- 1Department of Neurosurgery, Affiliated Hospital of North Sichuan Medical College, Nanchong, China
- 2School of Clinical Medicine, North Sichuan Medical College, Nanchong, China
- 3School of Dentistry, North Sichuan Medical College, Nanchong, China
- 4Department of Neurosurgery, the First Affiliated Hospital of Chongqing Medical University, Chongqing, China
- 5Department of Neurosurgery, West China Hospital, Sichuan University, Chengdu, China
Hydrocephalus is a neurological condition caused by aberrant circulation and/or obstructed cerebrospinal fluid (CSF) flow after cerebral ventricle abnormal dilatation. In the past 50 years, the diagnosis and treatment of hydrocephalus have remained understudied and underreported, and little progress has been made with respect to prevention or treatment. Further research on the pathogenesis of hydrocephalus is essential for developing new diagnostic, preventive, and therapeutic strategies. Various genetic and molecular abnormalities contribute to the mechanisms of hydrocephalus, including gene deletions or mutations, the activation of cellular inflammatory signaling pathways, alterations in water channel proteins, and disruptions in iron metabolism. Several studies have demonstrated that modulating the expression of key proteins, including TGF-β, VEGF, Wnt, AQP, NF-κB, and NKCC, can significantly influence the onset and progression of hydrocephalus. This review summarizes and discusses key mechanisms that may be involved in the pathogenesis of hydrocephalus at both the genetic and molecular levels. While obstructive hydrocephalus can often be addressed by removing the obstruction, most cases require treatment strategies that involve merely slowing disease progression by correcting CSF circulation patterns. There have been few new research breakthroughs in the prevention and treatment of hydrocephalus.
1 Introduction
Hydrocephalus is a common neurological condition and is defined as the progressive distension of the brain ventricular system induced by a disorder of cerebrospinal fluid (CSF) microcirculatory homeostasis and characterized by an abnormal accumulation of CSF (Kahle et al., 2016; Hochstetler et al., 2022). Hydrocephalus is typically classified as either obstructive hydrocephalus or communicating hydrocephalus based on to the characteristics of CSF circulation. While obstructive hydrocephalus can often be resolved by removing the obstruction (Mekbib et al., 2023), other forms of hydrocephalus must be treated by slowing disease progression and correcting CSF circulation patterns. However, there have been few significant clinical breakthroughs in the treatment of the underlying causes of hydrocephalus, likely due to the condition’s insidious and complex etiology.
Hydrocephalus can also be categorized as congenital, acquired, or idiopathic normal pressure hydrocephalus based on its underlying cause (Rekate, 2009). Specifically, the pathogenesis of congenital hydrocephalus, which is often linked to aqueductal stenosis, involves various molecular changes that are associated with genes that regulate brain growth and development, with approximately 40% of congenital hydrocephalus cases having a genetic origin (Kundishora et al., 2021; Duy et al., 2022; Zhang et al., 2006). Most cases of secondary hydrocephalus may be attributed to a single primary cause, such as stroke, traumatic brain injury, brain tumor, infection, or craniectomy, involving multiple pathological processes, such as abnormal cerebrospinal fluid secretion and absorption, abnormal subarachnoid circulation, and decreased cerebral venous compliance (Xu, 2016; Chen et al., 2017). These pathological changes are also involved in signaling pathways such as inflammation, fibrosis, ion and transport channels, and vascular injury and reconstruction (Yamashiro, 2022; Huang et al., 2023; Toft-Bertelsen et al., 2022; Claassen and Park, 2022). Moreover,although a variety of hydrocephalus-related proteins have been found to be associated with idiopathic hydrocephalus, the etiology of idiopathic hydrocephalus has yet to be fully elucidated (Ishida et al., 2023).
In recent years, advances in research on the genetic and molecular mechanisms of hydrocephalus have led to the development of drugs and gene therapies targeting these mechanisms, which have shown promising effects in preclinical studies. These findings suggest that focusing on these genetic loci and molecular targets could be a potential approach for improving the clinical treatment of hydrocephalus (Davy and Robinson, 2003). In this review, we focus on the genetic and molecular changes involved in the pathogenesis of hydrocephalus in studies in animals and humans, and we discuss the potential value of these molecules in terms of diagnosing and treating hydrocephalus.
2 Genetic disorders of hydrocephalus
A substantial body of research has identified numerous genetic abnormalities associated with hydrocephalus, and the various genetic loci have been identified through studies conducted on animal models of hydrocephalus (Table 1). In recent years, with the development of genomics and molecular biology technologies, significant breakthroughs have been made in the study of genes associated with congenital hydrocephalus in humans (Table 2). Genetically abnormal hydrocephalus models exhibit histomorphologic alterations that closely resemble those observed in human congenital hydrocephalus, making these models valuable tools for investigating the genetic and pathological mechanisms underlying this condition. The majority of current research on genetic abnormalities associated with hydrocephalus has been conducted in rodent models, particularly rats and mice. The key genetic alterations identified in these models have been further validated in other animal systems. These animal models of hydrocephalus not only share significant histopathological features with human cases but also provide critical insights into the genetic and pathogenic processes contributing to brain injury. Compared with human models, animal models of hydrocephalus present many histopathological features, thus providing critical insights into the genetic and pathogenic processes contributing to brain injury (Zhang et al., 2006).
2.1 Genetic loci in mice with hydrocephalus
In the mouse model, three quantitative trait loci (QTL) have been identified on chromosome (Chr) 8, Chr 4, and Chr 7; these loci were labeled Vent8a, Vent4b, and Vent7c, respectively (Zygourakis and Rosen, 2003). Vent8a, which is located on Chr 8 close to the markers D8Mit94 and D8Mit189, is the major QTL that controls variance in ventricular size. Vent4b, which is located on Chr 4 near D4Mit237 and D4Mit214, and Vent7c, which is located on Chr 7 between D7Mit178 and D7Mit191, affect ventricular size in the developing embryo.
Mouse models that have been reported to have congenital hydrocephalus include congenital hydrocephalus-1 (hy1), hydrocephalus-2 (hy2), hydrocephalus-3 (hy3), spontaneous congenital hydrocephalus (ch), hydrocephalus and hop gait (Hyh), hemorrhagic hydrocephalus (Hhy), and obstructive hydrocephalus (oh). Hy1, hy2, and hy3 mice are autosomal recessive. Hy1 and hy2 mice are extinct, and no defective locus has been identified. These two strains share similar phenotypic characteristics, including dilation of the entire ventricular system within the first two weeks of life, which is potentially linked to conduit closure (Zhang et al., 2006; Kousi and Katsanis, 2016; Raimondi et al., 1976). Additionally, the oh strain, which carries an unidentified genetic locus, is autosomal recessive. In oh mice, the enlarged cerebral hemispheres compress the midbrain, leading to aqueductal occlusion and subsequent stenosis, ultimately culminating in obstructive hydrocephalus. Electron microscopy has revealed severe damage to subventricular cells and white matter, along with detachment of the ventricular meninges (Borit and Sidman, 1972). In contrast, a more extensive genetic analysis of hy3 mice revealed a mutation in the Bdnf gene on chromosome 8 of the transgenic OVE459 mouse strain. The insertion of a transgene resulted in an exonic rearrangement of the Hydin gene in OVE459 mice. Furthermore, a single CG base pair deletion in exon 15 of the Hydin gene was discovered in hy3 mice carrying a spontaneous hy3 mutant allele. The Hydin protein, which is homologous to the actin-binding protein Caldesmon, is expressed specifically in the ciliated ependymal cell layer of the lateral, third, and fourth ventricles in hy3 mice. It plays a crucial role in the formation, function, or maintenance of cilia, cilia-like structures, and ciliated epithelium (Davy and Robinson, 2003; Robinson et al., 2002; Lechtreck et al., 2008). Experimental evidence indicates an accumulation of lipids in the choroid plexus and ventricular membrane cells of hy3 mice, implicating a potential role for Hydin in maintaining cellular homeostasis and/or signaling processes (Lawson and Raimondi, 1973). Hydin is also essential for ciliary motility, suggesting that impaired CSF flow due to reduced ciliary pulsation may serve as a primary initiating factor for hydrocephalus. Alternatively, ciliary dysfunction may contribute to alterations in the ventricular layer and subsequent changes in CSF production (Dawe et al., 2007).
Congenital hydrocephalus (ch) mice with autosomal recessive mutations have been shown to have a mutation in Foxc1 (Mf1) on mouse chromosome 13, which is a member of the conserved forkhead/winged helix transcription factor gene family expressed in embryonic tissues (Kume et al., 1998). Hydrocephalus in ch mice with Foxc1 mutations has been shown to be associated with multiple developmental defects, including abnormal differentiation of arachnoid cells in the meninges and the absence of certain skull bones (Hong et al., 1999). Interestingly, the deletion of several genes related to the structure and function of ventricular meningeal cilia, such as Mdnah5 (Ibanez-Tallon et al., 2002), Spa6 (Sapiro et al., 2002), and Rsph9 (Zou et al., 2020), which cause hydrocephalus in other mouse models, has not been implicated in congenital hydrocephalus.
A mouse model with autosomal recessive hydrocephalus and a characteristic hop gait (Hyh) exhibits ventricular enlargement and abnormal locomotion at birth. Hyh mice are characterized by marked ventricular dilatation, a small cerebral cortex, an interhemispheric cyst arising from the third ventricle, agenesis of the corpus callosum, and abnormal neural cell development; these mice eventually die due to worsening hydrocephalus (Batiz et al., 2006; Chae et al., 2004; Rodríguez-Pérez et al., 2024).This model is frequently utilized to investigate the pathogenesis of obstructive congenital hydrocephalus (Bronson and Lane, 1990). The Hyh gene, located on chromosome 7 near the Gpi-1 (glucose phosphate isomerase-1) locus, has been identified as Napa, a gene critical for the normal development of the neuroepithelium lining the ventricles. Mutations in Napa result in midbrain aqueduct obstruction by postnatal day 1, leading to severe hydrocephalus (Jiménez et al., 2001; Wagner et al., 2003). This obstruction triggers a cascade of neuropathological events, including myelin degeneration, glial activation, excitotoxic neurochemical environments, and edema (Páez et al., 2007; García-Bonilla et al., 2018); The Napa gene encodes the soluble N-ethylmaleimide-sensitive factor (NSF) attachment protein α (α-SNAP), which is essential for cell membrane fusion. Mutations in α-SNAP in Hyh mutants cause defects in vesicular transport, leading to pronounced abnormalities in F-actin organization, as well as in the distribution of α-connexin, β-connexin, and E-cadherin (Chae et al., 2004; Rodríguez-Pérez et al., 2024), and the disorder displays 100% penetrance, with the mutation present only in affected mice (Hong et al., 2004; Batiz et al., 2009; Chae et al., 2002).
Another mouse model of congenital hydrocephalus is the hemorrhagic hydrocephalus (Hhy) model. Homozygous Hhy mutants, which follow an autosomal recessive inheritance pattern, are characterized by intracranial hemorrhage, hydrocephalus, and subcortical heterotopia. Notably, these mice exhibit no histological abnormalities in the subarachnoid space or the choroid plexus. The Hhy gene locus has been mapped to mouse chromosome 12, and evidence suggests that Ccdc85c, located within a 1-Mb region between the D12Mit28 and D12Nds2 markers on chromosome 12, may be genetically disrupted in Hhy mutants (Mori et al., 2012; Express Group, 2010). Genetic deletion of Rho family guanosine triphosphatase 3 (Rnd3) and regulation of Notch signaling activity, resulting in the overgrowth of aqueduct ependymal cells, has been shown to be associated with aqueductal stenosis, which is a significant factor in congenital hydrocephalus (Lin et al., 2013). Therefore, inhibition of the Notch signaling pathway may be an effective target for treating hydrocephalus. Genetic studies on hydrocephalus in mice have provided a wealth of molecular insights into the pathogenesis of congenital hydrocephalus. These studies have significantly deepened our understanding of the related genetic factors, laying a solid foundation for uncovering the mechanisms behind hydrocephalus. Furthermore, findings from mouse models offer potential directions for the early diagnosis and personalized treatment of hydrocephalus in the future. In-depth translational research on these genes holds the promise of providing new clinical strategies, thereby advancing the precision diagnosis and treatment of congenital hydrocephalus.
2.2 Rats with hydrocephalus
Significant strains of congenital hydrocephalus include the Texas strain (HTX) and LEW/Jms in rats. Enlargement of the ventricular system occurs in HTX rats during late gestation, resulting from the closure of the cerebral aqueducts and a reduction in the secretory cells of the subcommissural organ (SCO). The SCO is a circumventricular organ located in the dorsal aspect of the cerebral aqueduct and is the source of sialylated glycoproteins that form Reissner’s fibers (RFs) and remain CSF-soluble (Ortloff et al., 2013). Moreover, SCO-spondin is a specific glycoprotein associated with neuronal maturation in the developing brain and has been shown to be correlated with both aqueduct stenosis and enlarged lateral ventricle size in HTX rats (Zhang et al., 2006; Chae et al., 2002).
QTL mapping of the progeny of a backcross of HTX rats with the nonhydrocephalic Fischer F344 strain revealed four loci for hydrocephalus on Chr 9 (peak markers D9Rat2), 10 (between markers D10Rat136 and D10Rat135), 11 (peak markers D11Arb2 and D11Rat46) and 17 (peak markers D17mit4 and D17Rat154) (Zhang et al., 2006; Jones et al., 2004). The Chr 9 locus closest to the TGIF (or the 5 V-TG-3 V interacting factor) encodes a gene that modulates the transforming growth factor-β (TGF-β) signaling pathway. TGF-β1 is a fiber factor that is associated with several fibrotic diseases and is significantly elevated in HTX rats (Li et al., 2005) In addition, the overexpression of TGF-β1 leads to fibrosis of the soft brain and arachnoid membranes as well as collagen deposition in the extracellular matrix (ECM) of the subarachnoid space (Yan et al., 2016; Yang et al., 2022). A further gene array study in the midbrain region of HTX rats with congenital hydrocephalus suggested that abnormal expression of cholecystokinin (Cck), nuclear factor 1/X (Nfix), three galactose-binding soluble lectins (Lgals3), glutathione s-transferase a type (Gsta1), Xdh (xanthine dehydrogenase), a tissue factor pathway inhibitor (Tfpi-2) and the fork-head transcription factor BF-1 (Fkhr) may be associated with hydrocephalus (Miller et al., 2006). Recently, Wagner et al. (2003) performed copy number analysis on H-Tx rats, revealing the pathophysiological mechanisms by which abnormal Ptpn20 gene expression is associated with the development of hydrocephalus in HTX rats. The expression of Ptpn20 mRNA was significantly lower in hydrocephalic HTX rats than in non-hydrocephalic HTX rats. In contrast, the expression of phosphorylated Na-K-Cl cotransporter 1 (pNKCC1) in the choroid plexus was significantly increased in mice with Ptpn20 gene deletion, suggesting that the overexpression of pNKCC1 on the epithelial cells of the cerebral choroid plexus, which results in excessive cerebrospinal fluid secretion, may be involved in hydrocephalus in HTX rats.
Folate is an essential nutrient for multiple metabolic pathways, and in the brain, only 5-methyl tetrahydrofolate (5mTHF) can freely cross the blood–brain barrier (Bailey and Gregory, 1999; Dunlevy et al., 2006; Pietrzik et al., 2010). It has been shown that disruptions in folate metabolism and methylation, particularly in male H-Tx rats, may contribute to the development and inheritance of hydrocephalus. Furthermore, bioactive folic acid has been demonstrated to significantly reduce the risk of hydrocephalus in these rats by modulating DNA methylation (Mori et al., 2012). HTX rats with hydrocephalus exhibit a decrease in hepatic and cerebral nuclear FDH and parallel increases in hepatic nuclear methylfolate and cerebral methylfolate at postnatal ages 5, 15, and 20. In parallel with the increase in folate-binding proteins and enzymes, 10-formyltetrahydrofolate dehydrogenase (FDH) fails to be secreted, resulting in the inability of cortical cells to access the available 5mTHF in cerebrospinal fluid supplemented with THF or 5fTHF (Jimenez et al., 2019). Another related study revealed that in in vitro culture, when cells were soaked with CSF containing high 5mTHF/FRa and low FDH levels, the growth of arachnoid tissue was overstimulated, leading to dysfunction of arachnoid tissue (Naz et al., 2016) and suggesting that CSF folate imbalance may also induce congenital hydrocephalus. LEW/Jms rats exhibit strains similar to those of HTX rats, and the inheritance of hydrocephalus in these rats may be autosomal recessive or semidominant. Nevertheless, none of the loci have been identified (Jones et al., 2003; Sasaki et al., 1983).
However, it is important to note that while these studies provide valuable insights, relevant genetic investigations have not yet been conducted to fully elucidate the underlying mechanisms. Future studies should focus on identifying specific genetic variants related to folate metabolism and their potential role in the inheritance of hydrocephalus, as well as exploring therapeutic strategies targeting folate signaling pathways.
2.3 Genetic alterations in hydrocephalus in other animals
Zebrafish genes share approximately 70% homology with human genes, making them highly amenable to genetic analysis and editing. Therefore, zebrafish are frequently used as model organisms to study the development of hereditary diseases affecting the ventricular system (Howe et al., 2013). Knockdown of the L1camb gene in zebrafish through the injection of control morpholinos or morpholinos targeting the splicing or translation of L1camb mRNA leads to axonal outgrowth defects and myelination abnormalities, ultimately resulting in hydrocephalus (Linneberg et al., 2019). Additionally, the camel gene has also been closely linked to hydrocephalus (Yang et al., 2021). Knocking out the wdr16 gene via antisense Morpholino injection induces hydrocephalus in zebrafish, although these animals still present with intact ciliary motility and no significant changes in the ventricular laminae, thus suggesting that the wdr16 gene plays a role in cilia-mediated cell polarization (Hirschner et al., 2007). The Atp1a3 gene, which is associated with Na+/K+ ATPase, is strongly correlated with hydrocephalus. Targeted knockdown of Atp1a3a or Atp1a3b results in abnormal dilation of the cerebral ventricles in zebrafish, likely due to ionic imbalance across the plasma membrane, which leads to the accumulation of cerebrospinal fluid in the ventricles (Allocco et al., 2019). Furthermore, knockout and mutation of the lgi1b gene cause severe hydrocephalus and developmental brain defects, including apoptosis, in zebrafish; however, the exact mechanism remains unclear (Teng et al., 2011). By knocking down or overexpressing DIPA (a family consisting of Ccdc85a, Ccdc85b, and Ccdc85c), the interaction of DIPA with p120 is attenuated, leading to subcortical heterotopia and hemorrhagic hydrocephalus. This result is similar to the mechanism in mice with hemorrhagic hydrocephalus caused by the Ccdc85c mutation (Markham et al., 2014). In addition, combined gene knockouts can lead to hydrocephalus in zebrafish, and combined knockouts of the calb2a and calb2b genes lead to severe hydrocephalus, which may be associated with cilia (Bhoyar et al., 2019). Hydrocephalus is also frequently observed in zebrafish models of other diseases, such as when the dcdc2 or slc41a1 genes, along with other NPHP genes, are knocked down to model renal cysts (Schueler et al., 2015; Hurd et al., 2013). Animals such as primates, pigs, dogs, and rabbits are predominantly used to develop models of acquired hydrocephalus; however, research in these species is often constrained by ethical considerations, individual differences, and other factors. Therefore, genetic interventions are less commonly studied in these animals. The genes associated with hydrocephalus identified in previous studies require further validation in these animals (particularly those with complex cortical structures) to provide more comprehensive insights. Although the zebrafish hydrocephalus model shares certain similarities with humans in terms of genes, brain structure, and cellular features, significant differences exist in their ventricular structures. Unlike mammals, the zebrafish brain ventricular system consists of only three cavities, lacking the Sylvian aqueduct that connects the third and fourth ventricles. Additionally, only two of these cavities meet the morphological criteria for ventricles. Therefore, there remains considerable debate regarding the efficacy of the zebrafish hydrocephalus model as a model for studying human hydrocephalus (Mogi et al., 2012; Wang et al., 2024). Moreover, there are differences in the molecular mechanisms underlying the development of the choroid plexus in humans and zebrafish. Given these considerations, further exploration of the zebrafish ventricle development process and molecular expression differences, as well as the development of highly specific zebrafish models, is crucial for advancing our understanding of the molecular mechanisms involved in the pathogenesis of hydrocephalus.
2.4 Genetic alterations in humans
The genes related to hydrocephalus in humans primarily involve neurodevelopment, cerebrospinal fluid circulation, and the structural and functional regulation of the ventricles and the blood-cerebrospinal fluid barrier. Although numerous genes have been found to be associated with the development of hydrocephalus in animal studies, only six genes have been definitively proven to be closely related to congenital hydrocephalus in humans: L1CAM, AP1S2, MPDZ, FOXJ1, SMARCC1, and CCDC88C. L1CAM encodes the L1 protein, a transmembrane glycoprotein belonging to the immunoglobulin superfamily. It is primarily expressed in neurons during development and plays a critical role in neuronal adhesion, axonal growth and guidance, and myelination. Studies have demonstrated that mutations in the L1CAM gene are a major cause of X-linked hydrocephalus, with these mutations located between the DXS52 and F8C loci. Affected patients often exhibit severe ventricular enlargement accompanied by profound intellectual disabilities and developmental delays (Liu et al., 2024; Ahmed et al., 2023). The AP1S2 gene encodes a subunit of the adaptor protein complex 1 (AP-1), which is critical for vesicle formation and trafficking within the Golgi apparatus. Clinically, mutations in AP1S2 are commonly associated with Fried-Pettigrew syndrome, characterized by hydrocephalus, intellectual disabilities, mild facial anomalies, and basal ganglia calcification (Saillour et al., 2007). Mutations in MPDZ and CCDC88C disrupt cerebrospinal fluid circulation and absorption by affecting cellular structure and function. MPDZ mutations impair intercellular junctions, while CCDC88C mutations compromise cytoskeletal stability and formation, collectively leading to hydrocephalus. Notably, these two genes share significant similarities in their neuropathological manifestations. Studies have shown that the protein encoded by MPDZ directly binds to the DAPLE protein encoded by CCDC88C, acting as a scaffold to promote ependymal cell planar polarity by inhibiting the non-canonical Wnt signaling pathway (Tessier et al., 2023). Heterozygous de novo mutations in the FOXJ1 gene encode a critical forkhead transcription factor essential for the formation of motile cilia (Hou et al., 2023; Wallmeier et al., 2019). These mutations result in ciliopathy, characterized by hydrocephalus and randomized left–right body asymmetry. The SMARCC1 gene encodes a chromatin remodeling protein, SWI/SNF-related matrix-associated actin-dependent chromatin regulator subfamily C member 1 (BAF155). Mutations in SMARCC1 are closely associated with CH phenotypes and neural tube development defects (Jin et al., 2020; Hourvitz et al., 2023). However, beyond these genes, there remain numerous others and their associated pathways that have been only minimally explored. To fully elucidate the molecular mechanisms underlying hydrocephalus, further research is imperative. Whether through the development of relevant animal models or large-scale clinical studies, deeper investigation holds the potential to uncover new genetic insights and therapeutic targets for congenital hydrocephalus. Although numerous genes associated with hydrocephalus have been identified through animal studies, research on the genetic basis of congenital hydrocephalus in humans remains in its early stages. While recent years have seen some breakthroughs, the scope and depth of related studies are still limited. Against this backdrop, large-scale clinical and translational genetic studies hold great promise not only for elucidating the molecular mechanisms underlying congenital hydrocephalus but also for providing more precise targets for prenatal genetic diagnosis in potential cases. These findings lay the foundation for early intervention and risk management in high-risk populations, thereby reducing the incidence of congenital hydrocephalus and significantly improving the prognosis and quality of life for affected children. Meanwhile these advancements could pave the way for the development of targeted interventions and precision medicine approaches, offering new treatment options to reduce the disease burden and improve the outcomes and quality of life for affected patients.
3 Molecular changes in hydrocephalus
Genes play a key role in congenital hydrocephalus, but hydrocephalus are also characterized by abnormal expression levels of proteins that are typically associated with this condition. The impact of altered expression of key proteins in the development of secondary and idiopathic hydrocephalus has been extensively examined, with studies showing that modulating or intervening in the expression of these proteins can significantly influence the progression of hydrocephalus. Among the proteins studied in the field of hydrocephalus are TGF-β, VEGF, Wnt, AQP, NF-κB, and NKCC.
3.1 The transforming growth factor-beta pathway
Transforming growth factor-beta (TGF-β), a 25-kD nonglycosylated homodimer produced by various cell types, is a cytokine that is essential for the induction of the fibrotic response (Peng et al., 2022; Wang et al., 2018). There is strong evidence suggesting that intense contact occurs between TGF-β and hydrocephalus after stroke, especially in subarachnoid hemorrhage (Yang et al., 2022; Lee et al., 2013; Douglas et al., 2009). In the central nervous system (CNS), TGF-β is secreted by astrocytes, neurons, and microglia and has been reported to amplify fibrosis, leading to hydrocephalus in subarachnoid hemorrhage (SAH) (Lee et al., 2013; Robinson and Jantzie, 2022). The TGF-β family mediates signaling by binding to two serine/threonine kinase receptors on the cell surface, TGF-β RI and TGF-β RII. This interaction regulates extracellular matrix remodeling and drives the transition of fibroblasts into myofibroblasts, a critical process in fibrosis (Tzavlaki and Moustakas, 2020; Luo, 2017). In mouse models of SAH, TGF-β is expressed at high levels in CSF, leading to posttraumatic fibrotic scarring and angiogenesis, thereby resulting in chronic communicating hydrocephalus through the TGF-β/samds/CTGF signaling pathway (Figure 1). Dong et al. (2018) found that the expression of TGF-β1 in the CSF and brain parenchyma increased on the 21st day after SAH in a rat model. The protein levels of Smad2/3, pSmad2/3, and CTGF in the superficial tissues of the rat brain were significantly elevated following SAH, a response that was effectively suppressed by ICA II. Notably, TGF-β1 in CSF has been described to exhibit a biphasic response (Yan et al., 2016; Flood et al., 2001). The first peak of TGF-β1 primarily originates from an exogenous pathway, driven by the release of substantial amounts of pre-stored TGF-β1 from platelets during aSAH, which coincides with the process of platelet degranulation (Flood et al., 2001). The second peak is attributed to endogenous production mechanisms, wherein TGF-β1 acts both as a chemokine to attract inflammatory cells and platelets and synergistically interacts with other cytokines to stimulate local production of TGF-β1 in the CSF and choroid plexus (Yan et al., 2016; Kuo and Huang, 2021). Inhibiting the TGF-β1 signaling pathway could therefore mitigate chronic hydrocephalus in the aSAH model. LSKL peptide, a small molecule peptide and competitive antagonist of TGF-β1, suppresses TSP1-mediated TGF-β1 activity, thereby reducing subarachnoid fibrosis, preventing chronic hydrocephalus, and improving long-term neurocognitive outcomes after SAH (Liao et al., 2016). Similarly, Decorin, a natural antagonist of TGF-β, inhibits the downstream pathway by forming a complex with TGF-β and also acts as a competitive inhibitor (Derynck and Budi, 2019; Zhang et al., 2018). In a rat SAH model, Decorin effectively prevented extracellular matrix accumulation, subarachnoid fibrosis, and chronic hydrocephalus by inhibiting the heightened activity of the TGF-β1/Smad/CTGF axis (Yan et al., 2016). Moreover, Zhang et al. confirmed that HGF, MMP-9, and TGF-β1 may participate in the formation and prognosis of hydrocephalus after kaolin injection (Heep et al., 2004; Zhang et al., 2013). Cytologic research by Yue et al. (2016) reported that TGF-β1 activates the p38 signaling pathway in MMCs, which indicates that the p38 pathway is an important signaling pathway through which TGF-β1 induces the expression of CTGF. Studies have shown that the concentrations of TGF-β1 in the CSP of patients who suffer ICH-IVH or IVH-GMH are increased, especially in those with posthemorrhagic hydrocephalus (Whitelaw et al., 1999; Tsitouras and Sgouros, 2011). However, two studies reported that TGF-β inhibitors do not attenuate ventricular dilation after IVH in rats (Tubbs et al., 2003; Tuli et al., 2000). Taken together, the evidence discussed in this section suggests that inhibiting the TGF-β signaling pathway may be a powerful approach for treating hydrocephalus after hemorrhage.
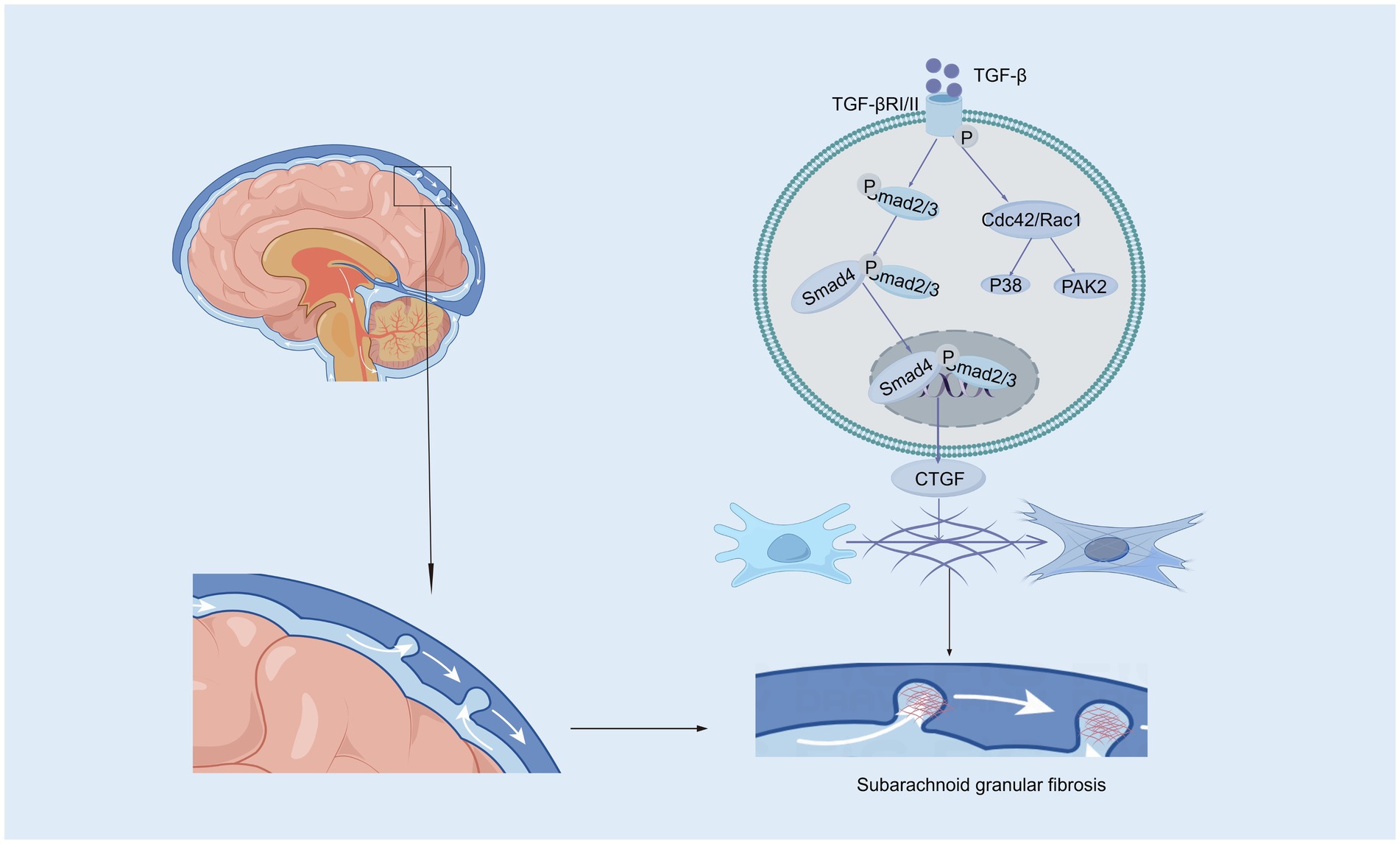
Figure 1. The canonical and non-canonical Smad signaling pathways induced by TGF-β. In the canonical Smad signaling pathway, TGF-β phosphorylates TGF-βRII, which recruits and phosphorylates TGF-βRI. The activated TGF-βRI subsequently phosphorylates Smad2 and Smad3 proteins. These activated Smad2 and Smad3 proteins then recruit Smad4 to form a complex, which translocates into the nucleus. Within the nucleus, the Smad complex interacts with specific DNA sequences and other transcription factors to promote the transcription and expression of target genes, such as CTGF (Connective Tissue Growth Factor). In the subarachnoid space, CTGF contributes to pia mater fibrosis by promoting the synthesis and deposition of extracellular matrix components. In the non-canonical Smad signaling pathway, the activated TGF-βRI/II complex can further activate Cdc42/Rac1, which in turn activates downstream factors such as the P38 and PAK2 signaling pathways.
3.2 Vascular endothelial growth factor
In the brain, VEGF is a potent growth factor that plays diverse roles in vasculogenesis and angiogenesis, mediates angiogenesis, neural migration, and neuroprotection, leading to angiogenesis and increased vascular permeability (Shim and Madsen, 2018; Grunewald et al., 2021). VEGF levels tend to be higher in the ventricular CSF of animals and patients with hydrocephalus (Yang et al., 2016; Naureen et al., 2014). Alternatively, VEGF/VEGFR-2 levels in the CP–CSF circulatory system may reflect the activity of the VEGF system in the brain, especially in periventricular areas (Yang et al., 2010). In rats, infusion of VEGF-A165 led to twofold enlargement of the ventricles, which had several other effects: elevation of VEGFR2 phosphorylation in the ependyma, alterations in β-catenin and E-cadherin levels, ependymal cell denudation, and altered ciliary staining on the ventricular surface (Shim et al., 2013). However, the ventricular response can vary in animal experiments depending on the infusion rate and how long the infusion rate is administered. Moreover, excess HB-EGF leads to a significant increase in VEGF and ventricular dilatation (Shim et al., 2016). VEGFR2 has been identified as the primary receptor for VEGF (Terman et al., 1992). The binding of VEGF induces VEGFR2 dimerization, which regulates the activation of Src kinase. This activation leads to the phosphorylation and internalization of VE-cadherin, while also reducing its interaction with associated proteins such as p120-catenin and β-catenin, thereby strengthening the endocytosis process (Dejana and Vestweber, 2013; Del Bigio, 2010; Delgado-Bellido et al., 2017; Li et al., 2016; Gavard and Gutkind, 2006; Xiao et al., 2005; Rahimi, 2017). Consequently, VE-cadherin expression on the cell membrane decreases, becomes unevenly distributed, and instead increases in the cytoplasm. This redistribution disrupts intercellular junctions, exacerbates the open-window effect, compromises the blood–brain barrier (BBB), and ultimately contributes to hydrocephalus development (Shen et al., 2022). Additionally, VEGFR2 dimerization enhances the activation of the small GTPase Rac through Src-dependent phosphorylation of the guanine nucleotide exchange factor Vav2. Activated Rac promotes the p21-activated kinase (PAK)-mediated phosphorylation of conserved motifs in the intracellular tail of VE-cadherin. This phosphorylation recruits β-arrestin-2 to the serine-phosphorylated VE-cadherin, further promoting its internalization into clathrin-coated vesicles. The resulting disassembly of intercellular junctions further disrupts the BBB and contributes to hydrocephalus development (Figure 2) (Apte et al., 2019; Lolansen et al., 2021). Interestingly, metformin can inhibit VEGF/VEGFR2/p-Src pathway activation, reverse the internalization of VE-cadherin, and ameliorate IVH-induced hydrocephalus in a rat model (Shen et al., 2022). Although VEGF is a potential therapeutic target for hydrocephalus, its role has been explored in relatively few studies, and its specific mechanisms have yet to be fully elucidated. Moreover, the efficacy of targeted therapies against VEGF still requires further investigation through translational research.
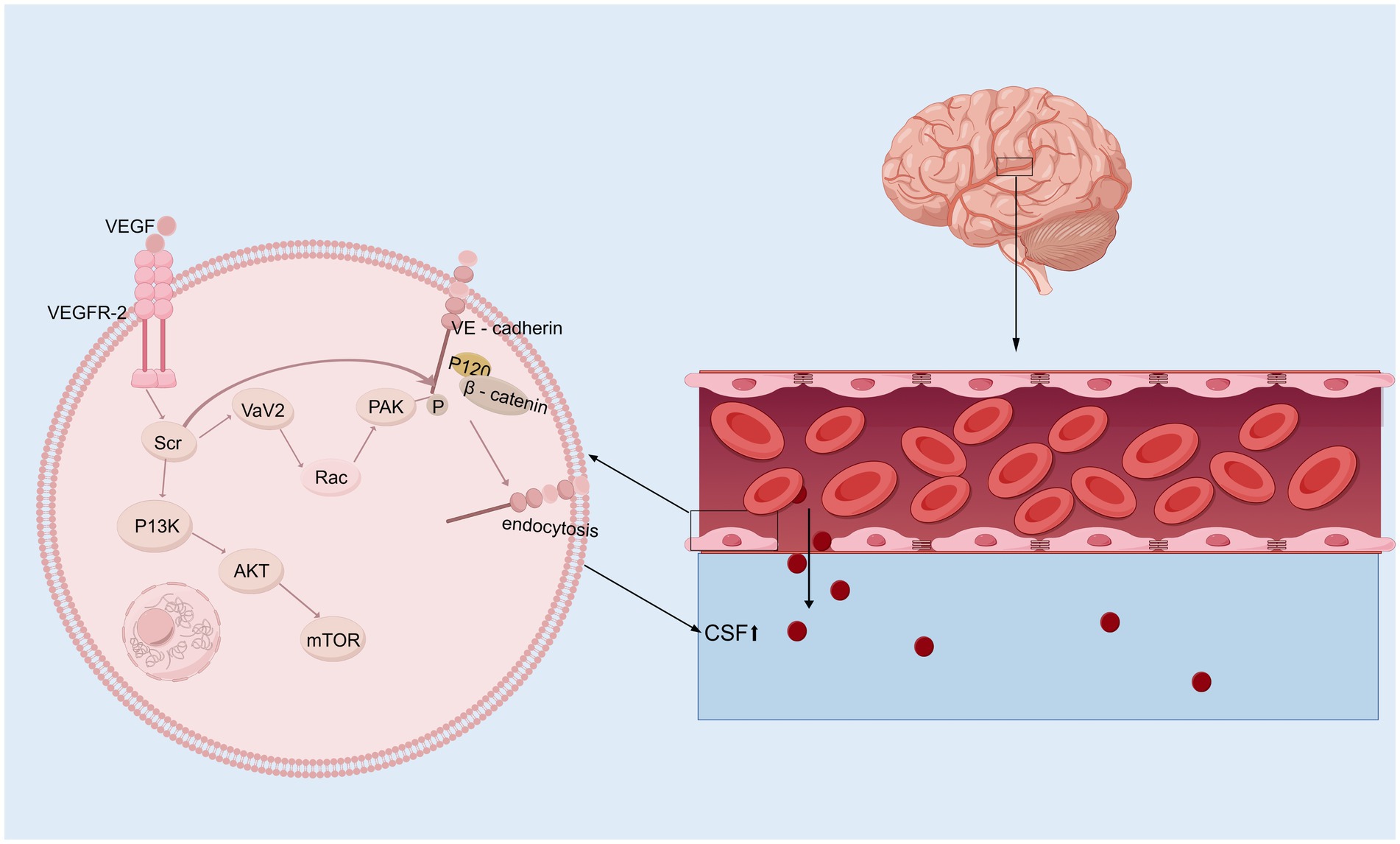
Figure 2. VEGF signaling pathway. Upon binding to its receptor VEGFR-2 on the surface of vascular endothelial cells, VEGF first activates Src kinase, which subsequently phosphorylates and activates Vav2. This activation facilitates the conversion of Rac from its GDP-bound inactive state to its GTP-bound active state. Activated Rac further activates PAK (p21-activated kinase). PAK, or alternatively the activated Src kinase, phosphorylates VE-cadherin (Vascular Endothelial Cadherin), allowing β-catenin to bind to its tail. This phosphorylation mediates VE-cadherin internalization, resulting in a “fenestration effect,” which contributes to the development of hydrocephalus. In addition, activated Src kinase can also mediate the PI3K/AKT/mTOR signaling pathway, initiating a series of downstream cascade reactions.
3.3 Wnt signaling pathway
The Wnt/β-catenin signaling pathway initiates a signaling cascade that is critical for the normal development of multiple organ systems; furthermore, this pathway plays a crucial role throughout all stages of brain development, such as neurostem cell development and subventricular zone development, and it is linked to many neurological disorders (Song et al., 2021; Clevers and Nusse, 2012). In the presence of the Wnt/β-catenin signaling pathway, the binding of Wnts to a frizzled receptor and low-density lipoprotein receptor-related protein 5/6 (LRP5/6) coreceptor triggers the recruitment of the cytoplasmic component, which is dishevelled and thus inhibits the phosphorylation of β-catenin via glycogen synthase kinase three beta (GSK-3b) (Noelanders and Vleminckx, 2017; Nusse and Clevers, 2017; Zhao et al., 2022). In the hydrocephalus rat model induced by low-concentration kaolin (3%), the Wnt signaling pathway was activated, leading to reactive gliosis, which can be reversed by secreted frizzled-related protein 1 (sFRP-l). In contrast, the development of hydrocephalus is delayed (Xu et al., 2015; Suryaningtyas et al., 2020). Moreover, the increase in Wnt/Wnt3a mRNA and protein expression was significant in rats with hydrocephalus induced by intraventricular injection of autologous blood, and deferoxamine alleviated this increase, suggesting that iron is a vital factor that activates the Wnt signaling pathway (Meng et al., 2015). A study on the role of the R595H-Trim71 mutation associated with congenital hydrocephalus in neural differentiation has shown that regulating the Wnt/β-catenin signaling pathway can effectively improve the neural differentiation defects in R595H-Trim71 mutant cells. These findings suggest that the Trim71 mutation may play a key role in the pathogenesis of congenital hydrocephalus through specific pathological mechanisms, providing a new direction for the development of precise therapeutic strategies for congenital hydrocephalus (Figure 3) (Liu et al., 2023; Cuevas et al., 2015).Further research is needed to establish definitive evidence of the relationships among ferroptosis, Wnt signaling, and hydrocephalus. Interestingly, the Wnt signaling pathway plays a pivotal role in organ fibrosis, such as renal fibrosis and liver fibrosis, and the mechanism of the Wnt signaling pathway in flexural meningeal fibrosis in hydrocephalus remains unclear.
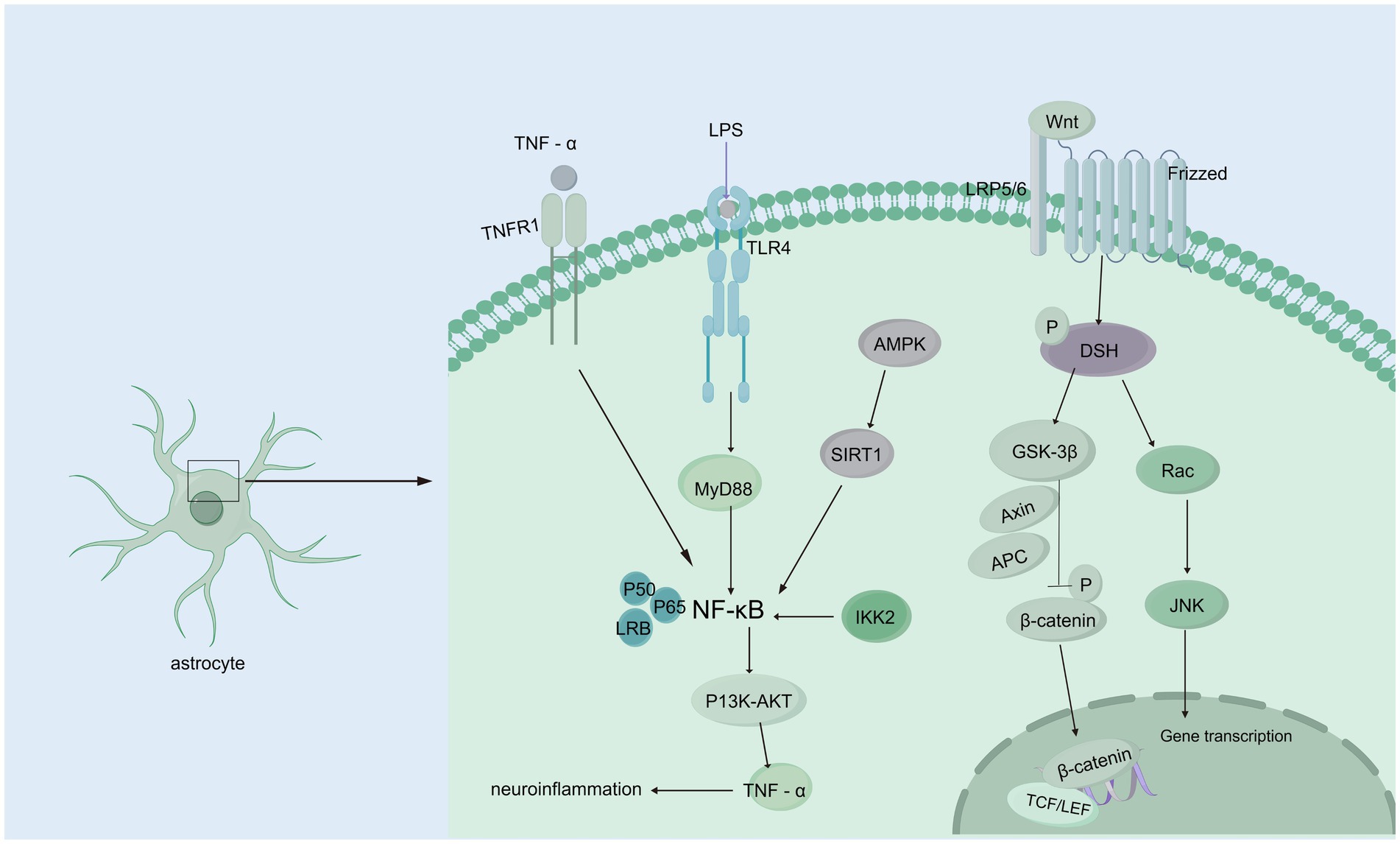
Figure 3. Wnt/β-catenin and NF-κB signaling pathways. Wnt/β-catenin signaling pathway: When Wnt ligands bind to the Frizzled receptor on the membrane of astrocytes, they activate DSH (Disheveled) protein, initiating downstream signaling. DSH inhibits GSK-3β (glycogen synthase kinase-3β) activity, preventing β-catenin phosphorylation. This leads to the accumulation of β-catenin in the cytoplasm, allowing it to translocate to the nucleus, where it binds to transcription factors such as TCF/LEF, regulating gene expression and interfering with CSF circulation and absorption, which contributes to hydrocephalus. Additionally, activated DSH can activate Rac, which further activates the JNK signaling pathway, affecting CSF homeostasis. NF-κB signaling pathway: TNF-α binds to the TNFR1 receptor, directly activating NF-κB signaling; TLR4 recognizes endogenous or exogenous ligands, activating NF-κB through the MyD88-dependent pathway. AMPK activation, caused by cellular energy imbalance, stimulates SIRT1, which subsequently activates NF-κB signaling. IKK2 activation can also trigger NF-κB signaling. Activated NF-κB regulates downstream PI3K/AKT signaling, upregulating TNF-α, forming a positive feedback loop that exacerbates inflammation and further contributes to hydrocephalus development.
3.4 Aquaporins
Aquaporins (AQPs) are integral membrane proteins that facilitate selective water and solute transport across cell membranes and maintain cellular homeostasis and fluid balance in neural compartments (Filippidis et al., 2016; Toader et al., 2023). The expression profiles of AQPs, particularly AQP4 and AQP1, have been demonstrated to be significantly expressed in the CNS, and dysregulated AQP expression is implicated in various brain pathologies (Filippidis et al., 2016). In the CNS, AQP4 is expressed predominantly in subpial astrocyte processes, which form the glial-limiting membrane, perivascular astrocyte endfeet, and the basolateral membrane of the ependymal and subependymal regions (Kitchen et al., 2020). AQP4 is variable in the early stage but higher in the later stage, indicating that it compensates for reducing the production of CSF and the mechanism for the clearance of excess interstitial fluid in hydrocephalus (Mao et al., 2006; Gonzalez-Marrero et al., 2022). In the spontaneously hypertensive rat model, AQP4 expression was significantly lower in ventricular cells and subventricular astrocytes of 12-month-old spontaneously hypertensive rats compared to control rats and 6-month-old spontaneously hypertensive rats. This suggests that changes in AQP4 expression in spontaneously hypertensive rats may play a more significant role in obstructing the pathway of CSF from the ventricles to the parenchyma, rather than merely decreasing the volume of ventricular CSF to prevent edema (Paul et al., 2011). In congenitally hydrocephalic H-Tx rats, a significant increase in the cerebral cortical expression of AQP4 was observed (Shen et al., 2006). If the gene is knocked out in mice, it disrupts gap junctions, which alters the ventricular zone and cerebrospinal fluid flow, leading to hydrocephalus development (Li et al., 2009). Significantly increased expression of AQP4 has been reported in several models, including the kaolin-induced hydrocephalus model in rats, Texas rats with congenital hydrocephalus, dogs with idiopathic obstructive hydrocephalus, and a rat model of inflammatory obstructive hydrocephalus. This increase in AQP4 expression was strongly correlated with the severity of hydrocephalus (Paul et al., 2011; Schmidt et al., 2016; Tourdias et al., 2009). Interestingly, the expression of AQP4 was not detected, which can be explained by the experimental results of Aghayev et al., who reported that the expression of AQP4 in mild hydrocephalus is not elevated (Aghayev et al., 2012). In recent years, the role of AQP4 in the glymphatic system has been gradually elucidated. Alterations in the glymphatic system associated with the downregulation or redistribution of AQP4 appear to play a role in the etiology of idiopathic normal-pressure hydrocephalus, but it remains unclear whether there is are direct associations between the glymphatic system, AQP4, and hydrocephalus. AQP1 is predominantly localized to the ventricular-facing plasma membrane of choroid plexus epithelial cells. Experimental evidence has shown that knockdown of AQP1 reduces osmotically-driven water permeability in choroid plexus epithelial cells, leading to a decrease in CSF production and a reduction in intraventricular pressure. These findings suggest that AQP1 plays a critical role in the regulation of CSF production (Zanotto et al., 2017). In the rat model of kaolin-induced hydrocephalus, AQP 1 expression decreased dramatically in the early stages of hydrocephalus by about 50% through the mechanism of endocytosis restoration (Wang et al., 2011) Additionally, a marked reduction in AQP1 expression in the choroid plexus epithelium was observed in spontaneously hypertensive rats. In Texas rats with congenital hydrocephalus, choroidal AQP1 expression was reduced early in life but normalized by postnatal day 26, prior to death (Paul et al., 2011). However, experiments involving AQP1 knockout and AQP4 knockout mice, which were injected intravenously with O17-labeled water (H2O17), revealed that water entering the lateral ventricles was significantly reduced in AQP4 knockout mice but not in AQP1 knockout mice, suggesting that AQP4 plays a critical role in cerebrospinal fluid formation (Igarashi et al., 2014). Moreover, the upregulation of AQP5 and downregulation of AQP1 with an apical localization in choroid plexus epithelial cells were observed in hydrocephalus following IVH (Sveinsdottir et al., 2014). The main functions of AQP5 include regulating water permeability, paracellular water transport, and cytoskeletal organization and stability (Express Group, 2010; Sidhaye et al., 2008; Sidhaye et al., 2012). In a rabbit model of IVH with posthemorrhagic ventricular dilatation and in vitro cultured human choroid plexus epithelial cells treated with posthemorrhagic cerebrospinal fluid and hemoglobin chloride, it was observed that AQP1 mRNA, a key regulator of CSF production, was downregulated. However, the protein level of apical epithelial cell-localized AQP1 was upregulated. Additionally, AQP5 was expressed in the choroid plexus, with both its mRNA expression and protein levels increasing during posthemorrhagic ventricular dilatation, specifically in apical epithelial cell localization (Sveinsdottir et al., 2014). AQP9 was expressed in astrocytes, cerebellar neurons, limbic vascular endothelium, glial border membrane, hypothalamic monolayer cells, and CA2 in the hippocampus (Badaut and Regli, 2004; Badaut et al., 2002). AQP11 was localized to the choroid plexus epithelium and cerebral capillary endothelium, suggesting its potential involvement in water transport within the choroid plexus and across the BBB in the brain (Filippidis et al., 2016). In addition, the relationships among AQP4, AQP1, and CSF transport have been progressively elucidated, revealing their critical roles in maintaining CSF homeostasis. However, despite the growing understanding of the functions of AQP5, AQP9, and AQP11 in CSF transport, their specific mechanisms in the development and progression of hydrocephalus still require further investigation, with the aim of providing new targets for the early diagnosis and treatment of hydrocephalus.
3.5 Nuclear factor-κB
Many neurological diseases, including hydrocephalus, are associated with neuroinflammation, and a significant regulator of inflammation is nuclear factor-κB (NF-κB). The expression of constitutively active IKK2 in astrocytes induces NF-κB activation, causing hippocampal malformation and resulting in early postnatal hydrocephalus associated with a lack of ependymal cilia (Lattke et al., 2012). Interestingly, NF-κB activation in astrocytes causes hydrocephalus only in the developing brain. NF-κB signaling in ependymal cells of the ventricle is increased following IVH (Simard et al., 2011). Moreover, in a kaolin-induced hydrocephalus model, toll-like receptor 4 (TLR4)-NF-κB signaling in the choroid plexus epithelium (CPE) stimulates CSF hypersecretion through the SPAK-NKCC1 cotransporter complex, thereby uncovering a novel kinase-regulated mechanism of CSF secretion (Heep et al., 2004; Xu et al., 2024) and the expression level of the TLR4–NF-κB signaling pathway was increased significantly in hydrocephalus after IVH. In an IVH rat model, TAK-242, which is a TLR4 inhibitor, effectively downregulated the TLR4–NF-κB signaling pathway, fibronectin, and laminin and significantly alleviated ventriculomegaly after IVH (Lin et al., 2022). In post-IVH hydrocephalus, the phospho-NF-κB (p-NF-κB) signaling pathway is activated, and metformin attenuates neuroinflammation and subsequent fibrosis after IVH by regulating the AMPK/SIRT1/NF-κB pathway (Cao et al., 2023).Additionally, it has been demonstrated that the aggregation of choroid plexus (ChP) macrophages exacerbates the inflammatory response of ChP epithelial cells via the TNF-α/TNFR1/NF-κB signaling cascade, leading to an increased secretion of CSF (Wang et al., 2024) In experimental models using ChP epithelial (CPE) cells to simulate the inflammatory conditions of IVH, it was observed that NKCC1, a key transporter involved in CSF secretion from the choroid plexus, is predominantly activated by interleukin-6 (IL-6) (Figure 3) (Johnsen et al., 2023).In addition, some studies have shown that NF-κB causes neuroinflammation via the PI3K-AKT/TNFAIP3 pathway in an experimental germinal matrix hemorrhage rat model, which can be reversed by rh-IFN-α (Li et al., 2020). However, it remains unclear whether inhibiting NF-κB through the JAK1-STAT1/TRAF3 pathway attenuates post-IVH hydrocephalus. Compelling evidence has confirmed that signaling pathways involving NF-κB are crucial for the pathogenesis of posthemorrhagic hydrocephalus (PPH) and may be effective targets for treating PPH. Research on the role of NF-κB in PPH is still limited, and its specific mechanisms have not been fully elucidated. Therefore, there is an urgent need for more systematic and in-depth basic and translational research to reveal the critical role of NF-κB in the development and progression of PPH, providing a theoretical basis and innovative ideas for the development of targeted therapeutic drugs for PPH.
3.6 Na+/K+/2Cl cotransporter
Na+/K+/2Cl− cotransporters (NKCCs) are located on the apical membrane of the choroid plexus in the central nervous system as essential mechanisms of cell volume regulation and contribute to approximately half of the production of CSF (Loscher and Kaila, 2022; Karimy et al., 2017). NKCC1 contributes to CSF formation by transporting Na+, K+, and Cl− transmembrane coupled water, which enables water to be transported (Lolansen et al., 2022). Bumetanide, a chloride importer antagonist of NKCC1, has been shown to attenuate hydrocephalus after IVH (Simard et al., 2010). And attenuated abnormal CPE secretion and hydrocephalus by inhibiting TLR4 / NF-κB / NKCC1 and AQP1 (Xu et al., 2024; Johnsen et al., 2023). An experimental study of genetic risk for hydrocephalus revealed that loss of the Ptpn20 gene in H-TX rats resulted in the development of communicating hydrocephalus, and the same result was observed in Ptpn20−/− mice, in which NKCC1 phosphorylation is maintained in choroid plexus epithelial cells (Xu et al., 2022). It has been demonstrated that serum lipid LPA enters the ventricular system during hemorrhagic events and acts directly on TRPV4, and activation of TRPV4 leads to hyperactivation of NKCC1, such that the elevated rate of CSF secretion appears to contribute to the ensuing ventricular dilation leading to PHH (Toft-Bertelsen et al., 2022), suggesting that the development of PHH is associated with CSF hypersecretion in part because of choroidal plexus Na+/K+ − ATPase and NKCC1 hyperactivation, but the underlying molecular coupling remains to be explored (Lolansen et al., 2022). In a hydrocephalus rat model after IVH, the phosphorylation of NKCC1 was increased via the activation of NLRP3 inflammasome components, which indicated the involvement of the NLRP3/p-NKCC1 pathway and Na+ and K+ flux in the PPH (Zhang et al., 2022). Intraventricular blood increases CSF [K+] and triggers cytosolic calcium activity in epithelial cells, followed by NKCC1 activation (Johnsen et al., 2023). Interestingly, with elevated CSF [K+], NKCC1 activation leads to a net flux of ions and osmotically obliges water movement from the CSF into the choroid plexus, resulting in compensated PHHs. Choroid plexus-targeted NKCC1 overexpression can be used to treat acute hydrocephalus after IVH (Sadegh et al., 2023). Moreover, overexpression of NKCC1 in the choroid plexus results in increased CSF [K+] clearance, which reduces ventriculomegaly in the critical period during postnatal neurodevelopment in mice (Xu et al., 2021). The evidence above suggests that NKCC1 plays a vital role in inflammation-dependent cerebrospinal fluid hypersecretion by the choroid plexus epithelium in posthemorrhagic hydrocephalus (Figure 4). Interestingly, CSF containing elevated levels of a subset of inflammatory markers expressed in the choroid plexus of rats and humans did not activate NKCC1 in iNPH patients (Lolansen et al., 2021). Although the overexpression of NKCC1 in posthemorrhagic hydrocephalus has been observed, its specific mechanism remains unclear. Therefore, it is crucial to further investigate how NKCC1 overexpression affects CSF secretion and the development of hydrocephalus, particularly by exploring its relationship with CSF dynamics at the molecular level. This will not only deepen our understanding of its role in the pathological process but also provide a theoretical foundation for developing targeted therapeutic strategies. Additionally, future research should focus on developing NKCC1-targeted interventions, offering new therapeutic targets for the clinical treatment of posthemorrhagic hydrocephalus and improving patient outcomes.
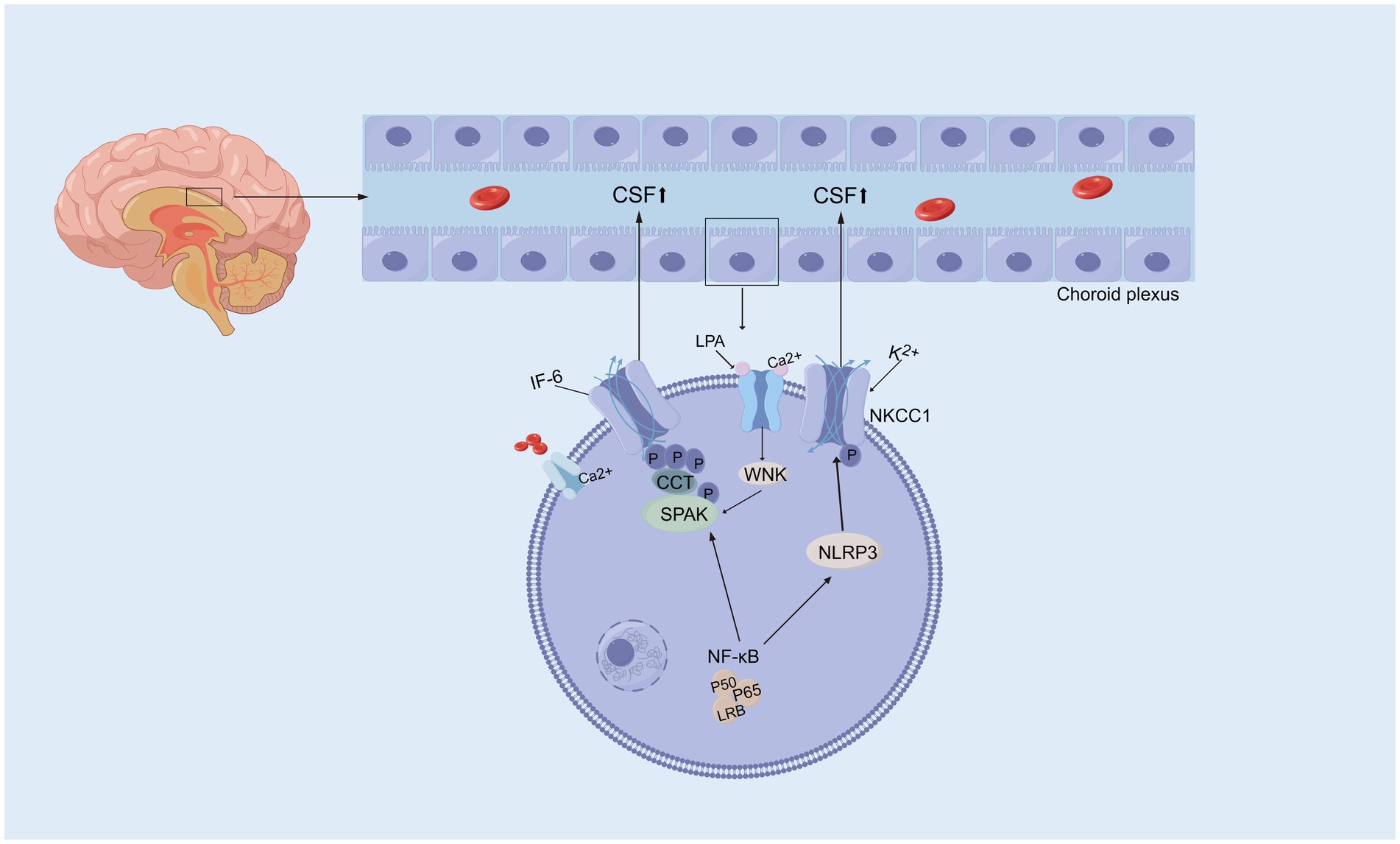
Figure 4. Activation of Na+/K+/2Cl cotransporter. Activated NF-κB initiates a signaling cascade that eventually activates SPAK, which phosphorylates and activates NKCC1, resulting in excessive CSF secretion and contributing to hydrocephalus. Moreover, activated NF-κB also influences NKCC1 activity by modulating the NLRP3 inflammasome, leading to abnormal CSF secretion and the promotion of hydrocephalus. Serum lipid LPA directly acts on TRPV4, mediating Ca2+ influx, which regulates the WNK-SPAK-mediated phosphorylation of NKCC1. This results in an increased CSF secretion rate and ventricular enlargement.
4 Conclusion and future perspectives
In summary, the development of hydrocephalus is a complex pathological process involving various genetic and molecular alterations. Any congenital or acquired factors that impact the structure and function of the ventricular system or disrupt the production, circulation, and absorption of cerebrospinal fluid can independently or synergistically lead to the onset of hydrocephalus. Congenital hydrocephalus is predominantly associated with abnormalities in the development of the ventricular system, whereas secondary hydrocephalus is linked to the dysregulation of multiple molecular pathways, including those related to inflammation, fibrosis, and injury.
Recent advancements in artificial intelligence (AI) and machine learning (ML) are playing an increasingly important role in understanding and predicting hydrocephalus. AI and ML models have demonstrated promising potential in analyzing imaging data to detect early changes in the brain associated with hydrocephalus, helping clinicians make more accurate diagnoses. Moreover, AI-driven predictive models are being developed to assess the functionality of ventriculoperitoneal shunts and provide real-time monitoring of disease progression. This technological shift not only enhances the detection of hydrocephalus but may also offers new possibilities for early intervention, especially in high-risk populations, by identifying critical molecular biomarkers and pathways associated with the disease. Integrating these approaches with molecular studies could eventually lead to the identification of novel targets for pharmacological treatments, optimizing the management of both congenital and secondary hydrocephalus.
Currently, several preclinical studies targeting the molecular mechanisms underlying secondary hydrocephalus have demonstrated promising results in preventing hydrocephalus and mitigating related damage. These findings suggest that the development of detection and intervention strategies targeting specific proteins or pathways, potentially assisted by AI and ML techniques, may hold significant potential for identifying high-risk patients and guiding the pharmacological treatment of secondary hydrocephalus. Furthermore, targeting gene alterations associated with hydrocephalus may play a critical role in screening for congenital hydrocephalus, thus offering new opportunities for early intervention.
To date, no drug has emerged from clinical trials that significantly improves hydrocephalus symptoms or reverses the progression of the disease. The complex pathological mechanisms of hydrocephalus make it challenging for a single drug to comprehensively regulate these mechanisms, resulting in limited therapeutic effectiveness. Furthermore, many drugs target widely distributed signaling pathways, which can lead to unpredictable side effects, thereby restricting the clinical application of these drugs. The integration of advanced methodologies could potentially guide the development of more specific treatments, enhancing therapeutic outcomes and reducing side effects. Further research into molecular mechanisms, improved diagnostics, and the development of targeted drugs is needed to fill this gap, ultimately improving the management and prognosis of hydrocephalus.
Author contributions
XD: Conceptualization, Data curation, Formal analysis, Funding acquisition, Investigation, Methodology, Project administration, Resources, Software, Supervision, Validation, Visualization, Writing – original draft, Writing – review & editing. YC: Writing – review & editing, Conceptualization, Data curation, Formal analysis, Investigation, Methodology, Project administration, Resources, Software, Supervision, Validation, Visualization, Writing – original draft. QD: Conceptualization, Data curation, Formal analysis, Investigation, Methodology, Project administration, Resources, Software, Supervision, Validation, Visualization, Writing – original draft, Writing – review & editing. JD: Writing – original draft, Writing – review & editing, Data curation. ZW: Data curation, Investigation, Writing – original draft, Writing – review & editing. JW: Data curation, Investigation, Writing – original draft, Writing – review & editing. XC: Data curation, Investigation, Writing – original draft, Writing – review & editing. LiZ: Writing – review & editing. LoZ: Writing – review & editing, Writing – original draft.
Funding
The author(s) declare that financial support was received for the research, authorship, and/or publication of this article. This work was supported by the joint development project of Guang'an People’s Hospital, Grant NO.2023LHFZ 03 (to Long Zhao); Innovation and Entrepreneurship Training Program for College Students in Sichuan Province, Grant No. S202210634079 (to Xuehai Deng), Grant No. 202310634033 (to Wang Zhong).
Acknowledgments
We would like to express our deepest gratitude to the Neurosurgery Department of North Sichuan Medical College for their invaluable guidance, support, and encouragement throughout this study. We also extend our sincere thanks to the Institute of Neurological Diseases for providing the necessary resources and facilities that made this research possible. Our appreciation goes to Affiliated Hospital of North Sichuan Medical College and North Sichuan Medical College for their financial support, which was crucial for the successful completion of this project. We would also like to thank Figdraw for providing us with a painting platform. We are also grateful to everyone for their contributions, insightful discussions, and constructive feedback. Lastly, we thank our families and friends for their continuous support and understanding during the course of this research.
Conflict of interest
The authors declare that the research was conducted in the absence of any commercial or financial relationships that could be construed as a potential conflict of interest.
Generative AI statement
The authors declare that no Generative AI was used in the creation of this manuscript.
Publisher’s note
All claims expressed in this article are solely those of the authors and do not necessarily represent those of their affiliated organizations, or those of the publisher, the editors and the reviewers. Any product that may be evaluated in this article, or claim that may be made by its manufacturer, is not guaranteed or endorsed by the publisher.
References
Adle-Biassette, H., Saugier-Veber, P., Fallet-Bianco, C., Delezoide, A. L., Razavi, F., Drouot, N., et al. (2013). Neuropathological review of 138 cases genetically tested for X-linked hydrocephalus: evidence for closely related clinical entities of unknown molecular bases. Acta Neuropathol. 126, 427–442. doi: 10.1007/s00401-013-1146-1
Aghayev, K., Bal, E., Rahimli, T., Mut, M., Balci, S., Vrionis, F., et al. (2012). Aquaporin-4 expression is not elevated in mild hydrocephalus. Acta Neurochir. 154, 753–759. doi: 10.1007/s00701-011-1241-9
Ahmed, R. R., Medhat, A. M., Hamdy, G. M., Effat, L. K. E., Abdel-Hamid, M. S., and Abdel-Salam, G. M. H. (2023). X-linked hydrocephalus with new L1CAM pathogenic variants: review of the Most prevalent molecular and phenotypic features. Molecul. Syndromol. 14, 283–292. doi: 10.1159/000529545
Al-Jezawi, N. K., Al-Shamsi, A. M., Suleiman, J., Ben-Salem, S., John, A., Vijayan, R., et al. (2018). Compound heterozygous variants in the multiple PDZ domain protein (MPDZ) cause a case of mild non-progressive communicating hydrocephalus. BMC Med. Genet. 19:34. doi: 10.1186/s12881-018-0540-x
Allocco, A. A., Jin, S. C., Duy, P. Q., Furey, C. G., Zeng, X., Dong, W., et al. (2019). Recessive inheritance of congenital hydrocephalus with other structural brain abnormalities caused by compound heterozygous mutations in ATP1A3. Front. Cell. Neurosci. 13:425. doi: 10.3389/fncel.2019.00425
Apte, R. S., Chen, D. S., and Ferrara, N. (2019). VEGF in signaling and disease: beyond discovery and development. Cell 176, 1248–1264. doi: 10.1016/j.cell.2019.01.021
Badaut, J., Lasbennes, F., Magistretti, P. J., and Regli, L. (2002). Aquaporins in brain: distribution, physiology, and pathophysiology. J. Cerebral Blood Flow Metabol. 22, 367–378. doi: 10.1097/00004647-200204000-00001
Badaut, J., and Regli, L. (2004). Distribution and possible roles of aquaporin 9 in the brain. Neuroscience 129, 971–981. doi: 10.1016/j.neuroscience.2004.06.035
Bailey, L. B., and Gregory, J. F. 3rd. (1999). Folate metabolism and requirements. J. Nutr. 129, 779–782. doi: 10.1093/jn/129.4.779
Banizs, B., Pike, M. M., Millican, C. L., Ferguson, W. B., Komlosi, P., Sheetz, J., et al. (2005). Dysfunctional cilia lead to altered ependyma and choroid plexus function, and result in the formation of hydrocephalus. Development 132, 5329–5339. doi: 10.1242/dev.02153
Batiz, L. F., Paez, P., Jimenez, A. J., Rodriguez, S., Wagner, C., Perez-Figares, J. M., et al. (2006). Heterogeneous expression of hydrocephalic phenotype in the hyh mice carrying a point mutation in alpha-SNAP. Neurobiol. Dis. 23, 152–168. doi: 10.1016/j.nbd.2006.02.009
Batiz, L. F., Roales-Bujan, R., Rodriguez-Perez, L. M., Matas, I. M., Paez, P., Roque, M., et al. (2009). A simple PCR-based genotyping method for M105I mutation of alpha-SNAP enhances the study of early pathological changes in hyh phenotype. Mol. Cell. Probes 23, 281–290. doi: 10.1016/j.mcp.2009.07.002
Bhoyar, R. C., Jadhao, A. G., Sabharwal, A., Ranjan, G., Sivasubbu, S., and Pinelli, C. (2019). Knockdown of calcium-binding calb2a and calb2b genes indicates the key regulator of the early development of the zebrafish, Danio rerio. Brain structure & function. 224, 627–642. doi: 10.1007/s00429-018-1797-8
Blackshear, P. J., Graves, J. P., Stumpo, D. J., Cobos, I., Rubenstein, J. L., and Zeldin, D. C. (2003). Graded phenotypic response to partial and complete deficiency of a brain-specific transcript variant of the winged helix transcription factor RFX4. Development 130, 4539–4552. doi: 10.1242/dev.00661
Borit, A., and Sidman, R. L. (1972). New mutant mouse with communicating hydrocephalus and secondary aqueductal stenosis. Acta Neuropathol. 21, 316–331. doi: 10.1007/BF00685139
Bousquet, I., Bozon, M., Castellani, V., Touraine, R., Piton, A., Gérard, B., et al. (2021). X-linked partial corpus callosum agenesis with mild intellectual disability: identification of a novel L1CAM pathogenic variant. Neurogenetics 22, 43–51. doi: 10.1007/s10048-020-00629-y
Bronson, R. T., and Lane, P. W. (1990). Hydrocephalus with hop gait (hyh): a new mutation on chromosome 7 in the mouse. Brain Res. Dev. Brain Res. 54, 131–136. doi: 10.1016/0165-3806(90)90073-8
Cao, Y., Liu, C., Li, G., Gao, W., Tang, H., Fan, S., et al. (2023). Metformin alleviates delayed hydrocephalus after intraventricular hemorrhage by inhibiting inflammation and fibrosis. Transl. Stroke Res. 14, 364–382. doi: 10.1007/s12975-022-01026-3
Cesca, F., Yabe, A., Spencer-Dene, B., Scholz-Starke, J., Medrihan, L., Maden, C. H., et al. (2012). Kidins220/ARMS mediates the integration of the neurotrophin and VEGF pathways in the vascular and nervous systems. Cell Death Differ. 19, 194–208. doi: 10.1038/cdd.2011.141
Chae, T. H., Allen, K. M., Davisson, M. T., Sweet, H. O., and Walsh, C. A. (2002). Mapping of the mouse hyh gene to a YAC/BAC contig on proximal chromosome 7. Mamm. Genome 13, 239–244. doi: 10.1007/s00335-001-2144-5
Chae, T. H., Kim, S., Marz, K. E., Hanson, P. I., and Walsh, C. A. (2004). The hyh mutation uncovers roles for alpha Snap in apical protein localization and control of neural cell fate. Nat. Genet. 36, 264–270. doi: 10.1038/ng1302
Chen, Q., Feng, Z., Tan, Q., Guo, J., Tang, J., Tan, L., et al. (2017). Post-hemorrhagic hydrocephalus: recent advances and new therapeutic insights. J. Neurol. Sci. 375, 220–230. doi: 10.1016/j.jns.2017.01.072
Claassen, J., and Park, S. (2022). Spontaneous subarachnoid haemorrhage. Lancet 400, 846–862. doi: 10.1016/S0140-6736(22)00938-2
Clevers, H., and Nusse, R. (2012). Wnt/beta-catenin signaling and disease. Cell 149, 1192–1205. doi: 10.1016/j.cell.2012.05.012
Cuevas, E., Rybak-Wolf, A., Rohde, A. M., Nguyen, D. T., and Wulczyn, F. G. (2015). Lin41/Trim71 is essential for mouse development and specifically expressed in postnatal ependymal cells of the brain. Front. Cell Dev. Biol. 3:20. doi: 10.3389/fcell.2015.00020
Davy, B. E., and Robinson, M. L. (2003). Congenital hydrocephalus in hy3 mice is caused by a frameshift mutation in Hydin, a large novel gene. Hum. Mol. Genet. 12, 1163–1170. doi: 10.1093/hmg/ddg122
Dawe, H. R., Shaw, M. K., Farr, H., and Gull, K. (2007). The hydrocephalus inducing gene product, Hydin, positions axonemal central pair microtubules. BMC Biol. 5:33. doi: 10.1186/1741-7007-5-33
Dejana, E., and Vestweber, D. (2013). The role of VE-cadherin in vascular morphogenesis and permeability control. Prog. Mol. Biol. Transl. Sci. 116, 119–144. doi: 10.1016/B978-0-12-394311-8.00006-6
Del Bigio, M. R. (2010). Ependymal cells: biology and pathology. Acta Neuropathol. 119, 55–73. doi: 10.1007/s00401-009-0624-y
Delgado-Bellido, D., Serrano-Saenz, S., Fernández-Cortés, M., and Oliver, F. J. (2017). Vasculogenic mimicry signaling revisited: focus on non-vascular VE-cadherin. Mol. Cancer 16:65. doi: 10.1186/s12943-017-0631-x
Derynck, R., and Budi, E. H. (2019). Specificity, versatility, and control of TGF-β family signaling. Sci. Signal. 12:6. doi: 10.1126/scisignal.aav5183
Dong, C., Ming, X., Ye, Z., Wang, P., Wang, L., Li, Z., et al. (2018). Icariside II attenuates chronic hydrocephalus in an experimental subarachnoid hemorrhage rat model. J. Pharm. Pharm. Sci. 21, 318–325. doi: 10.18433/jpps29811
Doublier, S., Duyckaerts, C., Seurin, D., and Binoux, M. (2000). Impaired brain development and hydrocephalus in a line of transgenic mice with liver-specific expression of human insulin-like growth factor binding protein-1. Growth Hormone IGF Res. 10, 267–274. doi: 10.1054/ghir.2000.0168
Douglas, M. R., Daniel, M., Lagord, C., Akinwunmi, J., Jackowski, A., Cooper, C., et al. (2009). High CSF transforming growth factor beta levels after subarachnoid haemorrhage: association with chronic communicating hydrocephalus. J. Neurol. Neurosurg. Psychiatry 80, 545–550. doi: 10.1136/jnnp.2008.155671
Dunlevy, L. P., Burren, K. A., Chitty, L. S., Copp, A. J., and Greene, N. D. (2006). Excess methionine suppresses the methylation cycle and inhibits neural tube closure in mouse embryos. FEBS Lett. 580, 2803–2807. doi: 10.1016/j.febslet.2006.04.020
Duy, P. Q., Weise, S. C., Marini, C., Li, X. J., Liang, D., Dahl, P. J., et al. (2022). Impaired neurogenesis alters brain biomechanics in a neuroprogenitor-based genetic subtype of congenital hydrocephalus. Nat. Neurosci. 25, 458–473. doi: 10.1038/s41593-022-01043-3
Eide, P. K., and Hansson, H. A. (2018). Astrogliosis and impaired aquaporin-4 and dystrophin systems in idiopathic normal pressure hydrocephalus. Neuropathol. Appl. Neurobiol. 44, 474–490. doi: 10.1111/nan.12420
Etchegaray, A., Juarez-Peñalva, S., Petracchi, F., and Igarzabal, L. (2020). Prenatal genetic considerations in congenital ventriculomegaly and hydrocephalus. Childs Nerv. Syst. 36, 1645–1660. doi: 10.1007/s00381-020-04526-5
Express Group (2010). Incidence of and risk factors for neonatal morbidity after active perinatal care: extremely preterm infants study in Sweden (EXPRESS). Acta paediatrica 99, 978–992. doi: 10.1111/j.1651-2227.2010.01846.x
Filippidis, A. S., Carozza, R. B., and Rekate, H. L. (2016). Aquaporins in brain edema and neuropathological conditions. Int. J. Mol. Sci. 18:1. doi: 10.3390/ijms18010055
Flood, C., Akinwunmi, J., Lagord, C., Daniel, M., Berry, M., Jackowski, A., et al. (2001). Transforming growth factor-beta1 in the cerebrospinal fluid of patients with subarachnoid hemorrhage: titers derived from exogenous and endogenous sources. J. Cerebral Blood Flow Metabol. 21, 157–162. doi: 10.1097/00004647-200102000-00007
Furey, C. G., Choi, J., Jin, S. C., Zeng, X., Timberlake, A. T., Nelson-Williams, C., et al. (2018). De novo mutation in genes regulating neural stem cell fate in human congenital hydrocephalus. Neuron 99, 302–14.e4. doi: 10.1016/j.neuron.2018.06.019
García-Bonilla, M., García-Martín, M. L., Muñoz-Hernández, M. C., Domínguez-Pinos, D., Martínez-León, M. I., Peñalver, A., et al. (2018). A distinct metabolite profile correlates with neurodegenerative conditions and the severity of congenital hydrocephalus. J. Neuropathol. Exp. Neurol. 77, 1122–1136. doi: 10.1093/jnen/nly097
Garcia-Bonilla, M., McAllister, J. P., and Limbrick, D. D. (2021). Genetics and molecular pathogenesis of human hydrocephalus. Neurol. India 69, S268–S274. doi: 10.4103/0028-3886.332249
Gavard, J., and Gutkind, J. S. (2006). VEGF controls endothelial-cell permeability by promoting the beta-arrestin-dependent endocytosis of VE-cadherin. Nat. Cell Biol. 8, 1223–1234. doi: 10.1038/ncb1486
Gavino, C., and Richard, S. (2011). Patched1 haploinsufficiency impairs ependymal cilia function of the quaking viable mice, leading to fatal hydrocephalus. Mol. Cell. Neurosci. 47, 100–107. doi: 10.1016/j.mcn.2011.03.004
Gonzalez, A. M., Podvin, S., Lin, S. Y., Miller, M. C., Botfield, H., Leadbeater, W. E., et al. (2011). Ecrg4 expression and its product augurin in the choroid plexus: impact on fetal brain development, cerebrospinal fluid homeostasis and neuroprogenitor cell response to CNS injury. Fluids Barr. CNS. 8:6. doi: 10.1186/2045-8118-8-6
Gonzalez-Marrero, I., Hernandez-Abad, L. G., Gonzalez-Gomez, M., Soto-Viera, M., Carmona-Calero, E. M., Castaneyra-Ruiz, L., et al. (2022). Altered expression of AQP1 and AQP4 in brain barriers and cerebrospinal fluid may affect cerebral water balance during chronic hypertension. Int. J. Mol. Sci. 23:12277. doi: 10.3390/ijms232012277
Grunewald, M., Kumar, S., Sharife, H., Volinsky, E., Gileles-Hillel, A., Licht, T., et al. (2021). Counteracting age-related VEGF signaling insufficiency promotes healthy aging and extends life span. Science 373:1. doi: 10.1126/science.abc8479
Heep, A., Stoffel-Wagner, B., Bartmann, P., Benseler, S., Schaller, C., Groneck, P., et al. (2004). Vascular endothelial growth factor and transforming growth factor-beta1 are highly expressed in the cerebrospinal fluid of premature infants with posthemorrhagic hydrocephalus. Pediatr. Res. 56, 768–774. doi: 10.1203/01.PDR.0000141524.32142.53
Hirschner, W., Pogoda, H. M., Kramer, C., Thiess, U., Hamprecht, B., Wiesmüller, K. H., et al. (2007). Biosynthesis of Wdr16, a marker protein for kinocilia-bearing cells, starts at the time of kinocilia formation in rat, and wdr16 gene knockdown causes hydrocephalus in zebrafish. J. Neurochem. 101, 274–288. doi: 10.1111/j.1471-4159.2007.04500.x
Hochstetler, A., Raskin, J., and Blazer-Yost, B. L. (2022). Hydrocephalus: historical analysis and considerations for treatment. Eur. J. Med. Res. 27:168. doi: 10.1186/s40001-022-00798-6
Hong, H. K., Chakravarti, A., and Takahashi, J. S. (2004). The gene for soluble N-ethylmaleimide sensitive factor attachment protein alpha is mutated in hydrocephaly with hop gait (hyh) mice. Proc. Natl. Acad. Sci. USA 101, 1748–1753. doi: 10.1073/pnas.0308268100
Hong, H. K., Lass, J. H., and Chakravarti, A. (1999). Pleiotropic skeletal and ocular phenotypes of the mouse mutation congenital hydrocephalus (ch/Mf1) arise from a winged helix/forkhead transcriptionfactor gene. Hum. Mol. Genet. 8, 625–637. doi: 10.1093/hmg/8.4.625
Hou, C. C., Li, D., Berry, B. C., Zheng, S., Carroll, R. S., Johnson, M. D., et al. (2023). Heterozygous FOXJ1 mutations cause incomplete ependymal cell differentiation and communicating hydrocephalus. Cell. Mol. Neurobiol. 43, 4103–4116. doi: 10.1007/s10571-023-01398-6
Hourvitz, N., Kurolap, A., Mory, A., Haratz, K. K., Kidron, D., Malinger, G., et al. (2023). SMARCC1 is a susceptibility gene for congenital hydrocephalus with an autosomal dominant inheritance mode and incomplete penetrance. Prenat. Diagn. 43, 1374–1377. doi: 10.1002/pd.6426
Howard, H. C., Mount, D. B., Rochefort, D., Byun, N., Dupré, N., Lu, J., et al. (2002). The K-cl cotransporter KCC3 is mutant in a severe peripheral neuropathy associated with agenesis of the corpus callosum. Nat. Genet. 32, 384–392. doi: 10.1038/ng1002
Howe, K., Clark, M. D., Torroja, C. F., Torrance, J., Berthelot, C., Muffato, M., et al. (2013). The zebrafish reference genome sequence and its relationship to the human genome. Nature 496, 498–503. doi: 10.1038/nature12111
Huang, Y. W., Zhang, Y., Feng, C., An, Y. H., Li, Z. P., and Yin, X. S. (2023). Systemic inflammation response index as a clinical outcome evaluating tool and prognostic indicator for hospitalized stroke patients: a systematic review and meta-analysis. Eur. J. Med. Res. 28:474. doi: 10.1186/s40001-023-01446-3
Hurd, T. W., Otto, E. A., Mishima, E., Gee, H. Y., Inoue, H., Inazu, M., et al. (2013). Mutation of the Mg2+ transporter SLC41A1 results in a nephronophthisis-like phenotype. J. Amer. Soc. Nephrol. 24, 967–977. doi: 10.1681/ASN.2012101034
Ibanez-Tallon, I., Gorokhova, S., and Heintz, N. (2002). Loss of function of axonemal dynein Mdnah5 causes primary ciliary dyskinesia and hydrocephalus. Hum. Mol. Genet. 11, 715–721. doi: 10.1093/hmg/11.6.715
Igarashi, H., Tsujita, M., Kwee, I. L., and Nakada, T. (2014). Water influx into cerebrospinal fluid is primarily controlled by aquaporin-4, not by aquaporin-1: 17O JJVCPE MRI study in knockout mice. Neuroreport 25, 39–43. doi: 10.1097/WNR.0000000000000042
Ishida, T., Murayama, T., and Kobayashi, S. (2023). Current research of idiopathic normal pressure hydrocephalus: pathogenesis, diagnosis and treatment. World J. Clin. Cases 11, 3706–3713. doi: 10.12998/wjcc.v11.i16.3706
Jacquet, B. V., Salinas-Mondragon, R., Liang, H., Therit, B., Buie, J. D., Dykstra, M., et al. (2009). FoxJ1-dependent gene expression is required for differentiation of radial glia into ependymal cells and a subset of astrocytes in the postnatal brain. Development 136, 4021–4031. doi: 10.1242/dev.041129
Jie, W., Andrade, K. C., Lin, X., Yang, X., Yue, X., and Chang, J. (2015). Pathophysiological functions of Rnd3/RhoE. Compr. Physiol. 6, 169–186. doi: 10.1002/cphy.c150018
Jimenez, A. R., Naz, N., and Miyan, J. A. (2019). Altered folate binding protein expression and folate delivery are associated with congenital hydrocephalus in the hydrocephalic Texas rat. J. Cerebral Blood Flow Metabol. 39, 2061–2073. doi: 10.1177/0271678X18776226
Jiménez, A. J., Tomé, M., Páez, P., Wagner, C., Rodríguez, S., Fernández-Llebrez, P., et al. (2001). A programmed ependymal denudation precedes congenital hydrocephalus in the hyh mutant mouse. J. Neuropathol. Exp. Neurol. 60, 1105–1119. doi: 10.1093/jnen/60.11.1105
Jin, S. C., Dong, W., Kundishora, A. J., Panchagnula, S., Moreno-De-Luca, A., Furey, C. G., et al. (2020). Exome sequencing implicates genetic disruption of prenatal neuro-gliogenesis in sporadic congenital hydrocephalus. Nat. Med. 26, 1754–1765. doi: 10.1038/s41591-020-1090-2
Johnsen, L., Friis, K. A., and Damkier, H. H. (2023). In vitro investigation of the effect of proinflammatory cytokines on mouse choroid plexus membrane transporters Ncbe and NKCC1. Fluids Barr CNS 20:71. doi: 10.1186/s12987-023-00474-9
Jones, H. C., Carter, B. J., and Morel, L. (2003). Characteristics of hydrocephalus expression in the LEW/Jms rat strain with inherited disease. Childs Nerv. Syst. 19, 11–18. doi: 10.1007/s00381-002-0671-3
Jones, H. C., Yehia, B., Chen, G. F., and Carter, B. J. (2004). Genetic analysis of inherited hydrocephalus in a rat model. Exp. Neurol. 190, 79–90. doi: 10.1016/j.expneurol.2004.06.019
Jouet, M., Rosenthal, A., MacFarlane, J., Kenwrick, S., and Donnai, D. (1993). A missense mutation confirms the L1 defect in X-linked hydrocephalus (HSAS). Nat. Genet. 4:331. doi: 10.1038/ng0893-331
Kahle, K. T., Kulkarni, A. V., Limbrick, D. D. Jr., and Warf, B. C. (2016). Hydrocephalus in children. Lancet 387, 788–799. doi: 10.1016/S0140-6736(15)60694-8
Karimy, J. K., Zhang, J., Kurland, D. B., Theriault, B. C., Duran, D., Stokum, J. A., et al. (2017). Inflammation-dependent cerebrospinal fluid hypersecretion by the choroid plexus epithelium in posthemorrhagic hydrocephalus. Nat. Med. 23, 997–1003. doi: 10.1038/nm.4361
Kibar, Z., Salem, S., Bosoi, C. M., Pauwels, E., De Marco, P., Merello, E., et al. (2011). Contribution of VANGL2 mutations to isolated neural tube defects. Clin. Genet. 80, 76–82. doi: 10.1111/j.1399-0004.2010.01515.x
Kitchen, P., Salman, M. M., Halsey, A. M., Clarke-Bland, C., MacDonald, J. A., Ishida, H., et al. (2020). Targeting Aquaporin-4 subcellular localization to treat central nervous system edema. Cell 181, 784–799.e19. doi: 10.1016/j.cell.2020.03.037
Kousi, M., and Katsanis, N. (2016). The genetic basis of hydrocephalus. Annu. Rev. Neurosci. 39, 409–435. doi: 10.1146/annurev-neuro-070815-014023
Krebs, D. L., Metcalf, D., Merson, T. D., Voss, A. K., Thomas, T., Zhang, J. G., et al. (2004). Development of hydrocephalus in mice lacking SOCS7. Proc. Natl. Acad. Sci. USA 101, 15446–15451. doi: 10.1073/pnas.0406870101
Kume, T., Deng, K. Y., Winfrey, V., Gould, D. B., Walter, M. A., and Hogan, B. L. (1998). The forkhead/winged helix gene Mf1 is disrupted in the pleiotropic mouse mutation congenital hydrocephalus. Cell 93, 985–996. doi: 10.1016/S0092-8674(00)81204-0
Kundishora, A. J., Singh, A. K., Allington, G., Duy, P. Q., Ryou, J., Alper, S. L., et al. (2021). Genomics of human congenital hydrocephalus. Childs Nerv. Syst. 37, 3325–3340. doi: 10.1007/s00381-021-05230-8
Kuo, L. T., and Huang, A. P. (2021). The pathogenesis of hydrocephalus following aneurysmal subarachnoid hemorrhage. Int. J. Mol. Sci. 22:3. doi: 10.3390/ijms22095050
Lattke, M., Magnutzki, A., Walther, P., Wirth, T., and Baumann, B. (2012). Nuclear factor kappaB activation impairs ependymal ciliogenesis and links neuroinflammation to hydrocephalus formation. J. Neurosci. 32, 11511–11523. doi: 10.1523/JNEUROSCI.0182-12.2012
Lawson, R. F., and Raimondi, A. J. (1973). Hydrocephalus-3, a murine mutant: I. Alterations in fine structure of choroid plexus and ependyma. Surg. Neurol. 1, 115–128
Lechtreck, K. F., Delmotte, P., Robinson, M. L., Sanderson, M. J., and Witman, G. B. (2008). Mutations in Hydin impair ciliary motility in mice. J. Cell Biol. 180, 633–643. doi: 10.1083/jcb.200710162
Lee, P., Monaco, E. A. 3rd, and Friedlander, R. M. (2013). Blocking TGF-beta activity and associated inflammation may halt hydrocephalus. Neurosurgery 73, N13–N14. doi: 10.1227/01.neu.0000438332.72566.f5
Li, X., Kong, H., Wu, W., Xiao, M., Sun, X., and Hu, G. (2009). Aquaporin-4 maintains ependymal integrity in adult mice. Neuroscience 162, 67–77. doi: 10.1016/j.neuroscience.2009.04.044
Li, X., Miyajima, M., and Arai, H. (2005). Analysis of TGF-beta2 and TGF-beta3 expression in the hydrocephalic H-Tx rat brain. Childs Nerv. Syst. 21, 32–38. doi: 10.1007/s00381-004-1034-z
Li, X., Padhan, N., Sjöström, E. O., Roche, F. P., Testini, C., Honkura, N., et al. (2016). VEGFR2 pY949 signalling regulates adherens junction integrity and metastatic spread. Nat. Commun. 7:11017. doi: 10.1038/ncomms11017
Li, P., Zhao, G., Chen, F., Ding, Y., Wang, T., Liu, S., et al. (2020). Rh-relaxin-2 attenuates degranulation of mast cells by inhibiting NF-kappaB through PI3K-AKT/TNFAIP3 pathway in an experimental germinal matrix hemorrhage rat model. J. Neuroinflammation 17:250. doi: 10.1186/s12974-020-01926-x
Lian, G., Chenn, A., Ekuta, V., Kanaujia, S., and Sheen, V. (2019). Formin 2 regulates lysosomal degradation of Wnt-associated β-catenin in neural progenitors. Cerebral Cortex 29, 1938–1952. doi: 10.1093/cercor/bhy073
Liao, F., Li, G., Yuan, W., Chen, Y., Zuo, Y., Rashid, K., et al. (2016). LSKL peptide alleviates subarachnoid fibrosis and hydrocephalus by inhibiting TSP1-mediated TGF-β1 signaling activity following subarachnoid hemorrhage in rats. Exp. Ther. Med. 12, 2537–2543. doi: 10.3892/etm.2016.3640
Lin, T., Ding, L., Lin, Y., Liu, C., Wang, C., Wu, D., et al. (2022). Pharmacological inhibition of TLR4-NF-kappaB signaling by TAK-242 attenuates hydrocephalus after intraventricular hemorrhage. Int. Immunopharmacol. 103:108486. doi: 10.1016/j.intimp.2021.108486
Lin, X., Liu, B., Yang, X., Yue, X., Diao, L., Wang, J., et al. (2013). Genetic deletion of Rnd3 results in aqueductal stenosis leading to hydrocephalus through up-regulation of notch signaling. Proc. Natl. Acad. Sci. USA 110, 8236–8241. doi: 10.1073/pnas.1219995110
Lindeman, G. J., Dagnino, L., Gaubatz, S., Xu, Y., Bronson, R. T., Warren, H. B., et al. (1998). A specific, nonproliferative role for E2F-5 in choroid plexus function revealed by gene targeting. Genes Dev. 12, 1092–1098. doi: 10.1101/gad.12.8.1092
Linneberg, C., Toft, C. L. F., Kjaer-Sorensen, K., and Laursen, L. S. (2019). L1cam-mediated developmental processes of the nervous system are differentially regulated by proteolytic processing. Sci. Rep. 9:3716. doi: 10.1038/s41598-019-39884-x
Liu, Q., Novak, M. K., Pepin, R. M., Maschhoff, K. R., and Hu, W. (2023). Different congenital hydrocephalus-associated mutations in Trim71 impair stem cell differentiation via distinct gain-of-function mechanisms. PLoS Biol. 21:e3001947. doi: 10.1371/journal.pbio.3001947
Liu, Q., Novak, M. K., Pepin, R. M., Maschhoff, K. R., Worner, K., Chen, X., et al. (2023). A congenital hydrocephalus-causing mutation in Trim71 induces stem cell defects via inhibiting Lsd1 mRNA translation. EMBO Rep. 24:e55843. doi: 10.15252/embr.202255843
Liu, X. Y., Song, X., Czosnyka, M., Robba, C., Czosnyka, Z., Summers, J. L., et al. (2024). Congenital hydrocephalus: a review of recent advances in genetic etiology and molecular mechanisms. Mil. Med. Res. 11:54. doi: 10.1186/s40779-024-00560-5
Lolansen, S. D., Rostgaard, N., Andreassen, S. N., Simonsen, A. H., Juhler, M., Hasselbalch, S. G., et al. (2021). Elevated CSF inflammatory markers in patients with idiopathic normal pressure hydrocephalus do not promote NKCC1 hyperactivity in rat choroid plexus. Fluids Barr. CNS. 18:54. doi: 10.1186/s12987-021-00289-6
Lolansen, S. D., Rostgaard, N., Barbuskaite, D., Capion, T., Olsen, M. H., Norager, N. H., et al. (2022). Posthemorrhagic hydrocephalus associates with elevated inflammation and CSF hypersecretion via activation of choroidal transporters. Fluids Barriers CNS 19:62. doi: 10.1186/s12987-022-00360-w
Lolansen, S. D., Rostgaard, N., Oernbo, E. K., Juhler, M., Simonsen, A. H., and MacAulay, N. (2021). Inflammatory markers in cerebrospinal fluid from patients with hydrocephalus: a systematic literature review. Dis. Markers 2021, 1–12. doi: 10.1155/2021/8834822
Loscher, W., and Kaila, K. (2022). CNS pharmacology of NKCC1 inhibitors. Neuropharmacology 205:108910. doi: 10.1016/j.neuropharm.2021.108910
Luo, K. (2017). Signaling cross talk between TGF-β/Smad and other signaling pathways. Cold Spring Harb. Perspect. Biol. 9, 15–7. doi: 10.1101/cshperspect.a022137
Luo, R., Jeong, S. J., Jin, Z., Strokes, N., Li, S., and Piao, X. (2011). G protein-coupled receptor 56 and collagen III, a receptor-ligand pair, regulates cortical development and lamination. Proc. Natl. Acad. Sci. USA 108, 12925–12930. doi: 10.1073/pnas.1104821108
Lyons, P. J., Sapio, M. R., and Fricker, L. D. (2013). Zebrafish cytosolic carboxypeptidases 1 and 5 are essential for embryonic development. J. Biol. Chem. 288, 30454–30462. doi: 10.1074/jbc.M113.497933
Makiyama, Y., Shoji, S., and Mizusawa, H. (1997). Hydrocephalus in the Otx2+/− mutant mouse. Exp. Neurol. 148, 215–221. doi: 10.1006/exnr.1997.6638
Mao, X., Enno, T. L., and Del Bigio, M. R. (2006). Aquaporin 4 changes in rat brain with severe hydrocephalus. Eur. J. Neurosci. 23, 2929–2936. doi: 10.1111/j.1460-9568.2006.04829.x
Marguet, F., Vezain, M., Marcorelles, P., Audebert-Bellanger, S., Cassinari, K., Drouot, N., et al. (2021). Neuropathological hallmarks of fetal hydrocephalus linked to CCDC88C pathogenic variants. Acta Neuropathol. Commun. 9:104. doi: 10.1186/s40478-021-01207-5
Markham, N. O., Doll, C. A., Dohn, M. R., Miller, R. K., Yu, H., Coffey, R. J., et al. (2014). DIPA-family coiled-coils bind conserved isoform-specific head domain of p120-catenin family: potential roles in hydrocephalus and heterotopia. Mol. Biol. Cell 25, 2592–2603. doi: 10.1091/mbc.e13-08-0492
Mashimo, T., Hadjebi, O., Amair-Pinedo, F., Tsurumi, T., Langa, F., Serikawa, T., et al. (2009). Progressive Purkinje cell degeneration in tambaleante mutant mice is a consequence of a missense mutation in HERC1 E3 ubiquitin ligase. PLoS Genet. 5:e1000784. doi: 10.1371/journal.pgen.1000784
Mastromoro, G., De Luca, A., Marchionni, E., Spagnuolo, A., Ventriglia, F., Manganaro, L., et al. (2021). External hydrocephalus as a prenatal feature of Noonan syndrome. Ann. Hum. Genet. 85, 249–252. doi: 10.1111/ahg.12436
Mekbib, K. Y., Muñoz, W., Allington, G., McGee, S., Mehta, N. H., Shofi, J. P., et al. (2023). Human genetics and molecular genomics of Chiari malformation type 1. Trends Mol. Med. 29, 1059–1075. doi: 10.1016/j.molmed.2023.08.013
Meng, H., Li, F., Hu, R., Yuan, Y., Gong, G., Hu, S., et al. (2015). Deferoxamine alleviates chronic hydrocephalus after intraventricular hemorrhage through iron chelation and Wnt1/Wnt3a inhibition. Brain Res. 1602, 44–52. doi: 10.1016/j.brainres.2014.08.039
Miller, J. M., Kumar, R., McAllister, J. P. 2nd, and Krause, G. S. (2006). Gene expression analysis of the development of congenital hydrocephalus in the H-Tx rat. Brain Res. 1075, 36–47. doi: 10.1016/j.brainres.2005.12.094
Mogi, K., Adachi, T., Izumi, S., and Toyoizumi, R. (2012). Visualisation of cerebrospinal fluid flow patterns in albino Xenopus larvae in vivo. Fluids Barr. CNS. 9:9. doi: 10.1186/2045-8118-9-9
Mori, N., Kuwamura, M., Tanaka, N., Hirano, R., Nabe, M., Ibuki, M., et al. (2012). Ccdc85c encoding a protein at apical junctions of radial glia is disrupted in hemorrhagic hydrocephalus (hhy) mice. Am. J. Pathol. 180, 314–327. doi: 10.1016/j.ajpath.2011.09.014
Naureen, I., Waheed, K. A., Rathore, A. W., Victor, S., Mallucci, C., Goodden, J. R., et al. (2014). Fingerprint changes in CSF composition associated with different aetiologies in human neonatal hydrocephalus: inflammatory cytokines. Childs Nerv. Syst. 30, 1155–1164. doi: 10.1007/s00381-014-2415-6
Naz, N., Jimenez, A. R., Sanjuan-Vilaplana, A., Gurney, M., and Miyan, J. (2016). Neonatal hydrocephalus is a result of a block in folate handling and metabolism involving 10-formyltetrahydrofolate dehydrogenase. J. Neurochem. 138, 610–623. doi: 10.1111/jnc.13686
Noelanders, R., and Vleminckx, K. (2017). How Wnt signaling builds the brain: bridging development and disease. Neuroscientist 23, 314–329. doi: 10.1177/1073858416667270
Nusse, R., and Clevers, H. (2017). Wnt/beta-catenin signaling, disease, and emerging therapeutic modalities. Cell 169, 985–999. doi: 10.1016/j.cell.2017.05.016
Ohata, S., Nakatani, J., Herranz-Pérez, V., Cheng, J., Belinson, H., Inubushi, T., et al. (2014). Loss of Dishevelleds disrupts planar polarity in ependymal motile cilia and results in hydrocephalus. Neuron 83, 558–571. doi: 10.1016/j.neuron.2014.06.022
Ohmiya, M., Fukumitsu, H., Nitta, A., Nomoto, H., Furukawa, Y., and Furukawa, S. (2001). Administration of FGF-2 to embryonic mouse brain induces hydrocephalic brain morphology and aberrant differentiation of neurons in the postnatal cerebral cortex. J. Neurosci. Res. 65, 228–235. doi: 10.1002/jnr.1146
Oi, S., Yamada, H., Sato, O., and Matsumoto, S. (1996). Experimental models of congenital hydrocephalus and comparable clinical problems in the fetal and neonatal periods. Child. Nerv. Syst. 12, 292–302. doi: 10.1007/BF00301016
Okamoto, N., Del Maestro, R., Valero, R., Monros, E., Poo, P., Kanemura, Y., et al. (2004). Hydrocephalus and Hirschsprung's disease with a mutation of L1CAM. J. Hum. Genet. 49, 334–337. doi: 10.1007/s10038-004-0153-4
Ortloff, A. R., Vio, K., Guerra, M., Jaramillo, K., Kaehne, T., Jones, H., et al. (2013). Role of the subcommissural organ in the pathogenesis of congenital hydrocephalus in the HTx rat. Cell Tissue Res. 352, 707–725. doi: 10.1007/s00441-013-1615-9
Páez, P., Bátiz, L. F., Roales-Buján, R., Rodríguez-Pérez, L. M., Rodríguez, S., Jiménez, A. J., et al. (2007). Patterned neuropathologic events occurring in hyh congenital hydrocephalic mutant mice. J. Neuropathol. Exp. Neurol. 66, 1082–1092. doi: 10.1097/nen.0b013e31815c1952
Pathak, N., Austin-Tse, C. A., Liu, Y., Vasilyev, A., and Drummond, I. A. (2014). Cytoplasmic carboxypeptidase 5 regulates tubulin glutamylation and zebrafish cilia formation and function. Mol. Biol. Cell 25, 1836–1844. doi: 10.1091/mbc.e13-01-0033
Paul, L., Madan, M., Rammling, M., Chigurupati, S., Chan, S. L., and Pattisapu, J. V. (2011). Expression of aquaporin 1 and 4 in a congenital hydrocephalus rat model. Neurosurgery 68, 462–473. doi: 10.1227/NEU.0b013e3182011860
Peng, D., Fu, M., Wang, M., Wei, Y., and Wei, X. (2022). Targeting TGF-beta signal transduction for fibrosis and cancer therapy. Mol. Cancer 21:104. doi: 10.1186/s12943-022-01569-x
Pietrzik, K., Bailey, L., and Shane, B. (2010). Folic acid and L-5-methyltetrahydrofolate: comparison of clinical pharmacokinetics and pharmacodynamics. Clin. Pharmacokinet. 49, 535–548. doi: 10.2165/11532990-000000000-00000
Putoux, A., Thomas, S., Coene, K. L., Davis, E. E., Alanay, Y., Ogur, G., et al. (2011). KIF7 mutations cause fetal hydrolethalus and acrocallosal syndromes. Nat. Genet. 43, 601–606. doi: 10.1038/ng.826
Rahimi, N. (2017). Defenders and challengers of endothelial barrier function. Front. Immunol. 8:1847. doi: 10.3389/fimmu.2017.01847
Raimondi, A. J., Clark, S. J., and McLone, D. G. (1976). Pathogenesis of aqueductal occlusion in congenital murine hydrocephalus. J. Neurosurg. 45, 66–77. doi: 10.3171/jns.1976.45.1.0066
Rekate, H. L. (2009). A contemporary definition and classification of hydrocephalus. Semin. Pediatr. Neurol. 16, 9–15. doi: 10.1016/j.spen.2009.01.002
Robinson, M. L., Allen, C. E., Davy, B. E., Durfee, W. J., Elder, F. F., Elliott, C. S., et al. (2002). Genetic mapping of an insertional hydrocephalus-inducing mutation allelic to hy3. Mamm. Genome 13, 625–632. doi: 10.1007/s00335-002-2201-8
Robinson, S., and Jantzie, L. L. (2022). Pathogenesis of posthemorrhagic hydrocephalus of prematurity: new horizons. Semin. Perinatol. 46:151596. doi: 10.1016/j.semperi.2022.151596
Rodríguez-Pérez, L. M., López-de-San-Sebastián, J., de Diego, I., Smith, A., Roales-Buján, R., Jiménez, A. J., et al. (2024). A selective defect in the glial wedge as part of the neuroepithelium disruption in hydrocephalus development in the mouse hyh model is associated with complete corpus callosum dysgenesis. Front. Cell. Neurosci. 18:1330412. doi: 10.3389/fncel.2024.1330412
Sadegh, C., Xu, H., Sutin, J., Fatou, B., Gupta, S., Pragana, A., et al. (2023). Choroid plexus-targeted NKCC1 overexpression to treat post-hemorrhagic hydrocephalus. Neuron 111, 1591–1608.e4. doi: 10.1016/j.neuron.2023.02.020
Saillour, Y., Zanni, G., Des Portes, V., Heron, D., Guibaud, L., Iba-Zizen, M. T., et al. (2007). Mutations in the AP1S2 gene encoding the sigma 2 subunit of the adaptor protein 1 complex are associated with syndromic X-linked mental retardation with hydrocephalus and calcifications in basal ganglia. J. Med. Genet. 44, 739–744. doi: 10.1136/jmg.2007.051334
Sapiro, R., Kostetskii, I., Olds-Clarke, P., Gerton, G. L., Radice, G. L., and Strauss, I. J. (2002). Male infertility, impaired sperm motility, and hydrocephalus in mice deficient in sperm-associated antigen 6. Mol. Cell. Biol. 22, 6298–6305. doi: 10.1128/MCB.22.17.6298-6305.2002
Sasaki, S., Goto, H., Nagano, H., Furuya, K., Omata, Y., Kanazawa, K., et al. (1983). Congenital hydrocephalus revealed in the inbred rat, LEW/Jms. Neurosurgery 13, 548–554. doi: 10.1227/00006123-198311000-00011
Sasaki, K., Shiba, K., Nakamura, A., Kawano, N., Satouh, Y., Yamaguchi, H., et al. (2019). Calaxin is required for cilia-driven determination of vertebrate laterality. Commun. Biol. 2:226. doi: 10.1038/s42003-019-0462-y
Schmidt, M. J., Rummel, C., Hauer, J., Kolecka, M., Ondreka, N., McClure, V., et al. (2016). Increased CSF aquaporin-4, and interleukin-6 levels in dogs with idiopathic communicating internal hydrocephalus and a decrease after ventriculo-peritoneal shunting. Fluids Barr. CNS 13:12. doi: 10.1186/s12987-016-0034-1
Schueler, M., Braun, D. A., Chandrasekar, G., Gee, H. Y., Klasson, T. D., Halbritter, J., et al. (2015). DCDC2 mutations cause a renal-hepatic ciliopathy by disrupting Wnt signaling. Am. J. Hum. Genet. 96, 81–92. doi: 10.1016/j.ajhg.2014.12.002
Scott, C. E., Wynn, S. L., Sesay, A., Cruz, C., Cheung, M., Gomez Gaviro, M. V., et al. (2010). SOX9 induces and maintains neural stem cells. Nat. Neurosci. 13, 1181–1189. doi: 10.1038/nn.2646
Shen, X. Q., Miyajima, M., Ogino, I., and Arai, H. (2006). Expression of the water-channel protein aquaporin 4 in the H-Tx rat: possible compensatory role in spontaneously arrested hydrocephalus. J. Neurosurg. 105, 459–464. doi: 10.3171/ped.2006.105.6.459
Shen, D., Ye, X., Li, J., Hao, X., Jin, L., Jin, Y., et al. (2022). Metformin preserves VE-cadherin in choroid plexus and attenuates hydrocephalus via VEGF/VEGFR2/p-Src in an intraventricular hemorrhage rat model. Int. J. Mol. Sci. 23:8552. doi: 10.3390/ijms23158552
Shim, J. W., and Madsen, J. R. (2018). VEGF signaling in neurological disorders. Int. J. Mol. Sci. 19:1. doi: 10.3390/ijms19010275
Shim, J. W., Sandlund, J., Hameed, M. Q., Blazer-Yost, B., Zhou, F. C., Klagsbrun, M., et al. (2016). Excess HB-EGF, which promotes VEGF signaling, leads to hydrocephalus. Sci. Rep. 6:26794. doi: 10.1038/srep26794
Shim, J. W., Sandlund, J., Han, C. H., Hameed, M. Q., Connors, S., Klagsbrun, M., et al. (2013). VEGF, which is elevated in the CSF of patients with hydrocephalus, causes ventriculomegaly and ependymal changes in rats. Exp. Neurol. 247, 703–709. doi: 10.1016/j.expneurol.2013.03.011
Sidhaye, V. K., Chau, E., Srivastava, V., Sirimalle, S., Balabhadrapatruni, C., Aggarwal, N. R., et al. (2012). A novel role for aquaporin-5 in enhancing microtubule organization and stability. PLoS One 7:e38717. doi: 10.1371/journal.pone.0038717
Sidhaye, V. K., Schweitzer, K. S., Caterina, M. J., Shimoda, L., and King, L. S. (2008). Shear stress regulates aquaporin-5 and airway epithelial barrier function. Proc. Natl. Acad. Sci. USA 105, 3345–3350. doi: 10.1073/pnas.0712287105
Simard, J. M., Kahle, K. T., and Gerzanich, V. (2010). Molecular mechanisms of microvascular failure in central nervous system injury--synergistic roles of NKCC1 and SUR1/TRPM4. J. Neurosurg. 113, 622–629. doi: 10.3171/2009.11.JNS081052
Simard, P. F., Tosun, C., Melnichenko, L., Ivanova, S., Gerzanich, V., and Simard, J. M. (2011). Inflammation of the choroid plexus and ependymal layer of the ventricle following intraventricular hemorrhage. Transl. Stroke Res. 2, 227–231. doi: 10.1007/s12975-011-0070-8
Singh, A.K., Allington, G., Viviano, S., McGee, S., Kiziltug, E., and Ma, S. A novel SMARCC1 -mutant BAFopathy implicates epigenetic dysregulation of neural progenitors in hydrocephalus. Brain. (2023). 147, 1553–70. doi: 10.1093/brain/awad405
Somera, K. C., and Jones, H. (2002). Subcommissural organ dysfunction in H-Tx rats with early-onset hydrocephalus. European journal of pediatric surgery: official journal of Austrian Association of Pediatric Surgery [et al]. Z. Kinderchir. 12, S45–S47.
Somera, K. C., and Jones, H. C. (2004). Reduced subcommissural organ glycoprotein immunoreactivity precedes aqueduct closure and ventricular dilatation in H-Tx rat hydrocephalus. Cell Tissue Res. 315, 361–373. doi: 10.1007/s00441-003-0843-9
Song, S., Huang, H., Guan, X., Fiesler, V., Bhuiyan, M. I. H., Liu, R., et al. (2021). Activation of endothelial Wnt/beta-catenin signaling by protective astrocytes repairs BBB damage in ischemic stroke. Prog. Neurobiol. 199:101963. doi: 10.1016/j.pneurobio.2020.101963
Sotak, B. N., and Gleeson, J. G. (2012). Can't get there from here: cilia and hydrocephalus. Nat. Med. 18, 1742–1743. doi: 10.1038/nm.3011
Suryaningtyas, W., Parenrengi, M. A., Bajamal, A. H., and Rantam, F. A. (2020). Lipid peroxidation induces reactive Astrogliosis by activating WNT/beta-catenin pathway in hydrocephalus. Malays. J. Med. Sci. 27, 34–42. doi: 10.21315/mjms2020.27.3.4
Sveinsdottir, S., Gram, M., Cinthio, M., Sveinsdottir, K., Morgelin, M., and Ley, D. (2014). Altered expression of aquaporin 1 and 5 in the choroid plexus following preterm intraventricular hemorrhage. Dev. Neurosci. 36, 542–551. doi: 10.1159/000366058
Teng, Y., Xie, X., Walker, S., Saxena, M., Kozlowski, D. J., Mumm, J. S., et al. (2011). Loss of zebrafish lgi1b leads to hydrocephalus and sensitization to pentylenetetrazol induced seizure-like behavior. PLoS One 6:e24596. doi: 10.1371/journal.pone.0024596
Terman, B. I., Dougher-Vermazen, M., Carrion, M. E., Dimitrov, D., Armellino, D. C., Gospodarowicz, D., et al. (1992). Identification of the KDR tyrosine kinase as a receptor for vascular endothelial cell growth factor. Biochem. Biophys. Res. Commun. 187, 1579–1586. doi: 10.1016/0006-291X(92)90483-2
Tessier, A., Roux, N., Boutaud, L., Lunel, E., Hakkakian, L., Parisot, M., et al. (2023). Bi-allelic variations in CRB2, encoding the crumbs cell polarity complex component 2, lead to non-communicating hydrocephalus due to atresia of the aqueduct of sylvius and central canal of the medulla. Acta Neuropathol. Commun. 11:29. doi: 10.1186/s40478-023-01519-8
Toader, C., Tataru, C. P., Florian, I. A., Covache-Busuioc, R. A., Dumitrascu, D. I., Glavan, L. A., et al. (2023). From homeostasis to pathology: decoding the multifaceted impact of Aquaporins in the central nervous system. Int. J. Mol. Sci. 24:1. doi: 10.3390/ijms241814340
Toft-Bertelsen, T. L., Barbuskaite, D., Heerfordt, E. K., Lolansen, S. D., Andreassen, S. N., Rostgaard, N., et al. (2022). Lysophosphatidic acid as a CSF lipid in posthemorrhagic hydrocephalus that drives CSF accumulation via TRPV4-induced hyperactivation of NKCC1. Fluids Barriers CNS 19:69. doi: 10.1186/s12987-022-00361-9
Tourdias, T., Dragonu, I., Fushimi, Y., Deloire, M. S., Boiziau, C., Brochet, B., et al. (2009). Aquaporin 4 correlates with apparent diffusion coefficient and hydrocephalus severity in the rat brain: a combined MRI-histological study. NeuroImage 47, 659–666. doi: 10.1016/j.neuroimage.2009.04.070
Tsitouras, V., and Sgouros, S. (2011). Infantile posthemorrhagic hydrocephalus. Childs Nerv. Syst. 27, 1595–1608. doi: 10.1007/s00381-011-1521-y
Tubbs, R. S., Smyth, M. D., Wellons, J. C. 3rd, Blount, J. P., Grabb, P. A., and Oakes, W. J. (2003). Alternative uses for the subgaleal shunt in pediatric neurosurgery. Pediatr. Neurosurg. 39, 22–24. doi: 10.1159/000070875
Tuli, S., Drake, J., Lawless, J., Wigg, M., and Lamberti-Pasculli, M. (2000). Risk factors for repeated cerebrospinal shunt failures in pediatric patients with hydrocephalus. J. Neurosurg. 92, 31–38. doi: 10.3171/jns.2000.92.1.0031
Tzavlaki, K., and Moustakas, A. (2020). TGF-β Signaling. Biomolecules 10:3. doi: 10.3390/biom10030487
Wagner, C., Batiz, L. F., Rodríguez, S., Jiménez, A. J., Páez, P., Tomé, M., et al. (2003). Cellular mechanisms involved in the stenosis and obliteration of the cerebral aqueduct of hyh mutant mice developing congenital hydrocephalus. J. Neuropathol. Exp. Neurol. 62, 1019–1040. doi: 10.1093/jnen/62.10.1019
Wallmeier, J., Frank, D., Shoemark, A., Nöthe-Menchen, T., Cindric, S., Olbrich, H., et al. (2019). De novo mutations in FOXJ1 result in a motile ciliopathy with hydrocephalus and randomization of left/right body asymmetry. Am. J. Hum. Genet. 105, 1030–1039. doi: 10.1016/j.ajhg.2019.09.022
Wang, R., Chen, H., Wang, X., Huang, S., Xie, A., and Wu, X. (2021). Prenatal diagnosis of a nonsense mutation in the L1CAM gene resulting in congenital hydrocephalus: a case report and literature review. Exp. Ther. Med. 22:1416. doi: 10.3892/etm.2021.10807
Wang, X., Li, F., Xie, L., Crane, J., Zhen, G., Mishina, Y., et al. (2018). Inhibition of overactive TGF-beta attenuates progression of heterotopic ossification in mice. Nat. Commun. 9:551. doi: 10.1038/s41467-018-02988-5
Wang, Q., Liu, F., Li, Y., Zhang, H., Qi, X., Wu, K., et al. (2024). Choroid plexus CCL2–CCR2 signaling orchestrates macrophage recruitment and cerebrospinal fluid hypersecretion in hydrocephalus. Acta Pharm. Sin. B 14, 4544–4559. doi: 10.1016/j.apsb.2024.06.020
Wang, D., Nykanen, M., Yang, N., Winlaw, D., North, K., Verkman, A. S., et al. (2011). Altered cellular localization of aquaporin-1 in experimental hydrocephalus in mice and reduced ventriculomegaly in aquaporin-1 deficiency. Mol. Cell. Neurosci. 46, 318–324. doi: 10.1016/j.mcn.2010.10.003
Wang, K., Tang, Z., Yang, Y., Guo, Y., Liu, Z., Su, Z., et al. (2024). Zebrafish as a model organism for congenital hydrocephalus: characteristics and insights. Zebrafish 21, 361–384. doi: 10.1089/zeb.2024.0148
Whitelaw, A., Christie, S., and Pople, I. (1999). Transforming growth factor-beta1: a possible signal molecule for posthemorrhagic hydrocephalus? Pediatr. Res. 46, 576–580. doi: 10.1203/00006450-199911000-00014
Willems, P. J., Brouwer OFDijkstra, I., and Wilmink, J. (1987). X-linked hydrocephalus. Am. J. Med. Genet. 27, 921–928. doi: 10.1002/ajmg.1320270419
Xiao, K., Garner, J., Buckley, K. M., Vincent, P. A., Chiasson, C. M., Dejana, E., et al. (2005). p120-catenin regulates clathrin-dependent endocytosis of VE-cadherin. Mol. Biol. Cell 16, 5141–5151. doi: 10.1091/mbc.e05-05-0440
Xu, H. (2016). New concept of the pathogenesis and therapeutic orientation of acquired communicating hydrocephalus. Neurol. Sci. 37, 1387–1391. doi: 10.1007/s10072-016-2589-7
Xu, H., Fame, R. M., Sadegh, C., Sutin, J., Naranjo, C., Della, S., et al. (2021). Choroid plexus NKCC1 mediates cerebrospinal fluid clearance during mouse early postnatal development. Nat. Commun. 12:447. doi: 10.1038/s41467-020-20666-3
Xu, H., He, J., Du, H., Jing, X., and Liu, X. (2024). Evaluation of the choroid plexus epithelium inflammation TLR4/NF-κB/NKCC1 signal pathway activation in the development of hydrocephalus. CNS Neurosci. Ther. 30:e70085. doi: 10.1111/cns.70085
Xu, H., Miyajima, M., Nakajima, M., Ogino, I., Kawamura, K., Akiba, C., et al. (2022). Ptpn20 deletion in H-Tx rats enhances phosphorylation of the NKCC1 cotransporter in the choroid plexus: an evidence of genetic risk for hydrocephalus in an experimental study. Fluids Barr. CNS. 19:39. doi: 10.1186/s12987-022-00341-z
Xu, H., Xu, B., Wang, Z., Tan, G., and Shen, S. (2015). Inhibition of Wnt/beta-catenin signal is alleviated reactive gliosis in rats with hydrocephalus. Childs Nerv. Syst. 31, 227–234. doi: 10.1007/s00381-014-2613-2
Yamashiro, S. (2022). Treatment of secondary hydrocephalus after subarachnoid hemorrhage. No shinkei geka Neurol. Surg. 50, 411–418. doi: 10.11477/mf.1436204568
Yan, H., Chen, Y., Li, L., Jiang, J., Wu, G., Zuo, Y., et al. (2016). Decorin alleviated chronic hydrocephalus via inhibiting TGF-beta1/Smad/CTGF pathway after subarachnoid hemorrhage in rats. Brain Res. 1630, 241–253. doi: 10.1016/j.brainres.2015.11.004
Yang, J., Dombrowski, S. M., Deshpande, A., Krajcir, N., and Luciano, M. G. (2010). VEGF/VEGFR-2 changes in frontal cortex, choroid plexus, and CSF after chronic obstructive hydrocephalus. J. Neurol. Sci. 296, 39–46. doi: 10.1016/j.jns.2010.06.012
Yang, S., Emelyanov, A., You, M. S., Sin, M., and Korzh, V. (2021). Camel regulates development of the brain ventricular system. Cell Tissue Res. 383, 835–852. doi: 10.1007/s00441-020-03270-1
Yang, Y. C., Liu, S. H., Hsu, Y. H., Wu, Y. L., Chu, P. T., and Lin, P. C. (2022). Cerebrospinal fluid predictors of shunt-dependent hydrocephalus after hemorrhagic stroke: a systematic review and meta-analysis. Neurosurg. Rev. 45, 1847–1859. doi: 10.1007/s10143-022-01731-5
Yang, J., Shanahan, K. J., Shriver, L. P., and Luciano, M. G. (2016). Exercise-induced changes of cerebrospinal fluid vascular endothelial growth factor in adult chronic hydrocephalus patients. J. Clin. Neurosci. 24, 52–56. doi: 10.1016/j.jocn.2015.08.019
Yang, J., Simonneau, C., Kilker, R., Oakley, L., Byrne, M. D., Nichtova, Z., et al. (2019). Murine MPDZ-linked hydrocephalus is caused by hyperpermeability of the choroid plexus. EMBO Mol. Med. 11, 13–17. doi: 10.15252/emmm.201809540
Yang, L., Zeng, C., Zhang, Y., Wang, F., Takamiya, M., and Strähle, U. (2019). Functions of thioredoxin1 in brain development and in response to environmental chemicals in zebrafish embryos. Toxicol. Lett. 314, 43–52. doi: 10.1016/j.toxlet.2019.07.009
Yue, X. J., Guo, Y., Yang, H. J., Feng, Z. W., Li, T., and Xu, Y. M. (2016). Transforming growth factor-beta1 induces fibrosis in rat meningeal mesothelial cells via the p38 signaling pathway. Mol. Med. Rep. 14, 1709–1713. doi: 10.3892/mmr.2016.5411
Zanotto, C., Simao, F., Gasparin, M. S., Biasibetti, R., Tortorelli, L. S., Nardin, P., et al. (2017). Exendin-4 reverses biochemical and functional alterations in the blood-brain and blood-CSF barriers in diabetic rats. Mol. Neurobiol. 54, 2154–2166. doi: 10.1007/s12035-016-9798-1
Zechel, J., Gohil, H., Lust, W. D., and Cohen, A. (2002). Alterations in matrix metalloproteinase-9 levels and tissue inhibitor of matrix metalloproteinases-1 expression in a transforming growth factor-beta transgenic model of hydrocephalus. J. Neurosci. Res. 69, 662–668. doi: 10.1002/jnr.10326
Zhang, S., Chen, D., Huang, C., Bao, J., and Wang, Z. (2013). Expression of HGF, MMP-9 and TGF-beta1 in the CSF and cerebral tissue of adult rats with hydrocephalus. Int. J. Neurosci. 123, 392–399. doi: 10.3109/00207454.2012.762363
Zhang, T., Cui, S., Xiong, X., Liu, Y., Cao, Q., Xia, X. G., et al. (2023). PIH1D3-knockout rats exhibit full ciliopathy features and dysfunctional pre-assembly and loading of dynein arms in motile cilia. Front. Cell Dev. Biol. 11:1282787. doi: 10.3389/fcell.2023.1282787
Zhang, W., Ge, Y., Cheng, Q., Zhang, Q., Fang, L., and Zheng, J. (2018). Decorin is a pivotal effector in the extracellular matrix and tumour microenvironment. Oncotarget 9, 5480–5491. doi: 10.18632/oncotarget.23869
Zhang, Z., Tan, Q., Guo, P., Huang, S., Jia, Z., Liu, X., et al. (2022). NLRP3 inflammasome-mediated choroid plexus hypersecretion contributes to hydrocephalus after intraventricular hemorrhage via phosphorylated NKCC1 channels. J. Neuroinflammation 19:163. doi: 10.1186/s12974-022-02530-x
Zhang, J., Williams, M. A., and Rigamonti, D. (2006). Genetics of human hydrocephalus. J. Neurol. 253, 1255–1266. doi: 10.1007/s00415-006-0245-5
Zhao, Z., He, J., Chen, Y., Wang, Y., Wang, C., Tan, C., et al. (2022). The pathogenesis of idiopathic normal pressure hydrocephalus based on the understanding of AQP1 and AQP4. Front. Mol. Neurosci. 15:952036. doi: 10.3389/fnmol.2022.952036
Zhao, H., Ming, T., Tang, S., Ren, S., Yang, H., Liu, M., et al. (2022). Wnt signaling in colorectal cancer: pathogenic role and therapeutic target. Mol. Cancer 21:144. doi: 10.1186/s12943-022-01616-7
Zou, W., Lv, Y., Liu, Z. I., Xia, P., Li, H., and Jiao, J. (2020). Loss of Rsph9 causes neonatal hydrocephalus with abnormal development of motile cilia in mice. Sci. Rep. 10:12435. doi: 10.1038/s41598-020-69447-4
Keywords: hydrocephalus, genetic abnormality, animal model, molecular changes, cerebrospinal fluid
Citation: Deng X, Chen Y, Duan Q, Ding J, Wang Z, Wang J, Chen X, Zhou L and Zhao L (2025) Genetic and molecular mechanisms of hydrocephalus. Front. Mol. Neurosci. 17:1512455. doi: 10.3389/fnmol.2024.1512455
Edited by:
Babak Behnam, National Sanitation Foundation International, United StatesCopyright © 2025 Deng, Chen, Duan, Ding, Wang, Wang, Chen, Zhou and Zhao. This is an open-access article distributed under the terms of the Creative Commons Attribution License (CC BY). The use, distribution or reproduction in other forums is permitted, provided the original author(s) and the copyright owner(s) are credited and that the original publication in this journal is cited, in accordance with accepted academic practice. No use, distribution or reproduction is permitted which does not comply with these terms.
*Correspondence: Liangxue Zhou, bGlhbmd4dWVfemhvdUAxMjYuY29t; Long Zhao, Y2J5emhhb2xvbmdAMTYzLmNvbQ==
†These authors have contributed equally to this work and share first authorship