicaltrials.gov/ct2/show/NCT04480957?term=vaccine&cond=covid-19&draw=10 (accessed August 28, 2020).
NCT04516746 (2020). Phase III Double-blind, Placebo-controlled Study of AZD1222 for the Prevention of COVID-19 in Adults - Full Text View - ClinicalTrials.gov. Available online at: https://clinicaltrials.gov/ct2/show/NCT04516746?term=astrazeneca&cond=covid-19&draw=2&rank=1 (accessed August 27, 2020).
Neagu, M., Piperigkou, Z., Karamanou, K., Engin, A. B., Docea, A. O., Constantin, C., et al. (2017). Protein bio-corona: critical issue in immune nanotoxicology. Arch. Toxicol. 91, 1031–1048. doi: 10.1007/s00204-016-1797-5
Ng, S. C., and Tilg, H. (2020). COVID-19 and the gastrointestinal tract: more than meets the eye. Gut 69, 973–974. doi: 10.1136/gutjnl-2020-321195
Offringa, A., Montijn, R., Singh, S., Paul, M., Pinto, Y. M., and Pinto-Sietsma, S.-J. (2020). The mechanistic overview of SARS-CoV-2 using angiotensin-converting enzyme 2 to enter the cell for replication: possible treatment options related to the renin–angiotensin system. Eur. Hear. J. Cardiovasc. Pharmacother. 6, 317–325. doi: 10.1093/ehjcvp/pvaa053
Pardi, N., Hogan, M. J., Porter, F. W., and Weissman, D. (2018). mRNA vaccines-a new era in vaccinology. Nat. Rev. Drug Discov. 17, 261–279. doi: 10.1038/nrd.2017.243
Park, K. (2019). The beginning of the end of the nanomedicine hype. J. Control Release 305, 221–222. doi: 10.1016/j.jconrel.2019.05.044
Pharmaceuticals, E. (2020). Entos Pharmaceuticals Announces Selection of Lead DNA Vaccine Candidates for COVID-19 and a $4.2M Award to Move Forward with Phase I/II Human Trials | Business Wire. Available at: https://www.businesswire.com/news/home/20200625005802/en/Entos-Pharmaceuticals-Announces-Selection-Lead-DNA-Vaccine (accessed August 28, 2020).
Quan, L., Zhang, Y., Crielaard, B. J., Dusad, A., Lele, S. M., Rijcken, C. J. F., et al. (2014). Nanomedicines for inflammatory arthritis: head-to-head comparison of glucocorticoid-containing polymers, micelles, and liposomes. ACS Nano 8, 458–466. doi: 10.1021/nn4048205
Rao, K., Verma, P., Kumar, K., Verma, M. K., Siddiqui, A. H., Singh, S., et al. (2015). Review on newly identified coronavirus and its genomic organization. SSR Int. J. Life Sci. 2509–2519.
Reina, G., Peng, S., Jacquemin, L., Andrade, A. F., and Bianco, A. (2020). Hard nanomaterials in time of viral pandemics. ACS Nano. 15:acsnano.0c04117. doi: 10.1021/acsnano.0c04117
Samidurai, A., and Das, A. (2020). Cardiovascular complications associated with COVID-19 and potential therapeutic strategies. Int. J. Mol. Sci. 21, 1–28. doi: 10.3390/ijms21186790
Shaker, N., Abou-Zleikha, M., AlAmri, M., and Mehellou, Y. (2020). A Generative deep learning approach for the discovery of SARS CoV2 protease inhibitors. ChemRxiv doi: 10.26434/chemrxiv.12170337
Shin, M. D., and Shukla, S. (2020). COVID-19 vaccine development and a potential nanomaterial path forward. Nat. Nanotechnol. 15, 646–655.
Shin, M. D., Shukla, S., Chung, Y. H., Beiss, V., Chan, S. K., Ortega-Rivera, O. A., et al. (2020). COVID-19 vaccine development and a potential nanomaterial path forward. Nat. Nanotechnol. 15, 646–655. doi: 10.1038/s41565-020-0737-y
Shvedova, A. A., Kisin, E. R., Mercer, R., Murray, A. R., Johnson, V. J., Potapovich, A. I., et al. (2005). Unusual inflammatory and fibrogenic pulmonary responses to single-walled carbon nanotubes in mice. Am. J. Physiol. Lung Cell. Mol. Physiol. 289, 698–708. doi: 10.1152/ajplung.00084.2005
Sindhwani, S., Syed, A. M., Ngai, J., Kingston, B. R., Maiorino, L., Rothschild, J., et al. (2020). The entry of nanoparticles into solid tumours. Nat. Mater. 19, 566–575. doi: 10.1038/s41563-019-0566-2
Singh, A., Singh, R. S., Sarma, P., Batra, G., Joshi, R., Kaur, H., et al. (2020). A comprehensive review of animal models for coronaviruses: SARS-CoV-2, SARS-CoV, and MERS-CoV. Virol. Sin. 35, 290–304. doi: 10.1007/s12250-020-00252-z
Smith, T. R. F., Patel, A., Ramos, S., Elwood, D., Zhu, X., Yan, J., et al. (2020). Immunogenicity of a DNA vaccine candidate for COVID-19. Nat. Commun. 11:2601. doi: 10.1038/s41467-020-16505-0
Soler, M. J., Wysocki, J., and Batlle, D. (2013). ACE2 alterations in kidney disease. Nephrol. Dial. Transplant. 28, 2687–2697. doi: 10.1093/ndt/gft320
Song, J., Mauk, M. G., Hackett, B. A., Cherry, S., Bau, H. H., and Liu, C. (2016). Instrument-free point-of-care molecular detection of Zika virus. Anal. Chem. 88, 7289–7294. doi: 10.1021/acs.analchem.6b01632
Sportelli, M. C., Izzi, M., Kukushkina, E. A., Hossain, S. I., Picca, R. A., Ditaranto, N., et al. (2020). Can nanotechnology and materials science help the fight against sars-cov-2? Nanomaterials 10:802. doi: 10.3390/nano10040802
Stueckle, T. A., and Roberts, J. R. (2019). Perspective on current alternatives in nanotoxicology research. Appl. Vitr. Toxicol. 5, 111–113. doi: 10.1089/aivt.2019.29020.jrr
Talebian, S., Wallace, G. G., Schroeder, A., Stellacci, F., and Conde, J. (2020). Nanotechnology-based disinfectants and sensors for SARS-CoV-2. Nat. Nanotechnol. 15, 618–621. doi: 10.1038/s41565-020-0751-0
Tay, M. Z., Poh, C. M., Rénia, L., MacAry, P. A., and Ng, L. F. P. (2020). The trinity of COVID-19: immunity, inflammation and intervention. Nat. Rev. Immunol. 20, 363–374. doi: 10.1038/s41577-020-0311-8
Toelzer, C., Gupta, K., Yadav, S. K. N., Borucu, U., Davidson, A. D., Kavanagh Williamson, M., et al. (2020). Free fatty acid binding pocket in the locked structure of SARS-CoV-2 spike protein. Science 370, 725–730. doi: 10.1126/science.abd3255
Tsang, M. K., Ye, W., Wang, G., Li, J., Yang, M., and Hao, J. (2016). Ultrasensitive detection of ebola virus oligonucleotide based on upconversion nanoprobe/nanoporous membrane system. ACS Nano 10, 598–605. doi: 10.1021/acsnano.5b05622
Varga, Z., Flammer, A. J., Steiger, P., Haberecker, M., Andermatt, R., Zinkernagel, A. S., et al. (2020). Endothelial cell infection and endotheliitis in COVID-19. Lancet 395, 1417–1418. doi: 10.1016/S0140-6736(20)30937-5
Villapol, S. (2020). Gastrointestinal symptoms associated with COVID-19: impact on the gut microbiome. Transl. Res. 226, 57–69. doi: 10.1016/j.trsl.2020.08.004
Weiss, C., Carriere, M., Fusco, L., Fusco, L., Capua, I., Regla-Nava, J. A., et al. (2020). Toward nanotechnology-enabled approaches against the COVID-19 pandemic. ACS Nano 14, 6383–6406. doi: 10.1021/acsnano.0c03697
WHO (2020b). Draft Landscape of COVID-19 Candidate Vaccines. Available online at: https://www.who.int/publications/m/item/draft-landscape-of-covid-19-candidate-vaccines (accessed October 29, 2020).
WHO (2020c). Draft Landscape of COVID-19 Candidate Vaccines. Available online at: https://www.who.int/publications/m/item/draft-landscape-of-covid-19-candidate-vaccines (accessed September 25, 2020).
WHO (2020d). Weekly Epidemiological Update - 27 October 2020. Available online at: https://www.who.int/publications/m/item/weekly-epidemiological-update-−27-october-2020 (accessed October 29, 2020).
Xu, H., Zhong, L., Deng, J., Peng, J., Dan, H., Zeng, X., et al. (2020). High expression of ACE2 receptor of 2019-nCoV on the epithelial cells of oral mucosa. Int. J. Oral Sci. 12, 1–5. doi: 10.1038/s41368-020-0074-x
Yang, L., Han, Y., Nilsson-Payant, B. E., Gupta, V., Wang, P., Duan, X., et al. (2020). A human pluripotent stem cell-based platform to study SARS-CoV-2 tropism and model virus infection in human cells and organoids. Cell Stem Cell 27, 125–136.e7. doi: 10.1016/j.stem.2020.06.015
Zhang, J., Leifer, F., Rose, S., Chun, D. Y., Thaisz, J., Herr, T., et al. (2018). Amikacin liposome inhalation suspension (ALIS) penetrates non-tuberculous mycobacterial biofilms and enhances amikacin uptake into macrophages. Front. Microbiol. 9:915. doi: 10.3389/fmicb.2018.00915
Zhang, Q., Honko, A., Zhou, J., Gong, H., Downs, S. N., Vasquez, J. H., et al. (2020). Cellular nanosponges inhibit SARS-CoV-2 infectivity. Nano Lett. 20, 5570–5574. doi: 10.1021/acs.nanolett.0c02278
Keywords: COVID-19, nanotechnology, nanomedicine, drug delivery, nanotoxicology, nanovaccines, SARS-CoV-2, nanoparticles
Citation: Al-Ahmady ZS and Ali-Boucetta H (2020) Nanomedicine & Nanotoxicology Future Could Be Reshaped Post-COVID-19 Pandemic. Front. Nanotechnol. 2:610465. doi: 10.3389/fnano.2020.610465
Received: 25 September 2020; Accepted: 13 November 2020;
Published: 09 December 2020.
Edited by:
Ajeet Kaushik, Florida Polytechnic University, United StatesReviewed by:
Sneham Tiwari, Florida International University, United StatesCopyright © 2020 Al-Ahmady and Ali-Boucetta. This is an open-access article distributed under the terms of the Creative Commons Attribution License (CC BY). The use, distribution or reproduction in other forums is permitted, provided the original author(s) and the copyright owner(s) are credited and that the original publication in this journal is cited, in accordance with accepted academic practice. No use, distribution or reproduction is permitted which does not comply with these terms.
*Correspondence: Zahraa S. Al-Ahmady, emFocmFhLmFsLWFobWFkeUBudHUuYWMudWs=; Hanene Ali-Boucetta, aC5hbGlib3VjZXR0YUBiaGFtLmFjLnVr
Disclaimer: All claims expressed in this article are solely those of the authors and do not necessarily represent those of their affiliated organizations, or those of the publisher, the editors and the reviewers. Any product that may be evaluated in this article or claim that may be made by its manufacturer is not guaranteed or endorsed by the publisher.
Research integrity at Frontiers
Learn more about the work of our research integrity team to safeguard the quality of each article we publish.