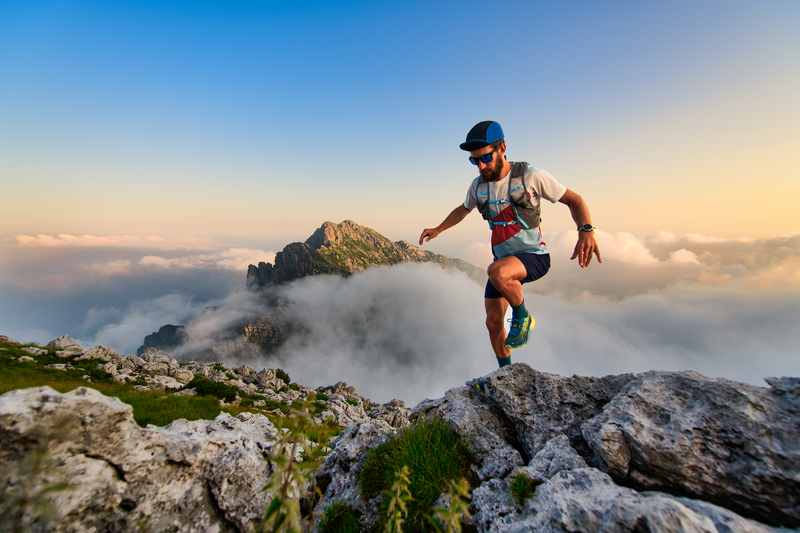
95% of researchers rate our articles as excellent or good
Learn more about the work of our research integrity team to safeguard the quality of each article we publish.
Find out more
ORIGINAL RESEARCH article
Front. Mol. Neurosci. , 18 March 2025
Sec. Methods and Model Organisms
Volume 18 - 2025 | https://doi.org/10.3389/fnmol.2025.1553438
Background/objectives: Autism spectrum disorder (ASD) is associated with excitatory-inhibitory imbalance and oxidative stress. GABA, an inhibitory neurotransmitter, and related nutritional therapies are promising in restoring these imbalances. GABAergic deficits and glutamate excitotoxicity are two essential signaling pathways that could be addressed to treat autism, thus medications targeting these pathways are critical for treating behavioral symptoms. In a rat model of autism produced by propionic acid (PPA), this study assessed the effects of GABA supplementation and combined nutritional therapy (probiotics, vitamin D3) and β-lactam as an activator of glutamate transporter.
Methods: Sixty rats were randomly assigned into six groups: Group I (Control), Group II (PPA-treated), Group III (Control-GABA), Group IV (Control-Combination), Group V (PPA-GABA), and Group VI (PPA-Combination). Social behavior was evaluated using the three-chamber test. Selected biochemical variables related to oxidative stress (GST, Catalase, Lipid peroxides, GSH and Vitamin C), GABA and glutamate signaling (EAAT2, KCC2, NKCC1, GABA, VD3, Glutamate and GABRA5) were measured in the brain homogenates of the six groups. The hippocampus was examined histopathologically to assess cellular integrity.
Results: The obtained data revealed that PPA treatment caused significant oxidative stress and neurotransmitter imbalances, characterized by reduced GABA and elevated glutamate levels. GABA supplementation alone produced moderate benefits in biochemical and behavioral markers, but combined therapy considerably restored GABA levels, reduced oxidative stress, and enhanced social interaction behaviors. Histopathology revealed that combination therapy mitigated neurodegenerative changes induced by PPA, preserving hippocampal cellular structure.
Conclusion: This study demonstrated that combined therapy (GABA, probiotics, vitamin D3, and β-lactam) were more effective than GABA alone in enhancing neurochemical balance and lowering oxidative stress in a PPA-induced mouse model of autism, indicating promise for treating symptoms.
Autism Spectrum Disorder (ASD) is a complex neurodevelopmental condition characterized by deficits in social communication and the presence of restrictive and repetitive behaviors (American Psychiatric Association, 2013). While the exact cause of ASD remains elusive, it is widely accepted that both genetic and environmental factors contribute to its pathogenesis (Emberti Gialloreti et al., 2019). Globally, ASD affects approximately 1 in 100 children (World Health Organization, 2021), with a prevalence of 2.51% children, with a male to female ratio of 3:1in Riyadh, Saudi Arabia (AlBatti et al., 2022).
Although ASD is considered a common disorder, the exact etiology remains unknown (Sauer et al., 2021). Moreover, the current therapeutic approaches are severely constrained by a lack of understanding of the etiology and the correlations between the environment and behaviors. Animal models are crucial for the identification of the etiology of ASD and the development of treatment strategies, as it is virtually impossible to obtain brain tissue from living humans in daily practice and research. There have been numerous environmental agents that have been employed to develop animal models of ASDs (Lawler et al., 2004). Propionic acid (PPA) is a prospective agent that induces various behavioral and neuro-inflammatory alterations in the rat model of autism. PPA is a metabolic by product of intestinal bacteria and possesses the capability to cross the gut–blood and gut–brain barriers. Following transmission, PPA accumulates within the cells, resulting in intracellular acidification (Bonnet et al., 2000; El-Ansary et al., 2012). Thus, it has the potential to influence the normal functioning of a number of neurotransmitters (El-Ansary et al., 2012). At present, PPA is regarded as a well-established agent for the development of an experimental model of ASD (Sever et al., 2022; Sahin et al., 2022; Abujamel et al., 2022; Khera et al., 2022; Abuaish et al., 2022; Sharma et al., 2022).
The imbalance between neurotransmitters with excitatory and inhibitory properties is recognized as an etiological factor for ASD (Canitano and Palumbi, 2021). Gamma-aminobutyric acid (GABA) is a critical inhibitory neurotransmitter in the central nervous system (CNS). The inhibitory effect of GABAergic neurotransmission is important for the regulation of neurotransmission and the development of the brain. GABA has garnered significant attention in the investigation of the etiologies of a variety of neurological disorders, such as schizophrenia, depression, and anxiety (Amin et al., 2006; Möhler, 2012). Additionally, experimental studies have shown that core symptoms of ASD were substantially associated with dysfunction in the GABAergic signaling pathway (Cellot and Cherubini, 2014). The accumulating evidence indicated that the Na+–K+–2Cl− cotransporter 1 (NKCC1) and K+–Cl− cotransporter 2 (KCC2) were significantly correlated with GABAergic signaling (Kaila et al., 2014). The chloride importer NKCC1 and the chloride exporter KCC2 are critical components of GABA receptors (Lam et al., 2023).
Probiotics have the potential to reduce the symptoms of ASD by modulating neuroactive compounds. Additionally, emotional behavior and central GABA receptor expression were regulated by Lactobacillus treatment in a mouse through the vagus nerve, which serves as a communication pathway between the brain and the gut (Bravo et al., 2011). Probiotics may enhance inhibitory neurotransmission by upregulating GABA production or the expression of GABA receptor genes (El-Ansary et al., 2018; Bin-Khattaf et al., 2024). This may help to improve the social interaction deficits associated with ASD and restore the excitatory/inhibitory (E/I) balance.
Glutamate is an excitatory neurotransmitter in the brain. It is released from cells into the extracellular fluid and subsequently removed by glutamate transporters. Excitatory synaptic transmissions are regulated by this transportation. In autism, glutamate level is elevated, while glutamine levels are reduced (Ghanizadeh, 2013). One of the main glutamate transporters in the brain is the excitatory amino acid transporter EAAT2 (or GLT1). The EAAT2 variety comprises approximately 90% of glutamate transporters in the brain (Yamada et al., 2006). The extracellular glutamate level is maintained at a lower level than the neurotoxic level by excitatory amino acid transporters (Ghanizadeh and Berk, 2015). Glutamate neurotoxicity may serve as a pathological mechanism for autism (Harada et al., 2011). An imbalance of inhibitory and excitatory systems in the neurobiology of autism (Polleux and Lauder, 2004) is indicated by an abnormal GABA to glutamate ratio (Harada et al., 2011).
The β-lactam antibiotics cefixime and ceftriaxone are third-generation cephalosporins. GLT1/EAAT2 expression is increased by ceftriaxone (Nizzardo et al., 2011). Rothstein and colleagues demonstrated that many β-lactam antibiotics are able to increase EAAT2 protein levels through transcriptional activation (Rothstein et al., 2005). Ceftriaxone reduces extracellular glutamate levels by increasing the expression of astrocyte glutamate transporters (Zeng et al., 2010).
Growing evidence suggests that vitamin D likely participates in the pathogenesis of ASD, and vitamin D deficiency may be one of the causes of ASD (Mostafa and Al-Ayadhi, 2012; Gong et al., 2014; Fernell et al., 2015; Vinkhuyzen et al., 2018). Neurotransmitters associated with vitamin D are responsible for the regulation of learning, memory, and emotions (Patrick and Ames, 2014; Pertile et al., 2018; Anjum et al., 2018). Research indicates that prolonged administration of vitamin D to rodents can promote the synthesis of GABA production in brain regions, including the prefrontal cortex, anterior cingulate cortex, and hippocampus (Wang et al., 2022).
The purpose of this study is to evaluate the effectiveness of GABA and combined nutritional therapies as an intervention strategy for treating autism by employing a PPA-induced rodent model of autism. By measuring various biomarkers in brain tissue samples including GABA, glutamate, KCC2, KNCC1, Gamma-Aminobutyric Acid Receptor Subunit Alpha-5 (GABRA5), EAAT2, vitamin D3 (VD3) and several oxidative stress biomarkers. The study aimed to elucidate the neurochemical changes associated with different treatment regimens. Through this investigation, it is hoped to provide insights into how GABA supplementation and its combinations (β-lactam, probiotics and vitamin D3) affect the neurochemical pathways in ASD, thereby contributing to the development of more effective therapeutic strategies for the management of autism symptoms.
Sixty young male western Albino rats, approximately 3 weeks old, weighing about 60–80 g, were obtained from the Experimental Surgery and Animal Laboratory at King Saud University (Riyadh, Saudi Arabia). Animals were housed in groups of 5 animals per cage at 22 ± 1°C and exposed to 12:12 h light–dark cycle and had access to food and water ad libitum. The Ethics Committee approved all procedures for Animal Research of King Saud University, Riyadh (IRB No: KSU-SE-23-58).
Sixty rats approximately 3 weeks old at the start of the treatment, were randomly assigned into six groups, with 10 rats in each group. Before the experiment began, all animals underwent a one-week acclimatization period to allow adaptation to the laboratory environment. Group I (Control) received phosphate-buffered saline (PBS) orally for 24 days. Group II (PPA group) received a neurotoxic dose of PPA (250 mg/kg body weight/day) orally via gavage for 3 days, followed by PBS for 21 days (El-Ansary et al., 2012). Group III (Control-GABA) received PBS for 3 days, then orally given GABA supplementation (ABA Pure powder, NOW®, 200 mg/kg body weight/day) for 21 days according to Abd El-Hady et al. (2017). Group IV (Control-combination) was administered PBS for 3 days, then orally given a combination therapy of GABA (200 mg/kg/day for 21 days), probiotics (Fiber probiotic, Proflora, 0.2 g/kg/day for 21 days), vitamin D3 (SUN-D 1000, California Greens, 1,000 IU/kg/day for 14 days) and β-lactam (Amoxicillin, HYMOX®, 200 mg/kg/day for 7 days) as described in previous studies (Bin-Khattaf et al., 2022; Alfawaz et al., 2014; Al-Suwailem et al., 2019). Group V (PPA-GABA), the GABA-therapeutic group, received the same oral PPA dose as Group II, then orally given GABA supplementation. Group VI (PPA-combination) was similarly orally administered PPA, then a combination therapy as described in Group IV (Figure 1).
Social behavior was evaluated using the three-chamber social test, a widely used method to assess social deficits in animal models of autism (Schwartzer et al., 2013; Lo et al., 2016). The testing apparatus was a clear plexiglass box measuring 80 cm × 40 cm × 40 cm, divided into three chambers, each connected by a 15 cm × 15 cm doorway. Between trials, the three-chamberm box was cleaned with 70% ethanol, dried with paper towels, and left to air dry. To acclimate to the environment, animals were brought into the testing room 1 h before the test. Each animal was individually placed in the center chamber and allowed to explore freely for 5 min. After this habituation period, a novel, same-sex conspecific rat of similar body weight was placed in one of two perforated holding containers on either side of the box. The test subject was then given 10 min to explore all three chambers. To avoid side bias, the position of the conspecific rat was alternated between trials. The test was recorded using a Digital HERO camcorder (GoPro, San Mateo, California, United States). Recorded videos were later analyzed for behavior coding using BORIS 7.9.16 software (Friard and Gamba, 2016) by examiners blinded to the treatment groups. Social interaction time, defined as the test subject’s orientation toward and investigation of the container holding the conspecific rat through sniffing or rearing behaviors, was measured. Additionally, time spent in each chamber was recorded, and the percent of time spent in the social chamber (containing the conspecific rat) relative to all chamber was calculated. Finally, Immobility (bouts of no movement) and mobility time were also recorded.
At the end of the experiment, the rats were euthanized by inhalation of an overdose of anesthetic (sevoflurane). The brain was rapidly removed from the skull, flash-frozen on dry ice, and then stored at −80°C. Each brain was divided into two halves: one half was designated for biochemical assays, and the other for histopathological analysis. The half designated for biochemical assays was dissected into small pieces and completely homogenized in 10 w/v volumes of double-distilled water. Measurement of the selected biochemical markers, including oxidative stress biomarkers [glutathione-S-transferase (GST), catalase, lipid peroxide, glutathione (GSH) and vitamin C] and EAAT2, KCC2, KNCC1, GABA, VD3, glutamate and GABRA5 in the brain homogenates of the study groups was performed using a spectrophotometric and Enzyme-Linked Immunoassay (ELISA). The sample size for each biomarker was 46.
• GST: This enzyme was assessed by measuring the conjugation of 1-chloro,2,4-dinitrobenzene (CDNB) with GSH. This was followed by observing the increase in the OD at 340 nm.
• Catalase: The total volume of the reaction mixture was 3 mL. It contained 1.5 mL of 0.2 M sodium phosphate buffer pH 7.2, 1.2 mL of 0.5 mM hydrogen peroxide and enzyme. The reaction was started by adding hydrogen peroxide (H2O2), and the rate of change in absorbance was measured at 240 nm for 2 min (Aebi, 1984). Values were expressed as μmoles of H2O2 dissociated/min/dL brain homogenates, that is, U/dL.
• Lipid Peroxidation: Lipid oxidation was evaluated by measuring the levels of lipid peroxidation by-products as thiobarbituric acid reactive substances (TBARS), namely malondialdehyde (MD), using the method of Ruiz-Larrea et al. (1994). Accordingly, the samples were heated with TBA at low pH and the formation of a pink chromogen was measured by absorbance at 532 nm. The concentration of lipid peroxides was calculated as μmoles/ml using the extinction coefficient of MD.
• GSH: GSH content was determined according to the method described by Beutler et al. (1963) using 5,5′-dithiobis 2-nitrobenzoic acid (DTNB) with sulfhydryl compounds to produce a relatively stable yellow color.
• Vitamin C: Assay of vitamin C was performed according to the method of Jagota and Dani (1982). A quantity of 0.2 mL of brain homogenates was mixed with 0.8 mL of 10% trichloroacetic acid (TCA) and incubated in ice for 5 min. The samples were then centrifuged for 10 min at 3,500 rpm and 4°C. An amount of 1.5 mL double distilled water was subsequently added to 0.5 mL of the supernatant. Eventually, 2 mL of Folin-phenol reagent were added, and absorbance was measured at 760 nm after 10 min.
The Rat EAAT2 ELISA kit (ELK7105, Wuhan, China) used a quantitative sandwich enzyme immunoassay. The microtiter plate was pre-coated with an EAAT2-specific antibody. After adding standards or samples and a biotin-conjugated EAAT2 antibody, avidin-HRP was added and incubated. The reaction was stopped with sulfuric acid, and the color change was measured at 450 nm. The detection range for EAAT2 was 0.16–10 ng/mL.
KCC2 levels were measured using an ELISA kit (ELK Biotechnology, ELK0481, Wuhan, China). The assay used a sandwich enzyme immunoassay with a microtiter plate pre-coated with an antibody specific to Rat KCC2. Standards or samples were added to the appropriate microtiter plate wells then with a biotin-conjugated KCC2 antibody. Next avidin-HRP were added. A substrate solution then produced a colorimetric reaction, with a detection range of 0.32–20 ng/mL.
The Rat NKCC1 ELISA kit (ELK 0482, Wuhan, China) quantitatively determined NKCC1 concentrations in brain tissue using a sandwich enzyme immunoassay. The microtiter plate was pre-coated with an NKCC1-specific antibody. After adding standards or samples, a biotin-conjugated NKCC1 antibody and avidin-HRP were added. The reaction was stopped with sulfuric acid, and the color change was measured at 450 nm. The detection range was 0.32–20 ng/mL.
The Rat GABA ELISA kit (ELK 9167, Wuhan, China) used a competitive inhibition enzyme immunoassay. The microtiter plate was pre-coated with GABA protein. After adding standards or samples and a biotin-conjugated GABA antibody, avidin-HRP was added and incubated. The reaction was stopped with sulfuric acid, and the color change was measured at 450 nm. The detection range was 31.25–2000 pg./mL.
The Rat VD3 ELISA kit (ELK 8950, Wuhan, China) used a competitive inhibition enzyme immunoassay. The microtiter plate was pre-coated with VD3 protein. After adding standards or samples and a biotin-conjugated VD3 antibody, avidin-HRP was added and incubated. The reaction was stopped with sulfuric acid, and the color change was measured at 450 nm. The detection range was 6.25–400 ng/mL.
Glutamate levels in brain tissue were measured using a double-sandwich ELISA kit (MyBioSource Ltd., San Diego, CA, United States; Cat. No: MBS269969). The pre-coated antibody was an anti-Rat Glutamate monoclonal antibody, and the detecting antibody was biotinylated polyclonal antibody. After adding samples and biotinylated antibodies, Avidin-peroxidase conjugates were added. The reaction was measured at 450 nm, with a detection range of 0.312–20 nmoL/mL.
GABRA5 levels in brain tissue were measured using a quantitative sandwich ELISA kit (MyBioSource Ltd., San Diego, CA, USA; Cat. No: MBS9335790). The microtiter plate was pre-coated with a GABRA5-specific antibody. The reaction was stopped with sulfuric acid, and the color change was measured at 450 nm. The detection range was 0.5–16 ng/mL.
Half of each rat’s brain (n = 12, with 2 brains from each experimental group) was fixed in 10% neutral buffered formalin. Then, they underwent routine histopathological processing, embedding in paraffin, sectioning to “5 μm thickness,” and staining with hematoxylin and eosin. The slides were then examined using light microscope by a certified surgical pathologist.
Statistical analysis was conducted using SPSS software (SPSS Inc., Chicago, IL, United States) version 22 for all analyses. Data were expressed as mean ± SD. One-way ANOVA and Kruskal-Wallis tests assessed group differences, followed by the Least Significant Difference (LSD) or Mann–Whitney tests for multiple comparisons. ROC curve analysis evaluated the diagnostic performance of biochemical parameters, reporting AUC, sensitivity, and specificity. Parameters with high AUC values were strong biomarker candidates. Stepwise multiple regression identified significant predictors, and standardized regression coefficients assessed their importance. Person correlation analysis explored relationships between biochemical parameters, reporting correlation coefficients (R) and p values. Animal behavior variables were analyzed by one- and two- way ANOVA followed by Tukey’s least significant difference test for multiple comparisons, with differences considered significant at p ≤ 0.05.
The behavioral assessment shown in Figure 2 evaluated interactions in a three-chamber social test across the six groups: control, PPA-treated, control-GABA, control-combination, PPA-GABA, and PPA-combination. Results showed that PPA-treated animals spent significantly less time interacting with a conspecific animal than control animals (p < 0.0001; Figure 2A). GABA and combination therapies improved social interactions in PPA-treated animals, with the combination group showing the most significant recovery (p < 0.05 vs. PPA; Figure 2A). In terms of interaction frequency, control animals had higher interaction episodes than PPA-treated animals (p < 0.001; Figure 2B). Both PPA-GABA and PPA-combination groups improved interaction frequency, with the combination group showing the most significant improvement (p < 0.001; Figure 2B). Similarly, control animals spent more time in the social chamber than PPA-treated animals (p < 0.001; Figure 2C), but this deficit was alleviated by both PPA-GABA and PPA-combination therapies (p < 0.05 vs. PPA; Figure 2C). The PPA group spent more time immobile (p = 0.0003; Figure 2D), while both GABA and combination therapies reduced immobility, with the combination group showing the most improvement (p = 0.001; Figure 2D). Control animals displayed more locomotion than PPA-treated animals (p = 0.003; Figure 2E), whereas the combination therapy group showed the most significant recovery (p = 0.0004 vs. PPA; Figure 2E). Lastly, while control animals showed a preference for social interaction over object interaction, PPA-treated animals did not (Figure 2F). Remarkably, the combination therapy restored this preference, with the PPA-combination group spending significantly more time interacting with the conspecific animal (p = 0.0003 vs. PPA; Figure 2F). Notably, PPA-treated animals exhibited deficits in social interaction, locomotor activity, and social preference, while combination therapy provided the most significant recovery in all behavioral measures.
Figure 2. Social interaction in the three-chamber social test following control (n = 7), propionic acid (PPA) treatment, (n = 7), control-GABA (n = 7), control-combination interventions (n = 7), PPA-GABA (n = 7), PPA-combination (n = 7): (A) time spent (seconds) by focal animals interacting with the conspecific animal (social); (B) number of occurrences (count) by focal animals interacting with the conspecific animal (social); (C) percent of time (%) spent in the social chamber relative to other chambers; (D) time spent (seconds) immobile during the test; (E) time spent (seconds) mobile during the test; (F) time spent (seconds) by focal animals interacting with the conspecific animal (social) and the empty (object) holding box during the three-chamber social test. Data presented are means ± standard error. Significant one-way and two-way ANOVAs were followed by multiple comparisons by Tukey’s least significance difference ****p < 0.0001, ***p < 0.001, **p ≤ 0.01, *p ≤ 0.05.
The results from Table 1 and Supplementary Figure 1 demonstrate significant biochemical alterations across the different treatment groups. The PPA-treated group showed decreased antioxidant defenses, with reduced GST, catalase, GSH, and Vitamin C levels, alongside increased oxidative stress biomarkers such as lipid peroxides. The combination therapy (GABA, probiotics, VD3, and β-lactam) in PPA-combination group significantly restored these antioxidant biomarkers, particularly catalase and GSH levels. Additionally, neurotransmitter balance was disrupted in the PPA group, with lower GABA and higher glutamate levels. Both GABA and combination therapeutic groups improved GABA levels, with the PPA- combination group achieving the greatest recovery. The PPA- combination group also reduced glutamate levels, normalized EAAT2 function, and restored the GABA/Glutamate ratio, which was essential for improving inhibitory neurotransmission and restoring chloride cotransporters homeostasis (KCC2/NKCC1 ratio). One can see that PPA induced oxidative stress and neurotransmitter imbalances, while combination therapy significantly restored these markers.
The results in Table 2 revealed significant correlations between various oxidative stress biomarkers and neurotransmitters system. There was a positive correlation between catalase and GSH (r = 0.311, p = 0.035), and between GSH with GABA (r = 0.401, p = 0.006) and GABA/ Glutamate (r = 0.432, p = 0.003). Vitamin C is positively correlated with GABA (r = 0.305, p = 0.039), and the KCC2/NKCC1 ratio positively correlates with the GABA/Glutamate ratio (r = 0.718, p = 0.000). Additionally, VD3 positively correlated with GABA and the GABA/Glutamate ratio. In contrast, negative correlations were observed between lipid peroxides and Vitamin C (r = −0.318, p = 0.031), lipid peroxides with EAAT2 (r = −0.295, p = 0.047) and KCC2 (r = −0.344, p = 0.019), and NKCC1 and GABA (r = −0.622, p = 0.000). Additionally, GSH negatively correlated with EAAT2 (r = −0.349, p = 0.017) and NKCC1 (r = −0.376, p = 0.010) and Glutamate (r = −0.325, p = 0.027). Lastly, Glutamate negatively correlated with GABA (r = −0.771, p = 0.000) and the KCC2/NKCC1 ratio (r = −0.609, p = 0.000). Thus, GABA levels were strongly correlated with antioxidant status, while oxidative stress negatively impacted neurotransmitter function.
The results from the multiple regression analyses across Tables 3–15 highlighted key predictors influencing various biomarkers. Table 3 indicated that GSH significantly predicted catalase levels (p = 0.035), explaining around 7.6% of the variance. Table 4 identified KCC2, vitamin C, and EAAT2 as significant negative predictors of lipid peroxides, explaining up to 25.5% of the variance. In Table 5, the GABA/Glutamate ratio and VD3 significantly predicted GSH levels (p = 0.003 and p = 0.013, respectively), with the GABA/Glutamate ratio being the strongest predictor. In Table 6, lipid peroxides negatively predicted vitamin C levels (p = 0.023), while GABA positively influenced vitamin C. Table 7 showed that the GABA/Glutamate ratio and lipid peroxides negatively predicted EAAT2 levels, with glutamate and KCC2/NKCC1 also having negative influences. Table 8 showed that VD3 and the KCC2/NKCC1 ratio significantly predicted KCC2 levels. VD3 having a negative relationship, while KCC2/NKCC1 positively influenced KCC2. Table 9 revealed that KCC2/NKCC1 was the strongest predictor of NKCC1 levels. Table 10 showed that the GABA/Glutamate ratio and Glutamate significantly predicted GABA levels, with a very high adjusted R-square (0.973). Table 11 highlighted the GABA/Glutamate ratio and GSH as significant predictors of VD3 levels, where the GABA/Glutamate ratio positively correlated with VD3, while GSH showed a negative association. Table 12 demonstrated that the GABA/Glutamate ratio and GABA were strong predictors of Glutamate levels, with the GABA/Glutamate ratio negatively correlating with Glutamate. Table 13 revealed that KCC2 was a significant predictor for GABRA5, showing a positive correlation. Table 14 indicated that NKCC1, KCC2 and GABA/Glutamate ratio were potent predictors for the KCC2/NKCC1 ratio. Table 15 identified GABA and Glutamate as key predictors of the GABA/Glutamate ratio, with GABA positively influencing the ratio and Glutamate having a negative effect. Overall, neurotransmitter imbalances and oxidative stress markers were highly predictive of biochemical changes.
The ROC analysis (Tables 16–20; Supplementary Figures 2–6) evaluated the diagnostic ability of various biomarkers to differentiate between treatment groups (PPA-treated, control-GABA, control-combination, PPA-GABA and PPA-combination) using the control group as a reference. The Area Under the Curve (AUC) values give insight into the accuracy of these biomarkers. In Table 16 and Supplementary Figure 2 (PPA-treated vs. control), GST, GSH, KCC2 and GABA showed relatively high AUC values (AUC > 0.75), with high sensitivity and specificity. For example, KCC2 showed good diagnostic accuracy (AUC = 0.844) with 75% sensitivity and 100% specificity. Table 17 and Supplementary Figure 3 (Control-GABA vs. control) highlighted catalase (AUC = 0.847, p = 0.016) and EAAT2 (AUC = 0.958, p = 0.001) as good predictors, while other parameters showed moderate diagnostic value. Table 18 and Supplementary Figure 4 (Control-Combination vs. control), GABA again showed strong diagnostic potential (AUC = 0.920), as did lipid peroxides and GABA/Glutamate ratio. Table 19 and Supplementary Figure 5 (PPA-GABA vs. control) demonstrated GABA and the GABA/Glutamate ratio as strong predictors (AUC = 1.000 for both), with high sensitivity and specificity. Likewise, Table 20 and Supplementary Figure 6 (PPA-Combination vs. control) revealed several parameters, including GABA, GSH, EAAT2, and KCC2/NKCC1 and GABA/Glutamate ratio, with AUC values of 1.000. Therefore, GABA, EAAT2, and KCC2 were the most reliable biomarkers for distinguishing treatment effects.
The Histopathology studies examined various parts of each brain sample (two rats from each group) such as the cerebrum, cerebellum, and hippocampus. Visible changes were observed in the hippocampus, as illustrated in Figure 3. In the control group, there was maintained cellular density with 5–6 cellular layers and no degenerative changes under high magnification (Figure 3A) in the CA4 region of the hippocampus. Rats in the group intoxicated with PPA (PPA-treated group) exhibited decreased cellular density and degenerative changes, including prominent eosinophilic cytoplasm and pyknotic nuclei (Figure 3B). Control-GABA group showed milder findings than PPA group, with mild degenerative changes observed (Figure 3C). Control-combination group displayed similar cellular density with no degenerative changes compared to the control group (Figure 3D). The rats receiving GABA therapy (PPA-GABA group) exhibited degenerative changes and reduced neuron density in the CA4 region, similar to PPA-treated group (Figure 3E). PPA-combination group did not show any significant changes compared to the control group (Figure 3F). The changes in cellular density and degenerative features varied across the different experimental groups, with PPA group showing the most pronounced alterations. It is worthy to note that PPA caused severe hippocampal degeneration, while combination therapy provided strong neuroprotection.
Figure 3. H&E-stained sections of the hippocampus with arrows pointing to the CA4 region (400X low power field). Inset: Higher magnification demonstrated the cellular density in the CA4 region of the hippocampus for each group. (A) Control group, (B) PPA-treated group, (C) Control-GABA group, (D) Control-combination group, (E) PPA- GABA group, and (F) PPA- combination group.
This study highlighted the multi-faceted impact of GABA supplementation, and combination therapy (GABA, probiotics, VD3, and EAAT2 activator, β-lactam) on neurotransmitter balance, oxidative stress and social behavior in PPA-induced rodent model of autism. The obtained results were in line with growing evidence suggesting that an imbalance between excitatory and inhibitory neurotransmission, particularly involving glutamate and GABA, is central to ASD pathophysiology (Rubenstein and Merzenich, 2003).
PPA administration induced marked biochemical, behavioral, and histopathological changes in the rodents, modeling the E/I imbalance seen in ASD. PPA caused a significant reduction in GABA levels while increasing glutamate excitotoxicity, mimicking the neural excitation dominance observed in ASD (El-Ansary et al., 2017; Ajram et al., 2019). This imbalance directly correlates with oxidative stress, as seen in the elevated levels of lipid peroxidation and decreased antioxidant defenses (GSH, catalase), which have been previously implicated in autism-related neuroinflammation and neurodegeneration (Usui et al., 2023).
Histopathologically, PPA-treated rats exhibited hippocampal neurodegeneration, a crucial region for social and cognitive processing (Long et al., 2024). Structural and functional abnormalities in the hippocampus are frequently reported in individuals with ASD (Long et al., 2024; Etherton et al., 2011; Guo et al., 2021), and the degeneration observed in this study suggested that oxidative damage and E/I imbalance contributed to its deterioration. Individuals with ASD are reported to have elevated glutamate levels in the amygdala and hippocampus complex, supporting this excitotoxicity link (Page et al., 2006). The PPA-treated rats also exhibited impaired social interactions and increased immobility, indicating anxiety-like behavior, both common features in ASD models (Schwartzer et al., 2013; Mirza and Sharma, 2018; Alò et al., 2021). These behavioral deficits were closely tied to reduced GABA levels, which impair social processing (Parrella et al., 2024). Abruzzo et al. (2021) showed that oxidative stress disrupts chloride co-transporters in the hippocampus, contributing to E/I imbalances and behavioral abnormalities in ASD models. Overall, oxidative damage and E/I imbalance in the hippocampus appeared to drive the behavioral and social impairments observed in PPA-treated rats, mimicking ASD-like symptoms.
GABA supplementation provided moderate improvements in both neurotransmitter balance and oxidative stress markers. Biochemically, GABA levels were increased, leading to a slight reduction in glutamate levels and an improvement in the E/I balance. This restoration of GABAergic signaling helped in reducing glutamate excitotoxicity, nevertheless the improvements were modest compared to the effects observed with combination therapy. GABA supplementation slightly increased the expression of GABRA5 which enhanced inhibitory neurotransmission (Jacob, 2019), however, it was not sufficient to fully counteract the elevated glutamate levels or oxidative stress present in PPA-treated rats.
Behaviorally, GABA-treated rats showed improved social interactions compared to the PPA-treated group, suggesting that enhancing GABAergic signaling could mitigate some of the social deficits associated with ASD. This observation supports evidence from other studies, such as the work of Cochran et al. (2015), which highlighted the central role of GABAergic deficits in ASD-related social impairments. However, the improvement was moderate, emphasizing the need for more comprehensive therapeutic approaches that target both GABAergic and glutamatergic systems. For instance, studies with GABA agonists like baclofen have shown that these agents can significantly ameliorate symptoms such as hyperactivity, impaired social interaction, and spatial memory deficits in gene-altered mice (Han et al., 2012; Han et al., 2014). In mouse models of E/I dysfunction, GABA_B receptor agonists like arbaclofen have been shown to restore aspects of the E/I balance, resulting in improved behavioral deficits (Gandal et al., 2012).
Histopathologically, while GABA supplementation helped in preserving some aspects of hippocampal structure, significant neurodegeneration and reduced neuron density remained, particularly in the CA4 region, similar to what was observed in the PPA-treated group. This aligns with the moderate behavioral improvements seen in social interactions, suggesting that oxidative stress and excitatory overactivity continued to drive damage despite partial restoration of GABAergic function. Similar outcomes have been observed in the BTBR mouse model of autism, where the hippocampal commissure is smaller than usual, Silverman et al. (2015) demonstrated that enhancing GABA signaling through arbaclofen improved sociability and reduced neural deficits. Additionally, GABRA5 receptors play a crucial role in maintaining inhibitory control in the hippocampus, and modulating these receptors can help rebalance E/I dynamics. Targeting these receptors has been shown to improve cognitive and social behaviors in ASD models (Jacob, 2019), however in this study, the neuroprotective effects of GABA supplementation were limited.
The combination of GABA, probiotics, VD3, and β-lactam was significantly the most effective across all metrics such as GABA/Glutamate and KCC2/NKCC1 ratios. This multi-faceted approach restored inhibitory effect of GABA, reduced glutamate excitotoxicity, and normalized oxidative stress markers to near-control levels. This suggested that the combination therapy effectively addressed both E/I imbalance and oxidative stress, leading to the most substantial improvements in social behavior and neural integrity (Salim, 2017; Li, 2022).
The behavioral and histopathological outcomes observed in the combination therapy group were highly compelling. Social interaction in the treated animals was restored to near-normal levels, and immobility was significantly reduced, indicating improvements in anxiety-like behavior. Histopathologically, the combination therapy provided substantial neuroprotection, nearly eliminating the neurodegenerative changes typically seen in the hippocampus of PPA-treated rats. These results were in line with previous studies which showed that multi-faceted approaches addressing both glutamate excitotoxicity and E/I imbalances can lead to significant behavioral recovery and preservation of neural integrity in ASD models.
Oral intake of GABA has been shown to impact the brain, as evidenced by changes in EEG readings and cognitive performance improvements (Almutairi et al., 2024). This is further supported by the findings of Tabouy et al. (2018) who demonstrated that treatment with L. reuteri decreased repetitive behaviors and increased GABA receptor gene expression (GABRA1, GABRA3, and GABRB1) as well as protein levels (GABRA1) in the hippocampus and prefrontal cortex (PFC) of Shank3 mutant mice, a model of ASD. Bravo et al. (2011) also highlighted how treatment with Lactobacillus regulated emotional behavior and central GABA receptor expression via the vagus nerve, which connects the brain and gut, suggesting the potential for probiotics to modulate the gut-brain axis in ASD treatment.
Moreover, probiotics that stimulate inhibitory neurotransmission by increasing GABA levels and its receptors may contribute to restore the E/I balance, which is often disrupted in ASD. This restoration could improve social interactions, as previously demonstrated in a study of El-Ansary et al. (2018). Additionally, it normalizes the PPA-induced increase in brain-derived neurotrophic factor (BDNF) transcript levels in the hippocampus, further supporting neuroplasticity and cognitive recovery (Abuaish et al., 2021).
GABA/Glutamate and KCC2/NKCC1 ratios in the brain tissue were significantly higher in PPA-combination group compared to all groups. This supported the hypothesis of El-Ansary (2020) which suggested that the increased expression of KCC2/NKCC1 lead to the most an inhibitory affect of GABA. These findings strongly supported that the alteration of KCC2 function in neurodevelopmental disorders leads to increased excitatory effects of GABA and abnormalities in social behavior and memory retention (Moore et al., 2019), a disruption that can be corrected through combination therapy in autism models. GABA_A receptors activation plays a crucial role in regulating KCC2 activity by stabilizing KCC2 at the plasma membrane, while inhibition of GABA_A receptors leads to decreased KCC2 stability through increased diffusion and endocytosis (Heubl et al., 2017). This mechanism is vital for maintaining chloride homeostasis and ensuring effective inhibitory neurotransmission in hippocampal circuits, which are essential for learning, memory, and overall neural regulation (Hamze et al., 2024). In the hippocampus, KCC2 mRNA levels are closely linked to GABA function, with overexpression of KCC2 shifting GABA’s action from excitatory to inhibitory, which helps maintaining the E/I balance (Ben-Ari, 2002). Proper timing of this GABA switch, mediated by KCC2, is crucial for brain development, and exposure to early-life stress can delay this switch, causing behavioral abnormalities (Furukawa et al., 2017). For instance, Tsukahara et al. (2016) found that repeated stress in mice reduced novel object recognition ability and social behavior, which was associated with decreased KCC2 levels in the hippocampus, highlighting the importance of KCC2 in maintaining healthy cognitive and social function.
VD3 has been closely linked to neuroprotection, primarily through its ability to reduce neuroinflammation and enhance GABA synthesis. Studies have shown that long-term treatment with vitamin D promotes GABA synthesis in crucial brain areas such as the prefrontal cortex, anterior cingulate cortex, and hippocampus (Staal et al., 2012). In addition to boosting GABA production, vitamin D exerts a potent antioxidant effect, inhibiting the production of nitric oxide synthase and upregulating glutathione levels, which helps reduce glial cell activation and neuroinflammation (DeLuca, 2016). These anti-inflammatory and neuroprotective properties are enhanced by vitamin D’s ability to upregulate antioxidant-related genes, including those encoding superoxide dismutase and thioredoxin reductase (Halicka et al., 2012). In ASD models, vitamin D deficiency has been linked to worsened ASD-like behaviors and lower serum vitamin D levels, which negatively impact brain development. In contrast, interventions using vitamin D have shown significant improvements in both the growth and behavior of ASD rats (Cannell, 2017). Furthermore, research indicates that low vitamin D levels are associated with reduced hippocampal volume and impaired structural connectivity, which can contribute to cognitive deficits seen in neurodegenerative disorders (Al-Amin et al., 2019).
While the β-lactam component was intended to increase EAAT2 expression and reduce glutamate toxicity (Takahashi et al., 2015), EAAT2 levels were surprisingly lower (17.51% of control) in the PPA-combination group. This decrease could be a compensatory response to the reduced extracellular glutamate levels resulting from the combination therapy. Research shows that glutamate transporters like EAAT2 dynamically adjust their density in response to changes in ambient glutamate (Borisova, 2016). In this context, the reduction in EAAT2 may help balance signaling by limiting glutamate uptake under conditions of low extracellular glutamate. Similar adaptive mechanisms have been observed in other neurological conditions, where reduced EAAT2 levels in certain brain regions appear to help maintain E/I balance (O'Donovan et al., 2017; Ryan et al., 2021).
Additionally, studies have shown that β-lactam antibiotics are associated with improved behavior and neuronal integrity. Research by Ho et al. (2014) and Feng et al. (2014) found that β-lactam antibiotics alleviated memory and cognitive impairments while significantly reducing cellular loss in the hippocampus, a region critical for learning and memory. These findings suggest that EAAT2 regulation is a complex process, adapting to the neurochemical environment influenced by therapies like β-lactam antibiotics. Future research is needed to further understand how EAAT2 dynamics contribute to managing excitotoxicity and maintaining neurotransmitter balance.
This study painted a cohesive picture of how PPA-induced disruptions in neurotransmitter balance and oxidative stress led to the behavioral and neural abnormalities seen in autism models. GABA supplementation alone partially alleviated these issues but was insufficient to fully restore function, highlighting the complexity of the disorder. In contrast, the combination therapy targeted multiple pathological mechanisms, offering a more comprehensive solution that significantly improved both neurochemical and behavioral outcomes.
Since the mechanism causing the sex-biased prevalence of ASDs is still poorly understood, animal models of the disorder could help us better understand how biological sex influences these disorders. Regretfully, the use of only male rats in this study and the lack of female rats may be viewed as limitations. Future research on both sexes is necessary.
This study demonstrated that GABA supplementation and combined nutritional therapies (GABA, probiotics, VD3, and β-lactam) offer significant neuroprotective effects in a PPA-induced rodent model of autism. While GABA alone showed improvements in some oxidative stress markers and partial restoration of excitatory-inhibitory balance, the combination therapy was more effective in reversing neurochemical and behavioral deficits associated with PPA-induced neurotoxicity. The combination therapy significantly restored GABA and glutamate levels improved the GABA/Glutamate and KCC2/NKCC1 ratios, and enhanced antioxidant defenses, particularly GSH levels. Histopathological analysis confirmed that the combination treatment preserved hippocampal cellular integrity, which was severely compromised in the PPA-treated group. These findings suggested that targeting multiple pathways, including GABAergic modulation, oxidative stress reduction, and glutamate transporter enhancement could be a promising therapeutic strategy for alleviating autism symptoms. Further research is needed to explore the long-term efficacy and safety of these interventions in clinical settings.
The original contributions presented in the study are included in the article/Supplementary material, further inquiries can be directed to the corresponding author.
The animal study was approved by The Ethics Committee approved all procedures for Animal Research of King Saud University, Riyadh (IRB No: KSU-SE-23-58). The study was conducted in accordance with the local legislation and institutional requirements.
ANA: Investigation, Methodology, Writing – original draft. AB: Conceptualization, Data curation, Supervision, Validation, Writing – review & editing. MA: Funding acquisition, Project administration, Resources, Supervision, Visualization, Writing – review & editing. SA: Supervision, Formal analysis, Methodology, Validation, Writing – review & editing. AA: Methodology, Investigation, Resources, Writing – review & editing. LA-A: Conceptualization, Funding acquisition, Supervision, Validation, Writing – review & editing. AE-A: Conceptualization, Supervision, Validation, Data curation, Visualization, Writing – review & editing.
The author(s) declare that no financial support was received for the research and/or publication of this article.
This project was funded by the National Plan for Science Technology and Innovation (MAARIFAH), King Abdulaziz City for Science and Technology; Kingdom of Saudi Arabia (award number: 08-MED 510-02).
The authors declare that the research was conducted in the absence of any commercial or financial relationships that could be construed as a potential conflict of interest.
The authors declare that no Gen AI was used in the creation of this manuscript.
All claims expressed in this article are solely those of the authors and do not necessarily represent those of their affiliated organizations, or those of the publisher, the editors and the reviewers. Any product that may be evaluated in this article, or claim that may be made by its manufacturer, is not guaranteed or endorsed by the publisher.
The Supplementary material for this article can be found online at: https://www.frontiersin.org/articles/10.3389/fnmol.2025.1553438/full#supplementary-material
Abd El-Hady, A. M., Gewefel, H. S., and Badawi, M. A. (2017). Gamma-aminobutyric acid ameliorates gamma rays-induced oxidative stress in the small intestine of rats. JoBAZ. 78:2. doi: 10.1186/s41936-017-0005-3
Abruzzo, P. M., Panisi, C., and Marini, M. (2021). The alteration of chloride homeostasis/GABAergic signaling in brain disorders: could oxidative stress play a role? Antioxidants (Basel). 10:1316. doi: 10.3390/antiox10081316
Abuaish, S., Al-Otaibi, N. M., and Aabed, K. (2022). The efficacy of fecal transplantation and Bifidobacterium supplementation in ameliorating propionic acid-induced behavioral and biochemical autistic features in juvenile male rats. J. Mol. Neurosci. 72, 372–381. doi: 10.1007/s12031-021-01959-8
Abuaish, S., Al-Otaibi, N. M., and Abujamel, T. S. (2021). Fecal transplant and Bifidobacterium treatments modulate gut Clostridium Bacteria and rescue social impairment and hippocampal BDNF expression in a rodent model of autism. Brain Sci. 11:1038. doi: 10.3390/brainsci11081038
Abujamel, T. S., Al-Otaibi, N. M., and Abuaish, S. (2022). Different alterations in gut microbiota between Bifidobacterium longum and fecal microbiota transplantation treatments in propionic acid rat model of autism. Nutrients 14:608. doi: 10.3390/nu14030608
Aebi, H. (1984). Catalase in vitro. Methods Enzymol. 105, 121–126. doi: 10.1016/s0076-6879(84)05016-3
Ajram, L. A., Pereira, A. C., Durieux, A. M. S., Velthius, H. E., Petrinovic, M. M., and McAlonan, G. M. (2019). The contribution of [1H] magnetic resonance spectroscopy to the study of excitation-inhibition in autism. Prog. Neuro-Psychopharmacol. Biol. Psychiatry 89, 236–244. doi: 10.1016/j.pnpbp.2018.09.010
Al-Amin, M., Bradford, D., and Sullivan, R. K. P. (2019). Vitamin D deficiency is associated with reduced hippocampal volume and disrupted structural connectivity in patients with mild cognitive impairment. Hum. Brain Mapp. 40, 394–406. doi: 10.1002/hbm.24380
AlBatti, T. H., Alsaghan, L. B., and Alsharif, M. F. (2022). Prevalence of autism spectrum disorder among Saudi children between 2 and 4 years old in Riyadh. Asian J. Psychiatr. 71:103054. doi: 10.1016/j.ajp.2022.103054
Alfawaz, H. A., Bhat, R. S., Al-Ayadhi, L., and El-Ansary, A. K. (2014). Protective and restorative potency of vitamin D on persistent biochemical autistic features induced in propionic acid-intoxicated rat pups. BMC Complement. Altern. Med. 14:416. doi: 10.1186/1472-6882-14-416
Almutairi, S., Sivadas, A., and Kwakowsky, A. (2024). The effect of Oral GABA on the nervous system: potential for therapeutic intervention. Forum Nutr. 4, 241–259. doi: 10.3390/nutraceuticals4020015
Alò, R., Olivito, I., Fazzari, G., Zizza, M., Di Vito, A., Avolio, E., et al. (2021). Correlation of distinct behaviors to the modified expression of cerebral Shank1,3 and BDNF in two autistic animal models. Behav. Brain Res. 404:113165. doi: 10.1016/j.bbr.2021.113165
Al-Suwailem, E., Abdi, S., and Bhat, R. S. (2019). Glutamate signaling defects in propionic acid orally administered to juvenile rats as an experimental animal model of autism. Neurochem. J. 13:90. doi: 10.1134/S1819712419010021
American Psychiatric Association (2013). Diagnostic and statistical manual of mental disorders. 5th Edn. Arlington, VA: American Psychiatric Publishing.
Amin, Z., Mason, G. F., Cavus, I., Krystal, J. H., Rothman, D. L., and Epperson, C. N. (2006). The interaction of neuroactive steroids and GABA in the development of neuropsychiatric disorders in women. Pharmacol. Biochem. Behav. 84, 635–643. doi: 10.1016/j.pbb.2006.06.007
Anjum, I., Jaffery, S. S., Fayyaz, M., Samoo, Z., and Anjum, S. (2018). The role of vitamin D in brain health: a Mini literature review. Cureus. 10:e2960. doi: 10.7759/cureus.2960
Ben-Ari, Y. (2002). Excitatory actions of gaba during development: the nature of the nurture. Nat. Rev. Neurosci. 3, 728–739. doi: 10.1038/nrn920
Beutler, E., Duron, O., and Kelly, B. M. (1963). Improved method for the determination of blood glutathione. J. Lab. Clin. Med. 61, 882–888.
Bin-Khattaf, R. M., Al-Dbass, A. M., Alonazi, M., Bhat, R. S., Al-Daihan, S., and El-Ansary, A. K. (2024). In a rodent model of autism, probiotics decrease gut leakiness in relation to gene expression of GABA receptors: emphasize how crucial the gut-brain axis. Transl. Neurosci. 15:20220354. doi: 10.1515/tnsci-2022-0354
Bin-Khattaf, R. M., Alonazi, M. A., and Al-Dbass, A. M. (2022). Probiotic ameliorating effects of altered GABA/glutamate signaling in a rodent model of autism. Meta 12:720. doi: 10.3390/metabo12080720
Bonnet, U., Bingmann, D., and Wiemann, M. (2000). Intracellular pH modulates spontaneous and epileptiform bioelectric activity of hippocampal CA3-neurones. Eur. Neuropsychopharmacol. 10, 97–103. doi: 10.1016/s0924-977x(99)00063-2
Borisova, T. (2016). Permanent dynamic transporter-mediated turnover of glutamate across the plasma membrane of presynaptic nerve terminals: arguments in favor and against. Rev. Neurosci. 27, 71–81. doi: 10.1515/revneuro-2015-0023
Bravo, J. A., Forsythe, P., Chew, M. V., Escaravage, E., Savignac, H. M., Dinan, T. G., et al. (2011). Ingestion of Lactobacillus strain regulates emotional behavior and central GABA receptor expression in a mouse via the vagus nerve. Proc. Natl. Acad. Sci. USA 108, 16050–16055. doi: 10.1073/pnas.1102999108
Canitano, R., and Palumbi, R. (2021). Excitation/inhibition modulators in autism Spectrum disorder: current clinical research. Front. Neurosci. 15:753274. doi: 10.3389/fnins.2021.753274
Cannell, J. J. (2017). Vitamin D and autism, what's new? Rev. Endocr. Metab. Disord. 18, 183–193. doi: 10.1007/s11154-017-9409-0
Cellot, G., and Cherubini, E. (2014). GABAergic signaling as therapeutic target for autism spectrum disorders. Front. Pediatr. 2:70. doi: 10.3389/fped.2014.00070
Cochran, D. M., Sikoglu, E. M., Hodge, S. M., Edden, R. A. E., Foley, A., Kennedy, D. N., et al. (2015). Relationship among glutamine, γ-aminobutyric acid, and social cognition in autism Spectrum disorders. J. Child Adolesc. Psychopharmacol. 25, 314–322. doi: 10.1089/cap.2014.0112
DeLuca, H. F. (2016). Vitamin D: historical overview. Vitam. Horm. 100, 1–20. doi: 10.1016/bs.vh.2015.11.001
El-Ansary, A. (2020). GABA and glutamate imbalance in autism and their reversal as novel hypothesis for effective treatment strategy. Autism Dev Disord. 18, 46–63. doi: 10.17759/autdd.2020180306
El-Ansary, A., Al-Salem, H. S., Asma, A., and Al-Dbass, A. (2017). Glutamate excitotoxicity induced by orally administered propionic acid, a short chain fatty acid can be ameliorated by bee pollen. Lipids Health Dis. 16:96. doi: 10.1186/s12944-017-0485-7
El-Ansary, A., Bacha, A. B., and Bjørklund, G. (2018). Probiotic treatment reduces the autistic-like excitation/inhibition imbalance in juvenile hamsters induced by orally administered propionic acid and clindamycin. Metab. Brain Dis. 33, 1155–1164. doi: 10.1007/s11011-018-0212-8
El-Ansary, A. K., Ben Bacha, A., and Kotb, M. (2012). Etiology of autistic features: the persisting neurotoxic effects of propionic acid. J. Neuroinflammation 9:74. doi: 10.1186/1742-2094-9-74
Emberti Gialloreti, L., Mazzone, L., Benvenuto, A., Fasano, A., Garcia Alcon, A., Kraneveld, A., et al. (2019). Risk and protective environmental factors associated with autism Spectrum disorder: evidence-based principles and recommendations. J. Clin. Med. 8:8. doi: 10.3390/jcm8020217
Etherton, M., Földy, C., Sharma, M., Tabuchi, K., Liu, X., Shamloo, M., et al. (2011). Autism-linked neuroligin-3 R451C mutation differentially alters hippocampal and cortical synaptic function. Proc. Natl. Acad. Sci. USA 108, 13764–13769. doi: 10.1073/pnas.1111093108
Feng, D., Wang, W., Dong, Y., Wu, L., Huang, J., Ma, Y., et al. (2014). Ceftriaxone alleviates early brain injury after subarachnoid hemorrhage by increasing excitatory amino acid transporter 2 expression via the PI3K/Akt/NF-κB signaling pathway. Neuroscience 268, 21–32. doi: 10.1016/j.neuroscience.2014.02.053
Fernell, E., Bejerot, S., Westerlund, J., Miniscalco, C., Simila, H., Eyles, D., et al. (2015). Autism spectrum disorder and low vitamin D at birth: a sibling control study. Mol. Autism. 6:3. doi: 10.1186/2040-2392-6-3
Friard, O., and Gamba, M. (2016). BORIS: a free, versatile open-source event-logging software for video/audio coding and live observations. Methods Ecol. Evol. 7, 1325–1330. doi: 10.1111/2041-210X.12584
Furukawa, M., Tsukahara, T., Tomita, K., Iwai, H., Sonomura, T., Miyawaki, S., et al. (2017). Neonatal maternal separation delays the GABA excitatory-to-inhibitory functional switch by inhibiting KCC2 expression. Biochem. Biophys. Res. Commun. 493, 1243–1249. doi: 10.1016/j.bbrc.2017.09.143
Gandal, M. J., Sisti, J., Klook, K., Ortinski, P. I., Leitman, V., Liang, Y., et al. (2012). GABAB-mediated rescue of altered excitatory-inhibitory balance, gamma synchrony and behavioral deficits following constitutive NMDAR-hypofunction. Transl. Psychiatry 2:e142. doi: 10.1038/tp.2012.69
Ghanizadeh, A. (2013). Increased glutamate and homocysteine and decreased glutamine levels in autism: a review and strategies for future studies of amino acids in autism. Dis. Markers 35, 281–286. doi: 10.1155/2013/536521
Ghanizadeh, A., and Berk, M. (2015). Beta-lactam antibiotics as a possible novel therapy for managing epilepsy and autism, a case report and review of literature. Iran J Child Neurol. 9, 99–102. doi: 10.22037/ijcn.v9i1.5493
Gong, Z. L., Luo, C. M., Wang, L., Shen, L., Wei, F., Tong, R. J., et al. (2014). Serum 25-hydroxyvitamin D levels in Chinese children with autism spectrum disorders. Neuroreport 25, 23–27. doi: 10.1097/WNR.0000000000000034
Guo, D., Peng, Y., and Wang, L. (2021). Autism-like social deficit generated by Dock4 deficiency is rescued by restoration of Rac1 activity and NMDA receptor function. Mol. Psychiatry 26, 1505–1519. doi: 10.1038/s41380-019-0472-7
Halicka, H. D., Zhao, H., Li, J., Traganos, F., Studzinski, G. P., and Darzynkiewicz, Z. (2012). Attenuation of constitutive DNA damage signaling by 1,25-dihydroxyvitamin D3. Aging (Albany NY) 4, 270–278. doi: 10.18632/aging.100450
Hamze, M., Brier, C., Buhler, E., Zhang, J., Medina, I., and Porcher, C. (2024). Regulation of neuronal chloride homeostasis by pro- and mature brain-derived neurotrophic factor (BDNF) via KCC2 cation–chloride cotransporters in rat cortical neurons. Int. J. Mol. Sci. 25:6253. doi: 10.3390/ijms25116253
Han, S., Tai, C., Jones, C. J., Scheuer, T., and Catterall, W. A. (2014). Enhancement of inhibitory neurotransmission by GABAA receptors having α2,3-subunits ameliorates behavioral deficits in a mouse model of autism. Neuron 81, 1282–1289. doi: 10.1016/j.neuron.2014.01.016
Han, S., Tai, C., Westenbroek, R. E., Yu, F. H., Cheah, C. S., Potter, G. B., et al. (2012). Autistic-like behaviour in Scn1a+/− mice and rescue by enhanced GABA-mediated neurotransmission. Nature 489, 385–390. doi: 10.1038/nature11356
Harada, M., Taki, M. M., Nose, A., Kubo, H., Mori, K., Nishitani, H., et al. (2011). Non-invasive evaluation of the GABAergic/glutamatergic system in autistic patients observed by MEGA-editing proton MR spectroscopy using a clinical 3 tesla instrument. J. Autism Dev. Disord. 41, 447–454. doi: 10.1007/s10803-010-1065-0
Heubl, M., Zhang, J., Pressey, J. C., al Awabdh, S., Renner, M., Gomez-Castro, F., et al. (2017). GABAA receptor dependent synaptic inhibition rapidly tunes KCC2 activity via the cl−-sensitive WNK1 kinase. Nat. Commun. 8:1776. doi: 10.1038/s41467-017-01749-0
Ho, S. C., Hsu, C. C., Pawlak, C. R., Tikhonova, M. A., Lai, T. J., Amstislavskaya, T. G., et al. (2014). Effects of ceftriaxone on the behavioral and neuronal changes in an MPTP-induced Parkinson's disease rat model. Behav. Brain Res. 268, 177–184. doi: 10.1016/j.bbr.2014.04.022
Jacob, T. C. (2019). Neurobiology and therapeutic potential of α5-GABA type a receptors. Front. Mol. Neurosci. 12:179. doi: 10.3389/fnmol.2019.00179
Jagota, S. K., and Dani, H. M. (1982). A new colorimetric technique for the estimation of vitamin C using Folin phenol reagent. Anal. Biochem. 127, 178–182. doi: 10.1016/0003-2697(82)90162-2
Kaila, K., Price, T. J., Payne, J. A., Puskarjov, M., and Voipio, J. (2014). Cation-chloride cotransporters in neuronal development, plasticity and disease. Nat. Rev. Neurosci. 15, 637–654. doi: 10.1038/nrn3819
Khera, R., Mehan, S., Bhalla, S., Kumar, S., Alshammari, A., Alharbi, M., et al. (2022). Guggulsterone mediated JAK/STAT and PPAR-gamma modulation prevents neurobehavioral and neurochemical abnormalities in propionic acid-induced experimental model of autism. Molecules 27:889. doi: 10.3390/molecules27030889
Lam, P., Newland, J., Faull, R. L. M., and Kwakowsky, A. (2023). Cation-chloride cotransporters KCC2 and NKCC1 as therapeutic targets in neurological and neuropsychiatric disorders. Molecules 28:1344. doi: 10.3390/molecules28031344
Lawler, C. P., Croen, L. A., Grether, J. K., and Van De Water, J. (2004). Identifying environmental contributions to autism: provocative clues and false leads. Ment. Retard. Dev. Disabil. Res. Rev. 10, 292–302. doi: 10.1002/mrdd.20043
Li, W. (2022). Excitation and inhibition imbalance in Rett syndrome. Front. Neurosci. 16:825063. doi: 10.3389/fnins.2022.825063
Lo, S. C., Scearce-Levie, K., and Sheng, M. (2016). Characterization of social behaviors in caspase-3 deficient mice. Sci. Rep. 6:18335. doi: 10.1038/srep18335
Long, J., Li, H., Liu, Y., Liao, X., Tang, Z., Han, K., et al. (2024). Insights into the structure and function of the hippocampus: implications for the pathophysiology and treatment of autism spectrum disorder. Front. Psychol. 15:1364858. doi: 10.3389/fpsyt.2024.1364858
Mirza, R., and Sharma, B. (2018). Selective modulator of peroxisome proliferator-activated receptor-α protects propionic acid induced autism-like phenotypes in rats. Life Sci. 214, 106–117. doi: 10.1016/j.lfs.2018.10.045
Möhler, H. (2012). The GABA system in anxiety and depression and its therapeutic potential. Neuropharmacology 62, 42–53. doi: 10.1016/j.neuropharm.2011.08.040
Moore, Y. E., Conway, L. C., Wobst, H. J., Brandon, N. J., Deeb, T. Z., and Moss, S. J. (2019). Developmental regulation of KCC2 phosphorylation has Long-term impacts on cognitive function. Front. Mol. Neurosci. 12:173. doi: 10.3389/fnmol.2019.00173
Mostafa, G. A., and Al-Ayadhi, L. Y. (2012). Reduced serum concentrations of 25-hydroxy vitamin D in children with autism: relation to autoimmunity. J. Neuroinflammation 9:201. doi: 10.1186/1742-2094-9-201
Nizzardo, M., Nardini, M., Ronchi, D., Salani, S., Donadoni, C., Fortunato, F., et al. (2011). Beta-lactam antibiotic offers neuroprotection in a spinal muscular atrophy model by multiple mechanisms. Exp. Neurol. 229, 214–225. doi: 10.1016/j.expneurol.2011.01.017
O'Donovan, S. M., Sullivan, C. R., and McCullumsmith, R. E. (2017). The role of glutamate transporters in the pathophysiology of neuropsychiatric disorders. NPJ Schizophr. 3:32. doi: 10.1038/s41537-017-0037-1
Page, L. A., Daly, E., and Schmitz, N. (2006). In vivo 1H-magnetic resonance spectroscopy study of amygdala-hippocampal and parietal regions in autism. Am. J. Psychiatry 163, 2189–2192. doi: 10.1176/ajp.2006.163.12.2189
Parrella, N. F., Hill, A. T., Dipnall, L. M., Loke, Y. J., Enticott, P. G., and Ford, T. C. (2024). Inhibitory dysfunction and social processing difficulties in autism: a comprehensive narrative review. J. Psychiatr. Res. 169, 113–125. doi: 10.1016/j.jpsychires.2023.11.014
Patrick, R. P., and Ames, B. N. (2014). Vitamin D hormone regulates serotonin synthesis. Part 1: relevance for autism. FASEB J. 28, 2398–2413. doi: 10.1096/fj.13-246546
Pertile, R. A. N., Cui, X., Hammond, L., and Eyles, D. W. (2018). Vitamin D regulation of GDNF/ret signaling in dopaminergic neurons. FASEB J. 32, 819–828. doi: 10.1096/fj.201700713R
Polleux, F., and Lauder, J. M. (2004). Toward a developmental neurobiology of autism. Ment. Retard. Dev. Disabil. Res. Rev. 10, 303–317. doi: 10.1002/mrdd.20044
Rothstein, J. D., Patel, S., Regan, M. R., Haenggeli, C., Huang, Y. H., Bergles, D. E., et al. (2005). Beta-lactam antibiotics offer neuroprotection by increasing glutamate transporter expression. Nature 433, 73–77. doi: 10.1038/nature03180
Rubenstein, J. L., and Merzenich, M. M. (2003). Model of autism: increased ratio of excitation/inhibition in key neural systems. Genes Brain Behav. 2, 255–267. doi: 10.1034/j.1601-183x.2003.00037.x
Ruiz-Larrea, M. B., Leal, A. M., Liza, M., Lacort, M., and de Groot, H. (1994). Antioxidant effects of estradiol and 2-hydroxyestradiol on iron-induced lipid peroxidation of rat liver microsomes. Steroids 59, 383–388. doi: 10.1016/0039-128x(94)90006-x
Ryan, R. M., Ingram, S. L., and Scimemi, A. (2021). Regulation of glutamate, GABA and dopamine transporter uptake, surface mobility and expression. Front. Cell. Neurosci. 15:670346. doi: 10.3389/fncel.2021.670346
Sahin, K., Orhan, C., Karatoprak, S., Tuzcu, M., Deeh, P. B. D., Ozercan, I. H., et al. (2022). Therapeutic effects of a novel form of biotin on propionic acid-induced autistic features in rats. Nutrients 14:1280. doi: 10.3390/nu14061280
Salim, S. (2017). Oxidative stress and the central nervous system. J. Pharmacol. Exp. Ther. 360, 201–205. doi: 10.1124/jpet.116.237503
Sauer, A. K., Stanton, J. E., Hans, S., and Grabrucker, A. M. (2021). “Autism Spectrum disorders: etiology and pathology” in Autism Spectrum Disorders. ed. A. M. Grabrucker (Brisbane (AU): Exon Publications).
Schwartzer, J. J., Careaga, M., Onore, C. E., Rushakoff, J. A., Berman, R. F., and Ashwood, P. (2013). Maternal immune activation and strain specific interactions in the development of autism-like behaviors in mice. Transl. Psychiatry 3:e240. doi: 10.1038/tp.2013.16
Sever, I. H., Ozkul, B., Bozkurt, M. F., and Erbas, O. (2022). Therapeutic effect of finasteride through its antiandrogenic and antioxidant role in a propionic acid-induced autism model: demonstrated by behavioral tests, histological findings and MR spectroscopy. Neurosci. Lett. 779:136622. doi: 10.1016/j.neulet.2022.136622
Sharma, A. R., Batra, G., Saini, L., Sharma, S., Mishra, A., Singla, R., et al. (2022). Valproic acid and propionic acid modulated mechanical pathways associated with autism Spectrum disorder at prenatal and neonatal exposure. CNS Neurol. Disord. Drug Targets 21, 399–408. doi: 10.2174/1871527320666210806165430
Silverman, J. L., Pride, M. C., Hayes, J. E., Puhger, K. R., Butler-Struben, H. M., Baker, S., et al. (2015). GABAB receptor agonist R-baclofen reverses social deficits and reduces repetitive behavior in two mouse models of autism. Neuropsychopharmacology 40, 2228–2239. doi: 10.1038/npp.2015.66
Staal, W. G., de Krom, M., and de Jonge, M. V. (2012). Brief report: the dopamine-3-receptor gene (DRD3) is associated with specific repetitive behavior in autism spectrum disorder (ASD). J. Autism Dev. Disord. 42, 885–888. doi: 10.1007/s10803-011-1312-z
Tabouy, L., Getselter, D., Ziv, O., Karpuj, M., Tabouy, T., Lukic, I., et al. (2018). Dysbiosis of microbiome and probiotic treatment in a genetic model of autism spectrum disorders. Brain Behav. Immun. 73, 310–319. doi: 10.1016/j.bbi.2018.05.015
Takahashi, K., Foster, J. B., and Lin, C. L. (2015). Glutamate transporter EAAT2: regulation, function, and potential as a therapeutic target for neurological and psychiatric disease. Cell. Mol. Life Sci. 72, 3489–3506. doi: 10.1007/s00018-015-1937-8
Tsukahara, T., Masuhara, M., Iwai, H., Sonomura, T., and Sato, T. (2016). The effect of repeated stress on KCC2 and NKCC1 immunoreactivity in the hippocampus of female mice. Data Brief 6, 521–525. doi: 10.1016/j.dib.2015.12.041
Usui, N., Kobayashi, H., and Shimada, S. (2023). Neuroinflammation and oxidative stress in the pathogenesis of autism Spectrum disorder. Int. J. Mol. Sci. 24:5487. doi: 10.3390/ijms24065487
Vinkhuyzen, A. A. E., Eyles, D. W., Burne, T. H. J., Blanken, L. M. E., Kruithof, C. J., Verhulst, F., et al. (2018). Gestational vitamin D deficiency and autism-related traits: the generation R study. Mol. Psychiatry 23, 240–246. doi: 10.1038/mp.2016.213
Wang, J., Huang, H., Liu, C., Zhang, Y., Wang, W., Zou, Z., et al. (2022). Research Progress on the role of vitamin D in autism Spectrum disorder. Front. Behav. Neurosci. 16:859151. doi: 10.3389/fnbeh.2022.859151
World Health Organization. Autism spectrum disorders. (2021). Available at: https://www.who.int/news-room/fact-sheets/detail/autism-spectrum-disorders (Accessed September 21, 2024).
Yamada, T., Kawahara, K., Kosugi, T., and Tanaka, M. (2006). Nitric oxide produced during sublethal ischemia is crucial for the preconditioning-induced down-regulation of glutamate transporter GLT-1 in neuron/astrocyte co-cultures. Neurochem. Res. 31, 49–56. doi: 10.1007/s11064-005-9077-4
Keywords: autism spectrum disorder, excitatory-inhibitory balance, oxidative stress, EAAT2, KCC2, NKCC1, vitamin D3, GABRA5
Citation: Alabdali AN, Ben Bacha A, Alonazi M, Abuaish S, Almotairi A, Al-Ayadhi L and El-Ansary AK (2025) Impact of GABA and nutritional supplements on neurochemical biomarkers in autism: a PPA rodent model study. Front. Mol. Neurosci. 18:1553438. doi: 10.3389/fnmol.2025.1553438
Received: 30 December 2024; Accepted: 03 March 2025;
Published: 18 March 2025.
Edited by:
Mazahir T. Hasan, IKERBASQUE Basque Foundation for Science, SpainReviewed by:
Laia Guardia-Escote, University of Barcelona, SpainCopyright © 2025 Alabdali, Ben Bacha, Alonazi, Abuaish, Almotairi, Al-Ayadhi and El-Ansary. This is an open-access article distributed under the terms of the Creative Commons Attribution License (CC BY). The use, distribution or reproduction in other forums is permitted, provided the original author(s) and the copyright owner(s) are credited and that the original publication in this journal is cited, in accordance with accepted academic practice. No use, distribution or reproduction is permitted which does not comply with these terms.
*Correspondence: Afaf K. El-Ansary, YWZhZmtlbGFuc2FyeUBnbWFpbC5jb20=; Abir Ben Bacha, YWFsZ2hhbm91Y2dpQGtzdS5lZHUuc2E=
Disclaimer: All claims expressed in this article are solely those of the authors and do not necessarily represent those of their affiliated organizations, or those of the publisher, the editors and the reviewers. Any product that may be evaluated in this article or claim that may be made by its manufacturer is not guaranteed or endorsed by the publisher.
Research integrity at Frontiers
Learn more about the work of our research integrity team to safeguard the quality of each article we publish.